- 1College of Biology and the Environment, Nanjing Forestry University, Nanjing, China
- 2School of Pharmacy, Changzhou University, Changzhou, China
- 3College of Forestry, Nanjing Forestry University, Nanjing, China
The objective of this work was to develop an efficient approach for chemoenzymatically transforming biomass to furfurylamine by bridging chemocatalysis and biocatalysis in a deep eutectic solvent of EaCl:Gly–water. Using hydroxyapatite (HAP) as support, heterogeneous catalyst SO42−/SnO2–HAP was synthesized for transforming lignocellulosic biomass into furfural using organic acid as a co-catalyst. The turnover frequency (TOF) was correlated with the pKa value of the used organic acid. Corncob was transformed by oxalic acid (pKa = 1.25) (0.4 wt%) plus SO42−/SnO2–HAP (2.0 wt%) to produce furfural with a yield of 48.2% and a TOF of 6.33 h-1 in water. In deep eutectic solvent EaCl:Gly–water (1:2, v/v), co-catalysis with SO42−/SnO2–HAP and oxalic acid was utilized to transform corncob, rice straw, reed leaf, and sugarcane bagasse for the production of furfural with the yield of 42.4%–59.3% (based on the xylan content) at 180°C after 10 min. The formed furfural could be efficiently aminated to furfurylamine with E. coli CCZU-XLS160 cells in the presence of NH4Cl (as an amine donor). As a result of the biological amination of furfural derived from corncob, rice straw, reed leaf, and sugarcane bagasse for 24 h, the yields of furfurylamine reached >99%, with a productivity of 0.31–0.43 g furfurylamine per g xylan. In EaCl:Gly–water, an efficient chemoenzymatic catalysis strategy was employed to valorize lignocellulosic biomass into valuable furan chemicals.
1 Introduction
Biorefinery concept is highly promising for the sustainable utilization of biomass to manufacture energy molecules, platform chemicals, and functional materials (Himmel et al., 2007; Delidovich et al., 2016; He et al., 2017a; Riva et al., 2021). The cost-efficient transformation of lignocellulosic biomass has attracted much attention for achieving a sustainable society (Cai et al., 2014; Guenic et al., 2015). Lignocellulose is mainly composed of three primary components including lignin, hemicellulose, and cellulose (Gong et al., 2019; Riva et al., 2021), which is regarded as a sustainable bioresource because of its availability, abundance, low cost, and renewable characteristics (Hu et al., 2014; Kaiprommarat et al., 2016). Hemicellulose, an amorphous heteropolysaccharide, is mainly composed of different C5 and C6 sugar monomers (Peleteiro et al., 2014; Lee and Wu, 2021). Xylan, which consists of D-xylose units, represents the main component of hemicellulose (Morais et al., 2020). As an important xylan-based product, furfural (FF) is a key building block (Zang and Chen, 2015; He et al., 2017b; Sweygers et al., 2018), which was listed in the “Top10 + 4” chemicals by the US Department of Energy (DOE) in 2004 (Bozell and Petersen, 2010). FF is a good solvent. It has been utilized as a renewable material for biofuels, detergents, lubricants, polymers, resins, food additives, pharmaceuticals (antiseptics and disinfectors), and agrochemicals (herbicides, insecticides, and pesticides) (Agirrezabal-Telleria et al., 2014; Douthwaite et al., 2017; Peng et al., 2019; Gong et al., 2022). It is an important precursor for widely synthesizing furan bio-based chemicals such as 2-methylfuran, furoic acid, cyclopentanone, furfuryl alcohol, succinic acid, furfurylamine (FAM), tetrahydrofuran, γ-valerolactone, and maleic acid (Lange et al., 2012; Möller and Schröder, 2013; Feng et al., 2016; Qiu et al., 2020; Zheng et al., 2020).
FAM, as a valuable furan-based chemical, has a vital role in manufacturing chemical intermediates, bioactive molecules, medicines, etc. (Dong et al., 2020). Industrially, FAM can be prepared via the chemical amination of FF under high temperature/pressure conditions, and this kind of amination requires a harsh performance and expensive catalysts, accompanied with potential environmental pollution (Zhang et al., 2019). Distinct from the chemical amination of FAM, biological amination of FF has gained great interest due to its mild performance condition, simple reaction, high catalytic activity, low toxicity, and environmental friendliness. ω-Transaminase is a pyridoxamine 5′-phosphate (PLP)-dependent enzyme, which has good selectivity for reversibly transforming the exchange of ketone groups (C=O) and an amino group (-NH2). The biomass-derived FF (90 mM) was aminated into FAM (74%) by Escherichia coli CV-PRSFDuet expressing ω-transaminase at 35°C (Zhang et al., 2019). To chemoenzymatically valorize biomass into FAM in a tandem reaction with a chemocatalyst and biocatalyst, it is necessary to employ an efficient strategy for the conversion of biomass into FF with biocompatible catalysts in an eco-friendly reaction system.
In recent years, homogeneous and heterogeneous catalysts have been utilized for FF production. Various acids (H2SO4, HCl, H3PO4, oxalic acid, formic acid, acetic acid, etc.) and salts (AlCl3, CoCl2, CrCl3, MaCl2, MnCl2, NaCl, NiCl2, SnCl4, etc.) are used as homogeneous catalysts (Rong et al., 2012; Enslow and Bell, 2015), which can be uniformly distributed in solvents. These homogeneous catalysts have high catalytic activity. However, their high loading will cause serious environmental pollution, and their recycle is a challenge. Heterogeneous catalysts that can be prepared by using a series of supports (e.g., graphene, zeolite, niobium oxide, niobium phosphate, sepiolite, kaoline, fly ash, carbon nanotube, resin, and carbon) have gained much attention because of their large surface areas, strong acidity, low corrosion, easy separation, and good thermal stability (Garcia-Sancho et al., 2013; Pholjaroen et al., 2013; Ma et al., 2019; Zhu et al., 2020; Di et al., 2021; Li et al., 2021). Although heterogeneous catalysts are easy to be recovered and have low corrosion (Li et al., 2019), the catalytic efficiency is still not satisfactory. The co-catalysis of homogeneous and heterogeneous catalysts might be used for the efficient transformation of biomass into FF, which deserves in-depth exploration.
In the production of FF from lignocellulosic biomass, used solvents have a crucial role in the enhancement of FF productivity (Lee and Wu, 2021). It is known that some organic solvents can confine the undesired side reactions (e.g., FF condensation and FF resinification) in water (Lam et al., 2012). Various solvents (e.g., dimethyl sulfoxide, ethanol, hexane, ionic liquids, toluene, γ-valerolactone, and dioctyl phthalate) have been employed to transform biomass or D-xylose, which resulted in the increased FF productivity (Tau et al., 2016; Xu et al., 2017; Widsten et al., 2018; Ma et al., 2020). Recently, it is of great interest to select more eco-friendly and thermostable solvents for enhancing FF production. Deep eutectic solvents (DESs), which are composed of mixing HBAs (hydrogen-bond acceptors) with HBDs (hydrogen-bond donors) (Li et al., 2018), have recently gained tremendous attention for FF production because of their low vapor pressure, good reusability, low toxicity, high thermostability, and ease of preparation (Xu et al., 2020; Pan et al., 2022). In acetone–ChCl:EG–water at 180°C, D-xylose was transformed to FF in 0.5 h at 75% yield by AlCl3 (Chen and Wan, 2019). Thus, DESs can be utilized as promising solvents for improving FF production because of their unique properties, which would restrict the undesired side reactions and promote FF formation.
To enhance FAM yield from renewable biomass, a chemoenzymatic conversion was developed in a tandem reaction by bridging chemocatalysis and biocatalysis in an eco-friendly DES–water system (Scheme 1). Hydroxyapatites (HAPs, Ca10(PO4)6(OH)2), which are naturally occurring phosphate minerals, were used as a carrier to prepare sulfonated tine-based heterogeneous catalyst SO42−/SnO2–HAP. First, biomass was converted into FF via co-catalysis with a homogeneous catalyst and a heterogeneous catalyst in an EaCl:Gly–water system. An optimized system for the production of FF from lignocellulose was established by combined homogeneous organic acids with heterogeneous SO42−/SnO2–HAP. The turnover frequency (TOF) was correlated with the pKa value of organic acids. The catalytic reaction parameters (e.g., type of organic acid, organic acid loading, SO42−/SnO2–HAP loading, DES EaCl:Gly dosage, performance temperature, and catalytic time) were examined to improve FF production. Furthermore, biomass-valorized FF was biologically aminated into FAM in EaCl:Gly–water. An efficient chemoenzymatic strategy was employed to valorize biomass into valuable furan-based chemicals, realizing the high-value utilization of biomass.
2 Materials and methods
2.1 Reagents and materials
Corncob (CC), rice straw (RS), reed leaf (RL), and sugarcane bagasse (SB) were collected in a village market in Tieling city (Liaoning province, P.R. China). Hydroxyapatite (HAP), oxalic acid, SnCl4·5H2O, NaCl, and other chemicals were bought from Aladdin Industrial Inc. (Shanghai, China) and other commercial sources.
2.2 SO42−/SnO2–HAP and DES EaCl:Gly preparation
Sulfonated heterogeneous catalyst SO42−/SnO2–HAP was prepared with SnCl4·5H2O and HAP in a mass ratio of 1:3 in 1 L of ethanol by stirring. The preparation procedure of SO42−/SnO2–HAP was carried out according to the method proposed by Zhang et al. (2019). DES EaCl:Gly was prepared according to the procedure proposed by Xu et al. (2020).
2.3 Co-catalysis of CC organic acids and SO42−/SnO2–HAP in EaCl:Gly–water
To test the co-catalysis of biomass into FF with organic acids and SO42−/SnO2–HAP on the effects of FF formation, water (40 mL), CC (7.5 wt%), and SO42−/SnO2–HAP (2.0 wt%) were mixed with different pKa values of the organic acid (0.4 wt%) at 180°C for 10 min. For testing the oxalic acid dosage on the effect of FF generation, different loads of oxalic acid (0–0.6 wt%) were mixed with water (40 mL), CC (7.5 wt%), and SO42−/SnO2–HAP (2.0 wt%) in a reactor (180°C) for 10 min. To establish an appropriate DES–water system for improving FF production, several volumetric ratios of EaCl:Gly–water (0:1, 1:3, 1:2, 1:1, 2:1, and 3:1, v/v) were examined in the transformation of CC (7.5 wt%) via co-catalysis with EaCl:Gly–water (40 mL), SO42−/SnO2–HAP (2.0 wt%), and oxalic acid (0.4 wt%) in a reactor (180°C) for 10 min. To examine the performance temperature and reaction time of FF generation, co-catalysis with SO42−/SnO2–HAP (2.0 wt%), EaCl:Gly–water (40 mL; EaCl:Gly–water volumetric ratio 1:2), and oxalic acid (0.4 wt%) in a reactor (160–180°C) for 5–40 min was carried out. After the given residence time at the certain performance temperature, this reactor was placed in an ice-water bath to cool down quickly. The product FF was determined using high-performance liquid chromatography (HPLC). FF yield is defined as follows:
Molecular weights of D-xylose and FF are 150 and 96, respectively. The coefficient for catalyzing xylan in biomass into D-xylose is 0.88.
2.4 Bioamination of FF into FAM
The enzyme gene of ω-transaminase from C. violaceum ATCC 12472 (CV) was amplified. The linearized pET28aDuet-1 and purified CV were transformed into one E. coli DH5α and ligated by T5 exonuclease through an exonuclease-mediated seamless assembly. The obtained fragment was inserted into MCS-1 of pET28aDuet-1 after His-tag to obtain CV-pET28aDuet-1. L-Alanine dehydrogenase (AlaDH, GenBank ID 936557) from B. subtilis 168 was amplified. The fragments of CV and Ala were linked together via an overlap extension PCR. Ribosome binding sites were inserted between CV and AlaDH fragments. The ligated plasmid pET28a–CV–AlaDH was transformed into one E. coli BL21 (DE3). The constructed E. coli CCZU-XLS160 cells expressing CV ω-transaminase and AlaDH were cultured in a terrific broth by a supplement of ampicillin (100 mg/L) in a shaker (37°C; 180 rpm). When OD600 of CCZU-XLS160 cells reached 0.60, isopropyl β-D-1-thiogalactopyranosyl (IPTG) was added to the culture system at 20°C. After incubation for 20 h, CCZU-XLS160 cells were harvested for the biological amination of FF into FAM:
Biomass-derived FF, CCZU-XLS160 cells (0.05 g/mL), and NH4Cl (2 mol NH4Cl/mol FAL) were mixed in EaCl:Gly–water (1:2, v/v; pH 7.5) at 35°C. During the biological transamination, the samples were withdrawn. FF and FAM were quantified using HPLC.
2.5 Analytical methods
Components of CC were measured according to the procedure proposed by Selig et al. (2008). SO42−/SnO2–HAP was captured using FT-IR, XRD, BET, and SEM procedures proposed by Peng et al. (2019). FF and FAM were quantified using Waters-2414 HPLC by using an Aminex HPX-87H column (Bio-Rad Laboratories, Hercules, CA) (Zhang et al., 2019). The mixture was composed of 80 vol% water, 20 vol% CH3OH, and 0.1 wt% trifluoroacetic acid, which was used as an eluent at a flow rate of 0.8 mL/min. FAM was determined at 210 nm, and FF was assayed at 254 nm.
3 Results and discussion
3.1 Characteristics of SO42−/SnO2–HAP
Using hydroxyapatite (HAP) as support, a sulfonated HAP-based heterogeneous catalyst was prepared for transforming CC into FF. Relative to carrier support HAP, SO42−/SnO2–HAP had an enlarged surface area (51.9 m2/g) and decreased pore diameter (10.7 nm) (Table 1). Pore volumes of SO42-/SnO2–HAP were increased to 0.14 cm3/g. SEM illustrated that SO42−/SnO2–HAP had more voids (Figure 1), which caused the increased surface area and pore volume. FT-IR illustrated that the SO42−/SnO2–HAP surface was distinct with a carrier HAP surface (Figure 2A). The bond of ∼ 3,430 cm−1 was related to Si–OH stretching. The bond of ∼ 1,098 cm-1, which was related to asymmetric stretching vibrations of Si–O–Si (Gong et al., 2019), increased after the synthesis of SO42−/SnO2–HAP. The bond of ∼ 1,030 cm−1 was related to S=O (Peng et al., 2019), verifying the occurrence of SO42− on a catalyst. The bond of ∼ 790 cm−1 was related to Si–O–Si bending. XRD indicated that Sn ions modified the carrier HAP structure to some extent (Figure 2B). At 2θ = 20–30o, the SO42−/SnO2–HAP intensity dropped compared to that of carrier HAP.
3.2 Co-catalysis of CC with organic acids and SO42−/SnO2–HAP in water
The catalytic system’s acidity might greatly affect FF generation (Marcotullio and de Jong, 2010). Maleic acid (pKa = 1.92), glyoxalate (pKa = 3.18), fumaric acid (pKa = 3.02), malic acid (pKa = 3.46), citric acid (pKa = 3.13), formic acid (pKa = 3.77), oxalic acid (pKa = 1.25), succinic acid (pKa = 4.21), acetic acid (pKa = 4.76), and propionic acid (pKa = 4.87) (0.5 wt%) were used to assist SO42-/SnO2–HAP in the co-catalysis of CC into FF. The turnover frequency (TOF) of the catalytic reaction and pKa value of organic acids were well fitted into a curve equation (TOF = 74.1 × (pKa)−0.85; R2 = 0.9151) (Figure 3A). In the acidic condition, a lower pKa value of organic acids favored FF formation with a higher TOF (Figure 3A). H+ in the lower pKa value of carboxylic acids was easier to dissociate into water. An increased acidity of the catalytic system would enhance the hydrolysis of hemicelluloses in lignocellulose into pentoses (e.g., D-xylose) and accelerate their dehydration to form FF (Lee and Wu, 2021). The highest TOF reached 6.33 h−1 via the co-catalysis of CC using oxalic acid (pKa = 1.25) and SO42−/SnO2–HAP. Different dosages of oxalic acid (0.1–0.8 wt%) were separately supplemented to the catalytic system for assisting SO42−/SnO2–HAP catalysis of CC within 10 min at 180°C (Figure 3B). Upon raising the oxalic acid dose from 0.1 to 0.4 wt%, FF yields increased from 24.6% to 48.2%. Over 0.4 wt%, FF yields had no significant change. Hence, 0.4 wt% of oxalic acid was utilized as a suitable additive to assist the SO42−/SnO2–HAP catalysis of CC into FF.
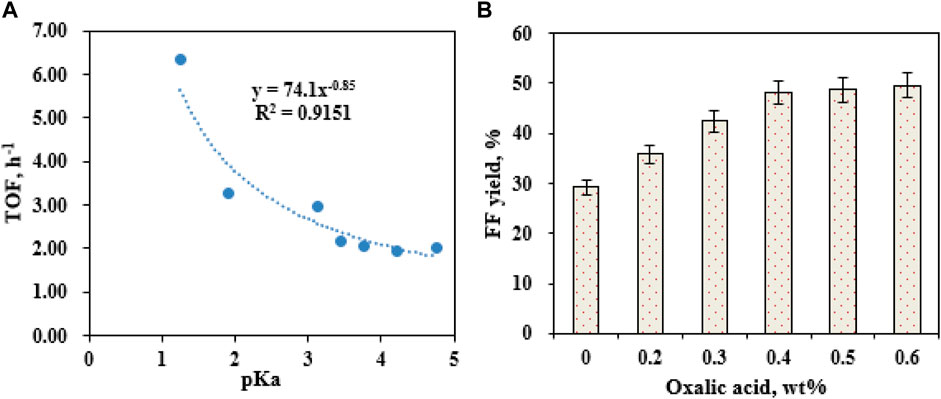
FIGURE 3. Effects of organic acids with different pKa values on the SO42−/SnO2–HAP-catalyzed conversion of CC to FF after 10 min at 180°C (A); effects of the oxalic acid dose (0.1–0.8 wt%) on FF generation after 10 min at 180°C (B).
3.3 Optimization of transforming CC into FF in DES EaCl:Gly–water
In the aqueous catalytic system, the low FF solubility might restrict FF generation, resulting in a decreased FF production (Li et al., 2021). DESs might be used as promising solvents for enhancing FF yields due to their unique properties, which would facilitate the generation of FF and confine undesired FF degradation or cross-polymerization (Pan et al., 2022). Using CC as a feedstock for producing FF, four factors including DES dosage, catalyst dose, performance temperature, and reaction time were examined for the effect of FF formation in DES EaCl:Gly–water.
When the ratio of DES–water is changed, the formed DES−water media might influence the production of FF (Pan et al., 2022). To examine EaCl:Gly dosage on FF generation, four volumetric ratios of EaCl:Gly–water (0:1, 1:3, 1:2, 1:1, 2:1, and 3:1; v/v) were individually utilized as catalytic reaction media (180°C) (Figure 4A). By raising the volumetric ratio of EaCl:Gly–water from 0:1 (EaCl:Gly, 0 vol%) to 1:2 (EaCl:Gly, 33.3 vol%), FF yields increased from 48.2% to 59.3%. Upon increasing the EaCl:Gly–water volumetric ratio from 1:2 to 1:1, FF yields increased slightly. Compared to the aqueous phase system, the merit of the DES–water system is that FF might be extracted into EaCl:Gly in situ quickly. Thus, not only the generation of by-products is confined but also the tedious performance steps for the extraction of FF are also saved. Over 1:1 (v/v), FF yields decreased from 59.3% to 50.1% in EaCl:Gly–water. In view of FF yields and EaCl:Gly dosage, the appropriate EaCl:Gly–water volumetric ratio was chosen as 1:2 (EaCl:Gly, 33.3 vol%). As the EaCl:Gly dose increased in EaCl:Gly–water, excessive solvent loading would reduce the contact opportunity of lignocellulose to catalysts and cause the reduction of FF yields (Li et al., 2021).
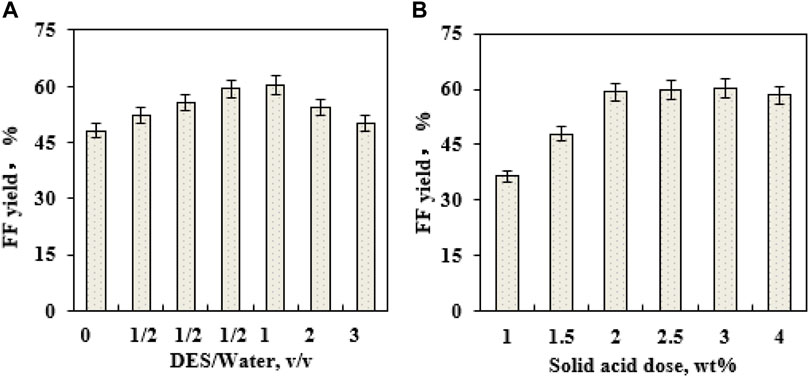
FIGURE 4. Effects of DES EaCl:Gly loading on FF generation (180°C; 10 min) (A); effects of the SO42−/SnO2–HAP dose on FF generation (180°C; 10 min) (B).
During the conversion of biomass into FF with solid acids, catalyst loading had a profound influence on FF production (Peng et al., 2019). It was observed that the loading of SO42−/SnO2–HAP (1–4 wt%) had a crucial effect on FF formation in EaCl:Gly–water (1:2, v/v) by supplementary oxalic acid (0.4 wt%) (Figure 4B). As the SO42−/SnO2–HAP dosage increased from 1 to 2 wt%, FF yields improved from 36.4% to 59.3%, and when SO42−/SnO2–HAP loading exceeded 2.0 wt%, FF yields showed no significant change. Thus, the optimal SO42−/SnO2–HAP dosage was 2.0 wt%. The performance temperature and reaction time were pronounced as vital parameters that affected the production of FF (Zhang et al., 2019). After the transformation of CC was conducted for 5–40 min in a reactor (160–180°C) containing 40 mL EaCl:Gly–water (1:2, v/v), it was found that the highest FF yield (59.3%) was obtained at 180°C after 10 min via co-catalysis with SO42−/SnO2–HAP (2.0 wt%) and oxalic acid (0.4 wt%) (Figure 5). By increasing the reaction time from 5 to 10 min, the FAL yield was increased significantly. Over 10 min, the FAL yield dropped gradually. Sn–sepiolite (3.0 wt%) catalyzed rice straw to FF (42% yield) in water after 20 min at 170°C (Peng et al., 2019). Sn–vermiculite (4.0 wt%) converted reed to FF (55.0 mM; 38.4% yield) in water at 170°C after 20 min (Zhu et al., 2020). Evidently, SO42−/SnO2–HAP could be utilized to catalyze CC into FF with a high yield (59.3%) in EaCl:Gly–water (1:2, v/v) containing oxalic acid (0.4 wt%).
In the EaCl:Gly–water system, lignocellulosic biomass was catalyzed to FF and its derivatives by synergetic catalysis with SO42−/SnO2–HAP and oxalic acid. Water molecules could break the complicated matrix of cellulose–lignin–hemicellulose, which would facilitate the promotion of the dissolution of hemicellulose (Lam et al., 2012). Carboxylic acids as Brönsted catalysts, which are also used as components of DESs, had a good biomass pretreatment ability (Yin et al., 2021). DES EaCl:Gly, which has good biomass pretreatment, could reduce the degradation of hemicellulose that enhanced the selective conversion of hemicellulose (Zhang et al., 2014). The xylan-derived D-xylose was dehydrated and opened in the presence of Cl− and finally closed to FF (Marcotullio and de Jong, 2010). FF could also be obtained via the transformation of xylulose derived from isomerized D-xylose (Choudhary et al., 2011; Li et al., 2014). Glucan-derived glucose was isomerized to fructose, which would be further dehydrated to form 5-HMF and then hydrolyzed to produce HCOOH and levulinic acid (Wang et al., 2017).
3.4 Reuse of SO42−/SnO2–HAP
Reusability is a key indicator in assessing the performance of a heterogeneous catalyst (Gong et al., 2019). In this work, the catalyst SO42−/SnO2–HAP was reused for eight cycles. After each catalytic cycle in EaCl:Gly–water (1:2, v/v), the mixture of biomass residue and SO42-/SnO2–HAP was isolated by filtration and further washed with distilled water three times. Then, this solid mixture was burned in an oven to remove biomass residue. The remaining solid was further sulfonated before starting the next batch. The activity of SO42−/SnO2–HAP was maintained during four consecutive tests (41.7%–59.3%) (Figure 6). FF yields decreased gradually after each batch. From the first to the fourth run, FF yields dropped from 59.3% to 51.6%. From the fifth to the sixth run, a significant decrease in FF yields (41.7%–47.8%) was obtained. After Sn–sepiolite was recovered and reused for six runs, the FF yield decreased from 42% to 35% (Peng et al., 2019). Evidently, SO42-/SnO2–HAP had good stability and reusability. An efficient recovery and reuse process could reduce the operation cost of FF production.
3.5 Valorization of biomass-derived FF to FAM in EaCl:Gly–water
DESs can be utilized as eco-friendly reaction solvents for chemocatalysis and biocatalysis (Ni et al., 2021). In 40 mL EaCl:Gly–water (1:2, v/v), a tandem conversion of biomass into FAM was attempted via a sequential acidified SO42−/SnO2–HAP chemocatalyst and a CCZU-XLS160 cell biocatalyst. Through co-catalysis with SO42−/SnO2–HAP (2.0 wt%) and oxalic acid (0.4 wt%), corncob (CC), sugarcane bagasse (SB), rice straw (RS), and reed leaf (RL) (3.0 g, 75 g/L) could be catalyzed into 114.9, 79.6, 74.5, and 50.8 mM FF at 180°C after 10 min (Table 2), respectively. FF yields were obtained as follows: Yield(CC) = 59.3% > Yield(SB) = 47.3% > Yield(RS) = 45.2% > Yield(RL) = 42.4%. Various types of agro- and forest-wastes could be used to produce FF (Guenic et al., 2015; Gong et al., 2019; Lee and Wu, 2021). In this work, different types of biomass samples with different contents of xylan were used as feedstock for the production of FF. Overall, CC was a good feedstock for FF production due to the high xylan content (34.1 wt%) and FF yield (59.3%).
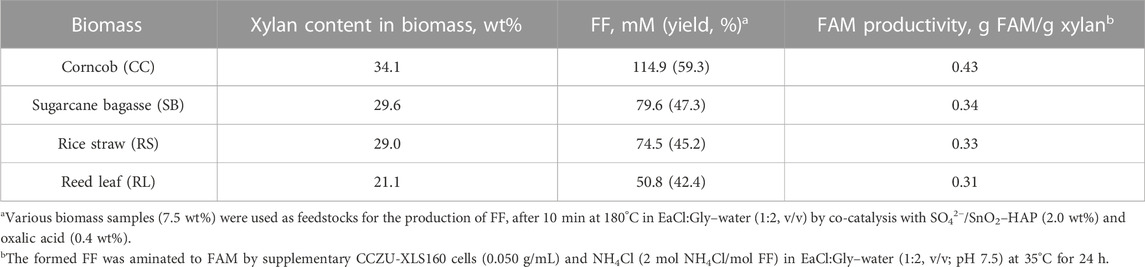
TABLE 2. Examination of the chemoenzymatic conversion potency toward different biomasses in EaCl:Gly–water (1:2, v/v).
The formed FF liquor, which was adjusted to pH 7.5, could be aminated into FAM by supplementary CCZU-XLS160 cells (0.050 g/mL) and NH4Cl (2 mol NH4Cl/mol FF) in EaCl:Gly–water (1:2, v/v). Time courses for the biotransformation of dilute CC-derived FF (110.0 mM) were monitored. By increasing the bioreaction time from 0 to 12 h, FF concentrations decreased quickly (Figure 7). After biological amination for 6 and 12 h, FAM concentrations reached 78.7 and 99.0 mM, respectively. When biological amination was carried out for 24 h, FF was fully transformed into FAM, achieving the productivity of 0.43 g FAM per g xylan. After biological transformations of SB-, RS-, and RL-derived FF for 24 h, the yields of FAM reached >99%, with the productivity of 0.31–0.34 g FAM per g xylan (Table 2). FF could be prepared from xylan in biomass via hydrolysis, dehydration, and cyclization reaction (Lee and Wu, 2021; Gong et al., 2022). Hence, the titer of FF derived from lignocellulosic biomass was mainly based on the xylan content in biomass. The biomass-derived FF could be wholly aminated to FAM by CCZU-XLS160 cells. It was observed that the generated FAM titer was largely determined by the xylan content of biomass in the tandem reaction with acidified SO42−/SnO2–HAP and CCZU-XLS160 cells. Hence, CC could produce high productivity of FAM via a chemoenzymatic approach in EaCl:Gly–water (1:2, v/v).
Lignocellulosic biomass is an available, inexpensive, and renewable source, which has been widely used for the production of value-added chemicals (Mika et al., 2018; Lee and Wu, 2021). FF is a key bio-based chemical. One of its common preparation methods is conducted via catalysis by using metal salts, mineral acids, and organic acids as homogeneous catalysts. Recently, heterogeneous catalysts are regarded as environmentally friendly catalysts, which can be easily recovered and reused as compared to some homogeneous ones (Gong et al., 2019). It is known that the establishment of an efficient valorization process would achieve high concentrations of FF (Weingarten et al., 2010; Metkar et al., 2015). DESs have gained considerable interest as reaction solvents for FF production due to their low toxicity, good reusability, high thermostability, and ease of preparation (Xu et al., 2020). In this work, biomass samples (7.5 wt%) were utilized as feedstocks for preparing FF after 10 min in EaCl:Gly–water (1:2, v/v; 180°C) by co-catalysis with SO42−/SnO2–HAP (2.0 wt%) and oxalic acid (0.4 wt%). Furthermore, biological amination of the prepared FF with whole-cell of CCZU-XLS160 could be used for the efficient production of FAM under an ambient performance condition. Tandem catalysis by bridging chemocatalysis and biocatalysis was constructed for the efficient and sustainable valorization of biomass into FAM. In the future, it is of great interest to establish a cost-effective catalytic process to obtain high FF concentration and thus further improve FAM productivity with high ω-transaminase activity.
4 Conclusion
To summarize, the establishment of an efficient chemoenzymatic approach for converting abundant, cheap, and renewable lignocellulosic biomass into value-added furans can be used as a sustainable strategy in the biorefinery process. In an eco-friendly reaction system, various types of lignocellulosic biomasses can be converted to FAM via sequential catalyses with solid acid catalysts and transaminase biocatalysts.
Using hydroxyapatite (HAP) as a carrier, solid acid catalyst SO42−/SnO2–HAP was prepared for catalyzing xylan-rich biomass in this work. Organic acids were used to assist newly synthesized SO42−/SnO2–HAP for FF production. It was found that oxalic acid (pKa = 1.25; 0.4 wt%) plus SO42−/SnO2–HAP (2.0 wt%) gave the highest FF yield in EaCl:Gly–water (1:2, v/v) at 180°C after 10 min. SO42−/SnO2–HAP had a good reusability performance, which could be reused for six runs.
FF liquors, which were obtained from different biomasses by co-catalyses with oxalic acid and SO42−/SnO2–HAP in EaCl:Gly–water, could be fully aminated into FAM with the yield >99% with CCZU-XLS160 cells within 24 h. This established chemoenzymatic conversion strategy could be utilized in the valorization of renewable lignocellulosic biomass to valuable furan-based compounds in the eco-friendly EaCl:Gly–water system.
Data availability statement
The original contributions presented in the study are included in the article/Supplementary Material; further inquiries can be directed to the corresponding authors.
Author contributions
WH: conceptualization, methodology, and writing original draft; Y-CH and JY: data curation, software, supervision, review, and revision of the manuscript. All authors have read and agreed to the published version of the manuscript.
Conflict of interest
The authors declare that the research was conducted in the absence of any commercial or financial relationships that could be construed as a potential conflict of interest.
Publisher’s note
All claims expressed in this article are solely those of the authors and do not necessarily represent those of their affiliated organizations, or those of the publisher, the editors, and the reviewers. Any product that may be evaluated in this article, or claim that may be made by its manufacturer, is not guaranteed or endorsed by the publisher.
References
Agirrezabal-Telleria, I., Gandarias, I., and Arias, P.-L. (2014). Heterogeneous acid-catalysts for the production of furan-derived compounds (furfural and hydroxymethylfurfural) from renewable carbohydrates: A review. Catal. Today 234, 42–58. doi:10.1016/j.cattod.2013.11.027
Bozell, J.-J., and Petersen, G.-R. (2010). Technology development for the production of biobased products from biorefinery carbohydrates-the US Department of Energy's "Top 10" revisited. Green Chem. 12, 539–554. doi:10.1039/b922014c
Cai, C.-M., Zhang, T.-Y., Kumar, R., and Wyman, C.-E. (2014). Integrated furfural production as a renewable fuel and chemical platform from lignocellulosic biomass. J. Chem. Technol. Biotechnol. 89, 2–10. doi:10.1002/jctb.4168
Chen, Z., and Wan, C.-X. (2019). A novel deep eutectic solvent/acetone biphasic system for high-yield furfural production. Bioresour. Technol. Rep. 8, 100318. doi:10.1016/j.biteb.2019.100318
Choudhary, V., Pinar, A.-B., Sandler, S.-I., Vlachos, D.-G., and Lobo, R.-F. (2011). Xylose isomerization to xylulose and its dehydration to furfural in aqueous media. ACS Catal. 1, 1724–1728. doi:10.1021/cs200461t
Delidovich, I., Hausoul, P.-J.-C., Deng, L., Pfutzenreuter, R., Rose, M., and Palkovits, R. (2016). Alternative monomers based on lignocellulose and their use for polymer production. Chem. Rev. 116, 1540–1599. doi:10.1021/acs.chemrev.5b00354
Di, J.-H., Gong, L., Yang, D., He, Y.-C., Tang, Z.-Y., and Ma, C.-L. (2021). Enhanced conversion of biomass to furfurylamine with high productivity by tandem catalysis with sulfonated perlite and ω-transaminase whole-cell biocatalyst. J. Biotechnol. 334, 26–34. doi:10.1016/j.jbiotec.2021.05.003
Dong, C.-L., Wang, H.-T., Du, H.-C., Peng, J.-B., Cai, Y., Guo, S., et al. (2020). Ru/HZSM-5 as an efficient and recyclable catalyst for reductive amination of furfural to furfurylamine. Mol. Catal. 482, 110755. doi:10.1016/j.mcat.2019.110755
Douthwaite, M., Huang, X.-Y., Iqbal, S., Miedziak, P.-J., Brett, G.-L., Kondrat, S.-A., et al. (2017). The controlled catalytic oxidation of furfural to furoic acid using AuPd/Mg(OH)(2). Catal. Sci. Technol. 7, 5284–5293. doi:10.1039/C7CY01025G
Enslow, K.-R., and Bell, A.-T. (2015). The role of metal halides in enhancing the dehydration of xylose to furfural. ChemCatChem 7, 479–489. doi:10.1002/cctc.201402842
Feng, L., Bo, C., Rui, M., Liang, J., Hua, S., and Song, H. (2016). Performance of Cu/TiO2-SiO2 catalysts in hydrogenation of furfural to furfuryl alcohol. Can. J. Chem. Eng. 94, 1368–1374. doi:10.1002/cjce.22503
Garcia-Sancho, C., Sadaba, I., Moreno-Tost, R., Merida-Robles, J., Santamaria-Gonzalez, J., Lopez-Granados, M., et al. (2013). Dehydration of xylose to furfural over MCM-41-supported niobium-oxide catalysts. ChemSusChem 6, 635–642. doi:10.1002/cssc.201200881
Gong, L., Xu, Z.-Y., Dong, J.-J., Li, H., Han, R.-Z., Xu, G.-C., et al. (2019). Composite coal fly ash solid acid catalyst in synergy with chloride for biphasic preparation of furfural from corn stover hydrolysate. Bioresour. Technol. 293, 122065. doi:10.1016/j.biortech.2019.122065
Gong, L., Zha, J., Pan, L., Ma, C., and He, Y.-C. (2022). Highly efficient conversion of sunflower stalk-hydrolysate to furfural by sunflower stalk residue-derived carbonaceous solid acid in deep eutectic solvent/organic solvent system. Bioresour. Technol. 351, 126945. doi:10.1016/j.biortech.2022.126945
Guenic, S.-L., Delbecq, F., Ceballos, C., and Len, C. (2015). Microwave-assisted dehydration of D-xylose into furfural by diluted inexpensive inorganic salts solution in a biphasic system. J. Mol. Catal. A Chem. 410, 1–7. doi:10.1016/j.molcata.2015.08.019
He, Y.-C., Ding, Y., Ma, C.-L., Di, J.-H., Jiang, C.-L., and Li, A.-T. (2017a). One-pot conversion of biomass-derived xylose to furfuralcohol by a chemo-enzymatic sequential acid-catalyzed dehydration and bioreduction. Green Chem. 19, 3844–3850. doi:10.1039/c7gc01256j
He, Y.-C., Jiang, C.-X., Chong, G.-G., Di, J.-H., Wu, Y.-F., Wang, B.-Q., et al. (2017b). Chemical-enzymatic conversion of corncob-derived xylose to furfuralcohol by the tandem catalysis with SO42−/SnO2-kaoline and E coli CCZU-T15 cells in toluene–water media. Bioresour. Technol. 245, 841–849. doi:10.1016/j.biortech.2017.08.219
Himmel, M.-E., Ding, S.-Y., Johnson, D.-K., Adney, W.-S., Nimlo, M.-R., Brady, J.-W., et al. (2007). Biomass recalcitrance: Engineering plants and enzymes for biofuels production. Science 315, 804–807. doi:10.1126/science.1137016
Hu, D.-X., Ju, X., Li, L.-Z., Hu, C.-Y., Yan, L.-S., Wu, T.-Y., et al. (2014). Improved in situ saccharification of cellulose pretreated by dimethyl sulfoxide/ionic liquid using cellulase from a newly isolated Paenibacillus sp. LLZ1. Bioresour. Technol. 201, 8–14. doi:10.1016/j.biortech.2015.11.039
Kaiprommarat, S., Kongparakul, S., Reubroycharoen, P., Guan, G.-Q., and Samar, C. (2016). Highly efficient sulfonic MCM-41 catalyst for furfural production: Furan-based biofuel agent. Fuel 174, 189–196. doi:10.1016/j.fuel.2016.02.011
Lam, E., Chong, J.-H., Majid, E., Liu, Y., Hrapovic, S., Leung, A.-C.-W., et al. (2012). Carbocatalytic dehydration of xylose to furfural in water. Carbon 50, 1033–1043. doi:10.1016/j.carbon.2011.10.007
Lange, J., Heide, E.-V.-D., Buijtenen, J.-V., and Price, R. (2012). Furfural–a promising platform for lignocellulosic biofuels. ChemSusChem 4, 150–166. doi:10.1002/cssc.201100648
Lee, C.-B.-T.-L., and Wu, T.-Y. (2021). A review on solvent systems for furfural production from lignocellulosic biomass. Renew. Sust. Energ Rev. 137, 110172. doi:10.1016/j.rser.2020.110172
Li, A.-L., Hou, X.-D., Lin, K.-P., Zhang, X., and Fu, M.-H. (2018). Rice straw pretreatment using deep eutectic solvents with different constituents molar ratios: Biomass fractionation, polysaccharides enzymatic digestion and solvent reuse. J. Biosci. Bioeng. 126, 346–354. doi:10.1016/j.jbiosc.2018.03.011
Li, H.-L., Deng, A.-J., Ren, J.-L., Liu, C.-Y., Lu, Q., Zhong, L.-J., et al. (2014). Catalytic hydrothermal pretreatment of corncob into xylose and furfural via solid acid catalyst. Bioresour. Technol. 158, 313–320. doi:10.1016/j.biortech.2014.02.059
Li, Q., Hu, Y., Tao, Y.-Y., Zhang, P.-Q., Ma, C.-L., Zhou, Y.-J., et al. (2021). Improving biocatalytic synthesis of furfuryl alcohol by effective conversion of D-xylose into furfural with tin-loaded sulfonated carbon nanotube in cyclopentylmethyl ether-water media. Catal. Lett. 148, 3189–3196. doi:10.1007/s10562-021-03570-3
Li, X.-Y., Yang, J.-X., Xu, R., Lu, L.-F., Kong, F.-K., Liang, M., et al. (2019). Kinetic study of furfural production from Eucalyptus sawdust using H-SAPO-34 as solid Brønsted acid and Lewis acid catalysts in biomass-derived solvents. Ind. Crop Prod. 135, 196–205. doi:10.1016/j.indcrop.2019.04.047
Ma, J., Li, W., Guan, S., Liu, Q., Li, Q., Zhu, C., et al. (2019). Efficient catalytic conversion of corn stalk and xylose into furfural over sulfonated graphene in γ-valerolactone. RSC Adv. 9, 10569–10577. doi:10.1039/c9ra01411j
Ma, Z., Liao, Z., Ma, C., He, Y., Gong, C.-J., and Yu, X. (2020). Chemoenzymatic conversion of sorghum durra stalk into furoic acid by a sequential microwave-assisted solid acid conversion and immobilized whole-cells biocatalysis. Bioresour. Technol. 311, 123474. doi:10.1016/j.biortech.2020.123474
Marcotullio, G., and de Jong, W. (2010). Chloride ions enhance furfural formation from D-xylose in dilute aqueous acidic solutions. Green Chem. 12, 1739–1746. doi:10.1039/b927424c
Metkar, P.-S., Till, E.-J., Corbin, D.-R., Pereira, C.-J., Hutchenson, K.-W., and Sengupta, S.-K. (2015). Reactive distillation process for the production of furfural using solid acid catalysts. Green Chem. 17, 1453–1466. doi:10.1039/c4gc01912a
Mika, L.-T., Csefalvay, E., and Nemeth, A. (2018). Catalytic conversion of carbohydrates to initial platform chemicals: Chemistry and sustainability. Chem. Rev. 118, 505–613. doi:10.1021/acs.chemrev.7b00395
Möller, M., and Schröder, U. (2013). Hydrothermal production of furfural from xylose and xylan as model compounds for hemicelluloses. RSC Adv. 3, 22253–22260. doi:10.1039/c3ra43108h
Morais, E.-S., Freire, M.-G., Freire, C., Coutinho, J., and Silvestre, A. (2020). Enhanced conversion of xylan into furfural using acidic deep eutectic solvents with dual solvent and catalyst behavior. ChemSusChem 13, 784–790. doi:10.1002/cssc.201902848
Ni, J., Li, Q., Gong, L., Liao, X.-L., Zhang, Z.-J., Ma, C., et al. (2021). Highly efficient chemoenzymatic cascade catalysis of biomass into furfurylamine by a heterogeneous shrimp shell-based chemocatalyst and an ω-transaminase biocatalyst in deep eutectic solvent–water. ACS Sustain Chem. Eng. 9, 13084–13095. doi:10.1021/acssuschemeng.1c05109
Pan, L., Li, Q., Tao, Y., Ma, C., Chai, H., Ai, Y., et al. (2022). An efficient chemoenzymatic strategy for valorisation of corncob to furfuryl alcohol in CA:Betaine-water. Ind. Crop. Prod. 186, 115203. doi:10.1016/j.indcrop.2022.115203
Peleteiro, S., Garrote, G., Santos, V., and Parajó, J.-C. (2014). Furan manufacture from softwood hemicelluloses by aqueous fractionation and further reaction in a catalyzed ionic liquid: A biorefinery approach. J. Clean. Prod. 76, 200–203. doi:10.1016/j.jclepro.2014.04.034
Peng, B., Ma, C.-L., Zhang, P.-Q., Wu, C.-Q., Wang, Z.-W., Li, A.-T., et al. (2019). An effective hybrid strategy for converting rice straw to furoic acid by tandem catalysis via Sn-sepiolite combined with recombinant E coli whole cells harboring horse liver alcohol dehydrogenase. Green Chem. 21, 5914–5923. doi:10.1039/C9GC02499A
Pholjaroen, B., Li, N., Wang, Z., Wang, A., and Zhang, T. (2013). Dehydration of xylose to furfural over niobium phosphate catalyst in biphasic solvent system. J. Energy Chem. 22, 826–832. doi:10.1016/S2095-4956(14)60260-6
Qiu, M., Guo, T., Li, D., and Qi, X. (2020). Highly efficient catalytic transfer hydrogenation of biomass-derived furfural to furfuryl alcohol using UiO-66 without metal catalysts. Appl. Catal. A Gen. 602, 117719. doi:10.1016/j.apcata.2020.117719
Riva, L., Fiorati, A., and Punta, C. (2021). Synthesis and application of cellulose-polyethyleneimine composites and nanocomposites: A concise review. Materials 14, 473. doi:10.3390/ma14030473
Rong, C.-G., Ding, X.-F., Zhu, Y.-C., Li, Y., Wang, L.-L., Qu, Y.-N., et al. (2012). Production of furfural from xylose at atmospheric pressure by dilute sulfuric acid and inorganic salts. Carbohyd Res. 350, 77–80. doi:10.1016/j.carres.2011.11.023
Selig, M., Weiss, N., and Ji, Y. (2008). Enzymatic saccharification of lignocellulosic biomass. Golden, Golden, Colorado: National Renewable Energy Laboratory.
Sweygers, N., Harrer, J., Dewil, R., and Appels, L. (2018). A microwave-assisted process for the in-situ production of 5-hydroxymethylfurfural and furfural from lignocellulosic polysaccharides in a biphasic reaction system. J. Clean. Prod. 187, 1014–1024. doi:10.1016/j.jclepro.2018.03.204
Tau, L.-Y., Nurabiyiah, M., and Nor, M.-M.-Y. (2016). Furfural production from oil Palm biomass using a biomass-derived supercritical ethanol solvent and formic acid catalyst. Procedia Eng. 148, 392–400. doi:10.1016/j.proeng.2016.06.495
Wang, C., Zhang, L., Zhou, T., Chen, J., and Xu, F. (2017). Synergy of Lewis and Brønsted acids on catalytic hydrothermal decomposition of carbohydrates and corncob acid hydrolysis residues to 5-hydroxymethylfurfural. Sci. Rep. 7, 40908–40909. doi:10.1038/srep40908
Weingarten, R., Cho, J., Conner, J.-W.-C., and Huber, W.-G. (2010). Kinetics of furfural production by dehydration of xylose in a biphasic reactor with microwave heating. Green Chem. 12, 1423–1429. doi:10.1039/c003459b
Widsten, P., Murton, K., and West, M. (2018). Production of 5-hydroxymethylfurfural and furfural from a mixed saccharide feedstock in biphasic solvent systems. Ind. Crop Prod. 119, 237–242. doi:10.1016/j.indcrop.2018.04.032
Xu, G., Li, H., Xing, W., Gong, L., Dong, J., and Ni, Y. (2020). Facilely reducing recalcitrance of lignocellulosic biomass by a newly developed ethylamine-based deep eutectic solvent for biobutanol fermentation. Biotechnol. Biofuels 13, 166. doi:10.1186/s13068-020-01806-9
Xu, J., Hou, H., Liu, B., and Hu, J. (2017). The integration of different pretreatments and ionic liquid processing of eucalyptus: Hemicellulosic products and regenerated cellulose fibers. Ind. Crop Prod. 101, 11–20. doi:10.1016/j.indcrop.2017.02.038
Yin, X., Wei, L., Pan, X., Liu, C., and Wang, K. (2021). The pretreatment of lignocelluloses with green solvent as biorefinery preprocess: A minor review. Front. Plant Sci. 12, 670061–670076. doi:10.3389/fpls.2021.670061
Zang, H., and Chen, E. Y. X. (2015). Organocatalytic upgrading of furfural and 5-hydroxymethyl furfural to C10 and C12 furoins with quantitative yield and atom-efficiency. Int. J. Mol. Sci. 16, 7143–7158. doi:10.3390/ijms16047143
Zhang, L.-X., Yu, H., Yu, H. B., Chen, Z., and Yang, L. (2014). Conversion of xylose and xylan into furfural in biorenewable choline chloride–oxalic acid deep eutectic solvent with the addition of metal chloride. Chin. Chem. Lett. 25, 1132–1136. doi:10.1016/j.cclet.2014.03.029
Zhang, P., Liao, X., Ma, C., Li, Q., and He, Y. (2019). Chemoenzymatic conversion of corncob to furfurylamine via tandem catalysis with tin-based solid acid and transaminase biocatalyst. ACS Sustain Chem. Eng. 7 (21), 17636–17642. doi:10.1021/acssuschemeng.9b03510
Zheng, Z.-J., Xu, Q., Tan, H., Zhou, F., and Ouyang, J. (2020). Selective biosynthesis of furoic acid from furfural by Pseudomonas Putida and identification of molybdate transporter involvement in furfural oxidation. Front. Chem. 8, 587456. doi:10.3389/fchem.2020.587456
Zhu, X., Ma, C.-L., Xu, J.-X., Xu, J.-X., and He, Y.-C. (2020). Sulfonated vermiculite-mediated catalysis of reed (phragmites communis) into furfural for enhancing the biosynthesis of 2-furoic acid with a dehydrogenase biocatalyst in a one-pot manner. Energ Fuel 34, 14573–14580. doi:10.1021/acs.energyfuels.0c02707
Keywords: lignocellulose, furfural, furfurylamine, SO42−/SnO2–HAP, deep eutectic solvent
Citation: He W, He Y-C and Ye J (2023) Efficient synthesis of furfurylamine from biomass via a hybrid strategy in an EaCl:Gly–water medium. Front. Bioeng. Biotechnol. 11:1144787. doi: 10.3389/fbioe.2023.1144787
Received: 15 January 2023; Accepted: 17 February 2023;
Published: 16 March 2023.
Edited by:
Chen Huang, Chinese Academy of Forestry, ChinaReviewed by:
Liangzhi Li, Suzhou University of Science and Technology, ChinaZhi-Jun Zhang, East China University of Science and Technology, China
Copyright © 2023 He, He and Ye. This is an open-access article distributed under the terms of the Creative Commons Attribution License (CC BY). The use, distribution or reproduction in other forums is permitted, provided the original author(s) and the copyright owner(s) are credited and that the original publication in this journal is cited, in accordance with accepted academic practice. No use, distribution or reproduction is permitted which does not comply with these terms.
*Correspondence: Yu-Cai He, aGV5dWNhaTIwMDFAMTYzLmNvbQ==; Jianren Ye, anJ5ZUBuamZ1LmVkdS5jbg==