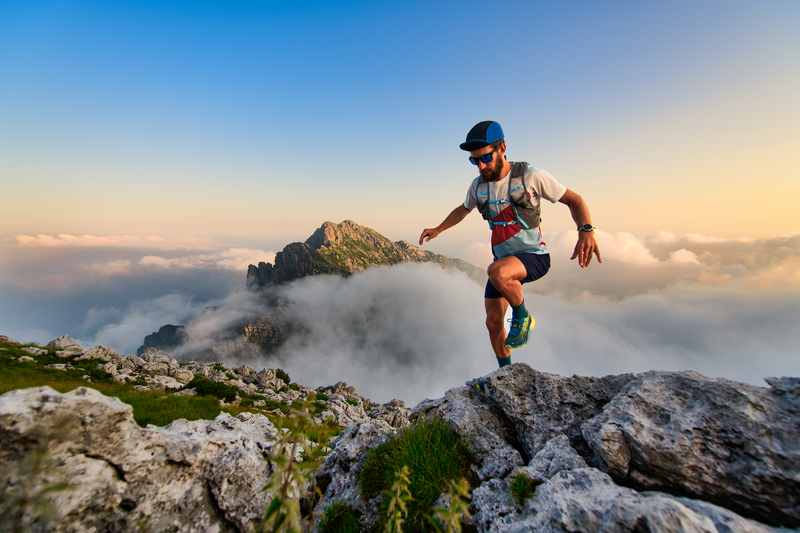
95% of researchers rate our articles as excellent or good
Learn more about the work of our research integrity team to safeguard the quality of each article we publish.
Find out more
REVIEW article
Front. Bioeng. Biotechnol. , 25 April 2023
Sec. Biosafety and Biosecurity
Volume 11 - 2023 | https://doi.org/10.3389/fbioe.2023.1124100
This article is part of the Research Topic Policy and Regulation in Bioengineering and Biotechnology View all 12 articles
Regulation of research on microbes that cause disease in humans has historically been focused on taxonomic lists of ‘bad bugs’. However, given our increased knowledge of these pathogens through inexpensive genome sequencing, 5 decades of research in microbial pathogenesis, and the burgeoning capacity of synthetic biologists, the limitations of this approach are apparent. With heightened scientific and public attention focused on biosafety and biosecurity, and an ongoing review by US authorities of dual-use research oversight, this article proposes the incorporation of sequences of concern (SoCs) into the biorisk management regime governing genetic engineering of pathogens. SoCs enable pathogenesis in all microbes infecting hosts that are ‘of concern’ to human civilization. Here we review the functions of SoCs (FunSoCs) and discuss how they might bring clarity to potentially problematic research outcomes involving infectious agents. We believe that annotation of SoCs with FunSoCs has the potential to improve the likelihood that dual use research of concern is recognized by both scientists and regulators before it occurs.
In 2022, the National Institutes of Health (NIH) and Office of Science and Technology Policy (OSTP) began a process to evaluate the effectiveness of dual-use research oversight in the United States and determine whether the current approach sufficiently addresses future potential threats in biological research (Tabak and Jorgenson, 2022). This review encompasses three policies: the March 2012 United States Government Policy for Oversight of Life Sciences Dual Use Research of Concern (United States Government, 2012), the September 2014 United States Government Policy for Institutional Oversight of Life Sciences Dual Use Research of Concern (United States Government, 2014), and the December 2017 Framework for Guiding Funding Decisions about Proposed Research Involving Enhanced Potential Pandemic Pathogens (P3CO Framework) (Department of Health and Human Services, 2017). The March 2012 and September 2014 dual use research of concern (DURC) policies are complementary and will be considered together since they are both based on a shared list of pathogens and experiments that are subject to oversight. Under the DURC policies, research that is either conducted or funded by a federal agency on fifteen pathogens and toxins (Table 1) that is “reasonably anticipated” to produce one of seven experimental outcomes (Table 2) are subject to review by the funding agency. The list of pathogens is based on those deemed to be Tier 1 high-consequence biological threats by the Federal Select Agent Program (FSAP).
The list-based approach of the DURC policies has been criticized for its static nature and lack of coverage of potentially risky research with pathogens that are not on the Select Agent Tier 1 list. A 2018 study by the National Academies of Science, Engineering, and Medicine highlighted the variety of ways in which biological threats beyond those on this specific list could be generated thanks to our improved understanding of which genotypes generate potentially harmful phenotypes and the diffusion of the expertise, techniques, and technologies needed to apply this knowledge to develop modified genomes with enhanced harmful attributes (National Academies of Science, Engineering, and Medicine, 2018).
The P3CO Framework provides for oversight of research funded by the Department of Health and Human Services (HHS) that is “reasonably anticipated” to enhance the lethality and/or transmissibility of a potential pandemic pathogen (PPP) which is a pathogen capable of “wide and uncontrollable spread” in human populations and able to cause “significant morbidity and/or mortality” in such a population. This type of research is known as “gain of function” since it results in a microbe with enhanced virulence, pathogenicity, transmissibility, or other attribute that poses a higher risk to the host population than the naturally occurring strain. Unlike the DURC policy, the P3CO Framework is not limited to a specified list of pathogens. However, both policies rely on an interpretation of which types of laboratory experiments can be “reasonably anticipated” to have the effects or outcomes covered by both policies. The Government Accountability Office (GAO) has highlighted the lack of a standard for judging what is “reasonably anticipated” as a weakness in the oversight of dual-use research (Government Accountability Office GAO, 2023).
Several of the more controversial “gain of function” experiments were the results of failures by scientists and/or funding agencies to “reasonably anticipate” the outcome of the proposed research. The canonical example is the insertion of the gene coding for the murine interleukin-4 (IL-4) immunomodulator into ectromelia virus (mousepox) by Australian scientists in 2001. This experiment resulted in a strain of the virus that was uniformly lethal to both susceptible and genetically resistant mice and, even more worryingly, killed 60% of mice vaccinated against the virus (Jackson et al., 2001). According to the authors of the study, “this came as a complete surprise and was totally unexpected.” However, it has been argued that previous work on IL-4 and poxviruses was such that the “available evidence fully predicted” that a recombinant mousepox IL-4 virus would be more virulent (Müllbacher and Lobigs, 2001), enhancing the harmful consequences of the agent [Table 2], disrupting host immunity [Table 2], and increasing the susceptibility of the host population to the virus [Table 2].
A more recent example also shows that potentially perilous engineering is not always identified in advance. In 2014, EcoHealth Alliance proposed a research project to the NIH to modify coronaviruses, including MERS and bat-related coronaviruses, to evaluate the pandemic risk they posed. NIH determined that these experiments did not fall under the scope of the P3CO Framework because the modifications were “not expected to generate viruses that would be more transmissible or more virulent in humans” despite this being the stated goal of the project. [Letter from NIH Director Francis Collins to Senator Charles Grassley, 28 July20211.
The NIH was criticized when it became apparent that the resulting chimeric viruses were, in fact, more virulent in humanized animal models than the original strain2. In contrast, the Defense Advanced Research Projects Agency (DARPA) rejected a proposal from EcoHealth Alliance to fund similar research with chimeric coronaviruses using genes associated with the spike protein of SARS-related coronaviruses found in bats3.
Some countries use taxonomy-based lists for export controls, but only a handful of other countries use such lists to exercise oversight for dual-use research as the United States does. In the United Kingdom, the three largest funders of life sciences research—the Biotechnology and Biological Sciences Research Council, the Medical Research Council, and the Wellcome Trust—review research proposals for dual-use potential using the same list of experiments of concern as in the United States. However, the dual-use review process in the United Kingdom is applicable to all life sciences research, not just that conducted with a list of pathogens as in the United States4. Canada requires research institutions to develop plans for managing biorisks, including dual-use issues, and processes for scientists to report to their institution if their research could result in the creation of a “human pathogen with increased virulence, pathogenicity, or communicability, that is resistant to preventative or therapeutic treatments, or produces a toxin with increased toxicity”5. Australia does not have an explicit dual-use oversight policy, but research with infectious agents and creation of genetically modified organisms, including as a result of “gain of function” experiments, is subject to monitoring and reporting6.
We have been engaged in a multi-year effort to understand the risks of biological sequences and the sorts of threats they pose to humanity. Those that are likely to cause problems if moved to another organism have been called sequences of concern (National Research Council, 2010) which we abbreviate as SoCs. Techniques to transfer sequences from one microbe to another and alter them in ways both minor and major are widely available. We contend that SoCs are not confined to microbes that have historically been feared for their capacity for weaponization, but rather are found in all parasites that have evolved with specific host organisms to cause disease in those organisms. These are commonly called pathogens.
Thousands of published investigations in microbial pathogenesis have provided ample reason to think that the direct activity of SoCs on constituent molecules of the host is the primary driver of successful infection and pathogenesis. In the absence of these microbial sequences—which associate with and modify host molecules—infection cannot occur. There are also SoCs that act indirectly—by either altering molecules of the parasite or facilitating the operation of direct-acting SoCs (e.g., bacterial secretion system components and chaperones), but these are of secondary importance. Lastly there is the consideration of gene expression. If neither the direct- nor the indirect-acting SoCs of a bacterial or eukaryotic parasite are expressed in sufficient abundance or in a timely, coordinated manner, then the microbe will not be able to successfully exploit the host organism. We also recognize that there are transcriptional, translational, and post-translational influences. There can also be epigenetic effects modulating expression. We are not asserting that SoCs are the only contributors to pathogenic phenotypes, merely that, for microbial parasites, they are the essential contributors to such phenotypes for host organisms with normal immune systems and intact barriers. Without these sequences, the encoding microbes could not cause disease in the healthy, immune-normal hosts with which they co-evolved as pathogens.
In our earlier publication we described how we reviewed thousands of papers to find thousands of virulence factors from bacterial, viral, and eukaryotic parasites that were good candidates for SoCs. We pondered if a sequence, following transfer to another microbe, would be likely to enhance its ability to colonize a susceptible host, increasing the pathological consequences of infection. If the answer was ‘probably yes’, then we detailed its host-relevant activities and incorporated it in our dataset. For ∼100 sequences, the authors demonstrated the ability of the transferred sequence to exhibit the same or similar pathogenic function in a different microbe that was previously associated with the expression of that sequence in the original microbe (Godbold et al., 2022).
We developed a controlled vocabulary to describe SoCs called Functions of Sequences of Concern (FunSoCs) (Godbold et al., 2022). We used it for both machine learning and bioinformatic software (Balaji et al., 2022). With FunSoCs, we attempted to capture both the activity and the consequences of these sequences on the host during infection. We identified four types of host damage caused by SoCs: (1) cytotoxicity or cell membrane disruption, (2) tissue degradation, (3) organ disabling, and (4) inflammation. We also described types of innate immune subversion resulting from SoC activity including: (i) suppression of host immune signaling (with many subtypes), (ii) resisting phagocytosis, (iii) neutralizing host complement, (iv) countering antimicrobial peptide, (v) resisting oxidative killing, (vi) neutralizing host immunoglobulin, (vii) defeating host cytokine, and (viii) inhibiting antigen presentation. Two types of direct SoC activity are characteristic of nearly all infectious organisms: adherence and invasion. There are two functions of direct-acting SoCs peculiar to intracellular pathogens: movement within a host cell and niche creation. Finally, some SoCs provide pathogens the ability to disseminate within the host organism by subverting host barriers. In addition, we note which of nine areas of host cell biology (transcription, ubiquitination, etc.) are targeted by SoCs (Godbold et al., 2022).
A valuable adjunct to the consequentialist focus of FunSoCs is the pathogenesis gene ontology (PathGO) developed by researchers at the Johns Hopkins University Applied Physics Laboratory7. PathGO consists of ∼170 terms which are rooted in biological process and molecular function terms of the Gene Ontology resource (Ashburner et al., 2000; Gene Ontology Consortium et al., 2021). PathGO terms identify the host molecules and pathways that are the targets of SoC activity, and we have employed these to further specify SoCs in our dataset.
In the following sections we address the relative abundance of SoCs in pathogen genomes with reference to SARS-CoV-2 and Bacillus anthracis. We discuss whether some SoCs are worse or more dangerous than others with respect to their host-affecting properties and provide some examples of SoCs with multiple functions from bacterial, viral, and eukaryotic pathogens of humans. Next, we emphasize the importance of immune subverting SoCs as these sequences appear critical for producing host susceptibility to microbes. Then we consider the appropriate criteria for determining what microbes from which SoCs should be appropriated. In the final sections we grapple with how annotated SoCs can be used to guide biorisk management decisions. We provide a rubric (Table 6) that exemplifies how they might be applied to the USG dual-use research of concern policy to simplify decision-making processes. We close by drawing out implications of using SoCs to supplement the current taxonomic list-based approach for dual-use research oversight.
SoCs are more abundant in viral genomes as a fraction of the total genetic material than in other parasites. Of microbes capable of causing disease in humans, viruses are the most genetically compact. Even the largest of these (poxviruses) possess genomes two to three times smaller than that of the smallest bacterial pathogen (Mycoplasma). They contain, proportionally, more sequences that confound host immunity than bacterial, fungal, or protozoal parasites. Viruses abound in sequences disrupting innate immune signaling (Godbold et al., 2022). The larger viral pathogens for humans have DNA genomes and can allocate single sequences to one or just a few functions like soaking up host cytokines to blunt the local immune response (Dunlop et al., 2003; Seet et al., 2003; Alvarez-de Miranda et al., 2021). RNA viruses are necessarily more compact with each protein serving many functions. SARS-CoV-2 is an example. Of the ∼27 sequences that are translated into proteins (Jungreis et al., 2021), at least 24 are SoCs and 18 of those suppress host cellular immune defenses including Membrane (M), Nsp1, Nsp3, Nsp5, Nsp6, Nsp7, Nsp10, Nsp12, Nsp13, Nsp14, Nsp15, Nucleocapsid (N), Orf3a, Orf6, Orb7b, Orf8, Orf9b, and Spike (S). The hypervariable Orf8 is the only one of these that has so far been demonstrated to be dispensable (Zinzula, 2021). The immune subverting activity for the sequences that suppress host immunity are summarized in Table 3.
Our annotations suggest that, in contrast to viruses, the great majority of the encoded sequences of nonviral microbes play no role in pathogenesis. These SoCs comprise, at most, a few per cent of the sequences of bacterial, fungal, and protozoal pathogen genomes. Some microbes do not have nearly so many. Out of ∼5,800 genes on a single chromosome and two plasmids, Bacillus anthracis encodes about a dozen proteins enabling pathogenesis including the ‘big three’ of protective antigen, edema factor, and lethal factor. The annotated proteins are shown in Table 4.
We think the following assertions are generally true about the relative danger of SoCs in microbial pathogenesis. First, SoCs that act directly on a host molecule are more concerning than those that act indirectly. Second, SoCs that have a damaging effect are more concerning than those that only provide adhesive, invasive, or disseminating capacities. We think that SoCs enabling dissemination of a pathogen that has already colonized a host are more concerning than adhesive or invasive SoCs. Third, SoCs that only provide within-cell motility or the ability to form an intracellular niche are the SoCs of lowest concern of the direct-acting SoCs. Fourth, SoCs that have multiple functions are more concerning than those that have a single function. Some sequences that enable adhesion can also subvert immunity. A subset of SoCs with many functions are detailed in Table 5. Immune subverting SoCs are a special case that we address in the next section.
Why are some organisms susceptible to infection by some microbes but not others? Why are immune-compromised persons subject to infection with a broader range of parasites than immune-normal persons? Why do defects in immune detectors and immune effectors of an organism allow microbes that are normally incapable of infection to become competent for infection and pathogenesis? A single amino acid change in an immune effector can mean the difference between life and death during challenge with a virus (Andoniou et al., 2014). The study of human immune deficiencies shows the critical importance of components of innate immunity for defense against the specific, usually narrow, set of parasites against which they defend (Casanova, 2015a; 2015b; Li et al., 2017).
What these phenomena have in common is a host with intact barriers and an immune system that fends off microbes that lack direct-acting sequences evolved to either counter or disrupt key components of the innate immune system of that host (Godbold et al., 2022). These direct-acting sequences, expressed in a combination that varies by parasitic microbe, produce a state of susceptibility in a host, allowing colonization by the parasite (Wickham et al., 2007; Kurupati et al., 2010). Such a set of immune subverting mechanisms is not generic. A parasite with a given set of innate immune subverting mechanisms is not able to subvert every immune system, but just the limited grouping of species with which it co-evolved as a pathogen. Its encoded molecular armamentarium is specific to counter a relatively narrow set of organism-specific innate signaling pathways and effectors and exploit a specific host biology—including barrier breaching. These encoded sequences are how the pathogen makes a host susceptible. Obviously jumps into new species can happen. In these cases, though, the new species, if it is not immune compromised, is always related to the original species with respect to the innate immune system. A mouse pathogen innate immune subverting mechanisms may (or may not) function on the human ortholog of the mouse innate immune protein. But the sequences encoded by a microbe that make plants susceptible to that particular pathogen by subverting the plant innate immune defenses do not, and cannot, make mammals susceptible to that same microbe because of the substantial differences in the innate immune systems of plants and mammals. Yersinia pestis infects two different sorts of hosts: insects (fleas) and mammals. The bacterium encodes different sets of sequences to exploit each host and employs temperature-based regulation to switch between them (Vadyvaloo et al., 2010).
We are asserting that the immune subverting mechanisms employed by a specific microbial pathogen are what produce susceptibility in the (typically) narrow range of hosts parasitized by that microbe. Whatever pathogenesis follows from this infection is not just a function of the parasite, but rather an emergent property of the gestalt of the host-parasite interactions shaped by the development of the adaptive immune response (Casadevall and Pirofski, 2003; Pirofski and Casadevall, 2015). For these reasons, we think it is possible that immune subverting mechanisms may be the ‘worst’ of SoCs since they essentially enable infection and appear (to us) to be the difference between pathogenic and non-pathogenic species. We have documented and annotated a few thousand SoCs from parasites of (mostly) humans, including over 500 that subvert host innate immunity. But this is probably just a tithe of the immune subverting SoCs encoded by human pathogens. While we think that the available evidence points strongly in the direction of these sequences being necessary for infection by making the host susceptible, at present this is merely a hypothesis that requires testing.
Every nonviral species in biology serves as a host to its own subset of microbial pathogens. And all those pathogens have sequences that directly exploit the biology of their host. But these are not necessarily SoCs. Why not? Because sequences of concern are only ‘concerning’ if they are from pathogens capable of infecting humans and other species that humans rely upon for survival. The specific bacteriophage sequences enabling exploitation of strains of Salmonella or Listeria are not SoCs because humans do not care about the wellbeing of those bacteria. The sequences that allow bacteriophage to exploit these bacteria cannot be used to cause harm to mammalian or crop plant hosts and so would not be considered SoCs. Sequences encoding virulence factors that are designated SoCs should be documented and annotated only from pathogens that afflict humans, our livestock, and our crop plants (Godbold et al., 2022).
As mentioned above, this requires a broadening of microbes beyond those placed on select agent lists. These lists are generally composed of organisms and toxins that have been weaponized or are viewed as being weaponizable. But sequences that effectively interact with human molecules, attach to host cells, invade them, subvert immunity, enable dissemination, and generate pathology are not limited to weaponizable microbes. If microbes that cause disease in immune-compromised people are included, there are at least 1,500 species that probably encode SoCs (Godbold et al., 2022).
That said, we are not sure where the line on infectious microbes should be drawn: all microbes capable of causing disease in any human, however immune-compromised? That would require mining SoCs from opportunistic pathogens. Or should SoCs be taken only from microbes capable of causing disease in immune-normal people? The latter would neglect documenting the many and varied SoCs that have been elegantly investigated in such ‘conditional’ pathogens as Pseudomonas aeruginosa.
We mention SoCs from pathogens of crop plants above, but we acknowledge that is the weakest area of our SoC annotation effort. This is the case for several reasons. The literature for microbial pathogenesis in plants lags at least 2 decades behind that of mammals. We have documented fewer than 300 SoCs from plant pathogens (viral, bacterial, and fungal). Our terminology for functions of SoCs from plant pathogens needs supplementation/improvement. Why? Because the innate immune system of plants, while at least as complex as that of mammals, is substantially different. The goals and ‘principles’ of immune defense are the same, but the individual cases and host molecules effecting the defensive effort are distinct. We are not as familiar with them. We plan on improving our understanding and our annotation effort for plant pathogen SoCs.
As the NSABB reviews the conduct of dual-use research oversight, it should consider how to incorporate our growing knowledge of SoCs into the biorisk management regime to ensure that life sciences research is conducted safely, securely, and responsibly. In the following section, we suggest several ways in which SoCs could be used to guide biorisk management decisions.
Since neither the DURC policies nor P3CO Framework provide guidance for scientists to judge whether proposed research is “reasonably anticipated” to result in a modified microbe with enhanced pathogenic properties, we propose leveraging our annotated SoCs as one indicator which could trigger greater scrutiny. We think our conception of SoCs provide clearer guidance than what currently exists. If the sequence being manipulated in the investigation is a direct-acting sequence of concern, and it is being expressed in an organism capable of causing disease in humans, then that work may require a higher level of oversight. This will depend on the likelihood that the resulting manipulated microbe poses a greater risk of infection or transmission than the unmodified microbe. So how might that risk be better adjudicated using SoCs annotated with FunSoCs and PathGO terms?
Among the experiments of concern listed in USG DURC policy (Table 2), our conception of SoCs and their functions (FunSoCs) can help illuminate potential risks. Research that involves any one of three research activities: (i) transfer of a SoC to a different pathogen, (ii) alteration of a SoC such that the existing abilities of the original pathogen might be enhanced, or (iii) transfer of an SoC to a nonpathogenic microbe would trigger oversight. Our reflections on how FunSoCs might be used to better understand DURC follow and are summarized in Table 6.
Damaging SoCs: We briefly detailed four categories of damaging SoCs in our previous work (Godbold et al., 2022) and recapitulated them above. Inserting damaging SoCs into a microbe could violate Table 2 as it would be expected to enhance the harmful consequence of the agent. Such a result might also follow the alteration of a damaging SoC in its native microbe.
Immune subverting SoCs: As we discuss above, SoCs that subvert innate immunity may be more consequential than damaging SoCs. Results of experiments involving addition of these sequences to other microbes as well as modifications that might enhance their immune subverting abilities are also the most difficult to anticipate prior to the experiment. Such could “disrupt immunity against the agent” [Table 2] or “enhance the susceptibility of a host population to the agent” [Table 2]. It could also “increase the harmful consequence of the agent” [Table 2]. Alterations in some poxviral immune-evading sequences can change the host tropism of the virus [Table 2] (Bratke et al., 2013; Rahman and McFadden, 2017; 2020). Of course, these modifications depend on the experimental system and could very well be allowed after review. The study of immune subverting mechanisms of microbes in experimental infections of host organisms has produced numerous and important breakthroughs in our understanding of immunity.
Adhesins and Invasins: Adhesive properties are particularly abundant in biology. Adhesins are the molecules which primarily condition what cell types and what taxa are targeted by an infectious agent. As a result, their transplantation into a new organism might enable a change in host tropism [Table 2] or enhance the susceptibility of a new host population [Table 2]. Likewise, alterations of adhesins with the intention of altering host cell tropism should trigger a review. For viruses and for many other infectious agents that have an intracellular life cycle, the principal attachment protein (adhesin) is also responsible for viral fusion and subsequent cellular invasion. But there are dozens of bacterial invasins, not also adhesins, which manipulate extracellular matrix molecules or the cytoskeleton thereby leading to invasion. It is conceivable that altering these within a pathogen could lead to changes in host tropism [Table 2] or enhance the susceptibility of a new host population [Table 2]. Expressing adhesins/invasins from pathogens in nonpathogenic species will not generally violate DURC rules as they do not, by themselves, make a nonpathogenic microbe pathogenic (Schubert et al., 2004; Uchiyama et al., 2006; Pisano et al., 2012; Schmidgen et al., 2014).
Dissemination factors: There are disparate modes of action for dissemination factors, but the effect is that the infectious agent can spread within the host organism beyond what would be possible in the absence of the dissemination factor. This often occurs through the temporary subversion of host barriers. Addition of a foreign dissemination factor to an existing pathogen could lead to consequences that could increase the harmful effects of the agent [Table 2], increase the ability of the agent to disseminate in the host [Table 2], or even enhance the susceptibility of a host population to the agent [Table 2]. Modifications to a dissemination factor could conceivably affect each of these as well. Expression of a dissemination factor in a nonpathogen would be unlikely to make it pathogenic.
Of course, additions or alterations of SoCs to study the mechanism(s) would be less risky if performed in a microbe that was either not competent to replicate or otherwise incapable of causing human infection. If a SoC-based biosecurity regime were adopted, development of safer systems to study SoC function should be a focus of funding agencies.
As the global biorisk landscape evolves, it is necessary to update biorisk management policies and practices. As the NIH and OSTP reviews US dual-use research oversight policy, we think our approach to categorizing the functions of SoCs based on the published literature and using these as an aid for considering outcomes of organismal manipulation is a valuable addition and will strengthen existing policy. The rubric provided in Table 6, which maps the functions of SoCs onto different classes of experiments to suggest which DURC categories might be involved, could be helpful for considering the consequences of microbial modifications.
The United States has not provided any guidance for how to judge when the standard of “reasonably anticipated,” as used by the DURC policies and P3CO Framework, is met. This lack of detail and ambiguous terminology can be confusing for both researchers submitting proposals as well as scientists and funding agency officials involved in the peer review process. Therefore, NIH and OSTP should consider recommending that inserting or modifying SoCs with certain functions could be “reasonably anticipated” to lead to an enhanced phenotype covered by either set of policies. While this rule of thumb would not be the only determinant of whether an experiment was covered by DURC, it would increase the likelihood that potentially concerning research is subject to review under the appropriate policy. This approach will be particularly useful if NIH and OSTP adopts the recommendations from the National Science Advisory Board for Biosecurity (NSABB) to expand the scope and coverage of the P3CO and DURC policies. For example, NSABB proposed reducing the threshold for oversight of experiments with potential pandemic pathogens from those that are reasonably anticipated to generate a highly virulent or transmissible pathogen to those likely to generate a moderately virulent or transmissible pathogen. NSABB also recommended that the scope of the DURC policy be expanded from Tier 1 Select Agents to all human, animal, and plant pathogens (National Science Advisory Board for Biosecurity, 2023). These recommendations, when taken together, will subject a much broader swathe of pathogen research subject to oversight, necessitating the development of tools that can aid researchers and review entities in determining if proposed experiments could be “reasonably anticipated” to generate enhanced pathogens that require the implementation of risk mitigation measures prior to or following the research.
A similar lacuna in guidance for researchers on how to identify potential dual-use research exists in other countries that exercise some degree of oversight of dual-use research such as Australia, Canada, and the United Kingdom. These countries might also benefit from adopting functional criteria (like FunSoCs) into their education and awareness-raising activities to help scientists identify potential dual-use research. In addition, funding agencies in these countries could use FunSoCs as part of their screening process for grant proposals to determine if the research poses any dual-use risks that require mitigation.
For microbes that are increasingly synthetic, having their constituent sequences drawn from an expanding set of organisms, a screening approach based on taxonomy is likely to be of decreasing utility. In such cases a standard list of ‘bad sequences’ should be helpful in determining what microbes are likely to be concerning. An accurate computational assessment of the infectiousness of a synthetic microbe is not currently possible nor is it likely to be in the next decade. We think our work and that of others can provide pointers for how such as assessment might be attempted (Gemler et al., 2022; Godbold et al., 2022). Our criteria for functions of sequences of concern were described in our earlier publication and are available to the scientific community. Here we offer them as a useful framework for assessing risk in the context of dual-use research of concern.
SoCs cannot replace taxonomic lists of ‘bad bugs’, particularly in the case of viruses pathogenic for humans, which must remain part of any policy framework. But the addition of SoCs categorized by functions necessary for pathogenesis provides a useful supplement to such lists. The transfer of such sequences and their modification in ways that can be reasonably anticipated to enhance their damaging, disseminating, adhesive, invasive, or immune subverting effects should be noted in research proposals. Such a list of SoCs might allow the de-regulation of thousands of sequences from bacterial and eukaryotic pathogens that are presently deemed controlled. As suggested in Table 4 for Bacillus anthracis, over 99.5% of its 5,800 sequences play no distinct role in pathogenesis. Documenting and regulating the sequences that enable pathogenesis in nonviral organisms make it easier for researchers to investigate, without oversight, the biology of the remaining (and overwhelming) majority.
The revised guidance on DNA synthesis screening issued by the Department of Health and Human Services is undertaking a shift from a pathogen-based to a sequence-based approach8. Under the previous guidance, DNA synthesis providers were only required to screen orders against the genomes of a list of regulated pathogens. Under the revised guidance, “sequences that contribute to toxicity or pathogenicity” are considered sequences of concern that are covered by the guidance even if these are not encoded by a regulated biological agent. The NIH and OSTP could explore the desirability and feasibility of applying the broadly defined “sequences of concern” by the new HHS guidance to DURC oversight.
How can we be sure that the sequences enabling pathogenesis for these disease-causing microbes have been sufficiently investigated to find them all? This is something we cannot know, though there has been a great deal of work on most of the microbes found on select agent lists. Investing in research on the less well-investigated pathogens would help ensure that the most important pathogenic sequences are characterized. In addition, knowledge of the commonalities of sequences enabling pathogenesis that are a consequence of categorizing them might drive development of pathogen-agnostic therapeutics that may be able to neutralize widely shared mechanisms of pathogenesis.
One strategy to mitigate the risk of research involving SoCs is for funding authorities to encourage researchers to develop more, and more suitable, nonpathogenic microbial chassis to support the safe discovery of SoC functions. Once approved, these chassis could be used with decreased oversight. The use of non-replicating pseudoviruses can also be encouraged as a safer alternative to the insertion or modification of SoCs in pathogenic, replicating viruses.
A standardized and official list of SoCs with a set of approved annotations should be devised by governments whose scientists are involved in microbial pathogenesis research. How this list should be selected, maintained, and used is something that will need to be resolved. The process should involve consultation among experts in infectious diseases and policy as well as relevant biodefense professionals. The first question for such a group involves deciding which host taxa needing protection should be selected. Humans are the primary concern, but animals and plants that dominate a country’s agriculture should probably also be considered. Once the hosts are established, the pathogens that afflict these hosts can be determined. Then SoCs will be documented from this list of pathogens.
The availability of such a list and the type of information it should provide is also something to be decided. Should it be an open list of sequence names? A list of sequence names with accession numbers? The names, accession numbers, and a tabular list of problematic functions (i.e., damaging, immune subverting, adhesive, etc.)? Should terse but specific descriptions of pathogenic activity such as FunSoCs and PathGO be associated with each SoC? Or a more detailed description of how it interacts with host molecules? Should citations/references of the primary or secondary literature be required to justify the functional determinations for each sequence?
Who should have access to these lists? Should it be publicly available to the scientific community at large? Should only institutional review entities responsible for implementing DURC oversight of research conducted at their institution have access to this information? Should different groups have access to lists of differing comprehensiveness? The utility of such a tool for enhancing DURC oversight needs to be balanced with the information hazards presented by an accessible compilation of sequences that enable pathogenesis. Those making these decisions will be threading the needle to best serve the interests of public safety, open research, and international security.
Thoughtful researchers who work with pathogenic microbes are usually aware of the hazards involved in introducing changes into sequences involved in pathogenesis. We think SoCs annotated with FunSoCs will bring further clarity to help ascertain when more care should be taken in experiments, especially in fully replication-competent organisms. We believe that SoCs can be a useful component of the regulatory regime that governs sequences acceptable for insertion and alteration in pathogenic agents. We have delineated bioengineering situations that could be ‘reasonably anticipated’ to improve the disease-causing capacity of pathogens. We believe that these guidelines have the potential to reduce the risk of accidentally generating an ‘improved’ pathogen while promoting awareness of the phenotype effects of potentially concerning genotypic changes. We think considering SoCs by function improves the probability that potentially concerning research is subject to the appropriate level of oversight to ensure that such research is conducted safely, securely, and responsibly.
GG conceived, drafted and revised the article as well as collected, analyzed, and interpreted the data. GK conceived, drafted and revised the article. AK and MS collected and analyzed data, as well as critiqued and revised the article. FH, SA, TT, KT, and JS critiqued and revised the article. FH, TT, and KT acquired funding support for the research. TT and KT equally exercised oversight and supervision for the research.
GG, FH, AK, MS, KT, and TT were partially supported by the Fun GCAT program from the Office of the Director of National Intelligence (ODNI), Intelligence Advanced Research Projects Activity (IARPA), via the Army Research Office (ARO) under federal award no. W911NF-17-2-0089. GG, KT, and TT were also partially supported by the Centers for Disease Control (CDC) contract 75D30121C11180.
The views and conclusion contained herein are those of the authors and should not be interpreted as necessarily representing the official policies or endorsements, either expressed or implied, of the ODNI, IARPA, ARO, CDC, or the U.S. Government.
Authors GD, FH, AK, MS, SA, and KT were employed by Signature Science LLC.
The remaining authors declare that the research was conducted in the absence of any commercial or financial relationships that could be construed as a potential conflict of interest.
All claims expressed in this article are solely those of the authors and do not necessarily represent those of their affiliated organizations, or those of the publisher, the editors and the reviewers. Any product that may be evaluated in this article, or claim that may be made by its manufacturer, is not guaranteed or endorsed by the publisher.
1https://www.grassley.senate.gov/imo/media/doc/national_institutes_of_health_to_grassley_-_covid_origins_grant_oversight.pdf.
2https://theintercept.com/2021/09/09/covid-origins-gain-of-function-research/and https://theintercept.com/2021/10/21/virus-mers-wuhan-experiments/.
3https://theintercept.com/2021/09/23/coronavirus-research-grant-darpa/.
4https://cms.wellcome.org/sites/default/files/wtp059491.pdf.
5https://www.canada.ca/en/public-health/programs/consultation-biosafety-guideline-dual-use-life-science-research/document.html.
6https://www.nhmrc.gov.au/file/18130/download?token=anGdkE4f.
7https://github.com/jhuapl-bio/pathogenesis-gene-ontology.
8https://aspr.hhs.gov/legal/syndna/Pages/default.aspx.
Abrami, L., Reig, N., and van der Goot, F. G. (2005). Anthrax toxin: The long and winding road that leads to the kill. Trends Microbiol. 13, 72–78. doi:10.1016/j.tim.2004.12.004
Addetia, A., Lieberman, N. A. P., Phung, Q., Hsiang, T.-Y., Xie, H., Roychoudhury, P., et al. (2021). SARS-CoV-2 ORF6 disrupts bidirectional nucleocytoplasmic transport through interactions with Rae1 and Nup98. mBio 12, e00065–21. doi:10.1128/mBio.00065-21
Agrawal, A., Lingappa, J., Leppla, S. H., Agrawal, S., Jabbar, A., Quinn, C., et al. (2003). Impairment of dendritic cells and adaptive immunity by anthrax lethal toxin. Nature 424, 329–334. doi:10.1038/nature01794
Agrawal, A., and Pulendran, B. (2004). Anthrax lethal toxin: A weapon of multisystem destruction. Cell Mol. Life Sci. 61, 2859–2865. doi:10.1007/s00018-004-4251-4
Aktories, K., and Just, I. (2005). Clostridial Rho-inhibiting protein toxins. Curr. Top. Microbiol. Immunol. 291, 113–145. doi:10.1007/3-540-27511-8_7
Aktories, K., Schwan, C., and Jank, T. (2017). Clostridium difficile toxin biology. Annu. Rev. Microbiol. 71, 281–307. doi:10.1146/annurev-micro-090816-093458
Alvarez-de Miranda, F. J., Alonso-Sánchez, I., Alcamí, A., and Hernaez, B. (2021). TNF decoy receptors encoded by poxviruses. Pathogens 10, 1065. doi:10.3390/pathogens10081065
Anastasina, M., Le May, N., Bugai, A., Fu, Y., Söderholm, S., Gaelings, L., et al. (2016). Influenza virus NS1 protein binds cellular DNA to block transcription of antiviral genes. Biochim. Biophys. Acta 1859, 1440–1448. doi:10.1016/j.bbagrm.2016.09.005
Andoniou, C. E., Sutton, V. R., Wikstrom, M. E., Fleming, P., Thia, K. Y. T., Matthews, A. Y., et al. (2014). A natural genetic variant of granzyme B confers lethality to a common viral infection. PLoS Pathog. 10, e1004526. doi:10.1371/journal.ppat.1004526
Ashburner, M., Ball, C. A., Blake, J. A., Botstein, D., Butler, H., Cherry, J. M., et al. (2000). Gene ontology: Tool for the unification of biology. Nat. Genet. 25, 25–29. doi:10.1038/75556
Baer, A., Austin, D., Narayanan, A., Popova, T., Kainulainen, M., Bailey, C., et al. (2012). Induction of DNA damage signaling upon Rift Valley fever virus infection results in cell cycle arrest and increased viral replication. J. Biol. Chem. 287, 7399–7410. doi:10.1074/jbc.M111.296608
Balaji, A., Kille, B., Kappell, A. D., Godbold, G. D., Diep, M., Elworth, R. A. L., et al. (2022). SeqScreen: Accurate and sensitive functional screening of pathogenic sequences via ensemble learning. Genome Biol. 23, 133. doi:10.1186/s13059-022-02695-x
Balenga, N. A., Klichinsky, M., Xie, Z., Chan, E. C., Zhao, M., Jude, J., et al. (2015). A fungal protease allergen provokes airway hyper-responsiveness in asthma. Nat. Commun. 6, 6763. doi:10.1038/ncomms7763
Bamia, A., Marcato, V., Boissière, M., Mansuroglu, Z., Tamietti, C., Romani, M., et al. (2020). The NSs protein encoded by the virulent strain of rift valley fever virus targets the expression of Abl2 and the actin cytoskeleton of the host, affecting cell mobility, cell shape, and cell-cell adhesion. J. Virol. 95, e01768–20. doi:10.1128/JVI.01768-20
Barreda, D., Santiago, C., Rodríguez, J. R., Rodríguez, J. F., Casasnovas, J. M., Mérida, I., et al. (2021). SARS-CoV-2 spike protein and its receptor binding domain promote a proinflammatory activation profile on human dendritic cells. Cells 10, 3279. doi:10.3390/cells10123279
Barrett, C. T., Neal, H. E., Edmonds, K., Moncman, C. L., Thompson, R., Branttie, J. M., et al. (2021). Effect of clinical isolate or cleavage site mutations in the SARS-CoV-2 spike protein on protein stability, cleavage, and cell-cell fusion. J. Biol. Chem. 297, 100902. doi:10.1016/j.jbc.2021.100902
Bastaert, F., Kheir, S., Saint-Criq, V., Villeret, B., Dang, P. M.-C., El-Benna, J., et al. (2018). Pseudomonas aeruginosa LasB subverts alveolar macrophage activity by interfering with bacterial killing through downregulation of innate immune defense, reactive oxygen species generation, and complement activation. Front. Immunol. 9, 1675. doi:10.3389/fimmu.2018.01675
Beaufort, N., Corvazier, E., Hervieu, A., Choqueux, C., Dussiot, M., Louedec, L., et al. (2011). The thermolysin-like metalloproteinase and virulence factor LasB from pathogenic Pseudomonas aeruginosa induces anoikis of human vascular cells. Cell Microbiol. 13, 1149–1167. doi:10.1111/j.1462-5822.2011.01606.x
Behnsen, J., Lessing, F., Schindler, S., Wartenberg, D., Jacobsen, I. D., Thoen, M., et al. (2010). Secreted Aspergillus fumigatus protease Alp1 degrades human complement proteins C3, C4, and C5. Infect. Immun. 78, 3585–3594. doi:10.1128/IAI.01353-09
Bergsbaken, T., Fink, S. L., and Cookson, B. T. (2009). Pyroptosis: Host cell death and inflammation. Nat. Rev. Microbiol. 7, 99–109. doi:10.1038/nrmicro2070
Berhane, S., Aresté, C., Ablack, J. N., Ryan, G. B., Blackbourn, D. J., Mymryk, J. S., et al. (2011). Adenovirus E1A interacts directly with, and regulates the level of expression of, the immunoproteasome component MECL1. Virology 421, 149–158. doi:10.1016/j.virol.2011.09.025
Bishop, B. L., Lodolce, J. P., Kolodziej, L. E., Boone, D. L., and Tang, W. J. (2010). The role of anthrolysin O in gut epithelial barrier disruption during Bacillus anthracis infection. Biochem. Biophys. Res. Commun. 394, 254–259. doi:10.1016/j.bbrc.2010.02.091
Biswal, M., Lu, J., and Song, J. (2022). SARS-CoV-2 nucleocapsid protein targets a conserved surface groove of the NTF2-like domain of G3BP1. J. Mol. Biol. 434, 167516. doi:10.1016/j.jmb.2022.167516
Blocker, A., Gounon, P., Larquet, E., Niebuhr, K., Cabiaux, V., Parsot, C., et al. (1999). The tripartite type III secreton of Shigella flexneri inserts IpaB and IpaC into host membranes. J. Cell Biol. 147, 683–693. doi:10.1083/jcb.147.3.683
Bonjardim, C. A. (2005). Interferons (IFNs) are key cytokines in both innate and adaptive antiviral immune responses-and viruses counteract IFN action. Microbes Infect. 7, 569–578. doi:10.1016/j.micinf.2005.02.001
Braga, L., Ali, H., Secco, I., Chiavacci, E., Neves, G., Goldhill, D., et al. (2021). Drugs that inhibit TMEM16 proteins block SARS-CoV-2 spike-induced syncytia. Nature 594, 88–93. doi:10.1038/s41586-021-03491-6
Brandherm, L., Kobaš, A. M., Klöhn, M., Brüggemann, Y., Pfaender, S., Rassow, J., et al. (2021). Phosphorylation of SARS-CoV-2 Orf9b regulates its targeting to two binding sites in TOM70 and recruitment of Hsp90. Int. J. Mol. Sci. 22, 9233. doi:10.3390/ijms22179233
Bratke, K. A., McLysaght, A., and Rothenburg, S. (2013). A survey of host range genes in poxvirus genomes. Infect. Genet. Evol. 14, 406–425. doi:10.1016/j.meegid.2012.12.002
Brosey, C. A., Houl, J. H., Katsonis, P., Balapiti-Modarage, L. P. F., Bommagani, S., Arvai, A., et al. (2021). Targeting SARS-CoV-2 Nsp3 macrodomain structure with insights from human poly(ADP-ribose) glycohydrolase (PARG) structures with inhibitors. Prog. Biophys. Mol. Biol. 163, 171–186. doi:10.1016/j.pbiomolbio.2021.02.002
Buchrieser, J., Dufloo, J., Hubert, M., Monel, B., Planas, D., Rajah, M. M., et al. (2020). Syncytia formation by SARS-CoV-2-infected cells. EMBO J. 39, e106267. doi:10.15252/embj.2020106267
Cantuti-Castelvetri, L., Ojha, R., Pedro, L. D., Djannatian, M., Franz, J., Kuivanen, S., et al. (2020). Neuropilin-1 facilitates SARS-CoV-2 cell entry and infectivity. Science 370, 856–860. doi:10.1126/science.abd2985
Cao, X., Tian, Y., Nguyen, V., Zhang, Y., Gao, C., Yin, R., et al. (2020). Spike protein of SARS-CoV-2 activates macrophages and contributes to induction of acute lung inflammations in mice. bioRxiv 2020, 414706. doi:10.1101/2020.12.07.414706
Casadevall, A., and Pirofski, L. (2003). The damage-response framework of microbial pathogenesis. Nat. Rev. Microbiol. 1, 17–24. doi:10.1038/nrmicro732
Casanova, J.-L. (2015a). Human genetic basis of interindividual variability in the course of infection. Proc. Natl. Acad. Sci. U.S.A. 112, E7118–E7127. doi:10.1073/pnas.1521644112
Casanova, J.-L. (2015b). Severe infectious diseases of childhood as monogenic inborn errors of immunity. Proc. Natl. Acad. Sci. U.S.A. 112, E7128–E7137. doi:10.1073/pnas.1521651112
Chaimayo, C., Dunagan, M., Hayashi, T., Santoso, N., and Takimoto, T. (2018). Specificity and functional interplay between influenza virus PA-X and NS1 shutoff activity. PLoS Pathog. 14, e1007465. doi:10.1371/journal.ppat.1007465
Chen, D., Zheng, Q., Sun, L., Ji, M., Li, Y., Deng, H., et al. (2021). ORF3a of SARS-CoV-2 promotes lysosomal exocytosis-mediated viral egress. Dev. Cell 56, 3250–3263.e5. doi:10.1016/j.devcel.2021.10.006
Chien, C., Xu, Y., Xiao, R., Aramini, J. M., Sahasrabudhe, P. V., Krug, R. M., et al. (2004). Biophysical characterization of the complex between double-stranded RNA and the N-terminal domain of the NS1 protein from influenza A virus: Evidence for a novel RNA-binding mode. Biochemistry 43, 1950–1962. doi:10.1021/bi030176o
Chui, A. J., Okondo, M. C., Rao, S. D., Gai, K., Griswold, A. R., Johnson, D. C., et al. (2019). N-terminal degradation activates the NLRP1B inflammasome. Science 364, 82–85. doi:10.1126/science.aau1208
Corbeil, L. B. (2016). Histophilus somni surface proteins. Curr. Top. Microbiol. Immunol. 396, 89–107. doi:10.1007/82_2015_5011
Correy, G. J., Kneller, D. W., Phillips, G., Pant, S., Russi, S., Cohen, A. E., et al. (2022). The mechanisms of catalysis and ligand binding for the SARS-CoV-2 NSP3 macrodomain from neutron and X-ray diffraction at room temperature. bioRxiv 2022, 479477. doi:10.1101/2022.02.07.479477
Cowardin, C. A., Jackman, B. M., Noor, Z., Burgess, S. L., Feig, A. L., and Petri, W. A. (2016). Glucosylation drives the innate inflammatory response to Clostridium difficile toxin A. Infect. Immun. 84, 2317–2323. doi:10.1128/IAI.00327-16
Cybulski, R. J., Sanz, P., Alem, F., Stibitz, S., Bull, R. L., and O’Brien, A. D. (2009). Four superoxide dismutases contribute to Bacillus anthracis virulence and provide spores with redundant protection from oxidative stress. Infect. Immun. 77, 274–285. doi:10.1128/IAI.00515-08
Daly, J. L., Simonetti, B., Klein, K., Chen, K.-E., Williamson, M. K., Antón-Plágaro, C., et al. (2020). Neuropilin-1 is a host factor for SARS-CoV-2 infection. Science 370, 861–865. doi:10.1126/science.abd3072
Department of Health and Human Services, (2017). Framework for guiding funding decisions about proposed research involving enhanced potential pandemic pathogens. Available at: https://www.phe.gov/s3/dualuse/Documents/P3CO.pdf (Accessed June 7, 2022).
Díaz, J. (2020). SARS-CoV-2 molecular network structure. Front. Physiol. 11, 870. doi:10.3389/fphys.2020.00870
Du, J., An, R., Chen, L., Shen, Y., Chen, Y., Cheng, L., et al. (2014). Toxoplasma gondii virulence factor ROP18 inhibits the host NF-κB pathway by promoting p65 degradation. J. Biol. Chem. 289, 12578–12592. doi:10.1074/jbc.M113.544718
Dunlop, L. R., Oehlberg, K. A., Reid, J. J., Avci, D., and Rosengard, A. M. (2003). Variola virus immune evasion proteins. Microbes Infect. 5, 1049–1056. doi:10.1016/s1286-4579(03)00194-1
Ebrahimi, C. M., Kern, J. W., Sheen, T. R., Ebrahimi-Fardooee, M. A., van Sorge, N. M., Schneewind, O., et al. (2009). Penetration of the blood-brain barrier by Bacillus anthracis requires the pXO1-encoded BslA protein. J. Bacteriol. 191, 7165–7173. doi:10.1128/JB.00903-09
Feng, K., Min, Y.-Q., Sun, X., Deng, F., Li, P., Wang, H., et al. (2021). Interactome profiling reveals interaction of SARS-CoV-2 NSP13 with host factor STAT1 to suppress interferon signaling. J. Mol. Cell Biol. 13, 760–762. doi:10.1093/jmcb/mjab068
Fentress, S. J., Behnke, M. S., Dunay, I. R., Mashayekhi, M., Rommereim, L. M., Fox, B. A., et al. (2010). Phosphorylation of immunity-related GTPases by a Toxoplasma gondii-secreted kinase promotes macrophage survival and virulence. Cell Host Microbe 8, 484–495. doi:10.1016/j.chom.2010.11.005
Fernandez-Sesma, A., Marukian, S., Ebersole, B. J., Kaminski, D., Park, M.-S., Yuen, T., et al. (2006). Influenza virus evades innate and adaptive immunity via the NS1 protein. J. Virol. 80, 6295–6304. doi:10.1128/JVI.02381-05
Finkel, Y., Gluck, A., Nachshon, A., Winkler, R., Fisher, T., Rozman, B., et al. (2021). SARS-CoV-2 uses a multipronged strategy to impede host protein synthesis. Nature 594, 240–245. doi:10.1038/s41586-021-03610-3
Firoved, A. M., Miller, G. F., Moayeri, M., Kakkar, R., Shen, Y., Wiggins, J. F., et al. (2005). Bacillus anthracis edema toxin causes extensive tissue lesions and rapid lethality in mice. Am. J. Pathol. 167, 1309–1320. doi:10.1016/S0002-9440(10)61218-7
Fislová, T., and Kostolanský, F. (2005). The factors of virulence of influenza a virus. Acta Virol. 49, 147–157.
Flower, T. G., Buffalo, C. Z., Hooy, R. M., Allaire, M., Ren, X., and Hurley, J. H. (2020). Structure of SARS-CoV-2 ORF8, a rapidly evolving coronavirus protein implicated in immune evasion. bioRxiv 2020, 270637. doi:10.1101/2020.08.27.270637
Fonseca, G. J., Thillainadesan, G., Yousef, A. F., Ablack, J. N., Mossman, K. L., Torchia, J., et al. (2012). Adenovirus evasion of interferon-mediated innate immunity by direct antagonism of a cellular histone posttranslational modification. Cell Host Microbe 11, 597–606. doi:10.1016/j.chom.2012.05.005
Friebe, S., van der Goot, F. G., and Bürgi, J. (2016). The ins and outs of anthrax toxin. Toxins (Basel) 8, E69. doi:10.3390/toxins8030069
Fu, Y.-Z., Wang, S.-Y., Zheng, Z.-Q., Huang, Yi, Li, W.-W., Xu, Z.-S., et al. (2021). SARS-CoV-2 membrane glycoprotein M antagonizes the MAVS-mediated innate antiviral response. Cell Mol. Immunol. 18, 613–620. doi:10.1038/s41423-020-00571-x
Gack, M. U., Albrecht, R. A., Urano, T., Inn, K.-S., Huang, I.-C., Carnero, E., et al. (2009). Influenza A virus NS1 targets the ubiquitin ligase TRIM25 to evade recognition by the host viral RNA sensor RIG-I. Cell Host Microbe 5, 439–449. doi:10.1016/j.chom.2009.04.006
Gemler, B. T., Mukherjee, C., Howland, C. A., Huk, D., Shank, Z., Harbo, L. J., et al. (2022). Function-based classification of hazardous biological sequences: Demonstration of a new paradigm for biohazard assessments. Front. Bioeng. Biotechnol. 10, 979497. doi:10.3389/fbioe.2022.979497
Gene Ontology Consortium Carbon, S., Douglass, E., Good, B. M., Unni, D. R., Harris, N. L., Mungall, C. J., et al. (2021). The gene ontology resource: Enriching a GOld mine. Nucleic Acids Res. 49, D325–D334. doi:10.1093/nar/gkaa1113
Glenewinkel, F., Cohen, M. J., King, C. R., Kaspar, S., Bamberg-Lemper, S., Mymryk, J. S., et al. (2016). The adaptor protein DCAF7 mediates the interaction of the adenovirus E1A oncoprotein with the protein kinases DYRK1A and HIPK2. Sci. Rep. 6, 28241. doi:10.1038/srep28241
Godbold, G. D., Kappell, A. D., LeSassier, D. S., Treangen, T. J., and Ternus, K. L. (2022). Categorizing sequences of concern by function to better assess mechanisms of microbial pathogenesis. Infect. Immun. 90, e0033421. doi:10.1128/IAI.00334-21
Goldberg, A. B., Cho, E., Miller, C. J., Lou, H. J., and Turk, B. E. (2017). Identification of a substrate-selective exosite within the metalloproteinase anthrax lethal factor. J. Biol. Chem. 292, 814–825. doi:10.1074/jbc.M116.761734
Golovkine, G., Faudry, E., Bouillot, S., Voulhoux, R., Attrée, I., and Huber, P. (2014). VE-cadherin cleavage by LasB protease from Pseudomonas aeruginosa facilitates type III secretion system toxicity in endothelial cells. PLoS Pathog. 10, e1003939. doi:10.1371/journal.ppat.1003939
Gordon, D. E., Jang, G. M., Bouhaddou, M., Xu, J., Obernier, K., White, K. M., et al. (2020). A SARS-CoV-2 protein interaction map reveals targets for drug repurposing. Nature 583, 459–468. doi:10.1038/s41586-020-2286-9
Government Accountability Office (GAO) (2023). Public Health preparedness: HHS could improve oversight of research involving enhanced potential pandemic pathogens. Available at: https://www.gao.gov/assets/gao-23-105455.pdf (Accessed February 1, 2023).
Guichard, A., McGillivray, S. M., Cruz-Moreno, B., van Sorge, N. M., Nizet, V., and Bier, E. (2010). Anthrax toxins cooperatively inhibit endocytic recycling by the Rab11/Sec15 exocyst. Nature 467, 854–858. doi:10.1038/nature09446
Guo, G., Gao, M., Gao, X., Zhu, B., Huang, J., Luo, K., et al. (2021). SARS-CoV-2 non-structural protein 13 (nsp13) hijacks host deubiquitinase USP13 and counteracts host antiviral immune response. Signal Transduct. Target Ther. 6, 119. doi:10.1038/s41392-021-00509-3
Han, L., Zhuang, M.-W., Deng, J., Zheng, Y., Zhang, J., Nan, M.-L., et al. (2021). SARS-CoV-2 ORF9b antagonizes type I and III interferons by targeting multiple components of the RIG-I/MDA-5-MAVS, TLR3-TRIF, and cGAS-STING signaling pathways. J. Med. Virol. 93, 5376–5389. doi:10.1002/jmv.27050
Hathaway, L. J., Griffin, G. E., Sansonetti, P. J., and Edgeworth, J. D. (2002). Human monocytes kill Shigella flexneri but then die by apoptosis associated with suppression of proinflammatory cytokine production. Infect. Immun. 70, 3833–3842. doi:10.1128/IAI.70.7.3833-3842.2002
Head, J. A., Kalveram, B., and Ikegami, T. (2012). Functional analysis of Rift Valley fever virus NSs encoding a partial truncation. PLoS One 7, e45730. doi:10.1371/journal.pone.0045730
Heffernan, B. J., Thomason, B., Herring-Palmer, A., and Hanna, P. (2007). Bacillus anthracis anthrolysin O and three phospholipases C are functionally redundant in a murine model of inhalation anthrax. FEMS Microbiol. Lett. 271, 98–105. doi:10.1111/j.1574-6968.2007.00713.x
Hilbi, H., Moss, J. E., Hersh, D., Chen, Y., Arondel, J., Banerjee, S., et al. (1998). Shigella-induced apoptosis is dependent on caspase-1 which binds to IpaB. J. Biol. Chem. 273, 32895–32900. doi:10.1074/jbc.273.49.32895
Hsu, J. C.-C., Laurent-Rolle, M., Pawlak, J. B., Wilen, C. B., and Cresswell, P. (2021). Translational shutdown and evasion of the innate immune response by SARS-CoV-2 NSP14 protein. Proc. Natl. Acad. Sci. U. S. A. 118, e2101161118. doi:10.1073/pnas.2101161118
Huang, C., Lokugamage, K. G., Rozovics, J. M., Narayanan, K., Semler, B. L., and Makino, S. (2011). SARS coronavirus nsp1 protein induces template-dependent endonucleolytic cleavage of mRNAs: Viral mRNAs are resistant to nsp1-induced RNA cleavage. PLoS Pathog. 7, e1002433. doi:10.1371/journal.ppat.1002433
Hutt, J. A., Lovchik, J. A., Drysdale, M., Sherwood, R. L., Brasel, T., Lipscomb, M. F., et al. (2014). Lethal factor, but not edema factor, is required to cause fatal anthrax in cynomolgus macaques after pulmonary spore challenge. Am. J. Pathol. 184, 3205–3216. doi:10.1016/j.ajpath.2014.08.008
Ijiri, Y., Matsumoto, K., Kamata, R., Nishino, N., Okamura, R., Kambara, T., et al. (1994). Suppression of polymorphonuclear leucocyte chemotaxis by Pseudomonas aeruginosa elastase in vitro: A study of the mechanisms and the correlation with ring abscess in pseudomonal keratitis. Int. J. Exp. Pathol. 75, 441–451.
Iwai, H., Kim, M., Yoshikawa, Y., Ashida, H., Ogawa, M., Fujita, Y., et al. (2007). A bacterial effector targets Mad2L2, an APC inhibitor, to modulate host cell cycling. Cell 130, 611–623. doi:10.1016/j.cell.2007.06.043
Jackson, R. J., Ramsay, A. J., Christensen, C. D., Beaton, S., Hall, D. F., and Ramshaw, I. A. (2001). Expression of mouse interleukin-4 by a recombinant ectromelia virus suppresses cytolytic lymphocyte responses and overcomes genetic resistance to mousepox. J. Virol. 75, 1205–1210. doi:10.1128/JVI.75.3.1205-1210.2001
Jiang, H.-W., Zhang, H.-N., Meng, Q.-F., Xie, J., Li, Y., Chen, H., et al. (2020). SARS-CoV-2 Orf9b suppresses type I interferon responses by targeting TOM70. Cell Mol. Immunol. 17, 998–1000. doi:10.1038/s41423-020-0514-8
Jiao, J., Guan, H., Lippa, A. M., and Ricciardi, R. P. (2010). The N terminus of adenovirus type 12 E1A inhibits major histocompatibility complex class I expression by preventing phosphorylation of NF-κB p65 ser 276 through direct binding. J. Virol. 84, 7668–7674. doi:10.1128/JVI.02317-09
Jungreis, I., Sealfon, R., and Kellis, M. (2021). SARS-CoV-2 gene content and COVID-19 mutation impact by comparing 44 Sarbecovirus genomes. Nat. Commun. 12, 2642. doi:10.1038/s41467-021-22905-7
Kainulainen, M., Habjan, M., Hubel, P., Busch, L., Lau, S., Colinge, J., et al. (2014). Virulence factor NSs of rift valley fever virus recruits the F-box protein FBXO3 to degrade subunit p62 of general transcription factor TFIIH. J. Virol. 88, 3464–3473. doi:10.1128/JVI.02914-13
Kainulainen, M., Lau, S., Samuel, C. E., Hornung, V., and Weber, F. (2016). NSs virulence factor of rift valley fever virus engages the F-box proteins FBXW11 and β-TRCP1 to degrade the antiviral protein kinase PKR. J. Virol. 90, 6140–6147. doi:10.1128/JVI.00016-16
Kern, J., and Schneewind, O. (2010). BslA, the S-layer adhesin of B. anthracis, is a virulence factor for anthrax pathogenesis. Mol. Microbiol. 75, 324–332. doi:10.1111/j.1365-2958.2009.06958.x
Khan, S., Shafiei, M. S., Longoria, C., Schoggins, J., Savani, R. C., and Zaki, H. (2021). SARS-CoV-2 spike protein induces inflammation via TLR2-dependent activation of the NF-κB pathway. bioRxiv, 10, e68563. doi:10.7554/eLife.68563
King, C. R., Zhang, A., Tessier, T. M., Gameiro, S. F., and Mymryk, J. S. (2018). Hacking the cell: Network intrusion and exploitation by adenovirus E1A. mBio 9, e00390–18. doi:10.1128/mBio.00390-18
Klemm, T., Ebert, G., Calleja, D. J., Allison, C. C., Richardson, L. W., Bernardini, J. P., et al. (2020). Mechanism and inhibition of the papain-like protease, PLpro, of SARS-CoV-2. EMBO J. 39, e106275. doi:10.15252/embj.2020106275
Kreimendahl, S., and Rassow, J. (2020). The mitochondrial outer membrane protein tom70-mediator in protein traffic, membrane contact sites and innate immunity. Int. J. Mol. Sci. 21, E7262. doi:10.3390/ijms21197262
Kumar, N. A., Kunnakkadan, U., Thomas, S., and Johnson, J. B. (2020). In the crosshairs: RNA viruses OR complement? Front. Immunol. 11, 573583. doi:10.3389/fimmu.2020.573583
Kurupati, P., Turner, C. E., Tziona, I., Lawrenson, R. A., Alam, F. M., Nohadani, M., et al. (2010). Chemokine-cleaving Streptococcus pyogenes protease SpyCEP is necessary and sufficient for bacterial dissemination within soft tissues and the respiratory tract. Mol. Microbiol. 76, 1387–1397. doi:10.1111/j.1365-2958.2010.07065.x
Lafont, F., Tran Van Nhieu, G., Hanada, K., Sansonetti, P., and van der Goot, F. G. (2002). Initial steps of Shigella infection depend on the cholesterol/sphingolipid raft-mediated CD44-IpaB interaction. EMBO J. 21, 4449–4457. doi:10.1093/emboj/cdf457
Langer, M., Duggan, E. S., Booth, J. L., Patel, V. I., Zander, R. A., Silasi-Mansat, R., et al. (2012). Bacillus anthracis lethal toxin reduces human alveolar epithelial barrier function. Infect. Immun. 80, 4374–4387. doi:10.1128/IAI.01011-12
Lau, L., Gray, E. E., Brunette, R. L., and Stetson, D. B. (2015). DNA tumor virus oncogenes antagonize the cGAS-STING DNA-sensing pathway. Science 350, 568–571. doi:10.1126/science.aab3291
Le May, N., Mansuroglu, Z., Léger, P., Josse, T., Blot, G., Billecocq, A., et al. (2008). A SAP30 complex inhibits IFN-beta expression in Rift Valley fever virus infected cells. PLoS Pathog. 4, e13. doi:10.1371/journal.ppat.0040013
Leduc, D., Beaufort, N., de Bentzmann, S., Rousselle, J.-C., Namane, A., Chignard, M., et al. (2007). The Pseudomonas aeruginosa LasB metalloproteinase regulates the human urokinase-type plasminogen activator receptor through domain-specific endoproteolysis. Infect. Immun. 75, 3848–3858. doi:10.1128/IAI.00015-07
Lei, X., Dong, X., Ma, R., Wang, W., Xiao, X., Tian, Z., et al. (2020). Activation and evasion of type I interferon responses by SARS-CoV-2. Nat. Commun. 11, 3810. doi:10.1038/s41467-020-17665-9
Li, J.-Y., Liao, C.-H., Wang, Q., Tan, Y.-J., Luo, R., Qiu, Y., et al. (2020). The ORF6, ORF8 and nucleocapsid proteins of SARS-CoV-2 inhibit type I interferon signaling pathway. Virus Res. 286, 198074. doi:10.1016/j.virusres.2020.198074
Li, J., Vinh, D. C., Casanova, J.-L., and Puel, A. (2017). Inborn errors of immunity underlying fungal diseases in otherwise healthy individuals. Curr. Opin. Microbiol. 40, 46–57. doi:10.1016/j.mib.2017.10.016
Lin, X., Fu, B., Yin, S., Li, Z., Liu, H., Zhang, H., et al. (2021). ORF8 contributes to cytokine storm during SARS-CoV-2 infection by activating IL-17 pathway. iScience 24, 102293. doi:10.1016/j.isci.2021.102293
Liu, S., Zhang, Y., Moayeri, M., Liu, J., Crown, D., Fattah, R. J., et al. (2013). Key tissue targets responsible for anthrax-toxin-induced lethality. Nature 501, 63–68. doi:10.1038/nature12510
Liu, Y., Qin, C., Rao, Y., Ngo, C., Feng, J. J., Zhao, J., et al. (2021). SARS-CoV-2 Nsp5 demonstrates two distinct mechanisms targeting RIG-I and MAVS to evade the innate immune response. mBio 12, e0233521. doi:10.1128/mBio.02335-21
Lu, S., Ye, Q., Singh, D., Cao, Y., Diedrich, J. K., Yates, J. R., et al. (2021). The SARS-CoV-2 nucleocapsid phosphoprotein forms mutually exclusive condensates with RNA and the membrane-associated M protein. Nat. Commun. 12, 502. doi:10.1038/s41467-020-20768-y
Magro, C., Mulvey, J. J., Berlin, D., Nuovo, G., Salvatore, S., Harp, J., et al. (2020). Complement associated microvascular injury and thrombosis in the pathogenesis of severe COVID-19 infection: A report of five cases. Transl. Res. 220, 1–13. doi:10.1016/j.trsl.2020.04.007
Matheson, N. R., Potempa, J., and Travis, J. (2006). Interaction of a novel form of Pseudomonas aeruginosa alkaline protease (aeruginolysin) with interleukin-6 and interleukin-8. Biol. Chem. 387, 911–915. doi:10.1515/BC.2006.115
Matsuoka, K., Imahashi, N., Ohno, M., Ode, H., Nakata, Y., Kubota, M., et al. (2022). SARS-CoV-2 accessory protein ORF8 is secreted extracellularly as a glycoprotein homodimer. J. Biol. Chem. 298, 101724. doi:10.1016/j.jbc.2022.101724
McGillivray, S. M., Ebrahimi, C. M., Fisher, N., Sabet, M., Zhang, D. X., Chen, Y., et al. (2009). ClpX contributes to innate defense peptide resistance and virulence phenotypes of <i>Bacillus anthracis</i>. J. Innate Immun. 1, 494–506. doi:10.1159/000225955
Miao, G., Zhao, H., Li, Y., Ji, M., Chen, Y., Shi, Y., et al. (2021). ORF3a of the COVID-19 virus SARS-CoV-2 blocks HOPS complex-mediated assembly of the SNARE complex required for autolysosome formation. Dev. Cell 56, 427–442.e5. doi:10.1016/j.devcel.2020.12.010
Miller, C. S. (2005). Pleiotropic mechanisms of virus survival and persistence. Oral Surg. Oral Med. Oral Pathol. Oral Radiol. Endod. 100, S27–S36. doi:10.1016/j.tripleo.2005.03.017
Mishra, D., Suri, G. S., Kaur, G., and Tiwari, M. (2021). Comparative insight into the genomic landscape of SARS-CoV-2 and identification of mutations associated with the origin of infection and diversity. J. Med. Virol. 93, 2406–2419. doi:10.1002/jmv.26744
Miyamoto, Y., Itoh, Y., Suzuki, T., Tanaka, T., Sakai, Y., Koido, M., et al. (2022). SARS-CoV-2 ORF6 disrupts nucleocytoplasmic trafficking to advance viral replication. Commun. Biol. 5, 483. doi:10.1038/s42003-022-03427-4
Mosser, E. M., and Rest, R. F. (2006). The Bacillus anthracis cholesterol-dependent cytolysin, Anthrolysin O, kills human neutrophils, monocytes and macrophages. BMC Microbiol. 6, 56. doi:10.1186/1471-2180-6-56
Mounier, J., Boncompain, G., Senerovic, L., Lagache, T., Chrétien, F., Perez, F., et al. (2012). Shigella effector IpaB-induced cholesterol relocation disrupts the Golgi complex and recycling network to inhibit host cell secretion. Cell Host Microbe 12, 381–389. doi:10.1016/j.chom.2012.07.010
Mounier, J., Popoff, M. R., Enninga, J., Frame, M. C., Sansonetti, P. J., and Van Nhieu, G. T. (2009). The IpaC carboxyterminal effector domain mediates Src-dependent actin polymerization during Shigella invasion of epithelial cells. PLoS Pathog. 5, e1000271. doi:10.1371/journal.ppat.1000271
Moustaqil, M., Ollivier, E., Chiu, H.-P., Van Tol, S., Rudolffi-Soto, P., Stevens, C., et al. (2021). SARS-CoV-2 proteases PLpro and 3CLpro cleave IRF3 and critical modulators of inflammatory pathways (NLRP12 and TAB1): Implications for disease presentation across species. Emerg. Microbes Infect. 10, 178–195. doi:10.1080/22221751.2020.1870414
Mu, J., Fang, Y., Yang, Q., Shu, T., Wang, A., Huang, M., et al. (2020). SARS-CoV-2 N protein antagonizes type I interferon signaling by suppressing phosphorylation and nuclear translocation of STAT1 and STAT2. Cell Discov. 6, 65. doi:10.1038/s41421-020-00208-3
Mukherjee, D. V., Tonry, J. H., Kim, K. S., Ramarao, N., Popova, T. G., Bailey, C., et al. (2011). Bacillus anthracis protease InhA increases blood-brain barrier permeability and contributes to cerebral hemorrhages. PLoS ONE 6, e17921. doi:10.1371/journal.pone.0017921
Müllbacher, A., and Lobigs, M. (2001). Creation of killer poxvirus could have been predicted. J. Virol. 75, 8353–8355. doi:10.1128/jvi.75.18.8353-8355.2001
National Academies of Science, Engineering, and Medicine (2018). Biodefense in the age of synthetic biology. Available at: https://nap.nationalacademies.org/catalog/24890/biodefense-in-the-age-of-synthetic-biology#:∼:text=Making%20informed%20decisions%20about%20how,capabilities%20that%20could%20be%20misused.&text=Biodefense%20in%20the%20Age%20of%20Synthetic%20Biology%20explores,potential%20misuses%20of%20synthetic%20biology (Accessed June 7, 2022).
National Research Council (2010). Sequence-based classification of select agents. Washington, DC: A Brighter Line. doi:10.17226/12970
National Science Advisory Board for Biosecurity (2023). Proposed biosecurity oversight framework for the future of science. Available at: https://osp.od.nih.gov/wp-content/uploads/2023/01/DRAFT-NSABB-WG-Report.pdf (Accessed February 1, 2023).
Ng, J., Hirota, S. A., Gross, O., Li, Y., Ulke-Lemee, A., Potentier, M. S., et al. (2010). Clostridium difficile toxin-induced inflammation and intestinal injury are mediated by the inflammasome. Gastroenterology 139, 542–552. doi:10.1053/j.gastro.2010.04.005
Oh, S. J., and Shin, O. S. (2021). SARS-CoV-2 nucleocapsid protein targets RIG-I-like receptor pathways to inhibit the induction of interferon response. Cells 10, 530. doi:10.3390/cells10030530
Pan, Y., Tagawa, Y., Champion, A., Sandal, I., and Inzana, T. J. (2018). Histophilus somni survives in bovine macrophages by interfering with phagosome-lysosome fusion but requires IbpA for optimal serum resistance. Infect. Immun. 86, e00365–18. doi:10.1128/IAI.00365-18
Papatheodorou, P., Zamboglou, C., Genisyuerek, S., Guttenberg, G., and Aktories, K. (2010). Clostridial glucosylating toxins enter cells via clathrin-mediated endocytosis. PLoS One 5, e10673. doi:10.1371/journal.pone.0010673
Park, M. D. (2020). Immune evasion via SARS-CoV-2 ORF8 protein? Nat. Rev. Immunol. 20, 408. doi:10.1038/s41577-020-0360-z
Pirofski, L., and Casadevall, A. (2015). What is infectiveness and how is it involved in infection and immunity? BMC Immunol. 16, 13. doi:10.1186/s12865-015-0076-1
Pisano, F., Kochut, A., Uliczka, F., Geyer, R., Stolz, T., Thiermann, T., et al. (2012). In vivo-induced InvA-like autotransporters Ifp and InvC of Yersinia pseudotuberculosis promote interactions with intestinal epithelial cells and contribute to virulence. Infect. Immun. 80, 1050–1064. doi:10.1128/IAI.05715-11
Prelli Bozzo, C., Nchioua, R., Volcic, M., Koepke, L., Krüger, J., Schütz, D., et al. (2021). IFITM proteins promote SARS-CoV-2 infection and are targets for virus inhibition in vitro. Nat. Commun. 12, 4584. doi:10.1038/s41467-021-24817-y
Rack, J. G. M., Zorzini, V., Zhu, Z., Schuller, M., Ahel, D., and Ahel, I. (2020). Viral macrodomains: A structural and evolutionary assessment of the pharmacological potential. Open Biol. 10, 200237. doi:10.1098/rsob.200237
Rahman, M. M., and McFadden, G. (2017). Myxoma virus dsRNA binding protein M029 inhibits the type I IFN-induced antiviral state in a highly species-specific fashion. Viruses 9, E27. doi:10.3390/v9020027
Rahman, M. M., and McFadden, G. (2020). Myxoma virus-encoded host range protein M029: A multifunctional antagonist targeting multiple host antiviral and innate immune pathways. Vaccines (Basel) 8, E244. doi:10.3390/vaccines8020244
Ren, Y., Shu, T., Wu, D., Mu, J., Wang, C., Huang, M., et al. (2020). The ORF3a protein of SARS-CoV-2 induces apoptosis in cells. Cell Mol. Immunol. 17, 881–883. doi:10.1038/s41423-020-0485-9
Rocheleau, L., Laroche, G., Fu, K., Stewart, C. M., Mohamud, A. O., Côté, M., et al. (2021). Identification of a high-frequency intrahost SARS-CoV-2 spike variant with enhanced cytopathic and fusogenic effects. mBio 12, e0078821. doi:10.1128/mBio.00788-21
Roehrich, A. D., Martinez-Argudo, I., Johnson, S., Blocker, A. J., and Veenendaal, A. K. J. (2010). The extreme C terminus of Shigella flexneri IpaB is required for regulation of type III secretion, needle tip composition, and binding. Infect. Immun. 78, 1682–1691. doi:10.1128/IAI.00645-09
Russo, L. C., Tomasin, R., Matos, I. A., Manucci, A. C., Sowa, S. T., Dale, K., et al. (2021). The SARS-CoV-2 Nsp3 macrodomain reverses PARP9/DTX3L-dependent ADP-ribosylation induced by interferon signaling. J. Biol. Chem. 297, 101041. doi:10.1016/j.jbc.2021.101041
Saint-Criq, V., Villeret, B., Bastaert, F., Kheir, S., Hatton, A., Cazes, A., et al. (2018). Pseudomonas aeruginosa LasB protease impairs innate immunity in mice and humans by targeting a lung epithelial cystic fibrosis transmembrane regulator-IL-6-antimicrobial-repair pathway. Thorax 73, 49–61. doi:10.1136/thoraxjnl-2017-210298
Saputri, D. S., Li, S., van Eerden, F. J., Rozewicki, J., Xu, Z., Ismanto, H. S., et al. (2020). Flexible, functional, and familiar: Characteristics of SARS-CoV-2 spike protein evolution. Front. Microbiol. 11, 2112. doi:10.3389/fmicb.2020.02112
Schmidgen, T., Kaiser, P. O., Ballhorn, W., Franz, B., Göttig, S., Linke, D., et al. (2014). Heterologous expression of Bartonella adhesin A in Escherichia coli by exchange of trimeric autotransporter adhesin domains results in enhanced adhesion properties and a pathogenic phenotype. J. Bacteriol. 196, 2155–2165. doi:10.1128/JB.01461-13
Schroeder, G. N., and Hilbi, H. (2008). Molecular pathogenesis of Shigella spp.: Controlling host cell signaling, invasion, and death by type III secretion. Clin. Microbiol. Rev. 21, 134–156. doi:10.1128/CMR.00032-07
Schubert, A., Zakikhany, K., Pietrocola, G., Meinke, A., Speziale, P., Eikmanns, B. J., et al. (2004). The fibrinogen receptor FbsA promotes adherence of Streptococcus agalactiae to human epithelial cells. Infect. Immun. 72, 6197–6205. doi:10.1128/IAI.72.11.6197-6205.2004
Schubert, K., Karousis, E. D., Jomaa, A., Scaiola, A., Echeverria, B., Gurzeler, L.-A., et al. (2020). SARS-CoV-2 Nsp1 binds the ribosomal mRNA channel to inhibit translation. Nat. Struct. Mol. Biol. 27, 959–966. doi:10.1038/s41594-020-0511-8
Schuller, M., Correy, G. J., Gahbauer, S., Fearon, D., Wu, T., Díaz, R. E., et al. (2021). Fragment binding to the Nsp3 macrodomain of SARS-CoV-2 identified through crystallographic screening and computational docking. Sci. Adv. 7, eabf8711. doi:10.1126/sciadv.abf8711
Seet, B. T., Johnston, J. B., Brunetti, C. R., Barrett, J. W., Everett, H., Cameron, C., et al. (2003). Poxviruses and immune evasion. Annu. Rev. Immunol. 21, 377–423. doi:10.1146/annurev.immunol.21.120601.141049
Senerovic, L., Tsunoda, S. P., Goosmann, C., Brinkmann, V., Zychlinsky, A., Meissner, F., et al. (2012). Spontaneous formation of IpaB ion channels in host cell membranes reveals how Shigella induces pyroptosis in macrophages. Cell Death Dis. 3, e384. doi:10.1038/cddis.2012.124
Shemesh, M., Aktepe, T. E., Deerain, J. M., McAuley, J. L., Audsley, M. D., David, C. T., et al. (2021). SARS-CoV-2 suppresses IFNβ production mediated by NSP1, 5, 6, 15, ORF6 and ORF7b but does not suppress the effects of added interferon. PLoS Pathog. 17, e1009800. doi:10.1371/journal.ppat.1009800
Shi, F., Lv, Q., Wang, T., Xu, J., Xu, W., Shi, Y., et al. (2022). Coronaviruses Nsp5 antagonizes porcine gasdermin D-mediated pyroptosis by cleaving pore-forming p30 fragment. mBio 13, e0273921. doi:10.1128/mbio.02739-21
Shin, D., Mukherjee, R., Grewe, D., Bojkova, D., Baek, K., Bhattacharya, A., et al. (2020). Papain-like protease regulates SARS-CoV-2 viral spread and innate immunity. Nature 587, 657–662. doi:10.1038/s41586-020-2601-5
Shirato, K., and Kizaki, T. (2021). SARS-CoV-2 spike protein S1 subunit induces pro-inflammatory responses via toll-like receptor 4 signaling in murine and human macrophages. Heliyon 7, e06187. doi:10.1016/j.heliyon.2021.e06187
Sousa, S., Lecuit, M., and Cossart, P. (2005). Microbial strategies to target, cross or disrupt epithelia. Curr. Opin. Cell Biol. 17, 489–498. doi:10.1016/j.ceb.2005.08.013
Su, W.-Q., Yu, X.-J., and Zhou, C.-M. (2021). SARS-CoV-2 ORF3a induces incomplete autophagy via the unfolded protein response. Viruses 13, 2467. doi:10.3390/v13122467
Sui, L., Zhao, Y., Wang, W., Wu, P., Wang, Z., Yu, Y., et al. (2021). SARS-CoV-2 membrane protein inhibits type I interferon production through ubiquitin-mediated degradation of TBK1. Front. Immunol. 12, 662989. doi:10.3389/fimmu.2021.662989
Sun, J., LaRock, D. L., Skowronski, E. A., Kimmey, J. M., Olson, J., Jiang, Z., et al. (2020). The Pseudomonas aeruginosa protease LasB directly activates IL-1β. EBioMedicine 60, 102984. doi:10.1016/j.ebiom.2020.102984
Tabak, L. A., and Jorgenson, L. A. (2022). 2022 charge to the NSABB. Available at: https://osp.od.nih.gov/wp-content/uploads/Tabak_and_Jorgenson-2022_Charge_to_the_NSABB.pdf [Accessed May 11, 2022].
Tao, L., Tian, S., Zhang, J., Liu, Z., Robinson-McCarthy, L., Miyashita, S.-I., et al. (2019). Sulfated glycosaminoglycans and low-density lipoprotein receptor contribute to Clostridium difficile toxin A entry into cells. Nat. Microbiol. 4, 1760–1769. doi:10.1038/s41564-019-0464-z
Terasaki, K., Ramirez, S. I., and Makino, S. (2016). Mechanistic insight into the host transcription inhibition function of rift valley fever virus NSs and its importance in virulence. PLoS Negl. Trop. Dis. 10, e0005047. doi:10.1371/journal.pntd.0005047
Thammavongsa, V., Kern, J. W., Missiakas, D. M., and Schneewind, O. (2009). Staphylococcus aureus synthesizes adenosine to escape host immune responses. J. Exp. Med. 206, 2417–2427. doi:10.1084/jem.20090097
Thoms, M., Buschauer, R., Ameismeier, M., Koepke, L., Denk, T., Hirschenberger, M., et al. (2020). Structural basis for translational shutdown and immune evasion by the Nsp1 protein of SARS-CoV-2. Science 369, 1249–1255. doi:10.1126/science.abc8665
Tonry, J. H., McNichol, B. A., Ramarao, N., Chertow, D. S., Kim, K. S., Stibitz, S., et al. (2012). Bacillus anthracis protease InhA regulates BslA-mediated adhesion in human endothelial cells. Cell. Microbiol. 14, 1219–1230. doi:10.1111/j.1462-5822.2012.01791.x
Tournier, J.-N., Quesnel-Hellmann, A., Mathieu, J., Montecucco, C., Tang, W.-J., Mock, M., et al. (2005). Anthrax edema toxin cooperates with lethal toxin to impair cytokine secretion during infection of dendritic cells. J. Immunol. 174, 4934–4941. doi:10.4049/jimmunol.174.8.4934
Uchiyama, T., Kawano, H., and Kusuhara, Y. (2006). The major outer membrane protein rOmpB of spotted fever group rickettsiae functions in the rickettsial adherence to and invasion of Vero cells. Microbes Infect. 8, 801–809. doi:10.1016/j.micinf.2005.10.003
United States Government (2014). United States government policy for institutional oversight of life sciences dual use research of concern. Available at: https://www.phe.gov/s3/dualuse/Documents/durc-policy.pdf [Accessed June 7, 2022].
United States Government (2012). United States government policy for oversight of life sciences dual use research of concern. Available at: https://www.phe.gov/s3/dualuse/Documents/us-policy-durc-032812.pdf [Accessed June 7, 2022].
Vadyvaloo, V., Jarrett, C., Sturdevant, D. E., Sebbane, F., and Hinnebusch, B. J. (2010). Transit through the flea vector induces a pretransmission innate immunity resistance phenotype in Yersinia pestis. PLoS Pathog. 6, e1000783. doi:10.1371/journal.ppat.1000783
van Sorge, N. M., Ebrahimi, C. M., McGillivray, S. M., Quach, D., Sabet, M., Guiney, D. G., et al. (2008). Anthrax toxins inhibit neutrophil signaling pathways in brain endothelium and contribute to the pathogenesis of meningitis. PLoS One 3, e2964. doi:10.1371/journal.pone.0002964
Vazquez, C., Swanson, S. E., Negatu, S. G., Dittmar, M., Miller, J., Ramage, H. R., et al. (2021). SARS-CoV-2 viral proteins NSP1 and NSP13 inhibit interferon activation through distinct mechanisms. PLoS One 16, e0253089. doi:10.1371/journal.pone.0253089
Vuyisich, M., Sanders, C. K., and Graves, S. W. (2012). Binding and cell intoxication studies of anthrax lethal toxin. Mol. Biol. Rep. 39, 5897–5903. doi:10.1007/s11033-011-1401-2
Walls, A. C., Park, Y.-J., Tortorici, M. A., Wall, A., McGuire, A. T., and Veesler, D. (2020). Structure, function, and antigenicity of the SARS-CoV-2 spike glycoprotein. Cell 181, 281–292.e6. doi:10.1016/j.cell.2020.02.058
Wang, R., Yang, X., Chang, M., Xue, Z., Wang, W., Bai, L., et al. (2021a). ORF3a protein of severe acute respiratory syndrome coronavirus 2 inhibits interferon-activated janus kinase/signal transducer and activator of transcription signaling via elevating suppressor of cytokine signaling 1. Front. Microbiol. 12, 752597. doi:10.3389/fmicb.2021.752597
Wang, S., Dai, T., Qin, Z., Pan, T., Chu, F., Lou, L., et al. (2021b). Targeting liquid-liquid phase separation of SARS-CoV-2 nucleocapsid protein promotes innate antiviral immunity by elevating MAVS activity. Nat. Cell Biol. 23, 718–732. doi:10.1038/s41556-021-00710-0
Wang, W., Zhou, Z., Xiao, X., Tian, Z., Dong, X., Wang, C., et al. (2021c). SARS-CoV-2 nsp12 attenuates type I interferon production by inhibiting IRF3 nuclear translocation. Cell Mol. Immunol. 18, 945–953. doi:10.1038/s41423-020-00619-y
Wang, X., Pernicone, N., Pertz, L., Hua, D., Zhang, T., Listovsky, T., et al. (2019). REV7 has a dynamic adaptor region to accommodate small GTPase RAN/Shigella IpaB ligands, and its activity is regulated by the RanGTP/GDP switch. J. Biol. Chem. 294, 15733–15742. doi:10.1074/jbc.RA119.010123
Wang, Y., Jenkins, S. A., Gu, C., Shree, A., Martinez-Moczygemba, M., Herold, J., et al. (2016a). Bacillus anthracis spore surface protein BclA mediates complement factor H binding to spores and promotes spore persistence. PLoS Pathog. 12, e1005678. doi:10.1371/journal.ppat.1005678
Wang, Y., Wei, Y., Yuan, S., Tao, H., Dong, J., Zhang, Z., et al. (2016b). Bacillus anthracis S-layer protein BslA binds to extracellular matrix by interacting with laminin. BMC Microbiol. 16, 183. doi:10.1186/s12866-016-0802-8
Wei, Z., Schnupf, P., Poussin, M. A., Zenewicz, L. A., Shen, H., and Goldfine, H. (2005). Characterization of Listeria monocytogenes expressing anthrolysin O and phosphatidylinositol-specific phospholipase C from Bacillus anthracis. Infect. Immun. 73, 6639–6646. doi:10.1128/IAI.73.10.6639-6646.2005
Wickham, M. E., Brown, N. F., Boyle, E. C., Coombes, B. K., and Finlay, B. B. (2007). Virulence is positively selected by transmission success between mammalian hosts. Curr. Biol. 17, 783–788. doi:10.1016/j.cub.2007.03.067
Wu, J., Shi, Y., Pan, X., Wu, S., Hou, R., Zhang, Y., et al. (2021). SARS-CoV-2 ORF9b inhibits RIG-I-MAVS antiviral signaling by interrupting K63-linked ubiquitination of NEMO. Cell Rep. 34, 108761. doi:10.1016/j.celrep.2021.108761
Wu, L., Wang, X., Li, Y., Liu, Y., Su, D., Fu, T., et al. (2016). Toxoplasma gondii ROP18: Potential to manipulate host cell mitochondrial apoptosis. Parasitol. Res. 115, 2415–2422. doi:10.1007/s00436-016-4993-6
Xia, H., Cao, Z., Xie, X., Zhang, X., Chen, J. Y.-C., Wang, H., et al. (2020). Evasion of type I interferon by SARS-CoV-2. Cell Rep. 33, 108234. doi:10.1016/j.celrep.2020.108234
Xia, J., Kong, L., Zhou, L.-J., Wu, S.-Z., Yao, L.-J., He, C., et al. (2018). Genome-wide bimolecular fluorescence complementation-based proteomic analysis of toxoplasma gondii ROP18’s human interactome shows its key role in regulation of cell immunity and apoptosis. Front. Immunol. 9, 61. doi:10.3389/fimmu.2018.00061
Yamamoto, M., Ma, J. S., Mueller, C., Kamiyama, N., Saiga, H., Kubo, E., et al. (2011). ATF6β is a host cellular target of the Toxoplasma gondii virulence factor ROP18. J. Exp. Med. 208, 1533–1546. doi:10.1084/jem.20101660
Yang, S.-C., Hung, C.-F., Aljuffali, I. A., and Fang, J.-Y. (2015). The roles of the virulence factor IpaB in Shigella spp. in the escape from immune cells and invasion of epithelial cells. Microbiol. Res. 181, 43–51. doi:10.1016/j.micres.2015.08.006
Yang, Y., Wu, Y., Meng, X., Wang, Z., Younis, M., Liu, Y., et al. (2022). SARS-CoV-2 membrane protein causes the mitochondrial apoptosis and pulmonary edema via targeting BOK. Cell Death Differ. 29, 1395–1408. doi:10.1038/s41418-022-00928-x
Yang, Z., Hou, Y., Hao, T., Rho, H.-S., Wan, J., Luan, Y., et al. (2017). A human proteome array approach to identifying key host proteins targeted by toxoplasma kinase ROP18. Mol. Cell Proteomics 16, 469–484. doi:10.1074/mcp.M116.063602
Yao, L., Xu, L., Zhou, L., Wu, S., Zou, W., Chen, M., et al. (2021). Toxoplasma gondii type-I ROP18 targeting human E3 ligase TRIM21 for immune escape. Front. Cell Dev. Biol. 9, 685913. doi:10.3389/fcell.2021.685913
Youn, Y.-J., Lee, Y.-B., Kim, S.-H., Jin, H. K., Bae, J.-S., and Hong, C.-W. (2021). Nucleocapsid and spike proteins of SARS-CoV-2 drive neutrophil extracellular trap formation. Immune Netw. 21, e16. doi:10.4110/in.2021.21.e16
Yu, S., Zheng, X., Zhou, B., Li, J., Chen, M., Deng, R., et al. (2022). SARS-CoV-2 spike engagement of ACE2 primes S2’ site cleavage and fusion initiation. Proc. Natl. Acad. Sci. U. S. A. 119, e2111199119. doi:10.1073/pnas.2111199119
Yuen, C.-K., Lam, J.-Y., Wong, W.-M., Mak, L.-F., Wang, X., Chu, H., et al. (2020). SARS-CoV-2 nsp13, nsp14, nsp15 and orf6 function as potent interferon antagonists. Emerg. Microbes Infect. 9, 1418–1428. doi:10.1080/22221751.2020.1780953
Zekarias, B., Mattoo, S., Worby, C., Lehmann, J., Rosenbusch, R. F., and Corbeil, L. B. (2010). Histophilus somni IbpA DR2/Fic in virulence and immunoprotection at the natural host alveolar epithelial barrier. Infect. Immun. 78, 1850–1858. doi:10.1128/IAI.01277-09
Zenewicz, L. A., Wei, Z., Goldfine, H., and Shen, H. (2005). Phosphatidylinositol-specific phospholipase C of Bacillus anthracis down-modulates the immune response. J. Immunol. 174, 8011–8016. doi:10.4049/jimmunol.174.12.8011
Zhang, K., Miorin, L., Makio, T., Dehghan, I., Gao, S., Xie, Y., et al. (2021a). Nsp1 protein of SARS-CoV-2 disrupts the mRNA export machinery to inhibit host gene expression. Sci. Adv. 7, eabe7386. doi:10.1126/sciadv.abe7386
Zhang, Q., Chen, Z., Huang, C., Sun, J., Xue, M., Feng, T., et al. (2021b). Severe acute respiratory syndrome coronavirus 2 (SARS-CoV-2) membrane (M) and spike (S) proteins antagonize host type I interferon response. Front. Cell Infect. Microbiol. 11, 766922. doi:10.3389/fcimb.2021.766922
Zhang, X., Yang, Z., Pan, T., Long, X., Sun, Q., Wang, P.-H., et al. (2022). SARS-CoV-2 ORF3a induces RETREG1/FAM134B-dependent reticulophagy and triggers sequential ER stress and inflammatory responses during SARS-CoV-2 infection. Autophagy 18, 2576–2592. doi:10.1080/15548627.2022.2039992
Zhang, Y., Chen, Y., Li, Y., Huang, F., Luo, B., Yuan, Y., et al. (2021c). The ORF8 protein of SARS-CoV-2 mediates immune evasion through down-regulating MHC-Ι. Proc. Natl. Acad. Sci. U. S. A. 118, e2024202118. doi:10.1073/pnas.2024202118
Zhang, Y., Sun, H., Pei, R., Mao, B., Zhao, Z., Li, H., et al. (2021d). The SARS-CoV-2 protein ORF3a inhibits fusion of autophagosomes with lysosomes. Cell Discov. 7, 31. doi:10.1038/s41421-021-00268-z
Zheng, Y., Deng, J., Han, L., Zhuang, M.-W., Xu, Y., Zhang, J., et al. (2022). SARS-CoV-2 NSP5 and N protein counteract the RIG-I signaling pathway by suppressing the formation of stress granules. Signal Transduct. Target Ther. 7, 22. doi:10.1038/s41392-022-00878-3
Zheng, Y., Zhuang, M.-W., Han, L., Zhang, J., Nan, M.-L., Zhan, P., et al. (2020). Severe acute respiratory syndrome coronavirus 2 (SARS-CoV-2) membrane (M) protein inhibits type I and III interferon production by targeting RIG-I/MDA-5 signaling. Signal Transduct. Target Ther. 5, 299. doi:10.1038/s41392-020-00438-7
Zhu, Y., Ge, X., Xie, D., Wang, S., Chen, F., and Pan, S. (2021). Clinical strains of Pseudomonas aeruginosa secrete LasB elastase to induce hemorrhagic diffuse alveolar damage in mice. J. Inflamm. Res. 14, 3767–3780. doi:10.2147/JIR.S322960
Keywords: microbial pathogenesis, DURC, functions of sequences of concern, FunSoCs, biothreat, biorisk, ontology
Citation: Godbold GD, Hewitt FC, Kappell AD, Scholz MB, Agar SL, Treangen TJ, Ternus KL, Sandbrink JB and Koblentz GD (2023) Improved understanding of biorisk for research involving microbial modification using annotated sequences of concern. Front. Bioeng. Biotechnol. 11:1124100. doi: 10.3389/fbioe.2023.1124100
Received: 14 December 2022; Accepted: 11 April 2023;
Published: 25 April 2023.
Edited by:
Andrea Wilcks, University of Copenhagen, DenmarkReviewed by:
Mamoon Rashid, King Abdullah International Medical Research Center (KAIMRC), Saudi ArabiaCopyright © 2023 Godbold, Hewitt, Kappell, Scholz, Agar, Treangen, Ternus, Sandbrink and Koblentz. This is an open-access article distributed under the terms of the Creative Commons Attribution License (CC BY). The use, distribution or reproduction in other forums is permitted, provided the original author(s) and the copyright owner(s) are credited and that the original publication in this journal is cited, in accordance with accepted academic practice. No use, distribution or reproduction is permitted which does not comply with these terms.
*Correspondence: Gene D. Godbold, Z2dvZGJvbGRAc2lnbmF0dXJlc2NpZW5jZS5jb20=; Gregory D. Koblentz, Z2tvYmxlbnRAZ211LmVkdQ==
†ORCID: Gene D. Godbold, http://orcid.org/0000-0002-5702-4690; F. Curtis Hewitt, http://orcid.org/0000-0001-9546-5625; Anthony D. Kappell, http://orcid.org/0000-0003-3511-9207; Matthew Scholz, http://orcid.org/ 0000-0003-3686-1227; Stacy L. Agar, http://orcid.org/0000-0002-6500-434X; Todd J. Treangen, http://orcid.org/0000-0002-3760-564X; Krista L. Ternus, http://orcid.org/0000-0003-1138-5308; Jonas B. Sandbrink, http://orcid.org/0000-0002-8244-956X; Gregory D. Koblentz, http://orcid.org/0000-0002-0793-1209
Disclaimer: All claims expressed in this article are solely those of the authors and do not necessarily represent those of their affiliated organizations, or those of the publisher, the editors and the reviewers. Any product that may be evaluated in this article or claim that may be made by its manufacturer is not guaranteed or endorsed by the publisher.
Research integrity at Frontiers
Learn more about the work of our research integrity team to safeguard the quality of each article we publish.