- 1Xijing Hospital, Fourth Military Medical University, Xi’an, China
- 2The Key Laboratory of Biomedical Information Engineering of Ministry of Education, Xi’an Jiaotong University School of Life Science and Technology, Xi’an, China
- 3State Key Laboratory for Mechanical Behavior of Materials, Xi’an Jiaotong University, Xi’an, China
- 4Department of Dermatology, Xijing Hospital, Fourth Military Medical University, Xi’an, China
Background: Wound healing is a complicated process involving multiple cell components and can help the re-establishment of the skin’s barrier function. Previous studies have pointed out that bacterial infection and sustained inflammatory reactions are the main causes of the delay of wound closure and scar formation during wound healing. The effect of current approaches for scar-free wound repair still faces many challenges, and alternative therapeutic methods are urgently needed to be established.
Methods: The basic characteristics of the new-designed nanoparticles were clarified through the characterization of the material. The biocompatibility of the nanoparticles, as well as its effect on fibroblast function, anti-bacterial capacity, inflammation suppressive role, and the underlying mechanism were further verified by a panel of biochemical assays in vitro. Ultimately, pre-clinical rat model was employed to testify its role in wound healing and scar formation in vivo.
Results: Firstly, gallium-modified gelatin nanoparticles loaded with quercetin was successfully established, displaying good biocompatibility and facilitative effect on fibroblast function. In addition, the nanoparticles showed prominent anti-bacterial and inflammation-suppressive effects. What’s more important, the nanoparticles could also induce the polarization of macrophages from M1 to M2 phenotype to exert its inflammatory inhibitory role through TGF-β/Smad signaling pathway. Ultimately, in vivo experiment showed that the nanoparticles could effectively promote wound repair and inhibit scar formation during the process of wound healing.
Conclusion: Taken together, the new nanoparticles have good anti-bacterial and anti-scar formation effects and great potential in the field of skin wound repair, which provides a promising therapeutic strategy for wound treatment.
Introduction
Wound healing is a complicated process involving multiple cell components and can help the re-establishment of the skin’s barrier function (Gurtner et al., 2008). Impaired function of fibroblast, bacterial proliferation, and prolonged activation of inflammatory reactions at the wound site are the major reasons for delayed wound healing and excess scar formation (Morris et al., 2014; Nanditha and Kumar, 2022; Shams et al., 2022). Delayed wound healing and the resultant occurrence of hypertrophic scar (HS) not only leads to a reduction in cosmetic effects, but also might result in impaired function of adjacent joints, greatly affecting patients’ quality of life (QOL). Of note, HS has been identified as one of the major unaddressed functional and psychosocial challenges facing global health (Ogawa et al., 2021). Previously, several agents like corticosteroids, bleomycin and verapamil have been reported to ameliorate scarring during the process of wound healing (Giugliano et al., 2003; Yamamoto, 2006; Atiyeh, 2020). Nevertheless, since that skin wound healing is a complicated process with risks of infection, as well as the detailed mechanism underlying wound healing and scar formation remains far from understood, the effect of current approaches for scar-free wound repair still faces many challenges (Alster, 2003), and alternative therapeutic methods are needed to be established.
In recent years, accumulative evidence has demonstrated that some types of new biomaterials, such as hydrogel nanoparticles, are of great therapeutic potential in wound healing repair and anti-scar treatment. Hydrogel nanoparticles are an emerging biomaterial technology, displaying advantages including relatively larger coverage area, strong absorbability, strong slow-release capacity, and minor side effects, and have great application prospect in tissue repair, targeted drug delivery and regenerative medicine (Huang et al., 2022). It has also been reported that the injectable hydrogel nanoparticle preparation is suitable for closure of irregular wound and wound surface (Tariq et al., 2022), indicating the potential of hydrogel nanoparticle in repairing skin wound. Of note, impaired function of fibroblast, bacterial proliferation, and prolonged activation of inflammatory reactions are among the most important causes of the delay of wound healing and excessive scar formation (Morris et al., 2014; Nanditha and Kumar, 2022; Shams et al., 2022). Therefore, if any hydrogel nanoparticles preparation with the capacity to potentiate fibroblast function, suppress bacterial proliferation and inhibit excess inflammation can be developed, it will be an ideal method for wound treatment with simultaneous effects on promoting wound closure and inhibiting scar formation.
Gallium composite is an international emerging anti-bacterial material (Clarkin et al., 2019). The iron-eating property of bacteria is utilized to make the gallium ion that is highly similar to iron ion enter cells in place of iron, and then disrupt the iron metabolism of bacteria to reduce their survival rate to achieve the anti-bacterial effect (Wang et al., 2020). Of note, the anti-bacterial property of gallium ion has been verified in many studies (Qi et al., 2022; Zheng et al., 2022), and the Food and Drug Administration (FDA) also affirmed that gallium has good anti-bacterial activity and can be used in medicine (Rossato et al., 2022). While gallium ion-coated nanoparticles have been prepared in some studies and used to treat diseases like liver abscess and cancer (Xie et al., 2021; Yang et al., 2021). Their anti-bacterial effects on biological wound remain elusive. Quercetin is a flavonol compound among flavonoids, mainly exists in the form of glycosides, is widely distributed in multiple plants, fruits and traditional Chinese medicine and rick in red wine, and has anti-inflammatory, anti-oxidant, anti-bacterial, anti-cancer and anti-viral effects (Pinheiro et al., 2021). Some studies have suggested that the anti-inflammatory effect of quercetin is associated with its mechanisms of inhibiting the secretion of inflammatory factors and interfering with inflammatory signaling pathways (Chanjitwiriya et al., 2020; Dehghani et al., 2021). In previous studies, quercetin has been used to prevent peritoneal scar adhesion in abdominal surgery (Zeng et al., 2022). Nevertheless, its effect on suppressing sustained inflammation and preventing scar formation during skin wound healing warrants further investigation.
Inflammatory response is greatly implicated in the process of wound healing and scar formation, in which macrophage undergoing specific phenotypic and functional changes plays a cardinal role in all stages of the healing process. The dysregulation of the macrophages’ function is highly correlated with delayed wound healing and scarring (Sim et al., 2022). While the infiltration of the pro-inflammatory macrophage in the early phase of wound healing is necessary for the wound-healing progression, sustained existence of pro-inflammatory macrophages in remodeling phase could delay re-epithelialisation accompanied by an increased frequency of vascular leakage, immature granulation, and the persistence of neutrophils, which all contribute to delayed wound healing and increased scar formation (Ishida et al., 2008). Therefore, to suppress the infiltration of pro-inflammatory macrophage and induce macrophage polarization from M1 to M2 type in late phase is beneficial for wound healing.
In the present study, gallium-modified gelatin nanoparticles loaded with quercetin were firstly established, and its anti-bacterial and healing-promoting effects were testified in vitro. Then, its role in suppressing sustained inflammation and the underlying mechanism was investigated, focusing on the regulation of macrophage polarization. Furthermore, the therapeutic effect of this preparation on skin wound healing was examined in pre-clinical rats in vivo.
Material and methods
Agents, antibodies and animals
Gelatin (Gel), quercetin (QCT), gallium nitrate hydrate, and common chemical reagents were purchased from Sigma-Aldrich (United States). Dulbecco’s modified eagle medium (DMEM) and fetal bovine serum (FBS) were purchased from Gibco (United States). The following primary antibodies were purchased from Abcam (United States): Collagen 1 (COL1) (ab270993), Elastin (ab307151), Arginase 1 (Arg1) (ab203490), TGF beta Receptor II (TGFβR2) (ab259360), Smad2 (ab40855), p-Smad2 (ab280888). The following primary antibodies were purchased from Invitrogen (United States): CD206 (17-2061-82), CD86 (12-0862-82). TGFβR2 inhibitor LY2109761 were purchased from Selleck (United States). Cell viability/cytotoxicity detection kit were purchased from Solarbio (China). TNF-α, MCP-1, TGF-β3, IL-4 ELISA kit were purchased from eBioscience (United States).
Sprague-Dawley (SD) rats were purchased from Air Force Medical University. RAW264.7 cell line was obtained from National Collection of Authenticated Cell Cultures, The Academy of Sciences of China (SCSP-5036). E. coli and (ATCC 8739) and S. aureus (ATCC 6538) were obtained from the American Type Culture Collection (ATCC). All animal procedures were approved by the Experimental Animal Ethics Committee of Air Force Medical University.
Synthesis of QCT@GNP-Ga
GNP was synthesized according to a previous protocol (Xu et al., 2013). Briefly, 0.1 g of gelatin (225 Bloom strength) was dissolved in 10 mL of deionized water at 50°C. The gelatin solution’s pH value was adjusted to 7.0 by 0.1 M NaOH. The nanoparticles forming procedure was conducted by adding acetone dropwise to the gelatin solution under continuous stirring. Then, 200 μL of 40% w/w glyoxal was added and mixed by stirring for 3 h at 100 g to crosslink the nanoparticles. 1 mM glycine was used to quench the surface-accessible aldehyde groups of glyoxal. The nanoparticles were obtained by centrifugation at 10,000 g for 20 min and re-suspended in PBS. QCT was added when the pH of the gelatin solution was adjusted to 7.0. The gallium ionic-GNPs were prepared by placing 3 wt% GNP solution in a grooved mold. When dialysis membrane (MWCO 3500 Da) was covered, 0.1 M Ga(NO3)3 aqueous solution was added. After soaking for 4 h, Ga3+-cross-linked GNPs were finally produced.
Characterization of QCT@GNP-Ga
The chemical group alterations in the QCT@GNP and QCT@GNP-Ga were tested by a Fourier transform infrared (FTIR, Bruker, Germany) spectrometer with a wavenumber range of 4,000–500 cm−1. The morphologies of QCT@GNP and QCT@GNP-Ga samples were recorded by a transmission microscope (TEM, JEM-2100, JEOL, Japan).
Drug release from QCT@GNP-Ga
The medium was prepared with 1 mg/mL lysozyme phosphate-buffered saline (PBS) solution (pH = 7.4, pH = 6.8). 0.5 g of QCT@GNP or QCT@GNP-Ga samples were soaked in 10 mL of the medium, agitating at 100 rpm and 37°C. At certain time intervals (12 h, 24 h, 36 h, 48 h, 60 h, 72 h, 84 h, and 96 h), a 3-mL volume was taken for measurement, and fresh medium was added to replenish. To remove the interference of the BSA, high-performance liquid chromatography was performed to test the concentration of QCT at 355 nm. The accumulated release was calculated to clarify the drug release profile and explore the drug release mechanism.
Cell toxicity examination
The cells were seeded into 48-well plates at a density of 1.5 × 104 cells/well and became adherent in 6 h. Discarded the medium and replaced with 10% of QCT@GNP or QCT@GNP-Ga extract. After 24, 48, and 72 h of co-culture, the cells were stained with calcein-AM/propidium iodide to observe the morphology and proliferation via confocal laser microscopy (Lecia, Germany).
RNA extraction and qRT-PCR
Total RNA was extracted using EZNA Total RNA Kit II (OMEGA Bio-tek) and reverse transcription was performed using PrimeScript RTase (Takara Bio Inc.). The mRNA expression levels were measured with real-time quantitative reverse transcription PCR (qRT-PCR) using Premix Ex Taq (Takara) and standardized according to the endogenous control (β-actin) expression level. The cycle conditions were as follows: 95°C for 2 min, denaturation at 95°C for 5 s, annealing at 55°C for 10 s and finally extension at 72°C for 45 s. All the reactions were conducted in triplicate. The amplification and melt curves were used to identity the consistency of specific PCR product. The results were analyzed by using the 2−ΔΔCT method.
Protein extraction and immunoblotting analysis
After cells were lysed by RIPA buffer (Beyotime) added with PMSF (Beyotime), protein concentration was identified by the BCA method kit (Solarbio). Protein samples were collected using 10% SDS-PAGE (Beyotime) and transferred to the polyvinylidene fluoride membranes (PVDF, Millipore). After blocked with 5% skim milk for 1 h, the membrane was incubated with primary antibodies overnight at 4°C and then with the corresponding horse radish peroxidase (HRP)-conjugated secondary antibody (1:2000 dilution) for 1 h at RT. Finally, enhanced chemiluminescence substrate (ECL kit, Millipore) were used for visualization.
Immunohistochemical staining
Scar tissues were obtained, soaked in 4% paraformaldehyde fixative, and then embedded by paraffin and finally cut into 4 μm slices. The following procedures were performed using biotin-streptavidin peroxidase method (SPlink Detection Kit, ZSGB-Bio). According to the manufacturer’s instruction, the paraffin-embedded slices were dewaxed, rehydrated using graded ethanol diluent, subjected to antigen restoration, incubated with 30% H2O2-CH3OH, and blocked with goat serum. Then the slices were incubated with the corresponding primary antibodies, followed by the biotinylated goat anti-rabbit IgG and HRP-conjugated streptomycin. Finally, Diaminobenzidine (ZSGB-Bio) was used for chromogenic reaction. The slices were observed using optical microscope (Olympus).
Flow cytometry analysis
The cells treated with LPS and GNP-Ga or QCT@GNP-Ga were dissociated and resuspended. Fc block (Biosciences, United States) was used to prevent non-specific binding. All the detecting cells were stained with anti-CD86 and CD-206. Analysis was processed on a flow cytometer (BD Biosciences) with the FlowJo software (Tommy Digital Biology, Tokyo, Japan).
ELISA
Cell supernatants were used to quantify the TNF-α, MCP-1, TGF-β3, and IL-4 with the detection limits of 7 pg/mL. Total protein was examined using a commercial kit (BCA protein assay kit, Pierce, Rockford, IL).
Statistical analysis
Each experiment was performed at least three times, and statistical analyses of the data were performed using unpaired, two-tailed Student’s t-tests built into GraphPad Prism (GraphPad Software 8.0; San Diego, CA, United States). Data were presented as the mean ± S.D. p values of<0.05 were considered statistically significant.
Results
Characterization of QCT@GNP-Ga
The quercetin (QCT)-loaded gelatin nanoparticles (QCT@GNP) were prepared by dissolving, and then gallium ions were crosslinked onto the surface of nanoparticles by ion cross-linking to establish QCT@GNP-Ga (Figure 1A). Firstly, nanoparticles were observed under a transmission electron microscope (TEM), which showed that both QCT@GNP and QCT@GNP-Ga were of uniform and smooth spherical structures. The diameter of QCT@GNP and QCT@GNP-Ga was 14.6 ± 1.4 nm and 16.3 ± 1.9 nm, respectively (Figures 1B, C). Further, the molecular structure of the nanoparticle was detected by Fourier transform infrared spectrum. The results showed that the characteristic peak of gallium ion (Ga3+) was at 1,380 cm−1, while that of QCT was at 1,600 cm−1. The characteristic peaks of QCT@GNP were at 1,600 and 1,680 cm−1. Combined with the characteristics of the three above, the characteristic peaks of QCT@GNP-Ga were at 1,380, 1,600 and 1,680 cm−1. Of note, the characteristic peak of QCT@GNP at 1,600 cm−1 was shifted, indicating that the amido bonds in gelatin nanoparticles were strengthened under the action of gallium ion (Figure 1D). The controlled release of drugs by nanoparticles was further detected, which revealed that both QCT@GNP and QCT@GNP-Ga can be released stably in PBS solution for 96 h. The release curve of QCT@GNP-Ga is gentler (Figure 1E).
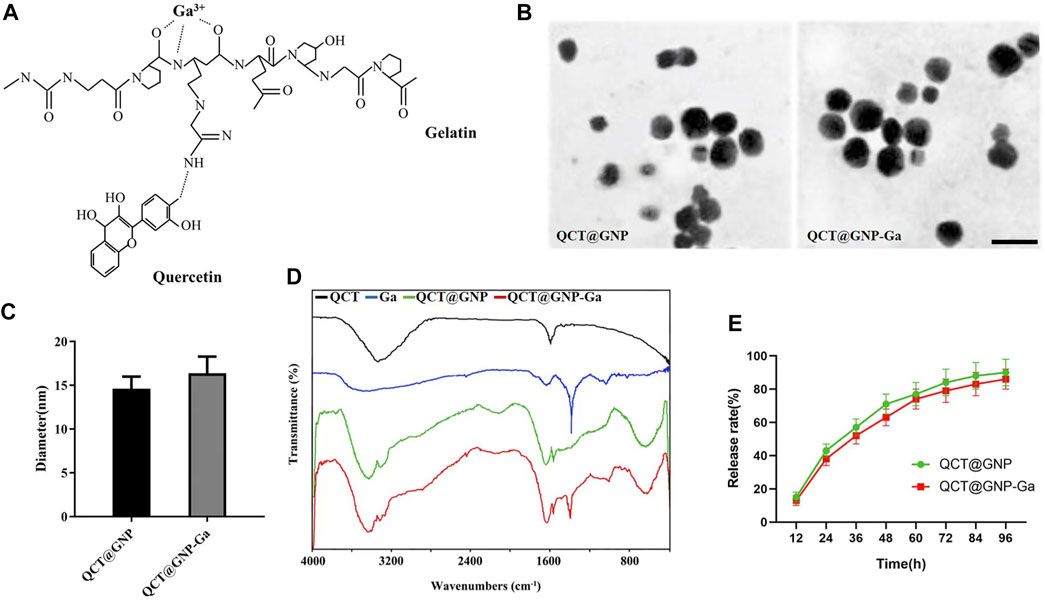
FIGURE 1. Characterization of QCT@GNP-Ga. (A) A schematic view of the design of gallium-modified gelatin nanoparticles loaded with quercetin. (B) Representative image TEM images of QCT@GNP and QCT@GNP-Ga, Scale bar = 20 nm. (C) Particle size of QCT@GNP and QCT@GNP-Ga. (D) FTIR spectra of QCT, Ga, QCT@GNP, and QCT@GNP-Ga. (E) QCT release from QCT@GNP and QCT@GNP-Ga.
Biocompatibility of QCT@GNP-Ga and its effect on function of fibroblasts
QCT@GNP-Ga was added to the medium of the mouse primary skin fibroblasts cultured in vitro to observe its effect on the function of fibroblasts. Firstly, the results of cell survival/death staining showed that there was no significant difference in the cell survival between the QCT@GNP group and the QCT@GNP-Ga group, indicating that both QCT@GNP and QCT@GNP-Ga have good biocompatibility with mouse fibroblasts and are not cytotoxic (Figures 2A, B). Besides, cell wound-healing assay showed that the cell migration in the QCT@GNP group and the QCT@GNP-Ga group at 48 h was significantly greater than that in the GNP group. However, the difference between the QCT@GNP group and the QCT@GNP-Ga group was insignificant (Figures 2C, D). Human skin is constructed with many proteins, among which Type I collagen (COL1) and elastin (ELN) that are derived from fibroblasts and constitute extracellular matrix (ECM) play a key role in providing strength and elasticity to the human skin and body (Lee et al., 2022). The expressions of COL1 and ELN can well reflect the ability of fibroblast to produce ECM that is related to process of wound healing and scar formation (Bui et al., 2022). qPCR and WB analysis showed that 48 h after the treatment with QCT@GNP or QCT@GNP-Ga, both the mRNA and protein levels of COL1 and ELN were significantly higher than those in the GNP group (Figures 2E–G). The above results showed that QCT@GNP-Ga has no cytotoxicity to mouse fibroblasts and can promote fibroblast migration and matrix secretion.
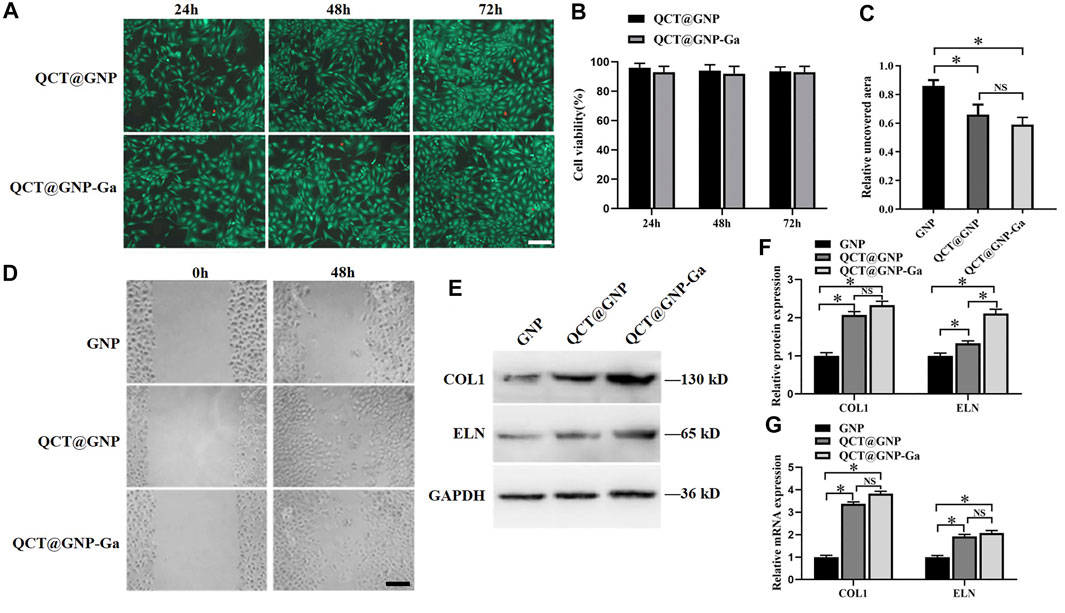
FIGURE 2. Effect of QCT@GNP-Ga on the cell function of primary fibroblasts. (A) Live/dead staining of fibroblasts treated with QCT@GNP or QCT@GNP-Ga. Scale bar = 100 μm. (B) Cell viability of fibroblasts treated with QCT@GNP or QCT@GNP-Ga for 24, 48, and 72 h. (C) The uncovered aera and (D) representative images of wound-healing assay of fibroblasts treated with GNP, QCT@GNP or QCT@GNP-Ga. (E) Western blot analysis and (E) statistical results of COL1, ELN protein expressions in fibroblasts treated with GNP, QCT@GNP or QCT@GNP-Ga. (G) qRT-PCR analysis of COL1, ELN mRNA expressions in fibroblasts treated with GNP, QCT@GNP or QCT@GNP-Ga for 48 h. Data are shown as mean ± SD, *p < 0.05 compared with GNP group.
Anti-bacterial activity of QCT@GNP-Ga
Since that bacterial proliferation at the wound site is one of the major reasons for delayed healing and abnormal scarring (Frykberg and Banks, 2015; Zhang et al., 2022), and Gallium and Quercetin are documented of anti-bacterial property (Hu et al., 2022; Qin J. et al., 2022), we went on to investigate whether QCT@GNP-Ga exerted great anti-bacterial effect. The surface of the agarose medium was coated with QCT@GNP-Ga and then inoculated with S. aureus and E. coli to observe its anti-bacterial activity. After 24 h of constant temperature culture at 37°C, many bacterial colonies were observed on the culture dish in the QCT@GNP group, while only a few bacterial colonies were observed in the QCT@GNP-Ga group. At 48 h, the culture dish in the QCT@GNP was covered with bacterial colonies, while only a few bacterial colonies were formed in the QCT@GNP-Ga group (Figures 3A, B). Further statistics analysis showed that the number of bacterial colonies formed in the QCT@GNP-Ga group at either 24 or 48 h was significantly smaller than that in the QCT@GNP group. The same results were obtained for S. aureus and E. coli (Figures 3C, D).
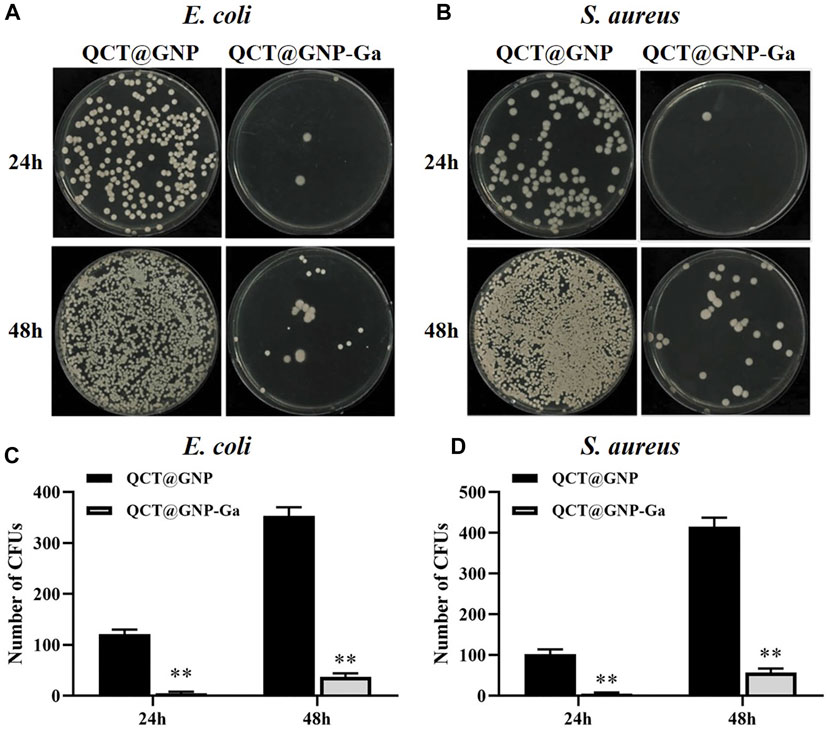
FIGURE 3. Anti-bacterial activity of QCT@GNP-Ga. (A, B) Images and (C, D) statistical results of colony forming unit (CFU) of E. coli and S. aureus treated with QCT@GNP or QCT@GNP-Ga. Data are shown as mean ± SD, *p < 0.05 compared with QCT@GNP group.
Induction of macrophages from M1 to M2 phenotype by QCT@GNP-Ga
It has been demonstrated that a proper inflammatory response is required for skin wound repair (Xu et al., 2010), while prolonged inflammation leads to pathological healing especially scarring (Morris et al., 2014). Macrophages are the most functionally diverse cells for generation and resolution of inflammation by phenotype polarization. Classically-activated macrophages (M1) secrete high levels of pro-inflammatory cytokines like IL-1β, IL-6, and TNF-α to exhibit pro-inflammatory properties (Koh and DiPietro, 2011), whereas alternatively-activated macrophages (M2) that secret high level IL-10 and TGF-β and low-level IL-12 play a role in resolution of inflammation (Gensel and Zhang, 2015). High population of macrophages could lead to sustained inflammation that might delay the process of wound healing (Guo et al., 2016). Therefore, we wondered whether QCT@GNP-Ga played a role in the regulation of macrophage polarization. To this end, mouse RAW264.7 macrophages were cultured in vitro and induced to polarize to M1 by LPS. Afterwards, these macrophages were treated with QCT@GNP-Ga for 5 days to observe its effect on the polarization of mouse macrophages. Flow cytometry analysis revealed that, after the induction by LPS, CD86 was highly-expressed in cells, while CD206 was merely expressed. After the treatment with QCT@GNP-Ga, the expression of CD86 in RAW264.7 cells decreased, while the expression of CD206 increased, indicating the polarization of macrophages from M1 to M2 phenotype (Figure 4A). Further ELISA assay showed that, in response to LPS stimulation, the expression of TNF-α and MCP-1 was significantly lower in the QCT@GNP-Ga group than that in the GNP group and GNP-Ga group, while the expression of TGF-β3 and IL-4 was prominently increased in QCT@GNP-Ga group (Figure 4B). In addition, qRT-PCR analysis showed that in response to LPS stimulation, the mRNA levels of TNF-α, MCP-1 and iNOS were significantly lower in the QCT@GNP-Ga group than those in the GNP group and GNP-Ga group, while the mRNA levels of TGF-β3, IL-4 and Arg-1 were significantly higher than those in the GNP group and GNP-Ga group (Figure 4C). Similarly, immunoblotting analysis proved that the expression of Arg-1 was prominently increased after the treatment with QCT@GNP-Ga (Figures 4D, E). Taken together, these results demonstrated that QCT@GNP-Ga could induce the phenotype switch of macrophages from M1 to M2, so that sustained inflammation could be mitigated to facilitate the process of wound healing.
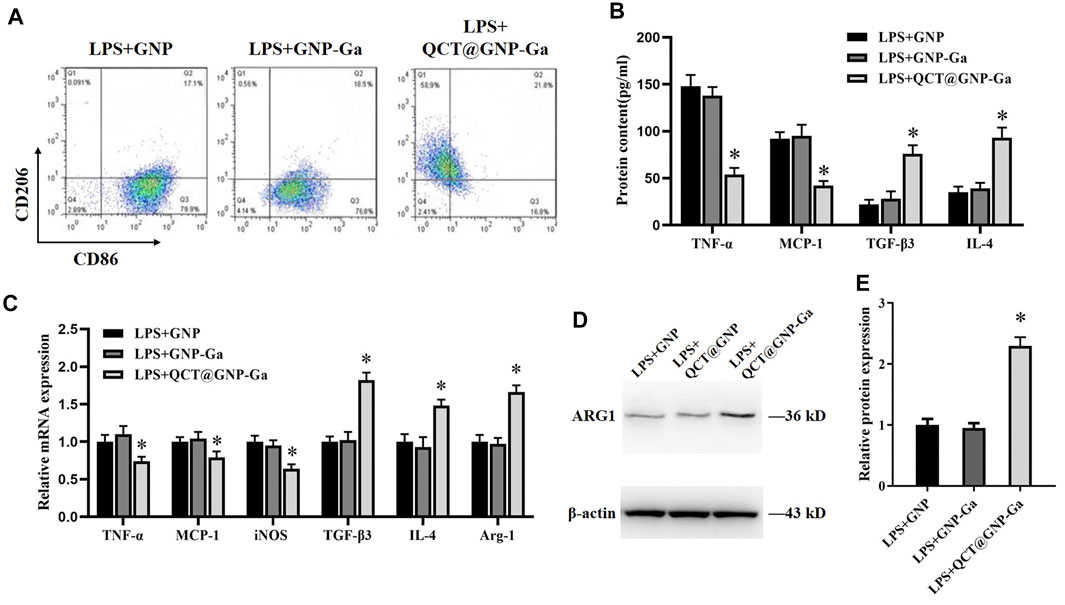
FIGURE 4. Induction of macrophages from M1 to M2 phenotype by QCT@GNP-Ga. (A) Representative images of FCM analysis of LPS induced RAW264.7 cells treated with GNP, GNP-Ga, or QCT@GNP-Ga. (B) EILSA analysis of TNF-α, MCP-1, TGF-β3, and IL-4 protein expressions in LPS induced RAW264.7 cells treated with GNP, GNP-Ga, or QCT@GNP-Ga. (C) qPCR analysis of TNF-α, MCP-1, iNOS, TGF-β3, IL-4, Arg-1 mRNA expressions in LPS induced RAW264.7 cells treated with GNP, GNP-Ga, or QCT@GNP-Ga. (D) Western blot analysis and (E) statistical results of ARG1 protein expressions in LPS induced RAW264.7 cells treated with GNP, GNP-Ga, or QCT@GNP-Ga. Data are shown as mean ± SD, *p < 0.05 compared with LPS + GNP group.
Regulation of polarization of macrophages by QCT@GNP-Ga through TGF-β/Smad pathway
Thereafter, we went on to elucidate the mechanism underlying the role of QCT@GNP-Ga in the regulation of macrophages polarization. To this end, QCT@GNP-Ga was added to the medium of the RAW264.7 cells cultured in vitro for 48 h, after which the expressions of related signaling pathways were detected. It was found that the protein expression of TGFβR2 was increased after the treatment with QCT@GNP-Ga compared with control GNP group (Figures 5A, B). In consistent, immunofluorescence staining analysis also showed that the expression of TGFβR2 was enhanced after the treatment with QCT@GNP-Ga (Figure 5C). In line with the upregulation of TGFβR2 after QCT@GNP-Ga treatment, the phosphorylation of Smad2 was also increased, indicating the activation of TGFβ-Smad signaling by QCT@GNP-Ga, and this effect could be effectively blocked by TGFβR2 inhibitor LY2109761 (Figures 5D, E). Flow cytometry analysis revealed that the expression of CD206 in cells increased after the treatment with QCT@GNP-Ga, while its expression was significantly reduced by the inhibitor LY2109761 (Figure 5F). In addition, while QCT@GNP-Ga treatment induced prominent downregulation of the expression and secretion of TNF-α and MCP-1, as well as the upregulation of the expression and secretion of TGF-β3 and IL-4, co-treatment with TGFβR2 inhibitor LY2109761 was capable of reversing this alteration trend (Figures 5G, H). Therefore, these results suggested that the activation of TGF-β/Smad pathway mediated the role of QCT@GNP-Ga in macrophages polarization from M1 to M2.
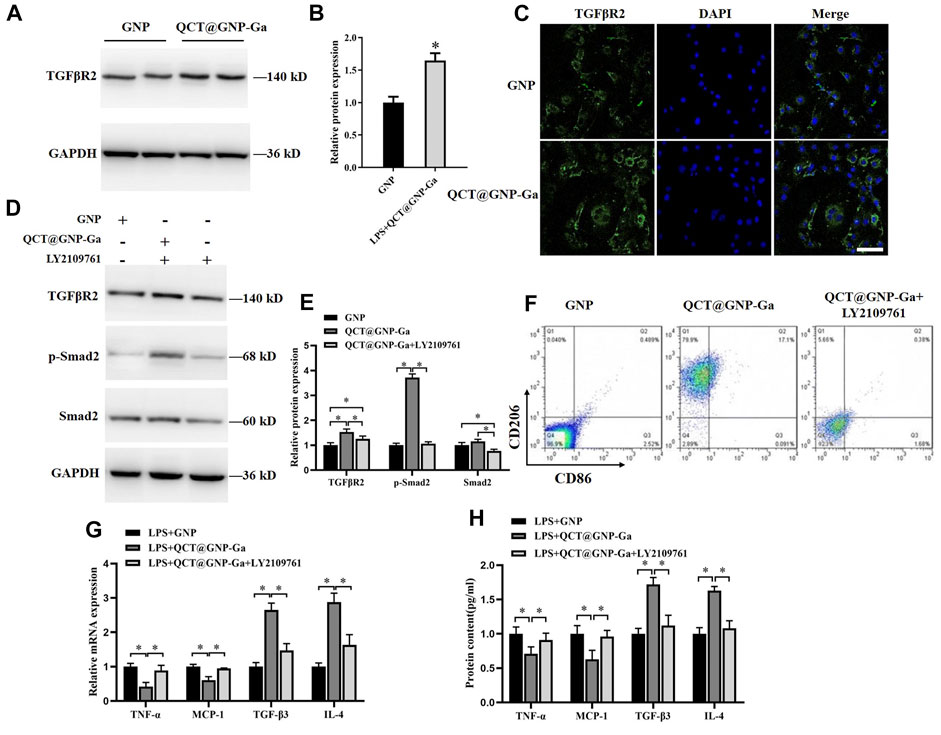
FIGURE 5. Regulation of polarization of macrophages by QCT@GNP-Ga through TGFβ/Smad pathway. (A) Western blot analysis, (B) statistical results, and (C) immunofluorescent staining analysis of TGFβR2 protein expressions in RAW264.7 cells treated with GNP or QCT@GNP-Ga. (D) Western blot analysis and (E) statistical results of TGFβR2, p-Smad2, and Smad2 protein expressions in RAW264.7 cells treated with GNP or QCT@GNP-Ga. (F) Representative images of FCM analysis of RAW264.7 cells treated with GNP or QCT@GNP-Ga or LY2109761. (G, H) Relative expression and secretion of TNF-α, MCP-1, TGF-β3 and IL-4 after the treatment with GNP or QCT@GNP-Ga or with LY2109761 in LPS-stimulated macrophage. Data are shown as mean ± SD, *p < 0.05, Scale bar = 20 μm.
In vivo repair effect of QCT@GNP-Ga on rat skin wound
Given that QCT@GNP-Ga could simultaneously potentiate fibroblast function, exert anti-bacterial property, and induce macrophages polarization from M1 to M2 to suppress sustained inflammation, we proposed that QCT@GNP-Ga might play a cardinal role in facilitating wound healing and restraining scar formation in vivo. A skin wound model was established on the back skin of SD rats, and then the injectable material for repairing skin wound prepared with the gelatin hydrogel loaded with QCT@GNP-Ga. After 14 days of injection, the average rates of wound healing in both the GNP-Ga group and the QCT@GNP-Ga groups were higher than that in the GNP group (Figures 6A, D). The results of HE staining showed that many fibrous scars were formed at wound locations in the GNP group, and local fibrocytes proliferated and were arranged in a compact and disordered manner. In contrary, the scar tissues in the GNP-Ga group were partially reduced, and were further reduced in the QCT@GNP-Ga group (Figure 6B). Concurrent immunohistochemical staining analysis showed that the TNFα-positive cells in the control group were distributed in scar tissues, while the TNFα-positive cells in the QCT@GNP-Ga group were significantly reduced (Figures 6C, E), indicating that the reduction of scar tissues was associated with the inhibition of inflammatory reactions. Taken together, QCT@GNP-Ga exerts great potential in facilitating wound healing and restraining scar formation in pre-clinical rat model.
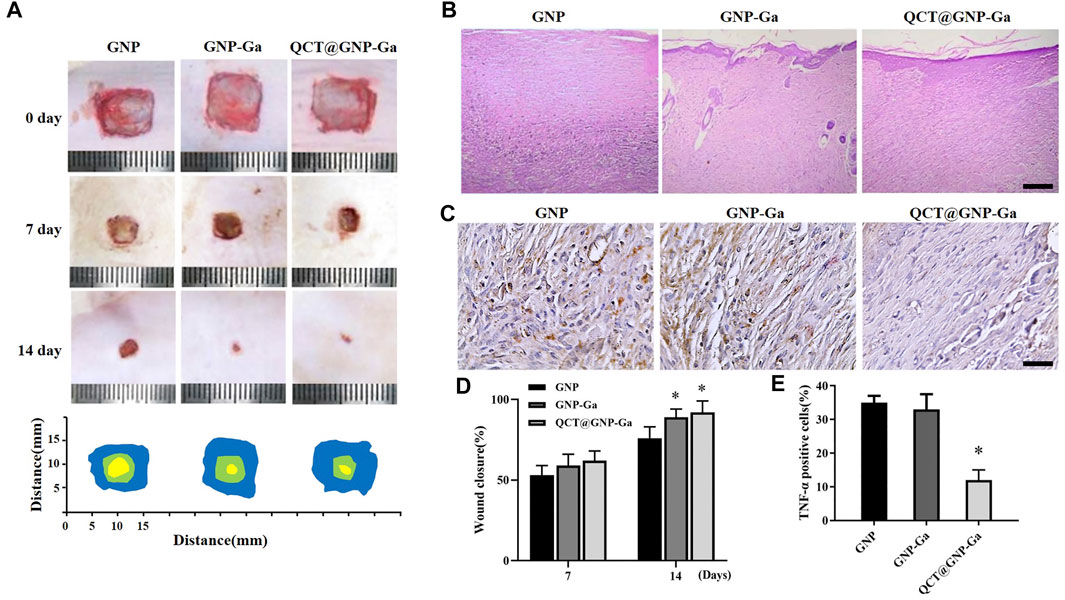
FIGURE 6. In vivo repair effect of QCT@GNP-Ga on rat skin wounds. (A) Representative images of wound healing covered by GNP, GNP-Ga or QCT@GNP-Ga from 0 to 14 days and (D) quantitative results. (B) Representative HE staining images of tissue from healing wounds of GNP, GNP-Ga, or QCT@GNP-Ga group, Scale bar = 100 μm. (C) Representative TNF-α immunohistochemical staining images and (E) quantitative results of tissue from healing wounds of GNP, GNP-Ga or QCT@GNP-Ga group, Scale bar = 20 μm. Data are shown as mean ± SD, *p < 0.05 compared with GNP group.
Discussion
With the improvement of people’s living standard, the attention to efficient wound healing paralleled with reduced scar formation has been gradually increased. In case of an open skin wound, it is often accompanied with bacterial attachment, causing wound infection and resultant severe scars (Leng et al., 2022). In addition, the impaired wound-healing function of fibroblast and sustained inflammatory reactions also restrain the process of wound closure and increase the incidence or severity of scar (Morris et al., 2014; Shams et al., 2022). In the present study, a novel anti-bacterial drug-loaded hydrogel nanoparticle was developed, which could effectively facilitate wound closure, inhibit bacterial proliferation, and ameliorate scar formation, which was related to its role in regulating macrophage polarization, providing a promising choice for the treatment of wound.
Since that nanoparticles have multiple advantages including high drug loading capacity, good biocompatibility, and the ability of the controlling drug release, they have been widely employed in investigations on tissue repair. As a drug carrier or antibacterial active substance, nanoparticles are applied to skin damage repair by mixing with hydrogel to form composite hydrogel (Sun et al., 2021; Burdusel et al., 2022; Qin P. et al., 2022). Silver particles, titanium oxide, gentamicin-containing nanoparticles, curcumin-containing nanoparticles, and other composite hydrogels have been confirmed to have anti-bacterial and wound healing-promoting effects (Javanmardi et al., 2018; Zahiri et al., 2020; Xiang et al., 2022). In recent years, gallium ion has been found to be advantageous in the field of antibacterial materials (Vinuesa and McConnell, 2021). Due to the unique anti-microbial mechanism of gallium, it can overcome the problem of resistance to traditional antibiotics, such as the prevention of drug absorption due to the permeability of bacterial cell wall. Gallium ions can imitate the metabolic pathway of iron ions to promote cell absorption, whereas cannot be reduced like iron ions (Kurtuldu et al., 2022). Therefore, the proliferation of bacteria is inhibited when the oxidation-reduction process necessary for the synthesis of the DNA and protein of bacteria is interrupted. In addition to the recognized broad-spectrum bactericidal activity of gallium ions, small doses of gallium ions can also suppress inflammatory reactions (Ma and Fu, 2010; Goss et al., 2018). Besides, some studies have demonstrated that gallium ions can also promote collagen synthesis and cell migration, thus facilitating the process of wound healing (Kircheva and Dudev, 2019; Castilla-Amoros et al., 2020). In this study, our data proved that the gallium ion-modified gelatin nanoparticles were efficient in promoting the wound-healing function of fibroblast, suppressing bacterial proliferation and inhibiting inflammatory reactions, providing an experimental foundation for wound treatment.
Quercetin is flavonoid rich in a variety of foods and has multiple biological characteristics, including anti-inflammatory reaction, anti-oxidative and some other properties (Liu et al., 2019; Mi et al., 2022). Some studies have pointed out that quercetin can reduce the generation of TNF-α, IL-6 and IL-1 in mononuclear U937 cells induced by LPS (Okoko and Oruambo, 2009). In addition, quercetin can inhibit the expressions of inflammation-related genes in RAW264.7 macrophages (Cui et al., 2019). The study by Gupta et al. (2016) proved that quercetin can effectively ameliorate the eosinophilic airway inflammation in allergic asthma. Moreover, Guan et al. (2021) demonstrated that quercetin can relieve rheumatoid arthritis (RA) by promoting the apoptosis of neutrophils. Supplementary to these previous reports, our data for the first time proved that quercetin was also effective in inhibiting the inflammatory reactions during skin scar formation. Inflammatory response participates in wound healing and scar formation, and macrophages that undergo phenotypic and functional changes play a critical role. The persistent existence of pro-inflammatory M1 macrophage in regional skin could prominently delay would healing (Ishida et al., 2008; Sim et al., 2022). To suppress the infiltration of pro-inflammatory macrophage and induce macrophage polarization from M1 to M2 type in late phase is beneficial for wound healing and reduced scar formation. To be specific, quercetin regulated the polarization of macrophages towards M2 through TGF-β/Smad signaling pathway to inhibit inflammation and thereby reduce scar formation, further supporting that the intervention of macrophages polarization might be a useful strategy to reduce scar formation risk during the process of wound healing.
Conclusion
In the present study, a new gallium ion-modified drug-loaded hydrogel nanoparticle was developed aiming to ameliorate infection and scar formation during the process of wound healing. The anti-bacterial, healing-promoting and scar formation-inhibitory effects of the nanoparticles were verified both in vitro and in vivo, with particular emphasis on the regulation of macrophages polarization via TGF-β/Smad pathway to suppress sustained inflammatory reactions at wounds. Our data demonstrated that gallium-modified gelatin nanoparticles loaded with quercetin could promote skin wound healing via the regulation of bacterial proliferation and macrophage polarization, and this preparation might be a promising choice to treat wound and suppress scar formation simultaneously, which needs further confirmation by clinical trial in the future.
Data availability statement
The raw data supporting the conclusion of this article will be made available by the authors, without undue reservation.
Ethics statement
The animal study was reviewed and approved by Laboratory Animal Welfare and Ethics Committee of Fourth Military Medical University.
Author contributions
NY: Methodology, investigation, formal analysis, writing—Original draft. NS: Methodology, investigation, writing—Original draft. ZY: Conceptualization, resources, methodology. HL: Conceptualization, supervision, resources, funding acquisition. WG: Conceptualization, writing—Original draft, writing—Review and editing, supervision, funding acquisition.
Funding
This work was supported by Natural Science Foundation of China (52150221), Natural Science Foundation of Shaanxi Province (2021JQ-015), Natural Science Foundation of Jiangsu Province (BK20200242), and Postdoctoral Science Foundation of China (2021M692546).
Conflict of interest
The authors declare that the research was conducted in the absence of any commercial or financial relationships that could be construed as a potential conflict of interest.
The handling editor, KL, declared a shared affiliation with the authors NS and HL at the time of review.
Publisher’s note
All claims expressed in this article are solely those of the authors and do not necessarily represent those of their affiliated organizations, or those of the publisher, the editors and the reviewers. Any product that may be evaluated in this article, or claim that may be made by its manufacturer, is not guaranteed or endorsed by the publisher.
References
Alster, T. (2003). Laser scar revision: Comparison study of 585-nm pulsed dye laser with and without intralesional corticosteroids. Dermatol Surg. 29 (1), 25–29. doi:10.1046/j.1524-4725.2003.29024.x
Atiyeh, B. S. (2020). Nonsurgical management of hypertrophic scars: Evidence-based therapies, standard practices, and emerging methods. Aesthetic Plast. Surg. 44 (4), 1320–1344. doi:10.1007/s00266-020-01820-0
Bui, V. D., Son, S., Xavier, W., Nguyen, V. Q., Jung, J. M., Lee, J., et al. (2022). Dissolving microneedles for long-term storage and transdermal delivery of extracellular vesicles. Biomaterials 287, 121644. doi:10.1016/j.biomaterials.2022.121644
Burdusel, A. C., Gherasim, O., Andronescu, E., Grumezescu, A. M., and Ficai, A. (2022). Inorganic nanoparticles in bone healing applications. Pharmaceutics 14 (4), 770. doi:10.3390/pharmaceutics14040770
Castilla-Amoros, L., Stoian, D., Pankhurst, J. R., Varandili, S. B., and Buonsanti, R. (2020). Exploring the chemical reactivity of gallium liquid metal nanoparticles in galvanic replacement. J. Am. Chem. Soc. 142, 19283–19290. doi:10.1021/jacs.0c09458
Chanjitwiriya, K., Roytrakul, S., and Kunthalert, D. (2020). Quercetin negatively regulates IL-1β production in Pseudomonas aeruginosa-infected human macrophages through the inhibition of MAPK/NLRP3 inflammasome pathways. PLoS One 15 (8), e0237752. doi:10.1371/journal.pone.0237752
Clarkin, O. M., Wu, B., Cahill, P. A., Brougham, D. F., Banerjee, D., Brady, S. A., et al. (2019). Novel injectable gallium-based self-setting glass-alginate hydrogel composite for cardiovascular tissue engineering. Carbohydr. Polym. 217, 152–159. doi:10.1016/j.carbpol.2019.04.016
Cui, S., Wu, Q., Wang, J., Li, M., Qian, J., and Li, S. (2019). Quercetin inhibits LPS-induced macrophage migration by suppressing the iNOS/FAK/paxillin pathway and modulating the cytoskeleton. Cell Adh Migr. 13, 1–12. doi:10.1080/19336918.2018.1486142
Dehghani, F., Sezavar Seyedi Jandaghi, S. H., Janani, L., Sarebanhassanabadi, M., Emamat, H., and Vafa, M. (2021). Effects of quercetin supplementation on inflammatory factors and quality of life in post-myocardial infarction patients: A double blind, placebo-controlled, randomized clinical trial. Phytother. Res. 35, 2085–2098. doi:10.1002/ptr.6955
Frykberg, R. G., and Banks, J. (2015). Challenges in the treatment of chronic wounds. Adv. Wound Care (New Rochelle) 4, 560–582. doi:10.1089/wound.2015.0635
Gensel, J. C., and Zhang, B. (2015). Macrophage activation and its role in repair and pathology after spinal cord injury. Brain Res. 1619, 1–11. doi:10.1016/j.brainres.2014.12.045
Giugliano, G., Pasquali, D., Notaro, A., Brongo, S., Nicoletti, G., D'andrea, F., et al. (2003). Verapamil inhibits interleukin-6 and vascular endothelial growth factor production in primary cultures of keloid fibroblasts. Br. J. Plast. Surg. 56, 804–809. doi:10.1016/s0007-1226(03)00384-9
Goss, C. H., Kaneko, Y., Khuu, L., Anderson, G. D., Ravishankar, S., Aitken, M. L., et al. (2018). Gallium disrupts bacterial iron metabolism and has therapeutic effects in mice and humans with lung infections. Sci. Transl. Med. 10 (460), eaat7520. doi:10.1126/scitranslmed.aat7520
Guan, F., Wang, Q., Bao, Y., and Chao, Y. (2021). Anti-rheumatic effect of quercetin and recent developments in nano formulation. RSC Adv. 11, 7280–7293. doi:10.1039/d0ra08817j
Guo, Y., Lin, C., Xu, P., Wu, S., Fu, X., Xia, W., et al. (2016). AGEs induced autophagy impairs cutaneous wound healing via stimulating macrophage polarization to M1 in diabetes. Sci. Rep. 6, 36416. doi:10.1038/srep36416
Gupta, K., Kumar, S., Gupta, R. K., Sharma, A., Verma, A. K., Stalin, K., et al. (2016). Reversion of asthmatic complications and mast cell signalling pathways in BALB/c mice model using quercetin nanocrystals. J. Biomed. Nanotechnol. 12, 717–731. doi:10.1166/jbn.2016.2197
Gurtner, G. C., Werner, S., Barrandon, Y., and Longaker, M. T. (2008). Wound repair and regeneration. Nature 453, 314–321. doi:10.1038/nature07039
Hu, Y., Huang, D., Li, Y., Li, Z., Cai, X., and Wang, F. (2022). Investigation on characterization of novel anti-bacterial chitosan/gelatin composite membranes loaded with quercetin via electrophoretic deposition. J. Biomater. Sci. Polym. Ed., 1–19. doi:10.1080/09205063.2022.2145701
Huang, S., Hong, X., Zhao, M., Liu, N., Liu, H., Zhao, J., et al. (2022). Nanocomposite hydrogels for biomedical applications. Bioeng. Transl. Med. 7, e10315. doi:10.1002/btm2.10315
Ishida, Y., Gao, J. L., and Murphy, P. M. (2008). Chemokine receptor CX3CR1 mediates skin wound healing by promoting macrophage and fibroblast accumulation and function. J. Immunol. 180, 569–579. doi:10.4049/jimmunol.180.1.569
Javanmardi, S., Ghojoghi, A., Divband, B., and Ashrafi, J. (2018). Titanium dioxide nanoparticle/gelatin: A potential burn wound healing biomaterial. Wounds 30, 372–379.
Kircheva, N., and Dudev, T. (2019). Novel insights into gallium's mechanism of therapeutic action: A DFT/PCM study of the interaction between Ga(3+) and ribonucleotide reductase substrates. J. Phys. Chem. B 123, 5444–5451. doi:10.1021/acs.jpcb.9b03145
Koh, T. J., and Dipietro, L. A. (2011). Inflammation and wound healing: The role of the macrophage. Expert Rev. Mol. Med. 13, e23. doi:10.1017/S1462399411001943
Kurtuldu, F., Mutlu, N., Boccaccini, A. R., and Galusek, D. (2022). Gallium containing bioactive materials: A review of anticancer, antibacterial, and osteogenic properties. Bioact. Mater 17, 125–146. doi:10.1016/j.bioactmat.2021.12.034
Lee, S., Choi, Y. J., Lee, S., Kang, K. S., Jang, T. S., and Kim, K. H. (2022). Protective effects of withagenin A diglucoside from Indian ginseng (withania somnifera) against human dermal fibroblast damaged by TNF-α stimulation. Antioxidants (Basel) 11 (11), 2248. doi:10.3390/antiox11112248
Leng, T., Wang, Y., Cheng, W., Wang, W., Qu, X., and Lei, B. (2022). Bioactive anti-inflammatory antibacterial metformin-contained hydrogel dressing accelerating wound healing. Biomater. Adv. 135, 212737. doi:10.1016/j.bioadv.2022.212737
Liu, X., Sun, N., Mo, N., Lu, S., Song, E., Ren, C., et al. (2019). Quercetin inhibits kidney fibrosis and the epithelial to mesenchymal transition of the renal tubular system involving suppression of the Sonic Hedgehog signaling pathway. Food Funct. 10, 3782–3797. doi:10.1039/c9fo00373h
Ma, Z., and Fu, Q. (2010). Comparison of the therapeutic effects of yeast-incorporated gallium with those of inorganic gallium on ovariectomized osteopenic rats. Biol. Trace Elem. Res. 134, 280–287. doi:10.1007/s12011-009-8472-0
Mi, Y., Zhong, L., Lu, S., Hu, P., Pan, Y., Ma, X., et al. (2022). Quercetin promotes cutaneous wound healing in mice through Wnt/β-catenin signaling pathway. J. Ethnopharmacol. 290, 115066. doi:10.1016/j.jep.2022.115066
Morris, M. W., Allukian, M., 3R. D., Herdrich, B. J., Caskey, R. C., Zgheib, C., Xu, J., et al. (2014). Modulation of the inflammatory response by increasing fetal wound size or interleukin-10 overexpression determines wound phenotype and scar formation. Wound Repair Regen. 22, 406–414. doi:10.1111/wrr.12180
Nanditha, C. K., and Kumar, G. S. V. (2022). Bioactive peptides laden nano and micro-sized particles enriched ECM inspired dressing for skin regeneration in diabetic wounds. Mater Today Bio 14, 100235. doi:10.1016/j.mtbio.2022.100235
Ogawa, R., Dohi, T., Tosa, M., Aoki, M., and Akaishi, S. (2021). The latest strategy for keloid and hypertrophic scar prevention and treatment: The nippon medical school (NMS) protocol. J. Nippon. Med. Sch. 88, 2–9. doi:10.1272/jnms.JNMS.2021_88-106
Okoko, T., and Oruambo, I. F. (2009). Inhibitory activity of quercetin and its metabolite on lipopolysaccharide-induced activation of macrophage U937 cells. Food Chem. Toxicol. 47, 809–812. doi:10.1016/j.fct.2009.01.013
Pinheiro, R. G. R., Pinheiro, M., and Neves, A. R. (2021). Nanotechnology innovations to enhance the therapeutic efficacy of quercetin. Nanomater. (Basel) 11, 2658. doi:10.3390/nano11102658
Qi, X., Zhang, H., Li, Y., Zhang, X., Ma, H., and Zhang, L. (2022). Nonfouling and antibacterial zwitterionic contact lenses loaded with heme-mimetic gallium porphyrin for treating keratitis. Langmuir 38, 14335–14344. doi:10.1021/acs.langmuir.2c02433
Qin J., J., Li, M., Yuan, M., Shi, X., Song, J., He, Y., et al. (2022). Gallium(III)-Mediated dual-cross-linked alginate hydrogels with antibacterial properties for promoting infected wound healing. ACS Appl. Mater Interfaces 14, 22426–22442. doi:10.1021/acsami.2c02497
Qin P., P., Tang, J., Sun, D., Yang, Y., Liu, N., Li, Y., et al. (2022). Zn(2+) cross-linked alginate carrying hollow silica nanoparticles loaded with RL-QN15 peptides provides promising treatment for chronic skin wounds. ACS Appl. Mater Interfaces 14, 29491–29505. doi:10.1021/acsami.2c03583
Rossato, L., Arantes, J. P., Ribeiro, S. M., and Simionatto, S. (2022). Antibacterial activity of gallium nitrate against polymyxin-resistant Klebsiella pneumoniae strains. Diagn Microbiol. Infect. Dis. 102, 115569. doi:10.1016/j.diagmicrobio.2021.115569
Shams, F., Moravvej, H., Hosseinzadeh, S., Mostafavi, E., Bayat, H., Kazemi, B., et al. (2022). Overexpression of VEGF in dermal fibroblast cells accelerates the angiogenesis and wound healing function: In vitro and in vivo studies. Sci. Rep. 12, 18529. doi:10.1038/s41598-022-23304-8
Sim, S. L., Kumari, S., Kaur, S., and Khosrotehrani, K. (2022). Macrophages in skin wounds: Functions and therapeutic potential. Biomolecules 12 (11), 1659. doi:10.3390/biom12111659
Sun, L., He, L., Wu, W., Luo, L., Han, M., Liu, Y., et al. (2021). Fibroblast membrane-camouflaged nanoparticles for inflammation treatment in the early stage. Int. J. Oral Sci. 13, 39. doi:10.1038/s41368-021-00144-2
Tariq, L., Arafah, A., Ali, S., Beigh, S., Dar, M. A., Dar, T. U. H., et al. (2022). Nanogel-Based transdermal drug delivery system: A therapeutic strategy with under discussed potential. Curr. Top. Med. Chem. 23, 44–61. doi:10.2174/1568026622666220818112728
Vinuesa, V., and Mcconnell, M. J. (2021). Recent advances in iron chelation and gallium-based therapies for antibiotic resistant bacterial infections. Int. J. Mol. Sci. 22, 2876. doi:10.3390/ijms22062876
Wang, X., Liu, X., Bi, P., Zhang, Y., Li, L., Guo, J., et al. (2020). Electrochemically enabled embedded three-dimensional printing of freestanding gallium wire-like structures. ACS Appl. Mater Interfaces 12, 53966–53972. doi:10.1021/acsami.0c16438
Xiang, J., Bai, Y., Huang, Y., Lang, S., Li, J., Ji, Y., et al. (2022). A zwitterionic silver nanoparticle-incorporating injectable hydrogel with a durable and efficient antibacterial effect for accelerated wound healing. J. Mater Chem. B 10, 7979–7994. doi:10.1039/d2tb01493a
Xie, T., Qi, Y., Li, Y., Zhang, F., Li, W., Zhong, D., et al. (2021). Ultrasmall Ga-ICG nanoparticles based gallium ion/photodynamic synergistic therapy to eradicate biofilms and against drug-resistant bacterial liver abscess. Bioact. Mater 6, 3812–3823. doi:10.1016/j.bioactmat.2021.03.032
Xu, J. H., Gao, F. P., Liu, X. F., Zeng, Q., Guo, S. S., Tang, Z. Y., et al. (2013). Supramolecular gelatin nanoparticles as matrix metalloproteinase responsive cancer cell imaging probes. Chem. Commun. (Camb) 49, 4462–4464. doi:10.1039/c3cc00304c
Xu, Z., Xu, H., Ploplis, V. A., and Castellino, F. J. (2010). Factor VII deficiency impairs cutaneous wound healing in mice. Mol. Med. 16, 167–176. doi:10.2119/molmed.2009.00171
Yamamoto, T. (2006). Bleomycin and the skin. Br. J. Dermatol 155, 869–875. doi:10.1111/j.1365-2133.2006.07474.x
Yang, N., Gong, F., Zhou, Y., Hao, Y., Dong, Z., Lei, H., et al. (2021). A general in-situ reduction method to prepare core-shell liquid-metal/metal nanoparticles for photothermally enhanced catalytic cancer therapy. Biomaterials 277, 121125. doi:10.1016/j.biomaterials.2021.121125
Zahiri, M., Khanmohammadi, M., Goodarzi, A., Ababzadeh, S., Sagharjoghi Farahani, M., Mohandesnezhad, S., et al. (2020). Encapsulation of curcumin loaded chitosan nanoparticle within poly (epsilon-caprolactone) and gelatin fiber mat for wound healing and layered dermal reconstitution. Int. J. Biol. Macromol. 153, 1241–1250. doi:10.1016/j.ijbiomac.2019.10.255
Zeng, H., Liu, X., Zhang, Z., Song, X., Quan, J., Zheng, J., et al. (2022). Self-healing, injectable hydrogel based on dual dynamic covalent cross-linking against postoperative abdominal cavity adhesion. Acta Biomater. 151, 210–222. doi:10.1016/j.actbio.2022.08.030
Zhang, C., Yang, D., Wang, T. B., Nie, X., Chen, G., Wang, L. H., et al. (2022). Biodegradable hydrogels with photodynamic antibacterial activity promote wound healing and mitigate scar formation. Biomater. Sci. 11 (1), 288–297. doi:10.1039/d2bm01493a
Keywords: Gallium, nanoparticles, Quercetin, wound healing, macrophage
Citation: Yang N, Shi N, Yao Z, Liu H and Guo W (2023) Gallium-modified gelatin nanoparticles loaded with quercetin promote skin wound healing via the regulation of bacterial proliferation and macrophage polarization. Front. Bioeng. Biotechnol. 11:1124944. doi: 10.3389/fbioe.2023.1124944
Received: 15 December 2022; Accepted: 13 January 2023;
Published: 26 January 2023.
Edited by:
Jin Yan, Xi’an Jiaotong University, ChinaReviewed by:
Zhimin Tian, Northwestern Polytechnical University, ChinaJinran Lin, Fudan University, China
Copyright © 2023 Yang, Shi, Yao, Liu and Guo. This is an open-access article distributed under the terms of the Creative Commons Attribution License (CC BY). The use, distribution or reproduction in other forums is permitted, provided the original author(s) and the copyright owner(s) are credited and that the original publication in this journal is cited, in accordance with accepted academic practice. No use, distribution or reproduction is permitted which does not comply with these terms.
*Correspondence: Hang Liu, bGl1aGFuZ0B4anR1LmVkdS5jbg==; Weinan Guo, Z3Vvd25AZm1tdS5lZHUuY24=