- 1Faculty of Chinese Medicine, Macau University of Science and Technology, Taipa, Macao SAR, China
- 2Department of Orthopedics, Affiliated Traditional Chinese Medicine Hospital of Southwest Medical University, Luzhou, Sichuan, China
- 3Dr. Neher’s Biophysics Laboratory for Innovative Drug Discovery, State Key Laboratory of Quality Research in Chinese Medicine, Macau University of Science and Technology, Taipa, Macao SAR, China
Injuries at the tendon-bone interface are very common in the field of sports medicine, and healing at the tendon-bone interface is complex. Injuries to the tendon-bone interface can seriously affect a patient’s quality of life, so it is essential to restore stability and promote healing of the tendon-bone interface. In addition to surgical treatment, the healing of tendons and bones can also be properly combined with extracorporeal stimulation therapy during the recovery process. In this review, we discuss the effects of extracorporeal shock waves (ESWs), low-intensity pulsed ultrasound (LIPUS), and mechanical stress on tendon-bone healing, focusing on the possible mechanisms of action of mechanical stress on tendon-bone healing in terms of transcription factors and biomolecules. The aim is to provide possible therapeutic approaches for subsequent clinical treatment.
Introduction
Tendon injury is one of the most common clinical diseases, and the global prevalence of tendon disease has been on the rise (Ding et al., 2021). Tendon-bone insertion injuries are also very common, such as rotator cuff and anterior cruciate ligament (ACL) injuries (Xu et al., 2021). And rotator cuff injury and anterior cruciate ligament injury are common soft tissue injuries in the sports medicine field (Tang et al., 2020). Rotator cuff injury is the main cause of shoulder joint pain and weakness, and it even leads to disability (Chalmers et al., 2020; Salvatore et al., 2020). Surgery is the primary treatment for rotator cuff injuries, resulting in increased medical costs, and postoperative retears remain an open problem (Sgroi and Cilenti, 2018; Longo et al., 2020). The preferred treatment for ACL injuries is ACL reconstruction, but follow-up surveys have found that reinjury after reconstruction occurs in far more patients than most other similar treatments (Ahmed et al., 2017; Grassi et al., 2017). Inadequate tendon-bone healing after ACL reconstruction can lead to a variety of secondary symptoms including knee laxity, instability of motion, meniscal and cartilage damage and even early post-traumatic osteoarthritis (Levine et al., 2013; Musahl et al., 2019). Successful tendon-bone healing is the key to restoring physiological function to these injuries, but it remains a clinical challenge (Lebaschi et al., 2018). Tendon adhesions and poor tendon healing not only result in limited movement and pain but also severely affect the patient’s quality of life and can require a second surgery (Chen S. H. et al., 2019; Liu et al., 2019). The normal tendon-bone junction includes four layers of structure: bone, mineralized fibrocartilage, non-mineralized fibrocartilage and tendon (Shi et al., 2020). As early as 1997, researchers outlined the process of tendon-bone healing in a rabbit model, where inflammatory cells could be observed at the tendon bone interface 1 week after surgery, scar tissue appeared after 2 weeks, scar tissue reorganised to form a dense connective tissue matrix after 4 weeks, and by week 6,sharpey’s fibres could be observed (Liu et al., 1997). The process of tendon-bone healing was later divided into four phases: the inflammatory phase, the proliferative phase, the matrix formation phase and the matrix regeneration phase (Lui et al., 2010). This shows that tendon-bone healing is a complex process Figure 1B. Meanwhile, tendon-bone healing is affected by a variety of factors and is prone to scar tissue formation (Lü et al., 2019), the limited mechanical properties of the scar tissue predispose the tendon to re-injury (Yang et al., 2023). In recent years, researchers have been searching for ways to facilitate the tendon-to-bone healing process. Techniques such as biomaterials (Lacheta and Braun, 2021), platelet-rich plasma (PRP) (Peng et al., 2022), cytokines (Zhu et al., 2022), stem cells (Choi et al., 2022) and exosomes (Li et al., 2022; Zou et al., 2023) have been widely used in studies to promote tendon-to-bone healing with promising results. With the development of modern technology and knowledge in biology, ideas and methods for tendon-bone healing have been provided.
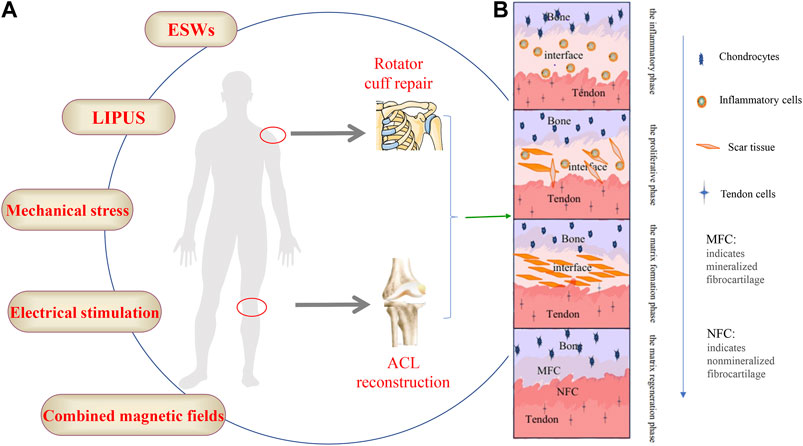
FIGURE 1. (A) The schematic diagram of the external stimulation methods, (B) The four stages of the bone-tendon healing process.
The study of external stimulation on tendon-bone healing is being increasingly emphasized, which is of great interest to sports medicine and orthopaedic clinics. Extracorporeal shock waves (ESWs), low-intensity pulsed ultrasound (LIPUS), mechanical loading and other extracorporeal stimulation have all been shown to have varying degrees of therapeutic effects on tendon-bone healing Figure 1A. In recent years, the role of these in vitro stimuli on tendon-bone healing has gradually been discovered, especially the potential mechanism of mechanical loading; however, there are still many questions to be investigated. In this review, we focus on the effects of ESWs, LIPUS, and mechanical loading on tendon-bone healing, with an emphasis on the possible mechanisms of action of mechanical loading.
The role of different types of stimulation on tendon-bone healing
ESWs
A shock wave is a special, non-linear type of pressure wave with a short rise time (approximately 10 µs) and a frequency ranging from 16 to 20 MHz (Ogden et al., 2001). Currently, extracorporeal shock wave therapy (ESWT) has been shown to have a beneficial effect on tendon healing. For example, ESWT with different parameters in rats with collagenase-induced Achilles tendinitis showed that low-energy ESWT eliminated tendon oedema, swelling and inflammatory cell infiltration, promoted tendon cell proliferation and neovascularization, and promoted transforming growth factor-beta 1 (TGF-β) and insulin-like growth factor-1 (IGF-1) expression during treatment, but high-energy ESWT was not beneficial for tendon repair in rats (Chen et al., 2004) Figure 2A. It can be inferred from this whether ESWT also promotes healing at the tendon bone interface, and it does. In a dose-response study of delayed tendon-bone insertion healing in a rabbit model treated with extracorporeal shockwave, the effect of delayed tendon-bone interface healing was similar in the low-dose ESW and high-dose ESW treatment groups, but did not show evidence of dose dependence, so clinical compliance would have been better (Chow et al., 2012). In another study of partial patellar resection in rabbits to establish a delayed healing model of the patella-patellar tendon complex with a single ESWT treatment, ESWT was found to promote osteogenesis, fibrocartilage regeneration and tissue reconstruction, thereby increasing the effect of tendon-bone repair (Wang et al., 2007). A study using ESWT in rats with osteoarthritis showed that ESWT promotes subchondral bone reconstruction and inhibits cartilage degeneration (Yu et al., 2017). In a study on the improvement of ACL reconstruction by extracorporeal shockwave, tibial tunnel enlargement was significantly reduced in the EWST group and there was reason to believe that ESWT could improve ACL reconstruction through vascular and tissue regeneration (Wang et al., 2014). Additionally, ESWT has an effect on stem cells in vitro, giving better potential for the differentiation of stem cells into lipogenic, osteogenic and fibrogenic cartilage (Raabe et al., 2013). Although the biological role of ESWT has been demonstrated in in vitro studies, the clinical value of ESWT in human tendinopathy awaits further clinical trials. ESWT has been shown to be effective at a certain stage of tendinopathy and may be indicated in the later stages of degenerative tendinopathy where conservative treatment has failed, but ESWT has no effect on the early stages of tendinopathy (Rees et al., 2009; Zwerver et al., 2011) (Specific information on the effect of ESWT on tendon bone healing is given in Table 1). Few studies have reported on the adverse effects of ESWT on tendon bone healing, and the disadvantages of ESWT in the musculoskeletal system are summarized here, most commonly the appearance of transient skin redness, pain and small hematomas, and in severe cases, possible migraine and fainting (Haake et al., 2002). However, the potential of ESWT needs to be further developed.
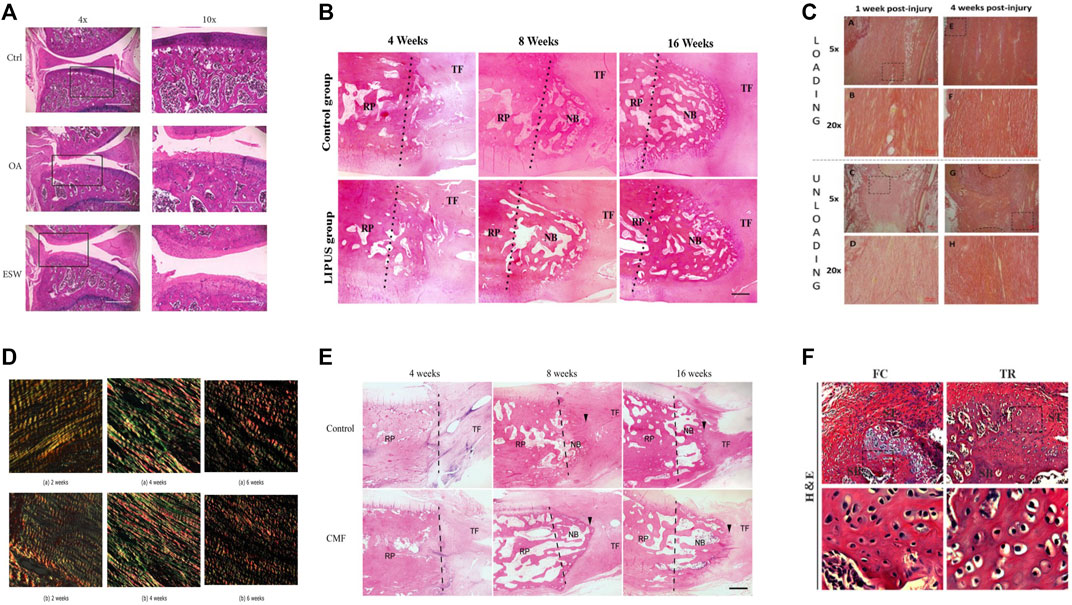
FIGURE 2. The typical histological or photograph images before and after treatment of each method. (A) Before and after ESWT with improved pathology. Reproduced with permission (Yu et al., 2017); (B) Comparative histology before and after LIPUS treatment. New bone formation at the proximal patella was observed in both groups at postoperative weeks 8 and 16. The LIPUS group showed more trabecular and marrow cavities than the control group at postoperative week 16. The dotted line represents the surface of osteotomy (NB, newly formed bone; RP, remaining patella; TF, tendon fiber). Reproduced with permission (Lu et al., 2016); (C) Before and after comparison chart of mechanical loading treatment. Adipocyte were seen within the callus tissue in both groups at 1 week, but it was more common in the loaded tendons, at 4 weeks, there was less adipocytes in the callus in both groups. Reproduced with permission (Khayyeri et al., 2020); (D) Before and after comparison of electrical stimulation treatment. Both collagen 1 and 3 were more highly expressed in the group receiving electrical stimulation than in the control group. Reproduced with permission (Casagrande et al., 2021); (E) Before and after comparison chart of combined magnetic field treatment. NB, newly formed bone; RP, residual patella; TF, tendon fiber; the arrowhead, directed at the regenerated fibrocartilage). The CMF group showed more trabecular bone, more marrow cavities, and more advanced remodeling from woven bone to trabecular lamellar bone than the control group. Reproduced with permission (Hu et al., 2015); (F) Histological diagram before and after exercise. Mice were allowed for free cage activities after surgery (FC group); Mice received treadmill running initiated on postoperative day 7 (TR group); In comparision to FC group, TR group showed better fibrocartilage regeneration which was characterized by more cartilage-like cells and richer proteoglycans accumulation at repaired site at postoperative week 4 and 8. Reproduced with permission (Liu et al., 2022).
LIPUS
LIPUS is a non-destructive modality in which mechanical energy is transmitted to biological tissues as high frequency acoustic pressure waves through the skin (Ying et al., 2012). LIPUS has been shown to have positive effects on muscles, ligaments, tendons and cartilage (Karnes and Burton, 2002; Takakura et al., 2002; Lai et al., 2021). In a rabbit patellar partial resection model treated with LIPUS, an increase in the area of new bone, bone mineral content, and enhanced repair of the patella-patellar tendon junction were observed (Hu et al., 2014). Interestingly, treatment with LIPUS in the early stages of ACL reconstruction demonstrates that LIPUS may promote tendon-bone healing (Ying et al., 2012). In addition, LIPUS accelerates tendon-bone healing by promoting osteogenesis and tissue reconstruction at the healing junction in a standard patellar partial resection model (Lu et al., 2006). LIPUS treatment was shown to have an anti-inflammatory effect on the healing of the bone-tendon junction and histologically, the LIPUS-treated group showed better remodelling of the lamellar bone and bone marrow cavity (Lu et al., 2016) Figure 2B. LIPUS can also promote scar formation and maturation (Jeremias Junior et al., 2011). However, there are still too few studies on LIPUS in humans. There is no sufficient clinical evidence to evaluate the effect on tendon-bone healing (Ying et al., 2012; Lai et al., 2021). Only some animal studies have shown that LIPUS can promote tendon-bone healing at different stages (Lu et al., 2008; Lovric et al., 2013) (Specific information on the effect of LIPUS on tendon-bone healing is given in the Table 1).
There are also many studies on the mechanisms by which LIPUS promotes tendon bone healing. Several animal studies have noted that LIPUS can improve angiogenesis. LIPUS was used to treat the patella-patellar tendon junction, and histologically significantly higher expression of vascular endothelial growth factor (VEGF) was observed in chondrocytes and osteoblasts than in controls (Lu et al., 2008). LIPUS significantly increased nitric oxide (NO) and hypoxia-inducible factor-1α-mediated expression of VEGF-A in human osteoblasts (Wang et al., 2004). LIPUS can enhance tendon bone healing by stimulating osteoblast differentiation and calcium matrix production to promote endochondral osteogenesis (Korstjens et al., 2004). On the other hand, the action of LIPUS on MSCs can increase the expression of type II collagen (Lee et al., 2006), thus promoting the differentiation of mesenchymal stem cells to promote tendon bone healing. A novel finding is that a potential mechanism for LIPUS treatment of tendon-bone interface repair may be macrophage polarization, dependent on phenotypic changes in macrophages (Xu et al., 2022), which may expand the field of clinical trials at a faster rate. Although the LIPUS mechanism has been more intensively studied, it has not yet been confirmed in human studies. Therefore, further clinical trials are recommended to promote early activity and active rehabilitation of patients.
Mechanical stress
Mechanical loading is an important element in soft tissue healing (Candiani et al., 2010; Khoshgoftar et al., 2011). Some studies have shown that in a goat partial patellectomy mode, the use of tensile load to promote bone-tendon healing was found to promote hyaline cartilage transformation into fibrocartilage, thereby accelerating the healing of the bone-tendon junction and the recovery of the fibrocartilage overlap zone (Wong et al., 2003). One experiment investigated the effect of stress shielding on the patellar tendon in a rabbit patellar tendon model using transverse tension and stress relaxation tests. The results indicated that stress shielding significantly decreased the tangent modulus, tensile strength, and strain at failure of collagen fascicles. Stress shielding significantly alters the transverse tensile and viscoelastic properties of the patellar tendon (Yamamoto et al., 2000). In a rat ACL reconstruction model, higher initial graft tension facilitates recovery of knee laxity and promotes graft healing in ACL reconstruction using free tendon grafts (Fu et al., 2013). It has been shown that mechanical loading can positively affect tendon healing by improving the biomechanical and collagen properties of the Achilles tendon earlier in the healing process, but that this effect diminishes over time (Khayyeri et al., 2020) Figure 2C. It is reasonable to speculate that mechanical loading may have the same effect on tendon bone healing, although this is subject to further verification. However, some studies have found that mechanical loads can also have adverse effects. A rat model of chronic rotator cuff tears was used to investigate the effect of repair tension on tendon-bone healing, showing that repair tension increased with detachment time, stimulating an increase in the amount of poor-quality scar tissue produced and thus adversely affecting tendon-bone healing (Gimbel et al., 2007). In conjunction with current research, the reason for these changes may be that increased repair tension may disrupt the insertion site and prevent proper integration of the tendon with the bone, while stimulating cellular activity and producing poor quality scarring, all of which need to be confirmed by further research (Gimbel et al., 2007). A study of repair tension in a rat ACL reconstruction model found that repair tension adversely affects vascular remodelling in grafts (Carbone et al., 2016). Therefore, patients with rotator cuff tears undergo surgery to repair tension due to minimization. Because the potential mechanism of action of repair tension leading to these changes has not yet been identified, it can be further explored in the future. Histological analyses of numerous animal experiments showed that compressive stress promotes cartilage formation and mechanical tension promotes the process of tendon-bone healing; however, shear force has little effect on the regeneration of the tendon-bone interface (Yamakado et al., 2002; Song et al., 2017). There are relatively few studies on the effect of mechanical strength on tendon-bone healing, and a related study using a patellar tendon repair model showed that low-intensity loads had better healing effects than high-intensity loads (Hettrich et al., 2014). In the future, it is possible to study the effects of mechanical loading in terms of time, amplitude and duration on tendon-bone healing (The specific effects of mechanical stress on tendon-bone healing are listed in the Table 1).
Other stimulation
According to previous studies, electrical stimulation, combined magnetic fields and exercise therapy have been shown to have a beneficial effect on tendon bone healing. In an experiment on low-frequency electrical stimulation after Achilles tendon resection and suturing in rats, it was observed histologically that collagen 1 and 3 were higher in the group receiving electrical stimulation than in the control group, so low-frequency electrical stimulation could promote collagen expression and increase the amount of collagen (Casagrande et al., 2021) Figure 2D, so it was further suggested that low-frequency electrical stimulation could promote tendon bone healing by promoting collagen expression. It has been shown that electrical stimulation can promote tissue regeneration by promoting cell proliferation and extracellular matrix production, cytokine secretion and vascular development (Ferrigno et al., 2020). However, very high intensity electrical stimulation can lead to cell death (Chen C. et al., 2019). Studies on combined magnetic fields are also relatively rare. When combined magnetic fields were applied to a rabbit patellar partial resection model, the area, length and bone density of newly formed bone were found to be significantly greater than those of the control group (Hu et al., 2015) Figure 2E. The combined magnetic field has been shown to enhance patello-patellar tendon healing by improving endochondral osteogenesis as well as fibrocartilage regeneration and remodelling (Xu et al., 2014). Similarly, postoperative immobilization and early passive motion in a rotator cuff injury model can protect the tendon bone interface and prevent stiffness, so delaying early passive motion is not harmful to tendon bone healing (Zhang et al., 2013). In a recent study, it was shown that early running exercise can delay rotator cuff healing by inactivating Wnt/β-catenin signalling through increased expression of neuropeptide Y (Chen Y. et al., 2021). This shows that the timing of the start of exercise is very important and needs to be further defined. Running exercise has also been shown to significantly increase IL-4 production, activation of the macrophage JAK/STAT signaling pathway, macrophage polarization, and the quality of tendon bone healing (Liu et al., 2022) Figure 2F. Although all of these treatment options are effective to a certain extent, there are some limitations. Because there are relatively few studies on these in vitro stimulations, optimising the current treatment remains a challenge in the absence of definitive results. (The specific roles are listed in Table 1).
Mechanisms of force-regulated tendon-bone healing
Transcription factors
Transcription factors are proteins that participate in the initiation or regulation of target gene transcription by binding to the corresponding promoter region of the target gene (Nakamichi and Asahara, 2021). An increasing number of studies show that transcription factors are involved in mechanical stress regulation of tendon-bone, tendon and ligament healing processes. Two major tendon-specific transcription factors, Scleraxis (Scx) and Mohawk (Mkx), have been identified to be regulated by mechanical stress. Scx is the first transcription factor reported to play an important role in tendon differentiation (Cserjesi et al., 1995). Scx is expressed in tendon progenitor cells and remains highly expressed throughout the differentiation of tendon cells into mature tendon cells (Schweitzer et al., 2001). During tendon differentiation and development, Scx regulates Tenomodulin (Tnmd) to some extent (Liu et al., 2021). Previous studies have shown that overexpression of Scx contributes to bone formation (Agarwal et al., 2017). In addition, studies in recent years have found that Scx (+) progenitor cells positively promote the formation of tendon-bone attachment sites (Blitz et al., 2013; Ideo et al., 2020). Scx has also been shown to be necessary for the development of tendon-bone attachment points (Killian and Thomopoulos, 2016). These studies alone suggest that Scx actively participates in and promotes tendon-bone healing. Scx and other tendon-related genes are elevated when mechanical stress is applied to tendon cells (Yang et al., 2019). One study further found that tension can lead to upregulation of Scx expression more so than compression (Takimoto et al., 2015). Mechanical stress can also maintain Scx expression through the TGF-β/Smad2/3 pathway (Maeda et al., 2011). Another transcription factor, Mkx, has been shown to be expressed in tendon and ligament tissues throughout the body of adult mice (Ito et al., 2010). However, the current study suggests that Mkx has both inhibitory (Anderson et al., 2009; Anderson et al., 2012) and activating (Liu et al., 2015; Otabe et al., 2015; Suzuki et al., 2016) effects. Because Mkx, as an inhibitory factor, controls muscle differentiation (Chuang et al., 2014), only the role of Mkx as an activating factor is discussed here. Mkx is overexpressed in human mesenchymal stem cells and can activate the TGF-β signalling pathway to promote elevated Scx expression (Liu et al., 2015; Otabe et al., 2015). The ability of Scx to actively participate in tendon-bone healing has been discussed previously. Mkx can indirectly regulate tendon-bone healing by activating the TGF-β signalling pathway to elevate Scx expression (Suzuki et al., 2016) (Figure 3). In summary, mechanical stress can promote tendon-bone healing by promoting the expression of the transcription factors Scx and Mkx, but their molecular mechanisms have not been elucidated.
Biological factors
Growth factors
IGF-1 and TGF-β both promote tendon-bone healing to some extent. IGF-1 stimulates the proliferation of various cell types, including tendon cells (Tsuzaki et al., 2000). IGF-1 stimulates collagen synthesis in fibroblasts, including tendon cells, and collagen synthesis is dependent on mechanical loading of the tendon (Hansen et al., 2013). Some studies have shown that IGF-1 stimulates collagen production indirectly through TGF-β1 (Ghahary et al., 2000). Topical administration of IGF-1 promotes collagen synthesis in equine tendons and improves healing in vivo (Durgam et al., 2012). This shows that IGF-1 promotes collagen synthesis, cell proliferation and protein synthesis and exerts anabolic effects on tendon healing. Regarding the effect of mechanical stress on IGF-1, it has been shown that mechanical stress upregulates the production of IGF-1 mRNA and related proteins in a time-dependent manner (Yan et al., 2021). Both cyclic stretching and intermittent mechanical stress can significantly increase IGF-1 expression (Tang et al., 2006; Pumklin et al., 2015). It has been further shown that fluid shear promotes IGF-1 signalling in osteoblasts in a PKA-zeta-dependent manner (Triplett et al., 2007). In addition, stretching stimulates the autocrine secretion of IGF-1 (Perrone et al., 1995; Xian et al., 2007). In conclusion, multiple modalities of mechanical loading can elevate the expression of IGF-1.
Upregulation of TGF-β expression in the New Zealand White Rabbit model promotes tendon-bone healing after ACL reconstruction by regulating TGF-β/MAPK signalling (Wang et al., 2017). There are many isoforms of TGF-β, among which TGF-β1 promotes cell proliferation and migration as well as type III collagen production to promote tendon-bone healing (Kashiwagi et al., 2004; Kim et al., 2011). In contrast, TGF-β3 expression was associated with reduced scar tissue formation after tendon repair (Kovacevic et al., 2011). In a rat model of rotator cuff repair (Kovacevic et al., 2011), it was reported that early postoperative enhancement with bone-conducting calcium-phosphate matrix at the healing tendon-bone junction led to new bone formation, increased fibrocartilage, and improved collagen organization. The addition of TGF-β3 significantly improved the strength of the repair 4 weeks after the operation and produced a more favourable type I/III collagen ratio. In a study on an in vitro fluid shear stress model to mechanically load tendon cells isolated from rats, it was found that fluid shear stress increased TGF-β1 expression but decreased TGF-β2 and TGF-β3 expression (Fong et al., 2005). In addition, the results of cyclic stretching of human tendon fibroblasts under serum-free conditions showed that mechanical stretching in the absence of serum promoted the proliferation of human tendon fibroblasts, upregulated the expression of collagen mRNA and increased the cellular production of type I collagen, part of which was mediated by mechanical stretching to promote the expression of TGF-β1 (Yang et al., 2004). This study also demonstrated that TGF-β1 mRNA expression increased with increasing stretch (Yang et al., 2004). Similar experiments have been performed previously, also by cyclic stretching of human tendon cells, and elevated concentrations of TGF-β were found (Skutek et al., 2001). These results emphasize that tendon mechanical loading induces collagen expression with TGF-β1 and IGF-I as mediators, thereby promoting tendon healing. In summary, mechanical stress can indirectly promote tendon-bone healing by increasing the expression of IGF-1 and TGF-β in various ways (Figure 3). However, studies on this mechanism need to be further validated.
Bone morphogenetic protein (BMP)
BMP may be regulated by mechanical loading. It has been shown that uniaxial cyclic tensile strain is sufficient to increase the expression of BMP-2 (Susperregui et al., 2008). In another study, cyclic tensile strain increased BMP-2 gene expression threefold after 6 h of mechanical loading (Yang et al., 2010). In a study exploring the effect of repeated stretching of rat patellar muscle isolated tendon-derived stem cells (TDSCs) on BMP-2 expression, it was shown that BMP-2 expression was increased in the 4% and 8% stretching groups and that BMP-2 mRNA expression was upregulated in the 4% stretching group (Rui et al., 2011). Other studies have shown that the expression of BMP-2, -6, and -7 mRNA is upregulated in osteoblast cell lines subjected to mechanical stretching, but the expression of BMP-4 does not reflect mechanical loading (Siddhivarn et al., 2007). In summary, some subtypes of the BMP family are regulated by mechanical load, but some subtypes are not, such as BMP-4. Therefore, further studies are needed to investigate which members of the BMP family are regulated by mechanical regulation. The effect of BMP on tendon-bone healing has been demonstrated in numerous studies. In a study of a rotator cuff injury model, BMP-2 promoted tendon-bone healing of rotator cuff tears via the Smad/RUNX2 pathway (Han et al., 2022) (Figure 3). In another rat ACL reconstruction model, combined treatment with BMP-2 and platelet-rich fibrin (PRF) facilitated increased levels of angiogenesis-promoting growth factors and alleviated the inflammatory response, thereby promoting tendon-bone healing (Han et al., 2019). BMP-4 (Chen H. et al., 2021) and BMP-7 (Yu et al., 2007) have also been shown to have an effect on tendon-bone healing. Therefore, it is reasonable to assume that mechanical loading can upregulate the expression of isoforms such as BMP-2 and BMP-7 and then further promote tendon-bone healing.
Matrix metalloproteinases (MMPs) and tissue inhibitors of matrix metalloproteinases (TIMPs)
Previous literature suggests that mechanical loading is capable of regulating MMP expression. For example, in studying the expression of MMP in isolated tendon stem/progenitor cells (TSPCs) from Achilles tendon in response to three different intensities of mechanical stress, it was found that the gene levels of MMP1, MMP2, and MMP3 in mechanically stimulated cells were similar to those in unstimulated cells, indicating that MMP1, MMP2, and MMP3 do not respond to mechanical stress. In contrast, the expression of MMP9, MMP13, and MMP14 was elevated in stretched cells and was independent of the magnitude of the applied stress (Popov et al., 2015). Repeated mechanical loading of fibroblasts revealed that MMP2 expression and activity were upregulated and that high frequency stimulation resulted in greater MMP2 upregulation (Huisman et al., 2016). Other studies and experiments have shown that in tendon cells (Sasaki et al., 2007) and osteoblasts (Wang et al., 2013), mechanical loading leads to elevated expression of MMP.
However, excessive expression of MMPs may disrupt tendon-to-bone healing. MMPs can focus on degrading components of the tissue extracellular matrix and have been shown to play a key role in tissue remodelling (Bedi et al., 2010). In particular, MMP9 and MMP13 contribute to the degradation of the extracellular matrix after injury, where MMP3, MMP4, and MMP14 are involved in matrix interpretation and mechanism remodelling during the healing process (Voleti et al., 2012). Previous studies have shown that the use of TIMPs, which are natural inhibitors of MMPs, may lead to a more mature and organized tendon-bone interface for healing in a rat rotator cuff repair model (Bedi et al., 2010) (Figure 3). It is thus clear that overexpression of MMPs adversely affects tendon-bone healing, but when both MMPs and TIMPs are kept in dynamic balance, effective tendon-bone healing can be promoted. It has been previously described that mechanical loading is able to modulate elevated expression of MMPs, but overexpression of MMPs in turn inhibits tendon-bone healing. Therefore, mechanical loading may in some ways cause MMP overexpression to have a detrimental effect on tendon-bone healing. Therefore, the use of mechanical loading to promote tendon-bone healing could be accompanied by the addition of TIMPs to reduce the overexpression of MMPs. However, this idea needs to be further investigated.
Common gene expression
Tendon-bone healing is a complex process that involves many genes regulated to some extent by forces. As mentioned previously, the role of genes such as Scx, Mkx, IGF-1, TGF-β, MMP, and BMP in tendon-bone healing has been demonstrated, and all are also affected by mechanical loading. All four groups of BMP genes (OP-1/BMP-7, GDF-5/CDMP-1/BMP-14, GDF-6/CDMP2/BMP-13, and GDF-7/CDMP-3/BMP-12) are expressed during healing, but their modes of expression are different (Eliasson et al., 2008). This shows that gene expression during tendon-bone healing is complex, and some genes can be regulated by forces, but the gene expression patterns may be different during the healing process and need further investigation.
Conclusion and prospects
In recent years, there has been increasing interest in research on tendon-bone healing after the treatment of rotator cuff injuries and ACL injuries. The aim is for the patient to be able to return to the preinjury level of function and improve the patient’s quality of life. Therefore, research on tendon-bone healing is necessary, especially regarding what modalities are available to promote it. This paper focuses on studies related to the promotion of tendon-bone healing by external stimulation, such as ESWT, LIPUS, and mechanical loading. The focus is on the possible mechanisms of action of force on tendon-bone healing. This study provides stronger evidential support for subsequent mechanical stress of tendon-bone healing. However, since the therapeutic effects of ESWT and LIPUS have not been thoroughly validated in the clinical setting, further strengthening of clinical trials in this area is needed. In contrast, relatively less research has been done on the mechanism of action of force-stimulated tendon-bone healing, but for the transcription factors Scx and Mkx, which promote tendon-bone healing, the expression of these transcription factors is upregulated after stimulation by mechanical stress, as demonstrated by the study that mechanical stress maintains Scx expression through the TGF-β/Smad2/3 pathway (Maeda et al., 2011). The biomolecules IGF-1, TGF-β, and BMP, which promote tendon-bone healing, are also regulated by mechanical loading to some extent, which can upregulate the expression of IGF-1, TGF-β, and BMP mRNA. It is therefore reasonable to assume that force can promote tendon-bone healing by regulating the expression of transcription factors, biomolecules, and genes related to tendon-bone healing. However, more experimental studies in this area are needed to test this hypothesis. Interestingly, it has also been found that force is able to modulate the upregulation of MMP expression, while MMPs may adversely affect tendon-bone healing to some extent, but the adverse effects can be mitigated by the use of TIMPs. It is thus clear that there is likely an adverse effect of force on tendon-bone healing, to be demonstrated by further studies.
It has been shown that force can promote tendon-bone healing. Therefore, force stimulation is a guide to the clinical treatment of tendon-bone healing. There are early studies on ACL reconstruction for tendon-to-bone healing, such as a trial of cyclic loading using four different fixations in ACL reconstruction, which showed that rehabilitation should not be too aggressive during tendon-to-bone healing and that mechanical loading should not be performed immediately after surgery, as this could cause ACL laxity (Giurea et al., 1999). Further studies found that circulatory loading at the time of surgery restored the initial elongation of the graft (Giurea et al., 1999). A subsequent study further confirmed that delayed loading leads to better organization of the tendon-bone interface (Hettrich et al., 2014). Therefore, the force applied to the ACL reconstruction should be minimized during the tendon-to-bone healing process, and it is also important that the timing of mechanical loading be further determined. However, the timing, intensity and duration of force on tendon-bone healing is also very important. Further research is needed to determine the optimal timing, intensity and duration of action to achieve maximum tendon-bone healing at the time of clinical treatment.
Tendon-bone healing is a complex process involving many cells and their survival in the microenvironment. Previous studies on bone marrow stromal cells (Shi et al., 2020), bone marrow-derived stem cells (Chen W. et al., 2021), and bone marrow mesenchymal stem cells (Huang et al., 2020) have shown that these cells are effective in accelerating tendon-bone healing. Recently, a rather surprising finding was the existence of a delicate balance of the internal environment during tendon healing, i.e., a conflict between tendon stem cells and fibro-adipogenic progenitor (FAP) cells. FAP cells are mesenchymal stem cell-like progenitors found in many tissue types and differentiate into lipogenic, chondrogenic and osteogenic cells. Tendon stem cells differentiate into cartilage and osteoblasts but not into adipocytes (Bi et al., 2007). Better tendon-bone healing can only be achieved when the two are in balance. Therefore, future research should capture the balance between these two types of cells. It has been shown previously that mechanical stress has an effect on tendon stem cells (Govoni et al., 2016); however, more research is needed in this area. Similarly, there are even fewer studies on the effect of mechanical stress on FAP cells. Interestingly, studies have provided evidence that platelet-derived growth factor receptor alpha (PDGFRα) signalling promotes tendon regeneration and fibrosis. The authors conditionally knocked down PDGFRα in tubulin polymerization-promoting protein family member 3-expressing (Tppp3+) cells and tracked the fate of the cells to test whether PDGFRα signalling was required for tendon regeneration. The results confirmed that PDGFRα signalling promotes the proliferative capacity of tendon stem cells and is important for tendon cell differentiation (Harvey et al., 2019). It has been shown that when pressure is applied to the periodontal ligament, the PDGFRα signal gradually increases and then decreases (Zhang et al., 2016). However, it is still unknown whether mechanical stress can modulate PDGFRα signalling during tendon-bone healing. Future studies can focus on these two aspects.
In conclusion, accelerating tendon-bone healing has been shown to be possible with a variety of in vitro stimulation. In vitro stimulation is non-invasive, simple to perform and effective and therefore has a high appeal in promoting tendon-bone healing. However, these findings have been made in limited experimental studies. In future trials, there are still many issues to be resolved before clinical use, such as application technique, duration and timing of action. Also, the mechanism of action of in vitro stimulation on tendon-bone healing and whether mechanical stress modulates PDGFRα signalling will have to be investigated.
Author contributions
SF, YL, GW, DB, BQ, QZ, HL, and VW reviewed the literature and wrote the manuscript. SF, YL, and HL prepared the literature and figured. SF, YL, HL, and VW conceived the idea of the review and revised the manuscript. All authors approved the final manuscript for publication.
Funding
This study was supported by the Luzhou Science and Technology Plan Project (2021-SYF-25) and Scientific Research Project of Southwest Medical University (2021ZKMS051, 2021CXYZ01).
Conflict of interest
The authors declare that the research was conducted in the absence of any commercial or financial relationships that could be construed as a potential conflict of interest.
Publisher’s note
All claims expressed in this article are solely those of the authors and do not necessarily represent those of their affiliated organizations, or those of the publisher, the editors and the reviewers. Any product that may be evaluated in this article, or claim that may be made by its manufacturer, is not guaranteed or endorsed by the publisher.
References
Agarwal, S., Loder, S. J., Cholok, D., Peterson, J., Li, J., Breuler, C., et al. (2017). Scleraxis-lineage cells contribute to ectopic bone formation in muscle and tendon. Stem Cells 35 (3), 705–710. doi:10.1002/stem.2515
Ahmed, I., Salmon, L., Roe, J., and Pinczewski, L. (2017). The long-term clinical and radiological outcomes in patients who suffer recurrent injuries to the anterior cruciate ligament after reconstruction. Bone Jt. J. 99-B (3), 337–343. doi:10.1302/0301-620X.99B3.37863
Anderson, D. M., Beres, B. J., Wilson-Rawls, J., and Rawls, A. (2009). The homeobox gene Mohawk represses transcription by recruiting the sin3A/HDAC co-repressor complex. Dev. Dyn. 238 (3), 572–580. doi:10.1002/dvdy.21873
Anderson, D. M., George, R., Noyes, M. B., Rowton, M., Liu, W., Jiang, R., et al. (2012). Characterization of the DNA-binding properties of the Mohawk homeobox transcription factor. J. Biol. Chem. 287 (42), 35351–35359. doi:10.1074/jbc.M112.399386
Bedi, A., Kovacevic, D., Hettrich, C., Gulotta, L. V., Ehteshami, J. R., Warren, R. F., et al. (2010). The effect of matrix metalloproteinase inhibition on tendon-to-bone healing in a rotator cuff repair model. J. Shoulder Elb. Surg. 19 (3), 384–391. doi:10.1016/j.jse.2009.07.010
Bi, Y., Ehirchiou, D., Kilts, T. M., Inkson, C. A., Embree, M. C., Sonoyama, W., et al. (2007). Identification of tendon stem/progenitor cells and the role of the extracellular matrix in their niche. Nat. Med. 13 (10), 1219–1227. doi:10.1038/nm1630
Blitz, E., Sharir, A., Akiyama, H., and Zelzer, E. (2013). Tendon-bone attachment unit is formed modularly by a distinct pool of Scx- and Sox9-positive progenitors. Development 140 (13), 2680–2690. doi:10.1242/dev.093906
Candiani, G., Riboldi, S. A., Sadr, N., Lorenzoni, S., Neuenschwander, P., Montevecchi, F. M., et al. (2010). Cyclic mechanical stimulation favors myosin heavy chain accumulation in engineered skeletal muscle constructs. J. Appl. Biomater. Biomech. 8 (2), 68–75. doi:10.1177/228080001000800202
Carbone, A., Carballo, C., Ma, R., Wang, H., Deng, X., Dahia, C., et al. (2016). Indian hedgehog signaling and the role of graft tension in tendon-to-bone healing: Evaluation in a rat ACL reconstruction model. J. Orthop. Res. 34 (4), 641–649. doi:10.1002/jor.23066
Casagrande, S. M., Biondo-Simoes, M. L. P., Ioshii, S., Robes, R. R., Biondo-Simoes, R., and Boeno, B. R. O. (2021). Histological evaluation of the effect of low-frequency electric stimulation on healing Achilles tendons in rats. Acta Cir. Bras. 36 (1), e360103. doi:10.1590/ACB360103
Chalmers, P. N., Beck, L., Miller, M., Kawakami, J., Dukas, A. G., Burks, R. T., et al. (2020). Acromial morphology is not associated with rotator cuff tearing or repair healing. J. Shoulder Elb. Surg. 29 (11), 2229–2239. doi:10.1016/j.jse.2019.12.035
Chen, C., Bai, X., Ding, Y., and Lee, I. S. (2019a). Electrical stimulation as a novel tool for regulating cell behavior in tissue engineering. Biomater. Res. 23, 25. doi:10.1186/s40824-019-0176-8
Chen, H., Wang, Z., Zhou, L., Wu, B., Lu, H., Zhang, C., et al. (2021a). Recombinant human bone morphogenetic protein-4 enhances tendon-to-bone attachment healing in a murine model of rotator cuff tear. Ann. Transl. Med. 9 (7), 565. doi:10.21037/atm-20-6761
Chen, S. H., Chou, P. Y., Chen, Z. Y., and Lin, F. H. (2019b). Electrospun water-borne polyurethane nanofibrous membrane as a barrier for preventing postoperative peritendinous adhesion. Int. J. Mol. Sci. 20 (7), 1625. doi:10.3390/ijms20071625
Chen, W., Sun, Y., Gu, X., Cai, J., Liu, X., Zhang, X., et al. (2021b). Conditioned medium of human bone marrow-derived stem cells promotes tendon-bone healing of the rotator cuff in a rat model. Biomaterials 271, 120714. doi:10.1016/j.biomaterials.2021.120714
Chen, Y. J., Wang, C. J., Yang, K. D., Kuo, Y. R., Huang, H. C., Huang, Y. T., et al. (2004). Extracorporeal shock waves promote healing of collagenase-induced Achilles tendinitis and increase TGF-β1 and IGF-I expression. J. Orthop. Res. 22 (4), 854–861. doi:10.1016/j.orthres.2003.10.013
Chen, Y., Zhang, T., Wan, L., Wang, Z., Li, S., Hu, J., et al. (2021c). Early treadmill running delays rotator cuff healing via Neuropeptide Y mediated inactivation of the Wnt/β-catenin signaling. J. Orthop. Transl. 30, 103–111. doi:10.1016/j.jot.2021.08.004
Choi, J. H., Shim, I. K., Shin, M. J., Lee, Y. N., and Koh, K. H. (2022). Stem cell sheet interpositioned between the tendon and bone would be better for healing than stem cell sheet overlaid above the tendon-to-bone junction in rotator cuff repair of rats. PLoS One 17 (3), e0266030. doi:10.1371/journal.pone.0266030
Chow, D. H., Suen, P. K., Fu, L. H., Cheung, W. H., Leung, K. S., Wong, M. W., et al. (2012). Extracorporeal shockwave therapy for treatment of delayed tendon-bone insertion healing in a rabbit model: A dose-response study. Am. J. Sports Med. 40 (12), 2862–2871. doi:10.1177/0363546512461596
Chuang, H. N., Hsiao, K. M., Chang, H. Y., Wu, C. C., and Pan, H. (2014). The homeobox transcription factor Irxl1 negatively regulates MyoD expression and myoblast differentiation. FEBS J. 281 (13), 2990–3003. doi:10.1111/febs.12837
Cserjesi, P., Brown, D., Ligon, K. L., Lyons, G. E., Copeland, N. G., Gilbert, D. J., et al. (1995). Scleraxis: A basic helix-loop-helix protein that prefigures skeletal formation during mouse embryogenesis. Development 121 (4), 1099–1110. doi:10.1242/dev.121.4.1099
Ding, L., Wang, M., Qin, S., and Xu, L. (2021). The roles of MicroRNAs in tendon healing and regeneration. Front. Cell. Dev. Biol. 9, 687117. doi:10.3389/fcell.2021.687117
Durgam, S. S., Stewart, A. A., Pondenis, H. C., Gutierrez-Nibeyro, S. M., Evans, R. B., and Stewart, M. C. (2012). Comparison of equine tendon- and bone marrow-derived cells cultured on tendon matrix with or without insulin-like growth factor-I supplementation. Am. J. Vet. Res. 73 (1), 153–161. doi:10.2460/ajvr.73.1.153
Eliasson, P., Fahlgren, A., and Aspenberg, P. (2008). Mechanical load and BMP signaling during tendon repair: A role for follistatin? Clin. Orthop. Relat. Res. 466 (7), 1592–1597. doi:10.1007/s11999-008-0253-0
Ferrigno, B., Bordett, R., Duraisamy, N., Moskow, J., Arul, M. R., Rudraiah, S., et al. (2020). Bioactive polymeric materials and electrical stimulation strategies for musculoskeletal tissue repair and regeneration. Bioact. Mater 5 (3), 468–485. doi:10.1016/j.bioactmat.2020.03.010
Fong, K. D., Trindade, M. C., Wang, Z., Nacamuli, R. P., Pham, H., Fang, T. D., et al. (2005). Microarray analysis of mechanical shear effects on flexor tendon cells. Plast. Reconstr. Surg. 116 (5), 1393–1404. discussion 1405-1396. doi:10.1097/01.prs.0000182345.86453.4f
Fu, S. C., Cheng, W. H., Cheuk, Y. C., Mok, T. Y., Rolf, C. G., Yung, S. H., et al. (2013). Effect of graft tensioning on mechanical restoration in a rat model of anterior cruciate ligament reconstruction using free tendon graft. Knee Surg. Sports Traumatol. Arthrosc. 21 (5), 1226–1233. doi:10.1007/s00167-012-1974-x
Ghahary, A., Tredget, E. E., Shen, Q., Kilani, R. T., Scott, P. G., and Houle, Y. (2000). Mannose-6-phosphate/IGF-II receptors mediate the effects of IGF-1-induced latent transforming growth factor beta 1 on expression of type I collagen and collagenase in dermal fibroblasts. Growth factors. 17 (3), 167–176. doi:10.3109/08977190009001066
Gimbel, J. A., Van Kleunen, J. P., Lake, S. P., Williams, G. R., and Soslowsky, L. J. (2007). The role of repair tension on tendon to bone healing in an animal model of chronic rotator cuff tears. J. Biomech. 40 (3), 561–568. doi:10.1016/j.jbiomech.2006.02.010
Giurea, M., Zorilla, P., Amis, A. A., and Aichroth, P. (1999). Comparative pull-out and cyclic-loading strength tests of anchorage of hamstring tendon grafts in anterior cruciate ligament reconstruction. Am. J. Sports Med. 27 (5), 621–625. doi:10.1177/03635465990270051301
Govoni, M., Muscari, C., Lovecchio, J., Guarnieri, C., and Giordano, E. (2016). Mechanical actuation systems for the phenotype commitment of stem cell-based tendon and ligament tissue substitutes. Stem Cell. Rev. Rep. 12 (2), 189–201. doi:10.1007/s12015-015-9640-6
Grassi, A., Kim, C., Marcheggiani Muccioli, G. M., Zaffagnini, S., and Amendola, A. (2017). What is the mid-term failure rate of revision ACL reconstruction? A systematic review. Clin. Orthop. Relat. Res. 475 (10), 2484–2499. doi:10.1007/s11999-017-5379-5
Haake, M., Boddeker, I. R., Decker, T., Buch, M., Vogel, M., Labek, G., et al. (2002). Side-effects of extracorporeal shock wave therapy (ESWT) in the treatment of tennis elbow. Arch. Orthop. Trauma Surg. 122 (4), 222–228. doi:10.1007/s00402-001-0362-7
Han, L., Hu, Y. G., Jin, B., Xu, S. C., Zheng, X., and Fang, W. L. (2019). Sustained BMP-2 release and platelet rich fibrin synergistically promote tendon-bone healing after anterior cruciate ligament reconstruction in rat. Eur. Rev. Med. Pharmacol. Sci. 23 (20), 8705–8712. doi:10.26355/eurrev_201910_19264
Han, L., Liu, H., Fu, H., Hu, Y., Fang, W., and Liu, J. (2022). Exosome-delivered BMP-2 and polyaspartic acid promotes tendon bone healing in rotator cuff tear via Smad/RUNX2 signaling pathway. Bioengineered 13 (1), 1459–1475. doi:10.1080/21655979.2021.2019871
Hansen, M., Boesen, A., Holm, L., Flyvbjerg, A., Langberg, H., and Kjaer, M. (2013). Local administration of insulin-like growth factor-I (IGF-I) stimulates tendon collagen synthesis in humans. Scand. J. Med. Sci. Sports 23 (5), 614–619. doi:10.1111/j.1600-0838.2011.01431.x
Harvey, T., Flamenco, S., and Fan, C. M. (2019). A Tppp3(+)Pdgfra(+) tendon stem cell population contributes to regeneration and reveals a shared role for PDGF signalling in regeneration and fibrosis. Nat. Cell. Biol. 21 (12), 1490–1503. doi:10.1038/s41556-019-0417-z
Hettrich, C. M., Gasinu, S., Beamer, B. S., Stasiak, M., Fox, A., Birmingham, P., et al. (2014). The effect of mechanical load on tendon-to-bone healing in a rat model. Am. J. Sports Med. 42 (5), 1233–1241. doi:10.1177/0363546514526138
Hu, J., Qu, J., Xu, D., Zhang, T., Qin, L., and Lu, H. (2014). Combined application of low-intensity pulsed ultrasound and functional electrical stimulation accelerates bone-tendon junction healing in a rabbit model. J. Orthop. Res. 32 (2), 204–209. doi:10.1002/jor.22505
Hu, J., Zhang, T., Xu, D., Qu, J., Qin, L., Zhou, J., et al. (2015). Combined magnetic fields accelerate bone-tendon junction injury healing through osteogenesis. Scand. J. Med. Sci. Sports 25 (3), 398–405. doi:10.1111/sms.12251
Huang, Y., He, B., Wang, L., Yuan, B., Shu, H., Zhang, F., et al. (2020). Bone marrow mesenchymal stem cell-derived exosomes promote rotator cuff tendon-bone healing by promoting angiogenesis and regulating M1 macrophages in rats. Stem Cell. Res. Ther. 11 (1), 496. doi:10.1186/s13287-020-02005-x
Huisman, E., Lu, A., Jamil, S., Mousavizadeh, R., McCormack, R., Roberts, C., et al. (2016). Influence of repetitive mechanical loading on MMP2 activity in tendon fibroblasts. J. Orthop. Res. 34 (11), 1991–2000. doi:10.1002/jor.23207
Ideo, K., Tokunaga, T., Shukunami, C., Takimoto, A., Yoshimoto, Y., Yonemitsu, R., et al. (2020). Role of Scx+/Sox9+ cells as potential progenitor cells for postnatal supraspinatus enthesis formation and healing after injury in mice. PLoS One 15 (12), e0242286. doi:10.1371/journal.pone.0242286
Ito, Y., Toriuchi, N., Yoshitaka, T., Ueno-Kudoh, H., Sato, T., Yokoyama, S., et al. (2010). The Mohawk homeobox gene is a critical regulator of tendon differentiation. Proc. Natl. Acad. Sci. U. S. A. 107 (23), 10538–10542. doi:10.1073/pnas.1000525107
Jeremias Junior, S. L., Camanho, G. L., Bassit, A. C., Forgas, A., Ingham, S. J., and Abdalla, R. J. (2011). Low-intensity pulsed ultrasound accelerates healing in rat calcaneus tendon injuries. J. Orthop. Sports Phys. Ther. 41 (7), 526–531. doi:10.2519/jospt.2011.3468
Karnes, J. L., and Burton, H. W. (2002). Continuous therapeutic ultrasound accelerates repair of contraction-induced skeletal muscle damage in rats. Arch. Phys. Med. Rehabil. 83 (1), 1–4. doi:10.1053/apmr.2002.26254
Kashiwagi, K., Mochizuki, Y., Yasunaga, Y., Ishida, O., Deie, M., and Ochi, M. (2004). Effects of transforming growth factor-beta 1 on the early stages of healing of the Achilles tendon in a rat model. Scand. J. Plast. Reconstr. Surg. Hand Surg. 38 (4), 193–197. doi:10.1080/02844310410029110
Khayyeri, H., Hammerman, M., Turunen, M. J., Blomgran, P., Notermans, T., Guizar-Sicairos, M., et al. (2020). Diminishing effects of mechanical loading over time during rat Achilles tendon healing. PLoS One 15 (12), e0236681. doi:10.1371/journal.pone.0236681
Khoshgoftar, M., van Donkelaar, C. C., and Ito, K. (2011). Mechanical stimulation to stimulate formation of a physiological collagen architecture in tissue-engineered cartilage: A numerical study. Comput. Methods Biomech. Biomed. Engin 14 (2), 135–144. doi:10.1080/10255842.2010.519335
Killian, M. L., and Thomopoulos, S. (2016). Scleraxis is required for the development of a functional tendon enthesis. FASEB J. 30 (1), 301–311. doi:10.1096/fj.14-258236
Kim, H. M., Galatz, L. M., Das, R., Havlioglu, N., Rothermich, S. Y., and Thomopoulos, S. (2011). The role of transforming growth factor beta isoforms in tendon-to-bone healing. Connect. Tissue Res. 52 (2), 87–98. doi:10.3109/03008207.2010.483026
Korstjens, C. M., Nolte, P. A., Burger, E. H., Albers, G. H., Semeins, C. M., Aartman, I. H., et al. (2004). Stimulation of bone cell differentiation by low-intensity ultrasound--a histomorphometric in vitro study. J. Orthop. Res. 22 (3), 495–500. doi:10.1016/j.orthres.2003.09.011
Kovacevic, D., Fox, A. J., Bedi, A., Ying, L., Deng, X. H., Warren, R. F., et al. (2011). Calcium-phosphate matrix with or without TGF-β3 improves tendon-bone healing after rotator cuff repair. Am. J. Sports Med. 39 (4), 811–819. doi:10.1177/0363546511399378
Lacheta, L., and Braun, S. (2021). Limited evidence for biological treatment measures for cartilage and tendon injuries of the shoulder. Knee Surg. Sports Traumatol. Arthrosc. 30 (4), 1132–1137. doi:10.1007/s00167-021-06499-7
Lai, W. C., Iglesias, B. C., Mark, B. J., and Wang, D. (2021). Low-intensity pulsed ultrasound augments tendon, ligament, and bone-soft tissue healing in preclinical animal models: A systematic review. Arthroscopy 37 (7), 2318–2333.e3. doi:10.1016/j.arthro.2021.02.019
Lebaschi, A. H., Deng, X. H., Camp, C. L., Zong, J., Cong, G. T., Carballo, C. B., et al. (2018). Biomechanical, histologic, and molecular evaluation of tendon healing in a new murine model of rotator cuff repair. Arthroscopy 34 (4), 1173–1183. doi:10.1016/j.arthro.2017.10.045
Lee, H. J., Choi, B. H., Min, B. H., Son, Y. S., and Park, S. R. (2006). Low-intensity ultrasound stimulation enhances chondrogenic differentiation in alginate culture of mesenchymal stem cells. Artif. Organs 30 (9), 707–715. doi:10.1111/j.1525-1594.2006.00288.x
Levine, J. W., Kiapour, A. M., Quatman, C. E., Wordeman, S. C., Goel, V. K., Hewett, T. E., et al. (2013). Clinically relevant injury patterns after an anterior cruciate ligament injury provide insight into injury mechanisms. Am. J. Sports Med. 41 (2), 385–395. doi:10.1177/0363546512465167
Li, Z., Li, Q., Tong, K., Zhu, J., Wang, H., Chen, B., et al. (2022). BMSC-derived exosomes promote tendon-bone healing after anterior cruciate ligament reconstruction by regulating M1/M2 macrophage polarization in rats. Stem Cell. Res. Ther. 13 (1), 295. doi:10.1186/s13287-022-02975-0
Liu, C., Bai, J., Yu, K., Liu, G., Tian, S., and Tian, D. (2019). Biological amnion prevents flexor tendon adhesion in zone II: A controlled, multicentre clinical trial. Biomed. Res. Int. 2019, 1–9. doi:10.1155/2019/2354325
Liu, H., Xu, J., Lan, Y., Lim, H. W., and Jiang, R. (2021). The Scleraxis transcription factor directly regulates multiple distinct molecular and cellular processes during early tendon cell differentiation. Front. Cell. Dev. Biol. 9, 654397. doi:10.3389/fcell.2021.654397
Liu, H., Zhang, C., Zhu, S., Lu, P., Zhu, T., Gong, X., et al. (2015). Mohawk promotes the tenogenesis of mesenchymal stem cells through activation of the TGFβ signaling pathway. Stem Cells 33 (2), 443–455. doi:10.1002/stem.1866
Liu, S. H., Panossian, V., al-Shaikh, R., Tomin, E., Shepherd, E., Finerman, G. A., et al. (1997). Morphology and matrix composition during early tendon to bone healing. Clin. Orthop. Relat. Res. 339, 253–260. doi:10.1097/00003086-199706000-00034
Liu, Y., Wang, L., Li, S., Zhang, T., Chen, C., Hu, J., et al. (2022). Mechanical stimulation improves rotator cuff tendon-bone healing via activating IL-4/JAK/STAT signaling pathway mediated macrophage M2 polarization. J. Orthop. Transl. 37, 78–88. doi:10.1016/j.jot.2022.08.008
Longo, U. G., Berton, A., Risi Ambrogioni, L., Lo Presti, D., Carnevale, A., Candela, V., et al. (2020). Cost-effectiveness of supervised versus unsupervised rehabilitation for rotator-cuff repair: Systematic review and meta-analysis. Int. J. Environ. Res. Public Health 17 (8), 2852. doi:10.3390/ijerph17082852
Lovric, V., Ledger, M., Goldberg, J., Harper, W., Bertollo, N., Pelletier, M. H., et al. (2013). The effects of low-intensity pulsed ultrasound on tendon-bone healing in a transosseous-equivalent sheep rotator cuff model. Knee Surg. Sports Traumatol. Arthrosc. 21 (2), 466–475. doi:10.1007/s00167-012-1972-z
Lu, H., Liu, F., Chen, H., Chen, C., Qu, J., Xu, D., et al. (2016). The effect of low-intensity pulsed ultrasound on bone-tendon junction healing: Initiating after inflammation stage. J. Orthop. Res. 34 (10), 1697–1706. doi:10.1002/jor.23180
Lu, H., Qin, L., Cheung, W., Lee, K., Wong, W., and Leung, K. (2008). Low-intensity pulsed ultrasound accelerated bone-tendon junction healing through regulation of vascular endothelial growth factor expression and cartilage formation. Ultrasound Med. Biol. 34 (8), 1248–1260. doi:10.1016/j.ultrasmedbio.2008.01.009
Lu, H., Qin, L., Fok, P., Cheung, W., Lee, K., Guo, X., et al. (2006). Low-intensity pulsed ultrasound accelerates bone-tendon junction healing: A partial patellectomy model in rabbits. Am. J. Sports Med. 34 (8), 1287–1296. doi:10.1177/0363546506286788
Lü, J., Shi, Y., Wang, Y., Kang, X., Bian, X., Yuan, B., et al. (2019). Research progress of structured repair of tendon-bone interface. Zhongguo Xiu Fu Chong Jian Wai Ke Za Zhi 33 (9), 1064–1070. doi:10.7507/1002-1892.201811139
Lui, P., Zhang, P., Chan, K., and Qin, L. (2010). Biology and augmentation of tendon-bone insertion repair. J. Orthop. Surg. Res. 5, 59. doi:10.1186/1749-799X-5-59
Maeda, T., Sakabe, T., Sunaga, A., Sakai, K., Rivera, A. L., Keene, D. R., et al. (2011). Conversion of mechanical force into TGF-beta-mediated biochemical signals. Curr. Biol. 21 (11), 933–941. doi:10.1016/j.cub.2011.04.007
Musahl, V., Solomon, C. G., and Karlsson, J. (2019). Anterior cruciate ligament tear. N. Engl. J. Med. 380 (24), 2341–2348. doi:10.1056/NEJMcp1805931
Nakamichi, R., and Asahara, H. (2021). Regulation of tendon and ligament differentiation. Bone 143, 115609. doi:10.1016/j.bone.2020.115609
Ogden, J. A., Toth-Kischkat, A., and Schultheiss, R. (2001). Principles of shock wave therapy. Clin. Orthop. Relat. Res. 387, 8–17. doi:10.1097/00003086-200106000-00003
Otabe, K., Nakahara, H., Hasegawa, A., Matsukawa, T., Ayabe, F., Onizuka, N., et al. (2015). Transcription factor Mohawk controls tenogenic differentiation of bone marrow mesenchymal stem cells in vitro and in vivo. J. Orthop. Res. 33 (1), 1–8. doi:10.1002/jor.22750
Peng, Y., Wu, W., Li, X., Shangguan, H., Diao, L., Ma, H., et al. (2022). Effects of leukocyte-rich platelet-rich plasma and leukocyte-poor platelet-rich plasma on the healing of bone-tendon interface of rotator cuff in a mice model. Platelets 33 (7), 1075–1082. doi:10.1080/09537104.2022.2044462
Perrone, C. E., Fenwick-Smith, D., and Vandenburgh, H. H. (1995). Collagen and stretch modulate autocrine secretion of insulin-like growth factor-1 and insulin-like growth factor binding proteins from differentiated skeletal muscle cells. J. Biol. Chem. 270 (5), 2099–2106. doi:10.1074/jbc.270.5.2099
Popov, C., Burggraf, M., Kreja, L., Ignatius, A., Schieker, M., and Docheva, D. (2015). Mechanical stimulation of human tendon stem/progenitor cells results in upregulation of matrix proteins, integrins and MMPs, and activation of p38 and ERK1/2 kinases. BMC Mol. Biol. 16, 6. doi:10.1186/s12867-015-0036-6
Pumklin, J., Manokawinchoke, J., Bhalang, K., and Pavasant, P. (2015). Intermittent compressive stress enhanced insulin-like growth factor-1 expression in human periodontal ligament cells. Int. J. Cell. Biol. 2015, 1–9. doi:10.1155/2015/369874
Raabe, O., Shell, K., Goessl, A., Crispens, C., Delhasse, Y., Eva, A., et al. (2013). Effect of extracorporeal shock wave on proliferation and differentiation of equine adipose tissue-derived mesenchymal stem cells in vitro. Am. J. Stem Cells 2 (1), 62–73.
Rees, J. D., Maffulli, N., and Cook, J. (2009). Management of tendinopathy. Am. J. Sports Med. 37 (9), 1855–1867. doi:10.1177/0363546508324283
Rui, Y. F., Lui, P. P., Ni, M., Chan, L. S., Lee, Y. W., and Chan, K. M. (2011). Mechanical loading increased BMP-2 expression which promoted osteogenic differentiation of tendon-derived stem cells. J. Orthop. Res. 29 (3), 390–396. doi:10.1002/jor.21218
Salvatore, G., Longo, U. G., Candela, V., Berton, A., Migliorini, F., Petrillo, S., et al. (2020). Epidemiology of rotator cuff surgery in Italy: Regional variation in access to health care. Results from a 14-year nationwide registry. Musculoskelet. Surg. 104 (3), 329–335. doi:10.1007/s12306-019-00625-y
Sasaki, K., Takagi, M., Konttinen, Y. T., Sasaki, A., Tamaki, Y., Ogino, T., et al. (2007). Upregulation of matrix metalloproteinase (MMP)-1 and its activator MMP-3 of human osteoblast by uniaxial cyclic stimulation. J. Biomed. Mater Res. B Appl. Biomater. 80 (2), 491–498. doi:10.1002/jbm.b.30622
Schweitzer, R., Chyung, J. H., Murtaugh, L. C., Brent, A. E., Rosen, V., Olson, E. N., et al. (2001). Analysis of the tendon cell fate using Scleraxis, a specific marker for tendons and ligaments. Development 128 (19), 3855–3866. doi:10.1242/dev.128.19.3855
Sgroi, T. A., and Cilenti, M. (2018). Rotator cuff repair: Post-operative rehabilitation concepts. Curr. Rev. Musculoskelet. Med. 11 (1), 86–91. doi:10.1007/s12178-018-9462-7
Shi, Y., Kang, X., Wang, Y., Bian, X., He, G., Zhou, M., et al. (2020). Exosomes derived from bone marrow stromal cells (BMSCs) enhance tendon-bone healing by regulating macrophage polarization. Med. Sci. Monit. 26, e923328. doi:10.12659/msm.923328
Siddhivarn, C., Banes, A., Champagne, C., Riché, E. L., Weerapradist, W., and Offenbacher, S. (2007). Mechanical loading and delta12prostaglandin J2 induce bone morphogenetic protein-2, peroxisome proliferator-activated receptor gamma-1, and bone nodule formation in an osteoblastic cell line. J. Periodontal Res. 42 (5), 383–392. doi:10.1111/j.1600-0765.2006.00965.x
Skutek, M., van Griensven, M., Zeichen, J., Brauer, N., and Bosch, U. (2001). Cyclic mechanical stretching modulates secretion pattern of growth factors in human tendon fibroblasts. Eur. J. Appl. Physiol. 86 (1), 48–52. doi:10.1007/s004210100502
Song, F., Jiang, D., Wang, T., Wang, Y., Chen, F., Xu, G., et al. (2017). Mechanical loading improves tendon-bone healing in a rabbit anterior cruciate ligament reconstruction model by promoting proliferation and matrix formation of mesenchymal stem cells and tendon cells. Cell. Physiol. Biochem. 41 (3), 875–889. doi:10.1159/000460005
Susperregui, A. R., Viñals, F., Ho, P. W., Gillespie, M. T., Martin, T. J., and Ventura, F. (2008). BMP-2 regulation of PTHrP and osteoclastogenic factors during osteoblast differentiation of C2C12 cells. J. Cell. Physiol. 216 (1), 144–152. doi:10.1002/jcp.21389
Suzuki, H., Ito, Y., Shinohara, M., Yamashita, S., Ichinose, S., Kishida, A., et al. (2016). Gene targeting of the transcription factor Mohawk in rats causes heterotopic ossification of Achilles tendon via failed tenogenesis. Proc. Natl. Acad. Sci. U. S. A. 113 (28), 7840–7845. doi:10.1073/pnas.1522054113
Takakura, Y., Matsui, N., Yoshiya, S., Fujioka, H., Muratsu, H., Tsunoda, M., et al. (2002). Low-intensity pulsed ultrasound enhances early healing of medial collateral ligament injuries in rats. J. Ultrasound Med. 21 (3), 283–288. doi:10.7863/jum.2002.21.3.283
Takimoto, A., Kawatsu, M., Yoshimoto, Y., Kawamoto, T., Seiryu, M., Takano-Yamamoto, T., et al. (2015). Scleraxis and osterix antagonistically regulate tensile force-responsive remodeling of the periodontal ligament and alveolar bone. Development 142 (4), 787–796. doi:10.1242/dev.116228
Tang, L. L., Xian, C. Y., and Wang, Y. L. (2006). The MGF expression of osteoblasts in response to mechanical overload. Arch. Oral Biol. 51 (12), 1080–1085. doi:10.1016/j.archoralbio.2006.06.009
Tang, Y., Chen, C., Liu, F., Xie, S., Qu, J., Li, M., et al. (2020). Structure and ingredient-based biomimetic scaffolds combining with autologous bone marrow-derived mesenchymal stem cell sheets for bone-tendon healing. Biomaterials 241, 119837. doi:10.1016/j.biomaterials.2020.119837
Triplett, J. W., O'Riley, R., Tekulve, K., Norvell, S. M., and Pavalko, F. M. (2007). Mechanical loading by fluid shear stress enhances IGF-1 receptor signaling in osteoblasts in a PKCzeta-dependent manner. Mol. Cell. Biomech. 4 (1), 13–25.
Tsuzaki, M., Brigman, B. E., Yamamoto, J., Lawrence, W. T., Simmons, J. G., Mohapatra, N. K., et al. (2000). Insulin-like growth factor-I is expressed by avian flexor tendon cells. J. Orthop. Res. 18 (4), 546–556. doi:10.1002/jor.1100180406
Voleti, P. B., Buckley, M. R., and Soslowsky, L. J. (2012). Tendon healing: Repair and regeneration. Annu. Rev. Biomed. Eng. 14, 47–71. doi:10.1146/annurev-bioeng-071811-150122
Wang, C. J., Ko, J. Y., Chou, W. Y., Hsu, S. L., Ko, S. F., Huang, C. C., et al. (2014). Shockwave therapy improves anterior cruciate ligament reconstruction. J. Surg. Res. 188 (1), 110–118. doi:10.1016/j.jss.2014.01.050
Wang, F. S., Kuo, Y. R., Wang, C. J., Yang, K. D., Chang, P. R., Huang, Y. T., et al. (2004). Nitric oxide mediates ultrasound-induced hypoxia-inducible factor-1α activation and vascular endothelial growth factor-A expression in human osteoblasts. Bone 35 (1), 114–123. doi:10.1016/j.bone.2004.02.012
Wang, L., Qin, L., Lu, H.-B., Cheung, W.-H., Yang, H., Wong, W.-N., et al. (2007). Extracorporeal shock wave therapy in treatment of delayed bone-tendon healing. Am. J. Sports Med. 36 (2), 340–347. doi:10.1177/0363546507307402
Wang, R., Xu, B., and Xu, H. G. (2017). Up-regulation of TGF-β promotes tendon-to-bone healing after anterior cruciate ligament reconstruction using bone marrow-derived mesenchymal stem cells through the TGF-β/MAPK signaling pathway in a New Zealand white rabbit model. Cell. Physiol. Biochem. 41 (1), 213–226. doi:10.1159/000456046
Wang, T., Lin, Z., Day, R. E., Gardiner, B., Landao-Bassonga, E., Rubenson, J., et al. (2013). Programmable mechanical stimulation influences tendon homeostasis in a bioreactor system. Biotechnol. Bioeng. 110 (5), 1495–1507. doi:10.1002/bit.24809
Wong, M. W., Qin, L., Lee, K. M., Tai, K. O., Chong, W. S., Leung, K. S., et al. (2003). Healing of bone-tendon junction in a bone trough: A goat partial patellectomy model. Clin. Orthop. Relat. Res. 413, 291–302. doi:10.1097/01.blo.0000076802.53006.5b
Xian, C., Wang, Y., Zhang, B., and Tang, L. (2007). Effects of mechanical strain on the proliferation and expression of IGF-1 mRNA in rat osteoblasts. Sheng Wu Yi Xue Gong Cheng Xue Za Zhi 24 (2), 312–315.
Xu, D., Zhang, T., Qu, J., Hu, J., and Lu, H. (2014). Enhanced patella-patellar tendon healing using combined magnetic fields in a rabbit model. Am. J. Sports Med. 42 (10), 2495–2501. doi:10.1177/0363546514541539
Xu, Y., Zhang, W.-X., Wang, L.-N., Ming, Y.-Q., Li, Y.-L., and Ni, G.-X. (2021). Stem cell therapies in tendon-bone healing. World J. Stem Cells 13 (7), 753–775. doi:10.4252/wjsc.v13.i7.753
Xu, Z., Li, S., Wan, L., Hu, J., Lu, H., and Zhang, T. (2022). Role of low-intensity pulsed ultrasound in regulating macrophage polarization to accelerate tendon-bone interface repair. J. Orthop. Res. doi:10.1002/jor.25454
Yamakado, K., Kitaoka, K., Yamada, H., Hashiba, K., Nakamura, R., and Tomita, K. (2002). The influence of mechanical stress on graft healing in a bone tunnel. Arthroscopy 18 (1), 82–90. doi:10.1053/jars.2002.25966
Yamamoto, E., Hayashi, K., and Yamamoto, N. (2000). Effects of stress shielding on the transverse mechanical properties of rabbit patellar tendons. J. Biomech. Eng. 122 (6), 608–614. doi:10.1115/1.1319660
Yan, B., Zeng, C., Chen, Y., Huang, M., Yao, N., Zhang, J., et al. (2021). Mechanical stress-induced IGF-1 facilitates col-I and col-III synthesis via the IGF-1R/AKT/mTORC1 signaling pathway. Stem Cells Int. 2021, 1–11. doi:10.1155/2021/5553676
Yang, C., Teng, Y., Geng, B., Xiao, H., Chen, C., Chen, R., et al. (2023). Strategies for promoting tendon-bone healing: Current status and prospects. Front. Bioeng. Biotechnol. 11, 1118468. doi:10.3389/fbioe.2023.1118468
Yang, F., Zhang, A., and Richardson, D. W. (2019). Regulation of the tenogenic gene expression in equine tenocyte-derived induced pluripotent stem cells by mechanical loading and Mohawk. Stem Cell. Res. 39, 101489. doi:10.1016/j.scr.2019.101489
Yang, G., Crawford, R. C., and Wang, J. H. (2004). Proliferation and collagen production of human patellar tendon fibroblasts in response to cyclic uniaxial stretching in serum-free conditions. J. Biomech. 37 (10), 1543–1550. doi:10.1016/j.jbiomech.2004.01.005
Yang, X., Gong, P., Lin, Y., Zhang, L., Li, X., Yuan, Q., et al. (2010). Cyclic tensile stretch modulates osteogenic differentiation of adipose-derived stem cells via the BMP-2 pathway. Arch. Med. Sci. 6 (2), 152–159. doi:10.5114/aoms.2010.13886
Ying, Z. M., Lin, T., and Yan, S. G. (2012). Low-intensity pulsed ultrasound therapy: A potential strategy to stimulate tendon-bone junction healing. J. Zhejiang Univ. Sci. B 13 (12), 955–963. doi:10.1631/jzus.B1200129
Yu, L., Liu, S., Zhao, Z., Xia, L., Zhang, H., Lou, J., et al. (2017). Extracorporeal shock wave rebuilt subchondral bone in vivo and activated Wnt5a/Ca(2+) signaling in vitro. Biomed. Res. Int. 2017, 1–10. doi:10.1155/2017/1404650
Yu, Y., Bliss, J. P., Bruce, W. J., and Walsh, W. R. (2007). Bone morphogenetic proteins and Smad expression in ovine tendon-bone healing. Arthroscopy 23 (2), 205–210. doi:10.1016/j.arthro.2006.08.023
Zhang, L., Liu, W., Zhao, J., Ma, X., Shen, L., Zhang, Y., et al. (2016). Mechanical stress regulates osteogenic differentiation and RANKL/OPG ratio in periodontal ligament stem cells by the Wnt/β-catenin pathway. Biochim. Biophys. Acta 1860 (10), 2211–2219. doi:10.1016/j.bbagen.2016.05.003
Zhang, S., Li, H., Tao, H., Li, H., Cho, S., Hua, Y., et al. (2013). Delayed early passive motion is harmless to shoulder rotator cuff healing in a rabbit model. Am. J. Sports Med. 41 (8), 1885–1892. doi:10.1177/0363546513493251
Zhu, M., Lin Tay, M., Lim, K. S., Bolam, S. M., Tuari, D., Callon, K., et al. (2022). Novel growth factor combination for improving rotator cuff repair: A rat in vivo study. Am. J. Sports Med. 50 (4), 1044–1053. doi:10.1177/03635465211072557
Zou, J., Yang, W., Cui, W., Li, C., Ma, C., Ji, X., et al. (2023). Therapeutic potential and mechanisms of mesenchymal stem cell-derived exosomes as bioactive materials in tendon-bone healing. J. Nanobiotechnology 21 (1), 14. doi:10.1186/s12951-023-01778-6
Zwerver, J., Hartgens, F., Verhagen, E., van der Worp, H., van den Akker-Scheek, I., and Diercks, R. L. (2011). No effect of extracorporeal shockwave therapy on patellar tendinopathy in jumping athletes during the competitive season: A randomized clinical trial. Am. J. Sports Med. 39 (6), 1191–1199. doi:10.1177/0363546510395492
Keywords: tendon-bone healing, extracorporeal shock wave, low-intensity pulsed ultrasound, mechanical stress, mechanism of action
Citation: Fu S, Lan Y, Wang G, Bao D, Qin B, Zheng Q, Liu H and Wong VKW (2023) External stimulation: A potential therapeutic strategy for tendon-bone healing. Front. Bioeng. Biotechnol. 11:1150290. doi: 10.3389/fbioe.2023.1150290
Received: 24 January 2023; Accepted: 23 March 2023;
Published: 31 March 2023.
Edited by:
Lan Li, Nanjing Drum Tower Hospital, ChinaReviewed by:
Qing Luo, Chongqing University, ChinaZhang Bingyu, Chongqing University of Posts and Telecommunications, China
Hanwei Cui, Army Medical University, China
Copyright © 2023 Fu, Lan, Wang, Bao, Qin, Zheng, Liu and Wong. This is an open-access article distributed under the terms of the Creative Commons Attribution License (CC BY). The use, distribution or reproduction in other forums is permitted, provided the original author(s) and the copyright owner(s) are credited and that the original publication in this journal is cited, in accordance with accepted academic practice. No use, distribution or reproduction is permitted which does not comply with these terms.
*Correspondence: Huan Liu, MjAwMTYwNDBAMTYzLmNvbQ==; Vincent Kam Wai Wong, Ym93YWl3b25nQGdtYWlsLmNvbQ==
†These authors have contributed equally to this work and share first authorship