- 1Department of Spine Surgery, First Hospital of Jilin University, Changchun, China
- 2Department of Orthopedics, Affiliated Hospital of Beihua University, Jilin, China
- 3Department of Gynecology, Affiliated Hospital of Beihua University, Jilin, China
- 4Department of Orthopedics, Baicheng Central Hospital, Baicheng, China
Bones are important for maintaining motor function and providing support for internal organs. Bone diseases can impose a heavy burden on individuals and society. Although bone has a certain ability to repair itself, it is often difficult to repair itself alone when faced with critical-sized defects, such as severe trauma, surgery, or tumors. There is still a heavy reliance on metal implants and autologous or allogeneic bone grafts for bone defects that are difficult to self-heal. However, these grafts still have problems that are difficult to circumvent, such as metal implants that may require secondary surgical removal, lack of bone graft donors, and immune rejection. The rapid advance in tissue engineering and a better comprehension of the physiological mechanisms of bone regeneration have led to a new focus on promoting endogenous bone self-regeneration through the use of biomaterials as the medium. Although bone regeneration involves a variety of cells and signaling factors, and these complex signaling pathways and mechanisms of interaction have not been fully understood, macrophages undoubtedly play an essential role in bone regeneration. This review summarizes the design strategies that need to be considered for biomaterials to regulate macrophage function in bone regeneration. Subsequently, this review provides an overview of therapeutic strategies for biomaterials to intervene in all stages of bone regeneration by regulating macrophages.
1 Introduction
Bone defects are prevalent clinical manifestations, usually caused by trauma, surgery, and tumor. It can lead to pain, local dysfunction, and even death. Like most tissues in the body, bone tissue has a certain ability to repair and renew itself. Bone can heal well without scarring in the face of some small bone defects, but when the defect area exceeds the critical size or is combined with aging, infection, or metabolic disease, the repair of bone tissue may end up with non-union, mal-union, or delayed union. Previous epidemiological studies suggested the rate of impaired bone healing at 5%–10% (Newman et al., 2021). Although this number may decrease in recent years due to the development of medical technology, it still needs to be taken seriously in low-income countries, elderly, and patients with severe injuries (Ekegren et al., 2018). The financial burden of impaired bone healing is also heavy. In the United Kingdom, the cost of treatment of non-union is approximately £7,000–79,000 per person (Kanakaris and Giannoudis, 2007; Dahabreh et al., 2009; Ekegren et al., 2018). A previous survey in the United States indicated that the treatment cost of tibia shaft fracture patients with non-union was almost twice as much as patients without non-union, and the difference may be even greater when long-term medication and care costs were taken into account (Antonova et al., 2013).
At present, metal implants and autologous or allogeneic bone grafts are still the main clinical methods for the treatment of impaired bone healing. In the United States and Europe, more than half a million patients undergo bone defect repair surgery each year at a total cost of more than US $3 billion (Haugen et al., 2019). In Germany, more than 70,000 autologous or allogeneic bone grafts are performed each year, and autologous bone grafts account for more than 50% (Rupp et al., 2022). Although these treatments appear to be well-established and commercially available today, there are still many problems to be faced. Immune rejection of metallic implants has been effectively controlled in recent years due to continuous improvements in composition, but the different mechanical properties of metallic materials and natural bone tissue tend to lead to stress shielding, resulting in weakening of the surrounding healthy bone tissue (Pacheco, 2019; Liverani et al., 2021). In addition, the secondary removal of metal implants elevates the risk of surgery-related complications, such as anesthesia accidents, infections, and bleeding. Autologous bone graft can well circumvent the rejection problems caused by grafts and has similar mechanical properties and microstructure to normal bone tissue, so it is an excellent material for the treatment of impaired bone healing. Bone donors are primarily derived from bones in non-weight-bearing areas such as the iliac bone, which places a limit on the volume of donors that can be obtained (Schmidt, 2021). In addition, autologous bone grafts may also cause irreparable damage to the donor site. Allogeneic bone grafts can address the need for a large volume of donors to some extent, but this is accompanied by a higher risk of immune rejection and a stable source of donors that is still difficult to address (Penack et al., 2020; Potyondy et al., 2021). With the increasing understanding of bone regeneration mechanisms, the realization of bone regeneration through enhancing the endogenous repair ability of bone tissue has attracted increasing attention (Zhu et al., 2022a; Liu et al., 2022). Macrophage plays a crucial role in the complex regulatory network of bone regeneration composed of a variety of cells and factors, and is a good target for accelerating bone regeneration. The role of macrophage can be simply summarized as “a sweeper, a mediator and a instructor” (Niu et al., 2021).
As a subgroup of immune system cells, macrophages exist widely in various organs of the body, and their important role in tissue repair has been proved by a large number of previous studies (Wynn and Vannella, 2016; Chazaud, 2020). Considering the complex relationship between immune mechanism and bone regeneration, the function of macrophages in bone regeneration is not only manifested in phagocytes (Michalski and McCauley, 2017). Macrophages have a high degree of plasticity and perform different functions under receiving different stimuli (Locati et al., 2020). Macrophages also play a critical role in maintaining the stability of the extracellular matrix (ECM), regulating inflammation levels, immune surveillance, promoting osteoblast proliferation and differentiation, regulating bone formation and resorption, and promoting neovascularization (Lowery and Rosen, 2018; Schlundt et al., 2018; Schlundt et al., 2021). In the complex intercellular and intracellular cascade reactions, the transformation of macrophage phenotype M1/M2 has attracted particular attention. In general, the M1 phenotype showed pro-inflammatory effect and the M2 phenotype showed anti-inflammatory effect. In fact, most of the current studies also focuses on the regulation of macrophage polarization to accelerate bone regeneration. The development of biomaterials provides good tools for interfering with macrophages. In addition, well-designed biomaterials can also provide good local mechanical support, controlled drug release, and bionic cell survival environment (Zhao et al., 2021d). In this review, we summarize the important parameters that need to be kept in mind when designing biomaterials to regulate macrophage activity. Subsequently, we review the therapeutic substance for biomaterial regulation of macrophage and prospected future directions.
2 Bone structure and mechanism of bone healing
There are 206 bones in normal adult body, and these bones can be categorized into five categories according to their shape and function: long bone, short bone, flat bone, sesamoid bone, and irregular bone. At the early stage of embryonic development and bone regeneration, woven bone is formed first, which is softer than normal bone and has better elasticity. Later, the woven bone is replaced by mature lamellar bone (Shapiro and Wu, 2019). Lamellar bone is composed of compact bone, cancellous bone, periosteum, and bone marrow. The outer compact bone and the inner cancellous bone are the main components of bone (Wawrzyniak and Balawender, 2022). As the name implies, the compact bone consists of tightly arranged bone plates that are highly resistant to compression and torsion, with Haversian system containing blood vessels and nerves inside. The cancellous bone is composed of honeycomb-like trabeculae with large pores. The arrangement of trabecular bone is closely related to bone stress, which makes the bone achieve good strength with less material (Florencio-Silva et al., 2015; Wawrzyniak and Balawender, 2022). Bone marrow is filled with bone trabeculae and bone marrow cavity of long bones and has hematopoietic function (Pinho and Frenette, 2019; Lucas, 2021). The out surface of bone is covered with dense connective tissue, called periosteum, which has the role of protecting, nourishing, and renewing the bone tissue. Correspondingly, trabeculae and bone marrow cavities are also covered with periosteum, called endosteum, which has osteogenic and osteoclast functions (Wang et al., 2017b; Zhang et al., 2022b).
Depending on the severity of the injury, there are two mechanisms of bone regeneration; intramembranous ossification is seen in the case of minor injury and mechanical stability, and endochondral ossification is seen in the case of severe injury (Salhotra et al., 2020; Marcucio et al., 2023). Intramembranous osteogenesis does not require cartilage as an intermediate product and transitions directly from the initial inflammatory stage to the synthesis and metabolism of bone. Mesenchymal stem cells (MSCs) in the ossification site differentiate into osteoblasts, which produce ECM rich in type I collagen, and with further mineralization of the matrix, the osteoblasts encapsulated in it differentiate into mature osteocytes. At the same time, osteoclasts derived from the hematopoietic monocyte-macrophage system continuously absorb the matrix to ensure the shape and function (Song, 2022). Endochondral ossification requires cartilage tissue as the prerequisite for new bone matrix (Berendsen and Olsen, 2015). The regeneration process can be seperated into four stages: hematoma and inflammation, soft callus formation, hard callus formation, and remodeling (Niu et al., 2021) (Figure 1). In the hours after injury, local bleeding solidifies in situ to form a hematoma to prevent further bleeding. In addition, the hematoma provides multiple growth factors to start the subsequent cascade reactions. The necrotic tissue triggers a local inflammatory response in which a variety of inflammatory cells, including macrophages, are recruited, after which the hematoma gradually transformed into granulation tissue (Claes et al., 2012; Newman et al., 2021). Subsequently, chondrogenic cells appear at the fracture site to form hyaline cartilage, and osteoblasts absorb the calcified cartilage to produce new woven bone. Typically, soft callus is formed on the 14th day after the injury. Concurrently, a large number of new blood vessels support the local substance metabolism and the subsequent cascade reaction. As the cartilage is further resorbed and the woven bone is gradually replaced by the lamellar bone, soft callus is gradually replaced by hard callus with higher mechanical strength. In the final bone remodeling stage, with a series of resorption/remodeling events led by osteoclasts and osteoblasts, the structure and function of bone tissue can be precisely regenerated (Einhorn and Gerstenfeld, 2015; Marcucio et al., 2023). The role of macrophages can be found in all stages of bone healing. In the initial stage of inflammation, macrophages are not only responsible for the phagocytosis of tissue debris and foreign pathogens, but also play an important role in promoting angiogenesis and guiding the recruitment and differentiation of MSCs (Niu et al., 2021). In the subsequent callus and remodeling stages, macrophages not only directly differentiate into osteoclasts, but also secrete a variety of factors to regulate the differentiation of osteoblasts and the extracellular matrix microenvironment (Wang et al., 2013; Rucci and Teti, 2016; Xie et al., 2020). Notably, despite the strict chronological order of these phases, there is no separated temporal cut-off point between the phases, and there is a degree of temporal overlap between each phase (Maruyama et al., 2020). This healing characteristic also poses new challenges for promoting bone regeneration.
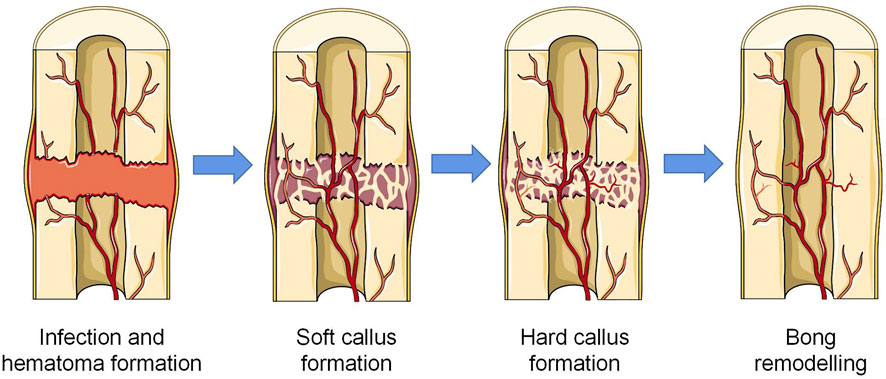
FIGURE 1. Four stages of endochondral osteogenesis (The Figure was partly generated using Servier Medical Art, provided by Servier, licensed under a Creative Commons Attribution 3.0 unported license).
3 Biomaterial design and macrophage regulation
3.1 Material design strategy
Although autologous bone grafting is still the gold standard for the treatment of impaired bone healing, the continuous development of tissue engineering can further achieve the regulation of cell activities or local microenvironments based on imitating the structure and function of natural bone tissue (Dimitriou et al., 2011). The use of biomaterials to achieve the multi-functionalization of scaffolds has attracted wide attention in recent years. The materials of scaffolds mainly include metal, ceramic, bio-glass, and polymer (Table.1). In summary, it is difficult to have a single material composition scaffold that can meet the ideal bone regeneration requirements, such as metal materials may lead to stress shielding, difficult degradation of ceramics and bio-glass, and the potential cytotoxicity of polymers (Gracis et al., 2015; Janaszewska et al., 2019; Raffa et al., 2021). Composite scaffolds that combine different kinds of components are expected to solve the performance deficiencies of single materials, while also further increasing the difficulty of scaffold system design and fabrication. For scaffolds to effectively promote bone regeneration by regulating macrophage activity, some properties need to be carefully designed.
3.1.1 Parameters to be considered as implants
As biomaterial scaffolds are in vivo implants that need to be retained in vivo for a period of time, some conventional performance parameters need to be considered first (Table.2). Safety indicators such as biocompatibility, cytotoxicity, and immunogenicity should be the key consideration (Marin et al., 2020; Zhu et al., 2020; Diemer et al., 2021). It is a prerequisite for biomaterials to function without causing local or even systemic adverse reactions in vivo. Although many materials have undergone rigorous safety evaluations for clinical use, such as polyethylene glycol (PEG), polycaprolactone (PCL), poly (lactic-co-glycolic acid) (PLGA), and collagen, their safe application in bone defects, not to mention completely new types of composites, still needs to be evaluated in detail, considering the stress, wear and tear and the local inflammatory microenvironment reacts with the biomaterial (Ho-Shui-Ling et al., 2018; Zhao et al., 2021b). Ideally, the scaffold in vivo will be completely replaced by new bone tissue to return to normal anatomical structure and function, so the biomaterial scaffold should be biodegradable. It is worth noting that the degradation rate should be in line with the rate of bone regeneration, too fast degradation will be difficult to provide effective support, and too slow degradation will impede the growth of blood vessels and bone matrix. Pre-adjusting the composition or physical parameters of composite materials or correlating the decomposition reaction with the substance which changes significantly in bone regeneration are common approaches to regulate the decomposition rate of materials. Miao et al. (2021) successfully controlled the time scale of scaffold degradation in vivo by adjusting the porosity and phase composition of strontium-doped tricalcium phosphate (Sr-TCP) microspheres in a composite scaffold system. Zheng et al. (2023) designed a composite hydrogel system to achieve adaptive reinforcement and degradation by responding to Ca2+ concentration and pH in the inflammatory microenvironment (Figure 2). Infection is an important cause of impaired bone healing. Serious contamination of open wounds, improper nursing of surgical incisions after operation, and low immunity of patients may lead to infection. Biomaterial with antibacterial activity is not a new concept. The concept of biomaterials with antimicrobial effects is not new and can be achieved by various means such as biomaterials combined with antibiotics, antimicrobial peptides, and metal particles with antimicrobial effects. Wu et al. (2021) achieved long-lasting antibacterial effects by loading N-halogen polymer coatings on the surface of titanium implants. Similarly, Hayashi et al. (2022) modified the surface of carbonate apatite with silver phosphate to make the scaffold exhibit good antibacterial activity. Interestingly, they also reported that the honeycomb structure also had the effect of preventing bacterial growth. The biomaterial should also have a certain mechanical strength, considering that the graft may have weight-bearing or support requirements in vivo. The biomaterial should mimic the mechanical properties of natural bone tissue as much as possible. The utility of biomaterial scaffolds in bone regeneration is usually evaluated from three dimensions: osteoinduction, osteoconduction, and osseointegration. These dimensions range from the induction of MSC differentiation at the cellular level to the binding of the graft to the local tissue of the host at the tissue level. It is worth emphasizing again that no material can satisfy all desirable design parameters. In practical clinical applications, it is often necessary to seek a balance between various parameters according to actual needs.
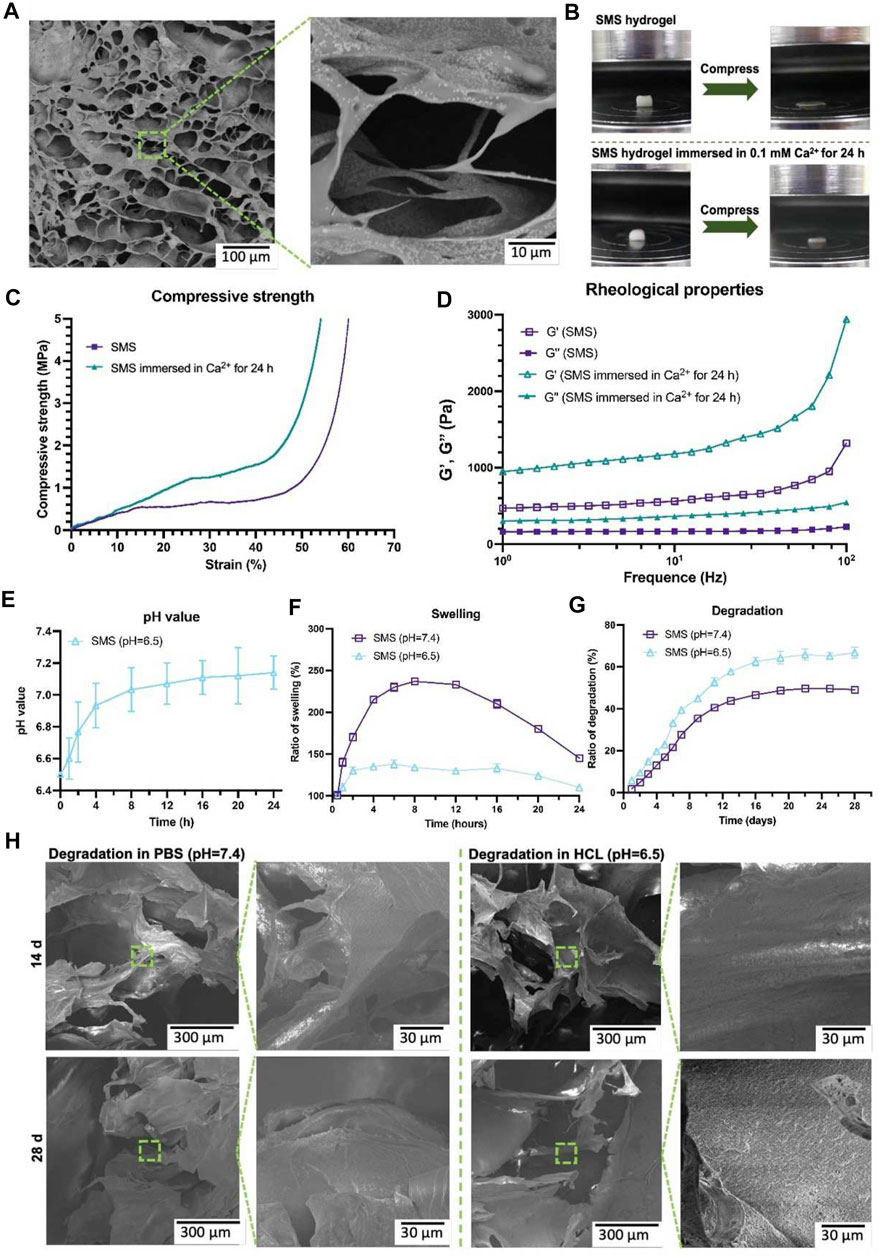
FIGURE 2. Microstructure of SMS hydrogel under SEM (A). Compression testing diagram (B). Strain curves of SMS hydrogel under different Ca2+ conditions (C). Rheological behavior of SMS hydrogel under different Ca2+ conditions (D). Effect of SMS hydrogel on PH value in HCl environment (E). Swelling of SMS hydrogel under different PH conditions in vitro (F). Degradation of SMS hydrogel under different PH conditions in vitro (G). Microstructure of hydrogel under SEM in different PH conditions (H). SMS: silk fibroin/mesoporous bioglass/sodium alginate. Reproduced with permission from (Zheng et al., 2023) (Creative Commons—Attribution-NonCommercial-NoDerivatives 4.0 International—CC BY-NC-ND 4.0).
3.1.2 Parameters to regulate macrophage
As scientists increasingly focus on the influence of material properties on macrophage activity, exploring the corresponding parameters adapted to the macrophages and applying them will undoubtedly lead to better regulation of macrophage activity (Table.2). Substances produced during scaffold degradation may be absorbed by macrophages or affect the local microenvironment. Meanwhile, scaffolds that are difficult to degrade often cause local chronic inflammation, which is an important adverse effect on bone regeneration. Wang et al. (2017a) reported that bidirectional calcium phosphate (BCP) ceramics promoted migration and osteogenic differentiation of MSCs by promoting the secretion of macrophages. Chen et al. (2014) demonstrated that Ca2+ generated from β-tricalcium phosphate (β-TCP) degradation can promote macrophage switching to the M2 phenotype and increase bone morphogenetic protein (BMP)-2 expression by activating calcium-sensing receptor (CaSR). Similarly, the effects of Mg2+, Zn2+, Sr2+, and Cu2+ on macrophage phenotype and secretory function have been demonstrated (Huang et al., 2019; Bai et al., 2021; Tan et al., 2021; You et al., 2022). To provide living space for new blood vessels and tissues, scaffolds often have certain pores, and the size of the pores will have an impact on macrophage activity. However, there are different conclusions about the suitable pore size for macrophages. Tylek et al. (2020) reported that scaffolds promoted differentiation of M2 phenotype macrophages when the pore size was reduced from 100 to 40 μm. Similarly, Wang et al. (2014) reported that 30 μm pore size was beneficial to the phenotypic differentiation of M2 macrophages. However, in the study by Li and co-workers (Li et al., 2022), scaffolds with the pore size of 600 μm had better M2 cell infiltration. These differences may be due to the different raw materials and fabrication processes of the scaffolds. The substrate stiffness provided by the scaffold also affects the activity of macrophages. Sridharan and co-workers (Sridharan et al., 2019) explored the effect of material stiffness on macrophage polarization, migration pattern, and function. The results indicated that macrophages cultured on high stiffness substrates (323 kPa) exhibited slow mesenchymal migration with pro-inflammatory phenotype and impaired phagocytosis, while macrophages cultured on softer substrates (11 kPa and 88 kPa) exhibited fast amoebae migration with anti-inflammatory and highly phagocytic phenotype. Considering that macrophages may adapt to different stiffness in different tissues. (Chen et al. (2020) compared the effects of different stiffness substrates on bone marrow-derived macrophage function. At low stiffness (2.55 ± 0.32 kPa), macrophages were more likely to differentiate into M1 phenotypes and secrete more pro-inflammatory factors, while at medium stiffness (34.88 ± 4.22 kPa), macrophages showed more M2 phenotypes and secrete more anti-inflammatory factors (Figure 3). Zhao et al. (2021a) prepared a periosteum-bone complex using porcine femur, and the stiffness of the treated periosteal part was reduced to 41.6 ± 3.7 kPa. The complex exhibited the ability to enhance M2 polarization of macrophages and promote osteogenic differentiation in vitro, and also exhibited the ability to stimulate bone regeneration in vivo.
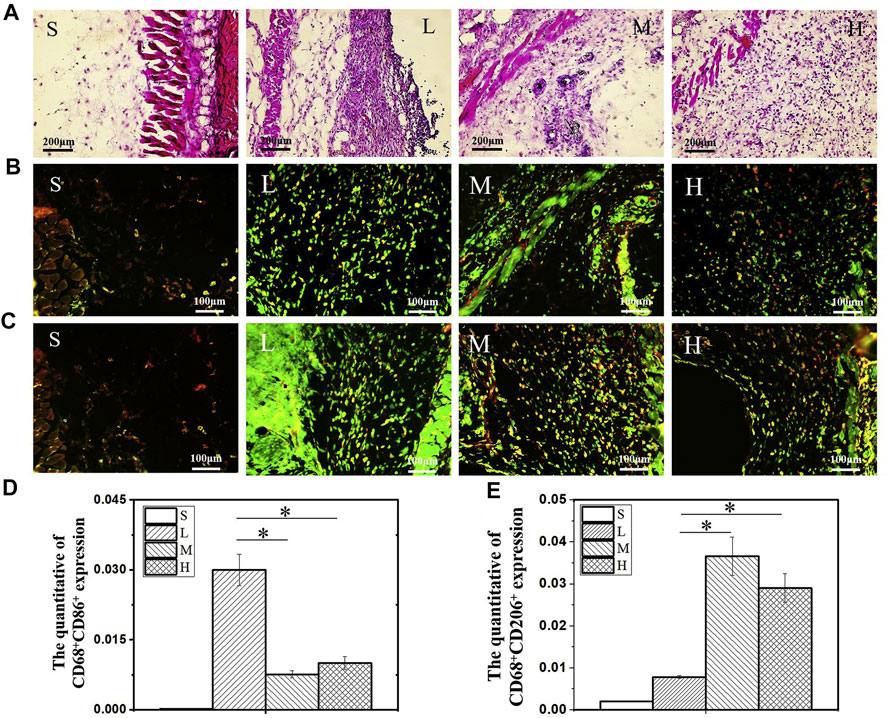
FIGURE 3. H & E staining image after 14 days of hydrogel subcutaneous implantation (A). Immunofluorescence staining image of CD68+CD86+ (B) and CD68+CD206+ (C) macrophages after 14 days of hydrogel subcutaneous implantation. Quantification analysis of CD68+CD86+ (D) and CD68+CD206+ (E) macrophages. S: sham, L: low stiffness, M: middle stiffness, H: high stiffness, n = 3, *p < 0.05. Reproduced with permission from (Chen et al., 2020).
With the increasing exploration of the microscopic scale of materials, some microscopic scale parameters have also been found to be closely related to the activity of macrophages. The scaffold provides attachment sites for cells in vivo. In addition to affecting macrophage attachment, the roughness of the surface is also thought to affect macrophage activity. Barth et al. (2013) compared the effect of different rough titanium metal surfaces on macrophage polarization and showed that rough surfaces were more favorable for polarization of the M2 phenotype. However, Wang and his co-workers (Wang et al., 2018) reported the opposite result, noting that rough surfaces promote more polarization of the M1 phenotype. Zhang et al. (2019) indicated that only roughness within a certain range caused macrophages to exhibit an anti-inflammatory tendency and polarize toward the M2 phenotype. Abaricia et al. (2020) further pointed out the important role of Wnt signaling pathway in macrophages in response to the material surface properties, the Wnt ligand mRNA was upregulated in macrophages in a surface modification-dependent manner. In addition to surface roughness, surface wettability is similarly thought to influence macrophage activity. Hamlet et al. (2012) reported that hydrophilic modification of rough titanium surfaces reduced the expression of key proinflammatory factors. Lv et al. (2018) reconfirmed that hydrophilic modification can make macrophages exhibit anti-inflammatory and pro-healing properties, and further explained the mechanism of this phenomenon as integrin β1 and β2 affecting macrophage activity through PI3K and NF-κB pathways, respectively. Interestingly, Hotchkiss et al. (2016) compared the effects of surfaces with different wetness and roughness on macrophages, the results indicated that the polarization of M2 phenotype was better promoted on the hydrophilic and rough scaffold surface, and the wetness of the surface had a more obvious immunomodulatory effect.
As detection and preparation processes continue to evolve, scaffolds can be observed and finely processed at smaller scales, leading to the further discovery that specific topography can affect macrophages. Chen et al. (2010) reported the effect of material topography on macrophage activity in the micrometer to nanometer range. They imprinted gratings with linewidth ranging from 2 μm to 250 nm on the flat material. Under different grating conditions, macrophages exhibited different cell morphology, secretion, and adhesion states. Luu and co-workers (Luu et al., 2015) further determined that grooves with width of 400–500 nm would have the best elongation rate of macrophages, and grooves would not affect the activation of inflammatory response but would enhance the polarization of macrophages to anti-inflammatory and pro-healing phenotype. Similarly, Yang et al. (2019) compared the effects of circular patterns with different diameters (4 μm, 12 μm and 36 μm) on macrophage activity. They observed that the larger patterns promoted macrophage polarization to M2 phenotype. Interestingly, they observed that the 4 μm pattern exhibited an effect of stimulating macrophage polarization to M1 phenotype. In addition, Nouri-Goushki et al. (2021) used 3D printing technology to analyse the effects of different heights and spacing of micro columns on macrophages, and the results indicated that high enough micro columns were conducive to the polarization of M2 phenotype. However, as with many parameters, contradictory experimental findings were also reported. Vassey et al. (2020) used algorithms to generate a database of up to 2,176 micropatterns and showed that micropillars with a diameter of 5–10 μm play a predominant role in macrophage attachment, and showed that smaller and denser surface features promote M2 phenotype polarization. Furthermore, in a study comparing three different topologies (random, aligned, and the lattice), the lattice topography showed a better ability to recruit macrophages and induce angiogenesis (Jin et al., 2021). These different results may be caused by different distance settings between individual micropatterns, or it is possible that different micropatterns indirectly lead to changes in the roughness and wettability of the material surface. Considering that macrophages are in a complex 3D microenvironment in vivo, the 3D model can undoubtedly reflect the state of cells more formally. Jiang et al. (2016) designed a scaffold considering 3D structure, which was expanded in thickness and thus had a larger cross-section spacing than the traditional membrane structure. This scaffold showed good macrophage penetration ability and a high M2/M1 ratio in subcutaneous implantation experiments. Fang et al. (2020) further reported the relationship between 3D matrix and macrophage morphology and fusion rate in the absence of exogenous cytokines. Although 3D culture has been extensively studied in stem cells, the effects of more detailed 3D topology on macrophages still need to be investigated experimentally.
At a more microscopic molecular level, the charges and chirality of the scaffold groups are thought to affect the function of macrophages. In a previous study, the surface of titanium implants was modified with divalent cations and macrophage polarization toward the M2 phenotype was significantly enhanced (Lee et al., 2016). Mao et al. (2022) further evaluated the effect of material surface charge on macrophages and bone regeneration. Scaffold with positive surface charges significantly inhibited M1 polarization of macrophages and enhanced osteogenic differentiation of MSCs. Many substances in the body have a certain chiral selection, and chiral modification of materials has been applied to the field of bone regeneration (Xu-Kai et al., 2022). The introduction of chiral groups on the surface of materials may affect the activity of macrophages. The production of interleukine (IL) −6 and regulated on activation, normal T cell expressed and secreted (RANTES) in macrophages was better produced by the L-chirality connection of amantadine containing peptidoglycan fragments (Manček-Keber et al., 2020). Jiang et al. (2022) reported that pathology-mimetic M-nanofibers promoted macrophage M2 phenotypic polarization more than physiology-mimetic simulated P-nanofibers, significantly inhibited inflammation and promoted tissue regeneration.
In addition to the parameters embodied in the material itself, some physical stimuli also affect the activity of macrophages. Ballotta et al. (2014) reported the bidirectional effect of different cyclic strains on the polarization of macrophages in scaffold. Under 7% cyclic strain, macrophages became polarized toward M2, while under 12% cyclic strain, macrophages became polarized toward M1 phenotype. One study investigated the effect of mechanical load on macrophages and indicated that mechanical load promoted the polarization of macrophages into the M1 phenotype (He et al., 2020) (Figure 4). Stimulation through physical fields such as magnetic field, electric field, and light field has also been proved to affect the activity of macrophages, which provides new means to more fully intervene the activity of macrophages. Wosik et al. (2018) reported that external magnetic fields can rearrange the cytoskeleton, organelles, and cationic channels of macrophages and further affect the expression of molecular markers in macrophages. Similarly, Hoare and co-workers (Hoare et al., 2016) reported the regulatory effect of electric field on macrophages. Macrophages showed a tendency to move towards the anode in the electric field and reached a peak when the electric field intensity was 300 mV/mm. Interestingly, monocytes, as the precursor of macrophages, showed a tendency to move toward the cathode. In addition, the electric field also affected the phagocytosis and secretion function of macrophages. Kang et al. (2018) developed a photosensitive nanocarrier that can further regulate the polarization of macrophages by regulating the concentration of Ca2+ in fine macrophages through near-infrared light.
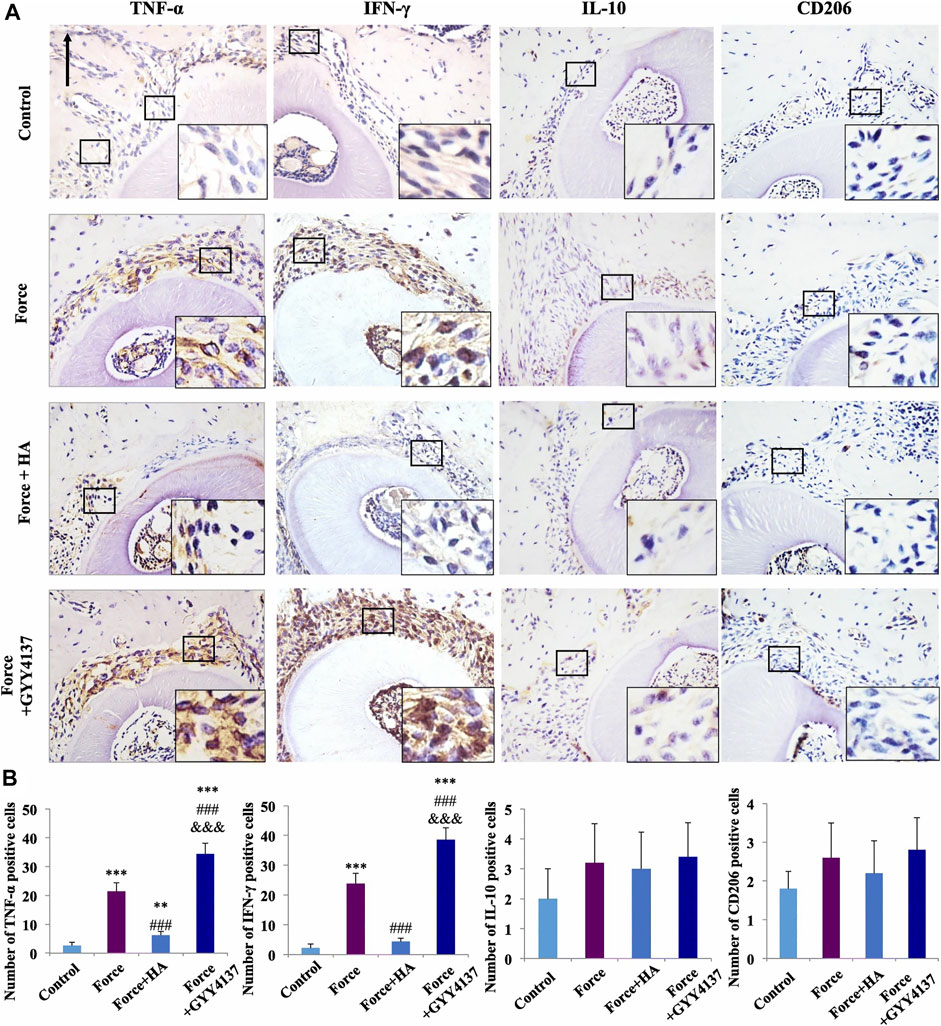
FIGURE 4. Immunohistochemical staining image of M1/M2 macrophage markers (A). Semiquantification analysis of M1/M2 macrophage (B). Scale bars: 100 μm **p < 0.01, ***p < 0.001 comapered with control. ###p < 0.001 comapered with force. and &&&p < 0.001 comapered with force + HA. Reproduced with permission from (He et al., 2020).
Although scientists have found many methods that can directly or indirectly interfere with macrophages and animal model techniques are becoming more sophisticated, considering the specific parameters suitable for human body and the huge differences caused by different body conditions and defect sites, a large quantity of preliminary experiments are still needed (Zhu et al., 2022b). In addition, in further clinical applications, reliable and economical production processes and standardization still need to be considered.
3.2 Therapeutic substance to regulate macrophage
Today, with the growing awareness that bone regeneration is an integrated process regulated by multiple factors, multifunctional scaffold systems are rapidly evolving. Their vivo characteristic ensures that they can intervene in multiple temporal stages of bone regeneration, and their high degree of manipulability ensures that they can meet as many environmental factors as possible that are appropriate for bone regeneration. Overall, macrophages are important targets for promoting endogenous bone regeneration, and the role of the scaffold system on macrophages is mainly reflected in the regulation of macrophage recruitment/proliferation and the M1/M2 phenotype ratio.
An adequate number of macrophages is a prerequisite for initiating and sustaining the bone regeneration cascade. Direct delivery of allogeneic or vitro induced autologous macrophages may result in immune rejection or disease transmission (Chu et al., 2020; Niu et al., 2021). Therefore, there are few reports of direct macrophages promoting bone regeneration, and the mainstream methods still tend to mobilize endogenous macrophages for bone regeneration. In addition, although the excessive inflammatory response is considered to be detrimental to bone regeneration, appropriate inflammatory response and M1 phenotype macrophages in the primary stage of regeneration are also necessary for bone regeneration (Alexander et al., 2011; Edderkaoui, 2017; Schlundt et al., 2018; Pountos et al., 2019). Combining scaffold systems with chemical compounds is a common approach. Chu et al. (2019b) modified traditional collagen membranes with epigallocatechin-3-gallate to achieve better M2 phenotypic macrophage recruitment and upregulation of many growth factors and osteogenic differentiation-related factors. In their subsequent studies, they further revealed that the recruitment of M2 phenotypic macrophages may be related to the C-C chemokine receptor type 2 signaling pathway (Chu et al., 2019a). SEW2871 is a macrophage recruitment agent. Kim et al. (2014) added SEW2871 and platelet-rich plasma to gelatin hydrogel, and the hydrogel showed pro-inflammatory effects after 3 days of application, while significant anti-inflammatory effects were observed 10 days after surgery. However, in their further study, they combined SEW2871 with fibrin hydrogel scaffolds (Tanaka et al., 2019). The fibrin hydrogel alone had better anti-inflammatory effect and promoted the polarization of macrophages than gelatin hydrogel. There was no significant difference in the migration activity of macrophages whether SEW2871 was contained or not. This may be due to the rapid release and degradation of the drug in the fibrin hydrogel. A previous study reported that a sequentially controlled drug release system modulated macrophage activity (Ma et al., 2022). The system sequentially released two peptides, LL37 and WP9QY. LL37, while having an antibacterial effect, promotes local inflammation in early stage, which contributes to the polarization of M1 phenotype macrophages. The subsequent release of WP9QY has anti-inflammatory effects and promotes phenotypic polarization of M2. In addition, WP9QY also promotes calcium deposition and osteogenic differentiation. Zhang et al. (2022a) reported a method using the inflammatory microenvironment to control drug release. MnCO carried in the scaffold system would produce Fenton-like reaction with H2O2 in the inflammatory microenvironment to release Mn2+ and CO, thus promoting the polarization of M2-phenotype macrophages. Some traditional medicines can also be combined with scafflod systems. Xiang and co-workers (Xiang et al., 2021) prepared a silk-gel scaffold containing sitagliptin. This direct delivery method achieved local drug concentrations that were difficult to achieve through oral administration and avoided potential side effects. At the same time, the scaffold showed good anti-inflammatory effects and promoted the polarization of M2-phenotype macrophages (Figure 5).
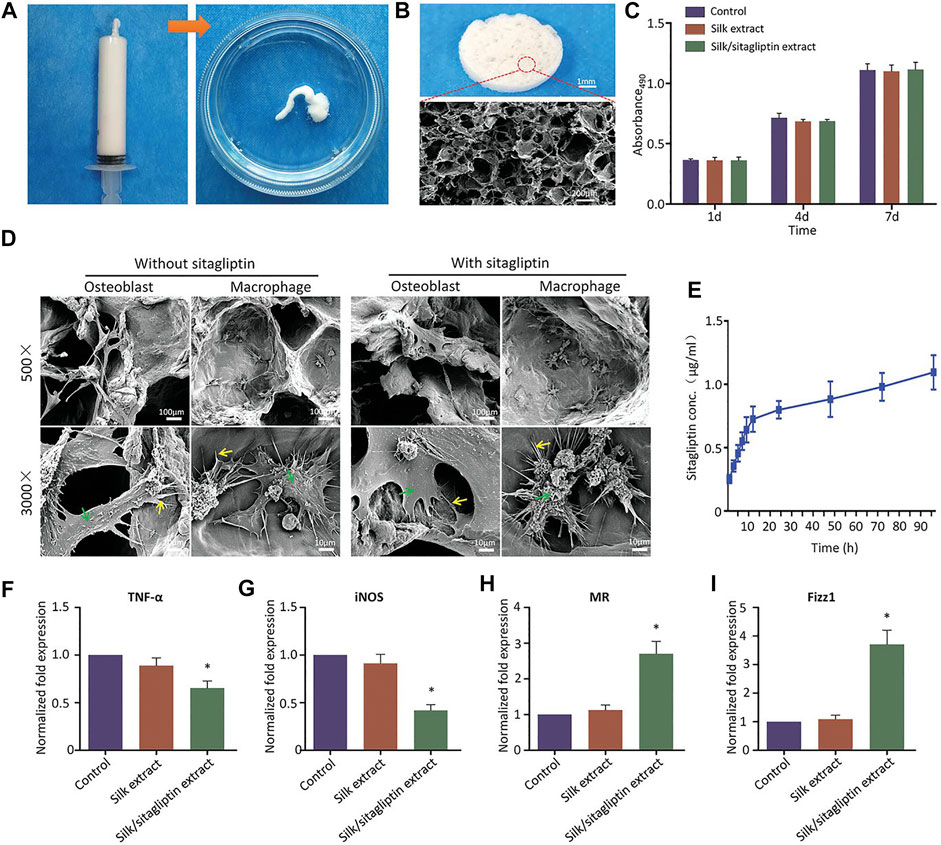
FIGURE 5. Injectable characterization of the scaffold system (A). Microstructure of the scaffold under SEM (B). The proliferation of mouse cells at day 1, 7, and 14 (C). The attachment of macrophages or osteoblasts to scaffolds under SEM (D). Drug release curve in the scaffold system (E). The expression of M1-related biomarkers after 24 h (F, G). The expression of M2-related markers after 24 h (H, I). *p < 0.05 comapered with control group. Reproduced with permission from (Xiang et al., 2021).
Some signaling substances also show broad application prospects. Bone morphogenetic proteins (BMP) belonging to the transforming growth factor-β (TGF-β) superfamily are important substances in inducing osteogenic differentiation. As previously reported, the serum content of BMP-4 in diabetic patients decreased (Yurekli et al., 2018). Sun et al. (2021) loaded BMP-4 into mesoporous silica nanoparticles. The addition of nanoparticles enhanced the mechanical strength of the scaffold system, and the continuous release of BMP-4 promoted the polarization of the phenotype of M2 macrophages (Figure 6). Meanwhile, BMP-4 released by nanoparticles and BMP-2 secreted by M2 macrophages jointly promoted osteogenic differentiation of stem cells. Similarly, Cui et al. (2020) achieved controlled expression of BMP-4 by enabling the scaffold to carry recombinant plasmids with BMP-4 gene fragments. Interleukin-4 (IL-4) has been confirmed having an excellent regulatory effect on macrophage polarization in a large number of previous studies (Van Dyken and Locksley, 2013; Pajarinen et al., 2021). Zhao et al. (2021c) injected IL-4 subcutaneously to assist immune regulation of the scaffold system, and the results indicated that IL-4 could effectively induce M2 polarization and thus accelerate bone regeneration. Zhang et al. (2020) prepared a hydrogel bead containing IL-4, which effectively promoted the M2 phenotype polarization of macrophages and increased the expression of TGF-β1. Similarly, Ueno and co-workers (Ueno et al., 2020) prepared a macroporous gelatin-based microband scaffold containing IL-4-secreting MSCs. In the mouse bone defect model, the scaffold enhanced M2 marker expression while enhancing macrophage migration. In addition, the scaffold did not inhibit M1 marker expression. Considering the necessity of appropriate intensity of inflammation for macrophages, it is necessary to ensure the availability of a sufficient number of M1 phenotype macrophages. Chan and co-workers (Chan et al., 2015) formerly reported that the addition of low-dose recombinant human tumor necrosis factor to the fracture site in 24 h after injury enhanced bone healing in animal fracture models. Luo et al. (2021) designed an interferon (IFN)-γ/Sr-dropped bioactive glass composite scaffold. This scaffold first releases IFN-γ in vivo to promote the polarization of macrophages into the M1 phenotype and then releases Sr2+ to contribute to the polarization of the M2 phenotype. Similarly, vascular endothelial growth factor has been shown to promote macrophage recruitment in the early stages of inflammation (Hu and Olsen, 2016).
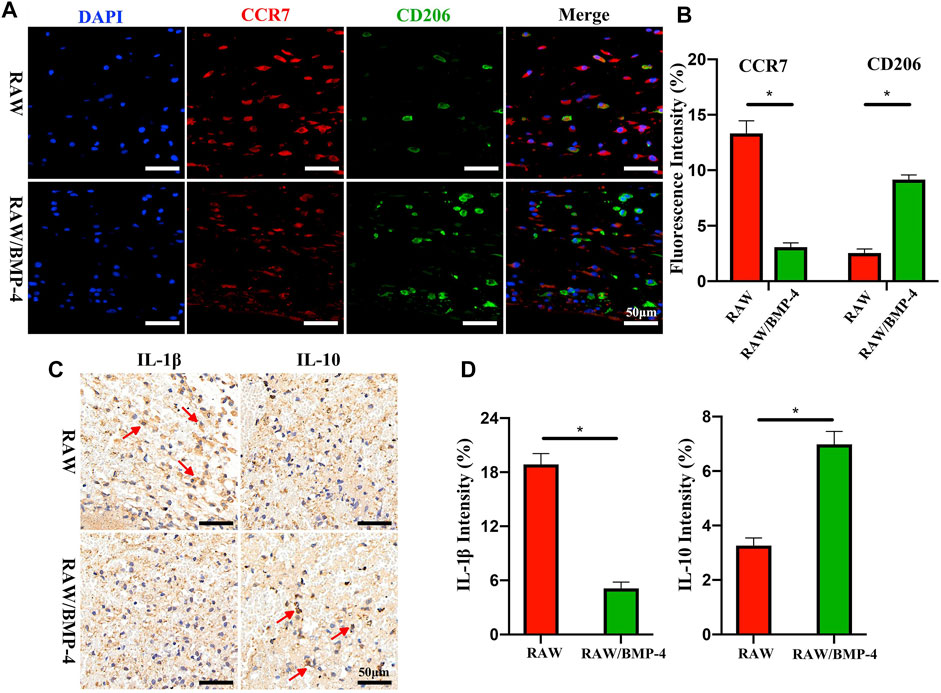
FIGURE 6. Fluorescence staining of DAPI and cells expressing CCR7 or CD206 (A). Quantitative analysis of CCR7 or CD206 (B). Immunohistochemical staining of IL-1β and IL-10 (C). Quantitative analysis of IL-1β and IL-10 (D). *p < 0.05. Reproduced with permission from (Sun et al., 2021).
With the continuous understanding of the information transmission mechanism in bone healing, the regulatory effects of some novel signaling substances such as RNA and exosomes are also worth expecting. However, there is relatively little research on its role in regulating the function of macrophages in bone regeneration. Castaño et al. (2020) designed a collagen nano-hydroxyapatite scaffold that could deliver the antagomiR-133a. With the local release of antagomiR-133a, the number of M2 phenotype macrophages was significantly elevated, resulting in a considerable increase in bone volume in the animal bone defect model. As a means of intercellular communication, exosomes contain a variety of RNAs. Jiang et al. (2021a) developed an extracellular matrix scaffold with exosomes derived from MSCs, and the addition of exosomes effectively promoted the phenotype polarization of macrophages M2. Further, Xu et al. (2022) transfected MSCs with viral vectors containing Smurf1-shRNA to obtain engineered exosomes, which were then phagocytosed by macrophages to promote the polarization of exosomes towards M2 phenotypes using microarc titanium oxide as the delivery scaffold.
Considering the different intervention times of different scaffolds for bone regeneration and the blurred boundary of the key transition from pro-inflammatory to anti-inflammatory in bone regeneration, the regulation of M1/M2 macrophage ratio requires flexible selection.
4 Conclusion and outlook
With the extension of life expectancy and the improvement of health concept, tissue engineering has a broad application prospect and clinical value in the treatment of bone defects that are difficult to self-heal. Although many implants have been put into clinical application, most of them are unable to meet the requirements of promoting endogenous bone healing, especially for patients with large defect size or poor physical conditions. Poor bone healing can bring a huge burden to patients both physically and mentally. Macrophage is an important target to regulate bone regeneration, and biomaterials can provide a good medium.
In this review, we review the parameters that influence macrophages during the material design phase and the types of therapeutic agents that can be selected to further regulate macrophage activity. Although there is a wealth of excellent research emerging, the vast majority is still far from practical clinical application. To put the scaffold system into clinical application, it is necessary to master the specific parameters suitable for human tissues. However, even without considering the differences in bone parameters under different ages and nutritional conditions, the parameter differences caused by different defect sites may also be huge. Secondly, due to the blurred boundaries of each segment of bone healing, the scaffold system may face different functional requirements at different time points and periods of intervention, which not only requires careful consideration at the beginning of scaffold system design but also requires clinicians to have a correct judgment. Finally, all implants should be subjected to a rigorous safety evaluation.
In future studies, with the continuous comprehension of the mechanism of bone regeneration and the function of macrophages, more valuable targets and regulatory mechanisms will be further clarified. The rapid development of nanomaterials and the continuous improvement of manufacturing processes such as 3D printing, electrospinning, and surface modification technology make it possible to break through the bottleneck of traditional material design (Zhou et al., 2021; Wan et al., 2022). More advanced detection and imaging techniques have also enabled the mimicry of bone structures to enter a more microscopic level. In addition, the cross-integration of artificial intelligence and other fields will also greatly save the time and cost of experiments required to screen suitable parameters.
Author contributions
ZoL provided the idea and wrote the original manuscript. JZ, ZhL, and HL revised the manuscript. CF provided the idea and revised the manuscript. All authors have read and agreed to the published version of the manuscript.
Acknowledgments
The authors would like to express their gratitude to EditSprings (https://www.editsprings.cn) for the expert linguistic services provided.
Conflict of interest
The authors declare that the research was conducted in the absence of any commercial or financial relationships that could be construed as a potential conflict of interest.
Publisher’s note
All claims expressed in this article are solely those of the authors and do not necessarily represent those of their affiliated organizations, or those of the publisher, the editors and the reviewers. Any product that may be evaluated in this article, or claim that may be made by its manufacturer, is not guaranteed or endorsed by the publisher.
References
Abaricia, J. O., Shah, A. H., Chaubal, M., Hotchkiss, K. M., and Olivares-Navarrete, R. (2020). Wnt signaling modulates macrophage polarization and is regulated by biomaterial surface properties. Biomaterials 243, 119920. doi:10.1016/j.biomaterials.2020.119920
Alexander, K. A., Chang, M. K., Maylin, E. R., Kohler, T., MüLLER, R., Wu, A. C., et al. (2011). Osteal macrophages promote in vivo intramembranous bone healing in a mouse tibial injury model. J. Bone Min. Res. 26, 1517–1532. doi:10.1002/jbmr.354
Antonova, E., Le, T. K., Burge, R., and Mershon, J. (2013). Tibia shaft fractures: Costly burden of nonunions. BMC Musculoskelet. Disord. 14, 42. doi:10.1186/1471-2474-14-42
Bai, X., Liu, W., Xu, L., Ye, Q., Zhou, H., Berg, C., et al. (2021). Sequential macrophage transition facilitates endogenous bone regeneration induced by Zn-doped porous microcrystalline bioactive glass. J. Mater Chem. B 9, 2885–2898. doi:10.1039/d0tb02884c
Ballotta, V., Driessen-Mol, A., Bouten, C. V., and Baaijens, F. P. (2014). Strain-dependent modulation of macrophage polarization within scaffolds. Biomaterials 35, 4919–4928. doi:10.1016/j.biomaterials.2014.03.002
Barth, K. A., Waterfield, J. D., and Brunette, D. M. (2013). The effect of surface roughness on RAW 264.7 macrophage phenotype. J. Biomed. Mater Res. A 101, 2679–2688. doi:10.1002/jbm.a.34562
Berendsen, A. D., and Olsen, B. R. (2015). Bone development. Bone 80, 14–18. doi:10.1016/j.bone.2015.04.035
CastañO, I. M., Raftery, R. M., Chen, G., Cavanagh, B., Quinn, B., Duffy, G. P., et al. (2020). Rapid bone repair with the recruitment of CD206(+)M2-like macrophages using non-viral scaffold-mediated miR-133a inhibition of host cells. Acta Biomater. 109, 267–279. doi:10.1016/j.actbio.2020.03.042
Chan, J. K., Glass, G. E., Ersek, A., Freidin, A., Williams, G. A., Gowers, K., et al. (2015). Low-dose TNF augments fracture healing in normal and osteoporotic bone by up-regulating the innate immune response. EMBO Mol. Med. 7, 547–561. doi:10.15252/emmm.201404487
Chazaud, B. (2020). Inflammation and skeletal muscle regeneration: Leave it to the macrophages. Trends Immunol. 41, 481–492. doi:10.1016/j.it.2020.04.006
Chen, M., Zhang, Y., Zhou, P., Liu, X., Zhao, H., Zhou, X., et al. (2020). Substrate stiffness modulates bone marrow-derived macrophage polarization through NF-κB signaling pathway. Bioact. Mater 5, 880–890. doi:10.1016/j.bioactmat.2020.05.004
Chen, S., Jones, J. A., Xu, Y., Low, H. Y., Anderson, J. M., and Leong, K. W. (2010). Characterization of topographical effects on macrophage behavior in a foreign body response model. Biomaterials 31, 3479–3491. doi:10.1016/j.biomaterials.2010.01.074
Chen, Z., Wu, C., Gu, W., Klein, T., Crawford, R., and Xiao, Y. (2014). Osteogenic differentiation of bone marrow MSCs by β-tricalcium phosphate stimulating macrophages via BMP2 signalling pathway. Biomaterials 35, 1507–1518. doi:10.1016/j.biomaterials.2013.11.014
Chu, C., Liu, L., Wang, Y., Yang, R., Hu, C., Rung, S., et al. (2019a). Evaluation of epigallocatechin-3-gallate (EGCG)-modified scaffold determines macrophage recruitment. Mater Sci. Eng. C Mater Biol. Appl. 100, 505–513. doi:10.1016/j.msec.2019.03.007
Chu, C., Wang, Y., Wang, Y., Yang, R., Liu, L., Rung, S., et al. (2019b). Evaluation of epigallocatechin-3-gallate (EGCG) modified collagen in guided bone regeneration (GBR) surgery and modulation of macrophage phenotype. Mater Sci. Eng. C Mater Biol. Appl. 99, 73–82. doi:10.1016/j.msec.2019.01.083
Chu, Z., Sun, C., Sun, L., Feng, C., Yang, F., Xu, Y., et al. (2020). Primed macrophages directly and specifically reject allografts. Cell Mol. Immunol. 17, 237–246. doi:10.1038/s41423-019-0226-0
Claes, L., Recknagel, S., and Ignatius, A. (2012). Fracture healing under healthy and inflammatory conditions. Nat. Rev. Rheumatol. 8, 133–143. doi:10.1038/nrrheum.2012.1
Cui, L., Zhang, J., Zou, J., Yang, X., Guo, H., Tian, H., et al. (2020). Electroactive composite scaffold with locally expressed osteoinductive factor for synergistic bone repair upon electrical stimulation. Biomaterials 230, 119617. doi:10.1016/j.biomaterials.2019.119617
Dahabreh, Z., Calori, G. M., Kanakaris, N. K., Nikolaou, V. S., and Giannoudis, P. V. (2009). A cost analysis of treatment of tibial fracture nonunion by bone grafting or bone morphogenetic protein-7. Int. Orthop. 33, 1407–1414. doi:10.1007/s00264-008-0709-6
Diemer, F., Stark, H., Helfgen, E. H., Enkling, N., Probstmeier, R., Winter, J., et al. (2021). In vitro cytotoxicity of different dental resin-cements on human cell lines. J. Mater Sci. Mater Med. 32, 4. doi:10.1007/s10856-020-06471-w
Dimitriou, R., Jones, E., Mcgonagle, D., and Giannoudis, P. V. (2011). Bone regeneration: Current concepts and future directions. BMC Med. 9, 66. doi:10.1186/1741-7015-9-66
Edderkaoui, B. (2017). Potential role of chemokines in fracture repair. Front. Endocrinol. (Lausanne) 8, 39. doi:10.3389/fendo.2017.00039
Einhorn, T. A., and Gerstenfeld, L. C. (2015). Fracture healing: Mechanisms and interventions. Nat. Rev. Rheumatol. 11, 45–54. doi:10.1038/nrrheum.2014.164
Ekegren, C. L., Edwards, E. R., De Steiger, R., and Gabbe, B. J. (2018). Incidence, costs and predictors of non-union, delayed union and mal-union following long bone fracture. Int. J. Environ. Res. Public Health 15, 2845. doi:10.3390/ijerph15122845
el-Rashidy, A. A., Roether, J. A., Harhaus, L., Kneser, U., and Boccaccini, A. R. (2017). Regenerating bone with bioactive glass scaffolds: A review of in vivo studies in bone defect models. Acta Biomater. 62, 1–28. doi:10.1016/j.actbio.2017.08.030
Fang, J. Y., Yang, Z., and Han, B. (2020). Switch of macrophage fusion competency by 3D matrices. Sci. Rep. 10, 10348. doi:10.1038/s41598-020-67056-9
Florencio-Silva, R., Sasso, G. R., Sasso-Cerri, E., SimõES, M. J., and Cerri, P. S. (2015). Biology of bone tissue: Structure, function, and factors that influence bone cells. Biomed. Res. Int. 2015, 1–17. doi:10.1155/2015/421746
Gao, C., Sow, W. T., Wang, Y., Wang, Y., Yang, D., Lee, B. H., et al. (2021). Hydrogel composite scaffolds with an attenuated immunogenicity component for bone tissue engineering applications. J. Mater Chem. B 9, 2033–2041. doi:10.1039/d0tb02588g
Gracis, S., Thompson, V. P., Ferencz, J. L., Silva, N. R., and Bonfante, E. A. (2015). A new classification system for all-ceramic and ceramic-like restorative materials. Int. J. Prosthodont 28, 227–235. doi:10.11607/ijp.4244
Hamlet, S., Alfarsi, M., George, R., and Ivanovski, S. (2012). The effect of hydrophilic titanium surface modification on macrophage inflammatory cytokine gene expression. Clin. Oral Implants Res. 23, 584–590. doi:10.1111/j.1600-0501.2011.02325.x
Haugen, H. J., Lyngstadaas, S. P., Rossi, F., and Perale, G. (2019). Bone grafts: Which is the ideal biomaterial? J. Clin. Periodontol. 46 (21), 92–102. doi:10.1111/jcpe.13058
Hayashi, K., Shimabukuro, M., and Ishikawa, K. (2022). Antibacterial honeycomb scaffolds for achieving infection prevention and bone regeneration. ACS Appl. Mater Interfaces 14, 3762–3772. doi:10.1021/acsami.1c20204
He, D., Liu, F., Cui, S., Jiang, N., Yu, H., Zhou, Y., et al. (2020). Mechanical load-induced H(2)S production by periodontal ligament stem cells activates M1 macrophages to promote bone remodeling and tooth movement via STAT1. Stem Cell Res. Ther. 11, 112. doi:10.1186/s13287-020-01607-9
Ho-Shui-Ling, A., Bolander, J., Rustom, L. E., Johnson, A. W., Luyten, F. P., and Picart, C. (2018). Bone regeneration strategies: Engineered scaffolds, bioactive molecules and stem cells current stage and future perspectives. Biomaterials 180, 143–162. doi:10.1016/j.biomaterials.2018.07.017
Hoare, J. I., Rajnicek, A. M., Mccaig, C. D., Barker, R. N., and Wilson, H. M. (2016). Electric fields are novel determinants of human macrophage functions. J. Leukoc. Biol. 99, 1141–1151. doi:10.1189/jlb.3a0815-390r
Hotchkiss, K. M., Reddy, G. B., Hyzy, S. L., Schwartz, Z., Boyan, B. D., and Olivares-Navarrete, R. (2016). Titanium surface characteristics, including topography and wettability, alter macrophage activation. Acta Biomater. 31, 425–434. doi:10.1016/j.actbio.2015.12.003
Hu, K., and Olsen, B. R. (2016). Osteoblast-derived VEGF regulates osteoblast differentiation and bone formation during bone repair. J. Clin. Invest. 126, 509–526. doi:10.1172/jci82585
Huang, Q., Ouyang, Z., Tan, Y., Wu, H., and Liu, Y. (2019). Activating macrophages for enhanced osteogenic and bactericidal performance by Cu ion release from micro/nano-topographical coating on a titanium substrate. Acta Biomater. 100, 415–426. doi:10.1016/j.actbio.2019.09.030
Janaszewska, A., Lazniewska, J., Trzepiński, P., Marcinkowska, M., and Klajnert-Maculewicz, B. (2019). Cytotoxicity of dendrimers. Biomolecules 9, 330. doi:10.3390/biom9080330
Jiang, J., Li, Z., Wang, H., Wang, Y., Carlson, M. A., Teusink, M. J., et al. (2016). Expanded 3D nanofiber scaffolds: Cell penetration, neovascularization, and host response. Adv. Healthc. Mater 5, 2993–3003. doi:10.1002/adhm.201600808
Jiang, S., Tian, G., Yang, Z., Gao, X., Wang, F., Li, J., et al. (2021a). Enhancement of acellular cartilage matrix scaffold by Wharton's jelly mesenchymal stem cell-derived exosomes to promote osteochondral regeneration. Bioact. Mater 6, 2711–2728. doi:10.1016/j.bioactmat.2021.01.031
Jiang, S., Wang, M., and He, J. (2021b). A review of biomimetic scaffolds for bone regeneration: Toward a cell-free strategy. Bioeng. Transl. Med. 6, e10206. doi:10.1002/btm2.10206
Jiang, S., Zeng, Q., Zhao, K., Liu, J., Sun, Q., Huang, K., et al. (2022). Chirality bias tissue homeostasis by manipulating immunological response. Adv. Mater 34, e2105136. doi:10.1002/adma.202105136
Jin, S., Yang, R., Chu, C., Hu, C., Zou, Q., Li, Y., et al. (2021). Topological structure of electrospun membrane regulates immune response, angiogenesis and bone regeneration. Acta Biomater. 129, 148–158. doi:10.1016/j.actbio.2021.05.042
Kanakaris, N. K., and Giannoudis, P. V. (2007). The health economics of the treatment of long-bone non-unions. Injury 38 (2), S77–S84. doi:10.1016/s0020-1383(07)80012-x
Kang, H., Zhang, K., Wong, D. S. H., Han, F., Li, B., and Bian, L. (2018). Near-infrared light-controlled regulation of intracellular calcium to modulate macrophage polarization. Biomaterials 178, 681–696. doi:10.1016/j.biomaterials.2018.03.007
Kim, Y. H., Furuya, H., and Tabata, Y. (2014). Enhancement of bone regeneration by dual release of a macrophage recruitment agent and platelet-rich plasma from gelatin hydrogels. Biomaterials 35, 214–224. doi:10.1016/j.biomaterials.2013.09.103
Lee, C. H., Kim, Y. J., Jang, J. H., and Park, J. W. (2016). Modulating macrophage polarization with divalent cations in nanostructured titanium implant surfaces. Nanotechnology 27, 085101. doi:10.1088/0957-4484/27/8/085101
Li, W., Dai, F., Zhang, S., Xu, F., Xu, Z., Liao, S., et al. (2022). Pore size of 3D-printed polycaprolactone/polyethylene glycol/hydroxyapatite scaffolds affects bone regeneration by modulating macrophage polarization and the foreign body response. ACS Appl. Mater Interfaces 14, 20693–20707. doi:10.1021/acsami.2c02001
Liu, H., Tian, Y., Zhao, C., and Ding, J. (2022). Editorial: Bioactive bone regenerative materials and bionic prosthesis interfaces. Front. Bioeng. Biotechnol. 10, 1111743. doi:10.3389/fbioe.2022.1111743
Liverani, E., Rogati, G., Pagani, S., Brogini, S., Fortunato, A., and Caravaggi, P. (2021). Mechanical interaction between additive-manufactured metal lattice structures and bone in compression: Implications for stress shielding of orthopaedic implants. J. Mech. Behav. Biomed. Mater 121, 104608. doi:10.1016/j.jmbbm.2021.104608
Locati, M., Curtale, G., and Mantovani, A. (2020). Diversity, mechanisms, and significance of macrophage plasticity. Annu. Rev. Pathol. 15, 123–147. doi:10.1146/annurev-pathmechdis-012418-012718
Lowery, J. W., and Rosen, V. (2018). Bone morphogenetic protein-based therapeutic approaches. Cold Spring Harb. Perspect. Biol. 10, a022327. doi:10.1101/cshperspect.a022327
Lucas, D. (2021). Structural organization of the bone marrow and its role in hematopoiesis. Curr. Opin. Hematol. 28, 36–42. doi:10.1097/moh.0000000000000621
Luo, M., Zhao, F., Liu, L., Yang, Z., Tian, T., Chen, X., et al. (2021). IFN-γ/SrBG composite scaffolds promote osteogenesis by sequential regulation of macrophages from M1 to M2. J. Mater Chem. B 9, 1867–1876. doi:10.1039/d0tb02333g
Luu, T. U., Gott, S. C., Woo, B. W., Rao, M. P., and Liu, W. F. (2015). Micro- and nanopatterned topographical cues for regulating macrophage cell shape and phenotype. ACS Appl. Mater Interfaces 7, 28665–28672. doi:10.1021/acsami.5b10589
Lv, L., Xie, Y., Li, K., Hu, T., Lu, X., Cao, Y., et al. (2018). Unveiling the mechanism of surface hydrophilicity-modulated macrophage polarization. Adv. Healthc. Mater 7, e1800675. doi:10.1002/adhm.201800675
Ma, S., Wang, C., Dong, Y., Jing, W., Wei, P., Peng, C., et al. (2022). Microsphere-gel composite system with mesenchymal stem cell recruitment, antibacterial, and immunomodulatory properties promote bone regeneration via sequential release of LL37 and W9 peptides. ACS Appl. Mater Interfaces 14, 38525–38540. doi:10.1021/acsami.2c10242
Manček-Keber, M., Ribić, R., Chain, F., Sinnaeve, D., Martins, J. C., Jerala, R., et al. (2020). Adamantane containing peptidoglycan fragments enhance RANTES and IL-6 production in lipopolysaccharide-induced macrophages. Molecules 25, 3707. doi:10.3390/molecules25163707
Mao, L., Bai, L., Wang, X., Chen, X., Zhang, D., Chen, F., et al. (2022). Enhanced cell osteogenesis and osteoimmunology regulated by piezoelectric biomaterials with controllable surface potential and charges. ACS Appl. Mater Interfaces 14, 44111–44124. doi:10.1021/acsami.2c11131
Marcucio, R. S., Miclau, T., 3R. D., and Bahney, C. S. (2023). A shifting paradigm: Transformation of cartilage to bone during bone repair. J. Dent. Res. 102, 13–20. doi:10.1177/00220345221125401
Marin, E., Boschetto, F., and Pezzotti, G. (2020). Biomaterials and biocompatibility: An historical overview. J. Biomed. Mater Res. A 108, 1617–1633. doi:10.1002/jbm.a.36930
Maruyama, M., Rhee, C., Utsunomiya, T., Zhang, N., Ueno, M., Yao, Z., et al. (2020). Modulation of the inflammatory response and bone healing. Front. Endocrinol. (Lausanne) 11, 386. doi:10.3389/fendo.2020.00386
Miao, Q., Jiang, N., Yang, Q., Hussein, I. M., Luo, Z., Wang, L., et al. (2021). Multi-stage controllable degradation of strontium-doped calcium sulfate hemihydrate-tricalcium phosphate microsphere composite as a substitute for osteoporotic bone defect repairing: Degradation behavior and bone response. Biomed. Mater 17, 015014. doi:10.1088/1748-605x/ac4323
Michalski, M. N., and Mccauley, L. K. (2017). Macrophages and skeletal health. Pharmacol. Ther. 174, 43–54. doi:10.1016/j.pharmthera.2017.02.017
Newman, H., Shih, Y. V., and Varghese, S. (2021). Resolution of inflammation in bone regeneration: From understandings to therapeutic applications. Biomaterials 277, 121114. doi:10.1016/j.biomaterials.2021.121114
Niu, Y., Wang, Z., Shi, Y., Dong, L., and Wang, C. (2021). Modulating macrophage activities to promote endogenous bone regeneration: Biological mechanisms and engineering approaches. Bioact. Mater 6, 244–261. doi:10.1016/j.bioactmat.2020.08.012
Nouri-Goushki, M., Isaakidou, A., Eijkel, B. I. M., Minneboo, M., Liu, Q., Boukany, P. E., et al. (2021). 3D printed submicron patterns orchestrate the response of macrophages. Nanoscale 13, 14304–14315. doi:10.1039/d1nr01557e
Pacheco, K. A. (2019). Allergy to surgical implants. Clin. Rev. Allergy Immunol. 56, 72–85. doi:10.1007/s12016-018-8707-y
Pajarinen, J., Lin, T., Nabeshima, A., Sato, T., Gibon, E., JäMSEN, E., et al. (2021). Interleukin-4 repairs wear particle induced osteolysis by modulating macrophage polarization and bone turnover. J. Biomed. Mater Res. A 109, 1512–1520. doi:10.1002/jbm.a.37142
Penack, O., Marchetti, M., Ruutu, T., Aljurf, M., Bacigalupo, A., Bonifazi, F., et al. (2020). Prophylaxis and management of graft versus host disease after stem-cell transplantation for haematological malignancies: Updated consensus recommendations of the European society for blood and marrow transplantation. Lancet Haematol. 7, e157–e167. doi:10.1016/s2352-3026(19)30256-x
Pinho, S., and Frenette, P. S. (2019). Haematopoietic stem cell activity and interactions with the niche. Nat. Rev. Mol. Cell Biol. 20, 303–320. doi:10.1038/s41580-019-0103-9
Potyondy, T., Uquillas, J. A., Tebon, P. J., Byambaa, B., Hasan, A., Tavafoghi, M., et al. (2021). Recent advances in 3D bioprinting of musculoskeletal tissues. Biofabrication 13, 022001. doi:10.1088/1758-5090/abc8de
Pountos, I., Walters, G., Panteli, M., Einhorn, T. A., and Giannoudis, P. V. (2019). Inflammatory profile and osteogenic potential of fracture haematoma in humans. J. Clin. Med. 9, 47. doi:10.3390/jcm9010047
Raffa, M. L., Nguyen, V. H., Hernigou, P., Flouzat-Lachaniette, C. H., and Haiat, G. (2021). Stress shielding at the bone-implant interface: Influence of surface roughness and of the bone-implant contact ratio. J. Orthop. Res. 39, 1174–1183. doi:10.1002/jor.24840
Roberts, T. T., and Rosenbaum, A. J. (2012). Bone grafts, bone substitutes and orthobiologics: The bridge between basic science and clinical advancements in fracture healing. Organogenesis 8, 114–124. doi:10.4161/org.23306
Rucci, N., and Teti, A. (2016). The "love-hate" relationship between osteoclasts and bone matrix. Matrix Biol. 52-54, 176–190. doi:10.1016/j.matbio.2016.02.009
Rupp, M., Klute, L., Baertl, S., Walter, N., Mannala, G. K., Frank, L., et al. (2022). The clinical use of bone graft substitutes in orthopedic surgery in Germany-A 10-years survey from 2008 to 2018 of 1,090,167 surgical interventions. J. Biomed. Mater Res. B Appl. Biomater. 110, 350–357. doi:10.1002/jbm.b.34911
Salhotra, A., Shah, H. N., Levi, B., and Longaker, M. T. (2020). Mechanisms of bone development and repair. Nat. Rev. Mol. Cell Biol. 21, 696–711. doi:10.1038/s41580-020-00279-w
Schlundt, C., el Khassawna, T., Serra, A., Dienelt, A., Wendler, S., Schell, H., et al. (2018). Macrophages in bone fracture healing: Their essential role in endochondral ossification. Bone 106, 78–89. doi:10.1016/j.bone.2015.10.019
Schlundt, C., Fischer, H., Bucher, C. H., Rendenbach, C., Duda, G. N., and Schmidt-Bleek, K. (2021). The multifaceted roles of macrophages in bone regeneration: A story of polarization, activation and time. Acta Biomater. 133, 46–57. doi:10.1016/j.actbio.2021.04.052
Schmidt, A. H. (2021). Autologous bone graft: Is it still the gold standard? Injury 52 (2), S18–s22. doi:10.1016/j.injury.2021.01.043
Shafaghi, R., Rodriguez, O., Schemitsch, E. H., Zalzal, P., Waldman, S. D., Papini, M., et al. (2019). A review of materials for managing bone loss in revision total knee arthroplasty. Mater Sci. Eng. C Mater Biol. Appl. 104, 109941. doi:10.1016/j.msec.2019.109941
Shapiro, F., and Wu, J. Y. (2019). Woven bone overview: Structural classification based on its integral role in developmental, repair and pathological bone formation throughout vertebrate groups. Eur. Cell Mater 38, 137–167. doi:10.22203/ecm.v038a11
Song, L. (2022). Effects of exercise or mechanical stimulation on bone development and bone repair. Stem Cells Int. 2022, 1–10. doi:10.1155/2022/5372229
Sridharan, R., Cavanagh, B., Cameron, A. R., Kelly, D. J., and O'Brien, F. J. (2019). Material stiffness influences the polarization state, function and migration mode of macrophages. Acta Biomater. 89, 47–59. doi:10.1016/j.actbio.2019.02.048
Sun, X., Ma, Z., Zhao, X., Jin, W., Zhang, C., Ma, J., et al. (2021). Three-dimensional bioprinting of multicell-laden scaffolds containing bone morphogenic protein-4 for promoting M2 macrophage polarization and accelerating bone defect repair in diabetes mellitus. Bioact. Mater 6, 757–769. doi:10.1016/j.bioactmat.2020.08.030
Tan, S., Wang, Y., Du, Y., Xiao, Y., and Zhang, S. (2021). Injectable bone cement with magnesium-containing microspheres enhances osteogenesis via anti-inflammatory immunoregulation. Bioact. Mater 6, 3411–3423. doi:10.1016/j.bioactmat.2021.03.006
Tanaka, R., Saito, Y., Fujiwara, Y., Jo, J. I., and Tabata, Y. (2019). Preparation of fibrin hydrogels to promote the recruitment of anti-inflammatory macrophages. Acta Biomater. 89, 152–165. doi:10.1016/j.actbio.2019.03.011
Turnbull, G., Clarke, J., Picard, F., Riches, P., Jia, L., Han, F., et al. (2018). 3D bioactive composite scaffolds for bone tissue engineering. Bioact. Mater 3, 278–314. doi:10.1016/j.bioactmat.2017.10.001
Tylek, T., Blum, C., Hrynevich, A., Schlegelmilch, K., Schilling, T., Dalton, P. D., et al. (2020). Precisely defined fiber scaffolds with 40 μm porosity induce elongation driven M2-like polarization of human macrophages. Biofabrication 12, 025007. doi:10.1088/1758-5090/ab5f4e
Ueno, M., Lo, C. W., Barati, D., Conrad, B., Lin, T., Kohno, Y., et al. (2020). Interleukin-4 overexpressing mesenchymal stem cells within gelatin-based microribbon hydrogels enhance bone healing in a murine long bone critical-size defect model. J. Biomed. Mater Res. A 108, 2240–2250. doi:10.1002/jbm.a.36982
Van Dyken, S. J., and Locksley, R. M. (2013). Interleukin-4- and interleukin-13-mediated alternatively activated macrophages: Roles in homeostasis and disease. Annu. Rev. Immunol. 31, 317–343. doi:10.1146/annurev-immunol-032712-095906
Vassey, M. J., Figueredo, G. P., Scurr, D. J., Vasilevich, A. S., Vermeulen, S., Carlier, A., et al. (2020). Immune modulation by design: Using topography to control human monocyte attachment and macrophage differentiation. Adv. Sci. (Weinh) 7, 1903392. doi:10.1002/advs.201903392
Wan, X., Zhao, Y., Li, Z., and Li, L. (2022). Emerging polymeric electrospun fibers: From structural diversity to application in flexible bioelectronics and tissue engineering. Exploration 2, 20210029. doi:10.1002/exp.20210029
Wang, J., Liu, D., Guo, B., Yang, X., Chen, X., Zhu, X., et al. (2017a). Role of biphasic calcium phosphate ceramic-mediated secretion of signaling molecules by macrophages in migration and osteoblastic differentiation of MSCs. Acta Biomater. 51, 447–460. doi:10.1016/j.actbio.2017.01.059
Wang, T., Zhang, X., and Bikle, D. D. (2017b). Osteogenic differentiation of periosteal cells during fracture healing. J. Cell Physiol. 232, 913–921. doi:10.1002/jcp.25641
Wang, W., and Yeung, K. W. K. (2017). Bone grafts and biomaterials substitutes for bone defect repair: A review. Bioact. Mater 2, 224–247. doi:10.1016/j.bioactmat.2017.05.007
Wang, X., Wang, Y., Bosshardt, D. D., Miron, R. J., and Zhang, Y. (2018). The role of macrophage polarization on fibroblast behavior-an in vitro investigation on titanium surfaces. Clin. Oral Investig. 22, 847–857. doi:10.1007/s00784-017-2161-8
Wang, X., Yu, Y. Y., Lieu, S., Yang, F., Lang, J., Lu, C., et al. (2013). MMP9 regulates the cellular response to inflammation after skeletal injury. Bone 52, 111–119. doi:10.1016/j.bone.2012.09.018
Wang, Z., Cui, Y., Wang, J., Yang, X., Wu, Y., Wang, K., et al. (2014). The effect of thick fibers and large pores of electrospun poly(ε-caprolactone) vascular grafts on macrophage polarization and arterial regeneration. Biomaterials 35, 5700–5710. doi:10.1016/j.biomaterials.2014.03.078
Wawrzyniak, A., and Balawender, K. (2022). Structural and metabolic changes in bone. Animals (Basel), 12.
Wosik, J., Chen, W., Qin, K., Ghobrial, R. M., Kubiak, J. Z., and Kloc, M. (2018). Magnetic field changes macrophage phenotype. Biophys. J. 114, 2001–2013. doi:10.1016/j.bpj.2018.03.002
Wu, S., Xu, J., Zou, L., Luo, S., Yao, R., Zheng, B., et al. (2021). Long-lasting renewable antibacterial porous polymeric coatings enable titanium biomaterials to prevent and treat peri-implant infection. Nat. Commun. 12, 3303. doi:10.1038/s41467-021-23069-0
Wynn, T. A., and Vannella, K. M. (2016). Macrophages in tissue repair, regeneration, and fibrosis. Immunity 44, 450–462. doi:10.1016/j.immuni.2016.02.015
Xiang, G., Liu, K., Wang, T., Hu, X., Wang, J., Gao, Z., et al. (2021). In situ regulation of macrophage polarization to enhance osseointegration under diabetic conditions using injectable silk/sitagliptin gel scaffolds. Adv. Sci. (Weinh) 8, 2002328. doi:10.1002/advs.202002328
Xie, Y., Hu, C., Feng, Y., Li, D., Ai, T., Huang, Y., et al. (2020). Osteoimmunomodulatory effects of biomaterial modification strategies on macrophage polarization and bone regeneration. Regen. Biomater. 7, 233–245. doi:10.1093/rb/rbaa006
Xu, H., Chai, Q., Xu, X., Li, Z., Bao, W., Man, Z., et al. (2022). Exosome-functionalized Ti6Al4V scaffolds promoting osseointegration by modulating endogenous osteogenesis and osteoimmunity. ACS Appl. Mater Interfaces 14, 46161–46175. doi:10.1021/acsami.2c11102
Xu-Kai, W., Jia-Zhen, Y., and Jian-Xun, D. (2022). Double network-enhanced chiral supramolecular hydrogel to promote osteogenesis. Chin. J. Appl. Chem. 39, 1627.
Yang, C., Zhao, C., Wang, X., Shi, M., Zhu, Y., Jing, L., et al. (2019). Stimulation of osteogenesis and angiogenesis by micro/nano hierarchical hydroxyapatite via macrophage immunomodulation. Nanoscale 11, 17699–17708. doi:10.1039/c9nr05730g
Yang, Z., Yi, P., Liu, Z., Zhang, W., Mei, L., Feng, C., et al. (2022). Stem cell-laden hydrogel-based 3D bioprinting for bone and cartilage tissue engineering. Front. Bioeng. Biotechnol. 10, 865770. doi:10.3389/fbioe.2022.865770
You, J., Zhang, Y., and Zhou, Y. (2022). Strontium functionalized in biomaterials for bone tissue engineering: A prominent role in osteoimmunomodulation. Front. Bioeng. Biotechnol. 10, 928799. doi:10.3389/fbioe.2022.928799
Yue, S., He, H., Li, B., and Hou, T. (2020). Hydrogel as a biomaterial for bone tissue engineering: A review. Nanomaterials (Basel), 10.
Yurekli, B. S., Kocabas, G. U., Aksit, M., Kutbay, N. O., Suner, A., Yurekli, I., et al. (2018). The low levels of bone morphogenic protein-4 and its antagonist noggin in type 2 diabetes. Horm. (Athens) 17, 247–253. doi:10.1007/s42000-018-0041-5
Zhang, J., Shi, H., Zhang, N., Hu, L., Jing, W., and Pan, J. (2020). Interleukin-4-loaded hydrogel scaffold regulates macrophages polarization to promote bone mesenchymal stem cells osteogenic differentiation via TGF-β1/Smad pathway for repair of bone defect. Cell Prolif. 53, e12907. doi:10.1111/cpr.12907
Zhang, J., Tong, D., Song, H., Ruan, R., Sun, Y., Lin, Y., et al. (2022a). Osteoimmunity-regulating biomimetically hierarchical scaffold for augmented bone regeneration. Adv. Mater 34, e2202044. doi:10.1002/adma.202202044
Zhang, W., Wang, N., Yang, M., Sun, T., Zhang, J., Zhao, Y., et al. (2022b). Periosteum and development of the tissue-engineered periosteum for guided bone regeneration. J. Orthop. Transl. 33, 41–54. doi:10.1016/j.jot.2022.01.002
Zhang, Y., Cheng, X., Jansen, J. A., Yang, F., and Van Den Beucken, J. (2019). Titanium surfaces characteristics modulate macrophage polarization. Mater Sci. Eng. C Mater Biol. Appl. 95, 143–151. doi:10.1016/j.msec.2018.10.065
Zhao, C., Qiu, P., Li, M., Liang, K., Tang, Z., Chen, P., et al. (2021a). The spatial form periosteal-bone complex promotes bone regeneration by coordinating macrophage polarization and osteogenic-angiogenic events. Mater Today Bio 12, 100142. doi:10.1016/j.mtbio.2021.100142
Zhao, D. W., Zuo, K. Q., Wang, K., Sun, Z. Y., Lu, Y. P., Cheng, L., et al. (2021c). Interleukin-4 assisted calcium-strontium-zinc-phosphate coating induces controllable macrophage polarization and promotes osseointegration on titanium implant. Mater Sci. Eng. C Mater Biol. Appl. 118, 111512. doi:10.1016/j.msec.2020.111512
Zhao, D., Zhu, T., Li, J., Cui, L., Zhang, Z., Zhuang, X., et al. (2021b). Poly(lactic-co-glycolic acid)-based composite bone-substitute materials. Bioact. Mater 6, 346–360. doi:10.1016/j.bioactmat.2020.08.016
Zhao, Y., Zhang, Z., Pan, Z., and Liu, Y. (2021d). Advanced bioactive nanomaterials for biomedical applications. Exploration 1, 20210089. doi:10.1002/exp.20210089
Zheng, A., Wang, X., Xin, X., Peng, L., Su, T., Cao, L., et al. (2023). Promoting lacunar bone regeneration with an injectable hydrogel adaptive to the microenvironment. Bioact. Mater 21, 403–421. doi:10.1016/j.bioactmat.2022.08.031
Zhou, J., Zhang, Z., Joseph, J., Zhang, X., Ferdows, B. E., Patel, D. N., et al. (2021). Biomaterials and nanomedicine for bone regeneration: Progress and future prospects. Exploration 1, 20210011. doi:10.1002/exp.20210011
Zhu, T., Cui, Y., Zhang, M., Zhao, D., Liu, G., and Ding, J. (2020). Engineered three-dimensional scaffolds for enhanced bone regeneration in osteonecrosis. Bioact. Mater 5, 584–601. doi:10.1016/j.bioactmat.2020.04.008
Zhu, T., Jiang, M., Zhang, M., Cui, L., Yang, X., Wang, X., et al. (2022a). Biofunctionalized composite scaffold to potentiate osteoconduction, angiogenesis, and favorable metabolic microenvironment for osteonecrosis therapy. Bioact. Mater 9, 446–460. doi:10.1016/j.bioactmat.2021.08.005
Keywords: tissue engineering, bone regeneration, biomaterial, macrophage, bone non-union
Citation: Liu Z, Zhu J, Li Z, Liu H and Fu C (2023) Biomaterial scaffolds regulate macrophage activity to accelerate bone regeneration. Front. Bioeng. Biotechnol. 11:1140393. doi: 10.3389/fbioe.2023.1140393
Received: 09 January 2023; Accepted: 26 January 2023;
Published: 02 February 2023.
Edited by:
Jinshan Guo, Southern Medical University, ChinaReviewed by:
Sihan Zhang, Southern Medical University, ChinaXiaoyuan Li, Northeast Normal University, China
Gong Cheng, Harvard University, United States
Copyright © 2023 Liu, Zhu, Li, Liu and Fu. This is an open-access article distributed under the terms of the Creative Commons Attribution License (CC BY). The use, distribution or reproduction in other forums is permitted, provided the original author(s) and the copyright owner(s) are credited and that the original publication in this journal is cited, in accordance with accepted academic practice. No use, distribution or reproduction is permitted which does not comply with these terms.
*Correspondence: Changfeng Fu, ZnVjZkBqbHUuZWR1LmNu