- 1The Second Affiliated Hospital of Chengdu Medical College, China National Nuclear Corporation 416 Hospital, Chengdu, Sichuan, China
- 2Center of Scientific Research, Chengdu Medical College, Chengdu, Sichuan, China
Acute kidney injury (AKI) is a clinical syndrome that results from a rapid decline in renal structure or renal functional impairment with the main pathological feature of sublethal and lethal damage to renal tubular cells. However, many potential therapeutic agents cannot achieve the desired therapeutic effect because of their poor pharmacokinetics and short retention time in the kidneys. With the recent emergence and progress of nanotechnology, nanodrugs with unique physicochemical properties could prolong circulation time, enhance efficient targeted delivery, and elevate the accumulation of therapeutics that can cross the glomerular filtration barrier and indicate comprehensive application prospects in the prevention and treatment of AKI. In this review, various types of nanosystems (such as liposomes, polymeric nanosystems, inorganic nanoparticles and cell-derived extracellular vesicles) are designed and applied to improve the pharmacokinetics of drug formation, which could further relieve the burden on the kidneys caused by the final cumulative dose of drugs in conventional treatments. Moreover, the passive or active targeting effect of nanosystems can also reduce the total therapeutic dose and off-target adverse effects on other organs. Nanodelivery systems for treating AKI that alleviate oxidative stress-induced renal cell damage and regulate the inflammatory kidney microenvironment are summarized.
1 Introduction
Acute kidney injury (AKI) is a clinical syndrome that results from a rapid decline in renal structure or renal functional impairment. It is considered a new global health problem due to its high incidence and rapid progression as well as its high mortality and disability rates (Kellum et al., 2021). Clinical causes include renal ischemia‒reperfusion (IR), nephrotoxic substances, sepsis, glomerulonephritis or interstitial nephritis, and extrarenal (such as prostatic hypertrophy) or intrarenal (such as kidney stones and thrombosis). The main pathological feature of AKI is sublethal and lethal damage to renal tubular cells. After AKI, the extant tubular cells undergo dedifferentiation, proliferation, and migration followed by redifferentiation to repair damaged tubular cells, which can be completely restored to intact tubules after mild injury (Sancho-Martínez et al., 2015). Moreover, severe or recurrent AKI often leads to abnormal repair of the kidney and renal fibrosis, accelerates renal function decline, and ultimately results in chronic kidney disease (CKD) and end-stage renal disease (ESRD) (Kaballo et al., 2017). Presently, the clinical treatment for AKI is mainly focused on supportive and symptomatic management as well as kidney transplantation. However, efficacy is still limited. Therefore, the development of a targeted therapeutic approach based on the pathophysiological mechanism of AKI has become a research Frontier and a hot topic (Gonsalez et al., 2019; Guo et al., 2019).
Nephron is composed of renal corpuscles and tubules. It is the basic structural and functional unit of the kidney to form urine. The renal corpuscles are composed of spherical glomeruli and renal sacs wrapped outside the glomeruli. The renal tubules are outside the renal sacs. The glomerulus is divided into three layers. The inner layer is the endothelial cell layer, which is a flat cell attached to the basement membrane of the glomerulus. There are numerous pores with a diameter of about 70–100 nm on it (Akilesh et al., 2008). The pores have a thin membrane. Such a structure allows larger molecules to easily pass through; The middle layer is the glomerular basement membrane, which is the main part of controlling the size of filtration molecules and the main part of mechanical barrier; The outer layer is the epithelial cell layer, namely the podocyte, which is the terminal differentiated cell. A variety of slit membrane proteins are intercalated with each other, forming a molecular sieve barrier that can prevent the leakage of medium and large molecular weight proteins. There is mesangial tissue between glomerular capillaries, including mesangial cells and mesangial matrix. Particles with a diameter of up to 5–7 nm under normal regulation can be filtered through the glomerulus and reach the top of the proximal tubular cells. The renal tubules are composed of proximal tubules, thin segments, distal tubules and connecting tubules. The proximal tubules are responsible for the active transport of endogenous and exogenous substances in the renal cells. The uptake of small molecules such as glucose, peptides, neurotransmitters and some large protein molecules all use active transport. Using this property to deliver drugs to proximal tubular cells through receptor-mediated endocytosis is a common delivery method of nanodrugs.
Since the kidney is the most important organ for elimination and excretion, many potential therapeutic agents cannot achieve a desired therapeutic effect because of their poor pharmacokinetics and short retention time in the kidneys (Aymanns et al., 2010). Therefore, therapeutic management strategies for AKI focus on the development of targeted therapeutics for the repair and regeneration of injured renal tubule cell (Liu C.-P. et al., 2019). In this review, we summarized the application of nanodelivery systems in AKI treatment by alleviating oxidative stress-induced renal cell damage and regulating the inflammatory kidney microenvironment. Various types of nanosystems have been designed and applied to improve the pharmacokinetics of drug formation, which could further relieve the burden on the kidneys caused by the final cumulative dose of a drug in conventional treatments (Figure 1). Moreover, the passive or active targeting effect of nanosystems can also reduce the total therapeutic dose and off-target adverse effects to other organs. Through this review, the recent progress in nanodrug-based anti-inflammatory strategies, by regulating intracellular oxidative stress, is emphasized and this may inspire the design of advanced nanodrugs and new promising strategies for AKI management.
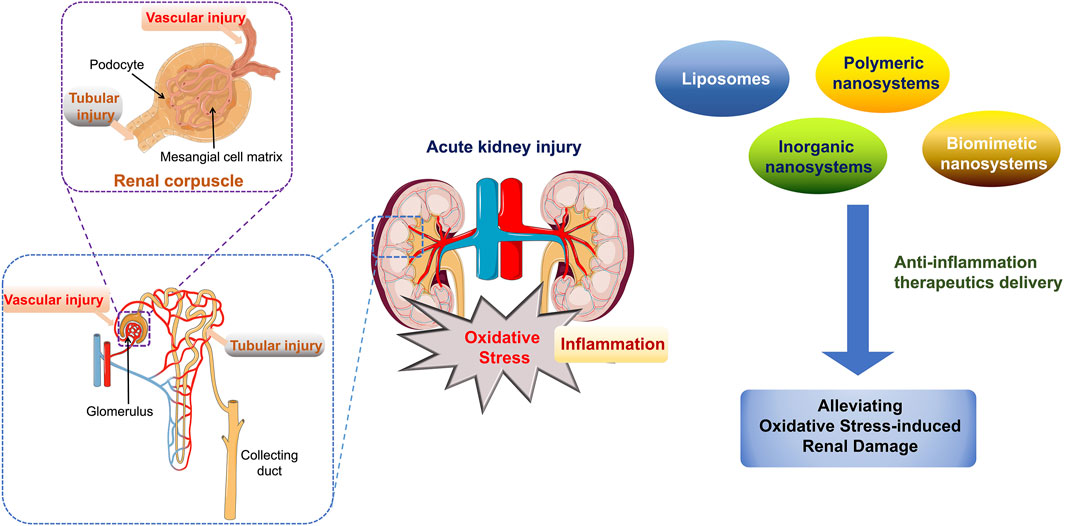
FIGURE 1. Schematic illustration of biomedical application of various nanosystems in AKI management that alleviate oxidative stress-induced renal cell damage.
2 The oxidative stress –related molecular biological mechanism of AKI
The pathophysiology of AKI involves in declining glomerular filtration rate (GFR) mediated by systemic or local hemodynamic alterations, reversible tubular injury, and tubular necrosis (Sharfuddin and Molitoris, 2011). During the process of renal tubular damage, injured tubular epithelial cells (TECs) may lead to apoptosis and necrosis (such as acute tubular necrosis) in severe injury and result in reduced excretion of metabolic waste products. Various disease characteristics, including renal ischemia-related cell damage and necrosis, inflammation and oxidative stress, and nephrotoxic injury, are observed during complex physiological and pathological processes (Bonventre and Yang, 2011). Many studies have demonstrated that a disrupted cellular redox system leads to dramatically increased reactive oxygen species (ROS) levels in renal tubular epithelial cells, which results in abnormal permeability of outer mitochondrial membranes and mitochondrial dysfunction when AKI occurs. Exacerbated oxidative stress induced by excess ROS in damaged mitochondria leads to apoptotic or necrotic cell death, and proinflammatory factors, such as tumor necrosis factor-α (TNF-α), monocyte chemotactic protein-1 (MCP-1), interleukin-8 (IL-8), IL-6, IL-1β, and transforming growth factor β (TGF-β) are subsequently released. Inflammation and chemokines participate in the chemotaxis and activation of leucocytes, which results in aggravated cellular inflammation (Sato and Yanagita, 2018). Simultaneously, inflammatory damage can also lead to structural damage and microvascular endothelial dysfunction in the tubulointerstitium, which leads to increased permeability (Schnaper, 2017). Moreover, injured microvascular endothelial cells and further leukocyte activation interact with adhesion molecules to actuate the immuno-inflammatory cascade. Most importantly, inflammatory effector cells are further activated and trigger the release of large amounts of inflammatory mediators, which leads to an amplified inflammatory response and further injury to renal tubular epithelial cells; this becomes a vicious cycle that ultimately leads to kidney dysfunction (Jang and Rabb, 2015; Chew and Hwang, 2019; Han and Lee, 2019).
In order to explore effective clinical therapeutic schedules, numerous studies have been reported to reveal the inflammatory response and cell signal transduction pathway during AKI, such as mitogen-activated protein kinase (MAPK), Toll-like receptor (TLR), and NF-κB signal pathway, which were considered as important inflammatory signaling after renal injury (Table 1).
3 The treatment of oxidative stress regulation for AKI
The restoration of renal perfusion and alleviation of intrarenal causes of AKI are the clinical guidelines for managing patients with AKI. Therapeutic options are required based on the different causes of AKI (Alge and Arthur, 2015; Gonsalez et al., 2019). However, there are currently no specific therapeutics for the prevention or treatment of AKI. Numerous studies have revealed that the pathogenesis of AKI involves a variety of pathological mechanisms, and hemodynamics and oxygen metabolism, inflammation, cell metabolism and oxidative stress, apoptosis, and pathways involved in cytothesis and fibrosis are potential therapeutic targets. This indicates that therapeutic agents for these pathological mechanisms and specifically targeting these signaling pathways may have potential applications for the prevention and management of AKI (Chen and Busse, 2017; Pickkers et al., 2022).
Recent studies have suggested that direct or indirect inflammation can significantly alleviate kidney injury in AKI animal models, which is manifested in a relative decrease in serum creatinine levels and a reduction in renal tubular necrosis (Andrade-Oliveira et al., 2015; Han and Lee, 2019). For instance, human recombinant alkaline phosphatase is an endogenous detoxification enzyme with anti-inflammatory effects. In a phase II trial of patients with sepsis-related AKI, it significantly improved long-term renal function and reduced mortality (Lazzareschi et al., 2022). Moreover, Sirtuin 1 (SIRT1) can inhibit the expression of inflammatory genes and reduce inflammatory tissue injury (Fan et al., 2013). Preclinical studies indicate that NAD+-dependent SIRT1 displays protective effects during inflammation-related renal injury by regulating oxidative metabolism in organisms. Therefore, improved SIRT1 activity protects and restores renal function by supplementing the precursor of NAD+ (Nicotinamide Riboside and Pterostilbene). It is important to note that this is an ongoing Phase II clinical trial (NCT04342975) (Guan et al., 2017; Chini et al., 2021).
Inflammation is accompanied by oxidative stress, and excess ROS are considered one of the key factors in AKI that provoke oxidative stress and excessive inflammatory response. Recent studies have revealed that the scavenging of ROS in the kidney can effectively regulate the microenvironment of injured renal tubular epithelial cells and alleviate oxidative stress-mediated pathological progression of AKI (Okamura and Pennathur, 2015). For instance, N-acetylcysteine (NAC) supplementation significantly reduced the incidence of AKI and overall mortality after cardiac surgery (Brown et al., 2009; Magner et al., 2022). In preclinical research, various natural antioxidant active ingredients, such as α-lipoic acid, curcumin, and selenium, can scavenge ROS through redox actions to balance intracellular redox. However, these active ingredients have not obtained satisfactory therapeutic effects during in vivo application, which is attributed to their rapid metabolism and excretion. As a result, they were seldom precisely delivered to injured kidney tissue and failed to protect endothelial and tubular cells (Iglesias et al., 2013; Jiang et al., 2013; Shanu et al., 2013; Trujillo et al., 2013; Cai et al., 2022).
Increasing evidence shows that the mitochondria produce approximately 90% of ROS in cells (Murphy and Hartley, 2018). Mitochondrial ROS in the local microenvironment of fragile tissues (such as kidneys) may initiate mitochondrial DNA (mtDNA) damage and activate inflammatory cascade reactions and promote end-stage organ damage (Ishimoto and Inagi, 2015; de Seigneux and Martin, 2017; Zhao M. et al., 2021). Among the agents acting on the mitochondria, Szeto-Schiller (SS) peptides are potential mitoprotective compounds that selectively bind to the cardiolipin of the inner membrane of mitochondria and protect against mitochondrial dysfunction. SS-31 is a small peptide that accumulates in the mitochondria as an ROS scavenger and can be rapidly transported to the heart, kidneys, lung, brain and other high perfusion organs via intravenous, intraperitoneal or subcutaneous administration (Birk et al., 2013). Preclinical studies have shown that SS-31 exhibits a specific effect on improving the mitochondrial function of renal tubular epithelial cells and is a potential candidate for AKI treatment (Szeto et al., 2011; Hou et al., 2016).
The renal glomerular filtration function based on molecular size and/or charge impedes the delivery of therapeutic agents after renal injury. The clinical treatment of AKI still mainly depends on renal replacement therapy (RRT) although the mortality risk is increased. Therapeutic strategies with enhanced accumulation of therapeutic targeted renal tubular cells are urgently needed in the clinic. With the recent emergence and progress of nanotechnology, nanomedicine with unique physicochemical properties could prolong circulation time, enhance the efficiency of targeted delivery, and elevate the accumulation of therapeutics in crossing the glomerular filtration barrier, which indicates comprehensive application prospects in the prevention and treatment of AKI (Huang Y. et al., 2021; Yu et al., 2021). Nano delivery systems as drug carriers display the diversity and specificity in reduced cytotoxicity, controllable biodistribution and pharmacokinetics, which could be applied in AKI therapy, as well as other renal disease management and diagnosis (Geo et al., 2022). Furthermore, a variety of nanomaterials with unique ROS-scavenging capabilities have also been applied for AKI treatment, and maintain the spatiotemporal equilibrium of biological redox systems, which has evoked nanocatalytic strategies for the regulation of ROS-related inflammation (Huang X. et al., 2021; Zhang C. et al., 2021; Dai et al., 2021).
Nanodrugs display not only targeted delivery and accumulation at specific regions but also regulate the pharmacokinetics and control the drug release in the disease microenvironment (Liu et al., 2023). Commonly, there are three kinds of targeting delivery strategy for kidney injury: 1) Passive targeting: Nanoparticles with a convertible size and surface charge are realized the delivery to renal mesangium (Li and Kataoka, 2021). Nanoparticles of a certain size can balance the affinity of proximal tubules, while biotinylated negative charge groups are easier to intercept in the kidney (Liang et al., 2016; Guo et al., 2017); 2) Active targeting: renal targeting is achieved by the specific combination of receptors on the surface of kidney cells and their ligands. For example, vascular adhesion molecule-1 is a cell surface receptor expressed on podocytes, which can promote receptor-mediated endocytosis for nanocarriers (Visweswaran et al., 2015); 3) Prolonging the circulation time of nanoparticles in vivo: for example, the exposure of enzymes/proteins to the compact folded structure of origami DNA is reduced, liver isolation, the opportunity of origami nanostructures with negative electricity to form protein halos is reduced, the uptake in the spleen and liver is reduced, and the excretion and accumulation of kidney are improved. In addition, it is also related to the water solubility and fat solubility, flexibility and rigidity, and density of nanoparticles (Jiang et al., 2018).
4 The application of nanosystems for oxidative stress regulation in AKI treatment
Recently, various types of nanosystems with controllable physical and chemical characteristics of size, charge, shape and surface ligand modification have been designed and fabricated to enhance the targeting delivery ability and improve therapeutic efficiency to improve the bioavailability of AKI therapeutic agents (Geo et al., 2022; Chen et al., 2023). In this section, liposomes, polymeric nanosystems, inorganic nanosystems and biomimetic nanosystems based on nanotechnology are explored as treatment strategies for AKI by alleviating the damage of oxidative stress to renal cells (Figure 2).
4.1 Liposomes and lipid nanoparticles
Liposomes are carrier systems used to deliver various therapeutic drugs in the research of various nanocarriers (Xing et al., 2016). The hydrophilic and hydrophobic chambers of liposomes enable them to have a high drug-loading capacity. Moreover, special targeting properties could be achieved when modified with ligands such as monoclonal antibodies (Cheng et al., 2022). For example, S.A. Asgeirdottir et al. prepared immune liposomes containing E-selectin antibodies and delivered the multifunctional anti-inflammatory drug dexamethasone (DXA) to inflamed glomerular endothelial cells, which not only selectively inhibit the expression of pro-inflammatory genes to reduce the renal damage caused by glomerulonephritis, but also prevented systemic side effects (Asgeirsdóttir et al., 2007). The high instability of miRNA in systemic circulation and enzyme degradation can also be solved by loading it into liposomes. The overexpression of this liposome in the kidney can significantly reduce the NF-kB-based inflammatory response of kidney cells induced by cisplatin (CDDP), and at the same time, by controlling the process of cell necrosis, prevent the release of endogenous inflammatory and proinflammatory components of cells after cell membrane rupture, thus reducing the severity of renal injury (Zhang S. et al., 2020).
Lipid nanoparticles refer to microcapsules with a much smaller particle size than liposomes, which can improve the solubility and biocompatibility of hydrophobic agents and have the flexibility of lipid modification. Peng Zhang et al. found that nanoliposomes have better renal targeting and can quickly enter the renal cell tissue through the intercellular space, similar to human fatty acids, and then efficiently and quickly enter the organelles inside renal cells. Additionally, they have no biological toxicity, do not produce allergic reactions, and reduce cell apoptosis to prevent renal injury caused by contrast agents (Zhang P. et al., 2021). Ligand-modified solid lipid nanoparticles also exhibited site-specific accumulation and controllable drug release profiles. Sialic acid (SA) is a ligand with a high affinity for the inflammatory vascular endothelium and significantly improves the therapeutic effect of dexamethasone-loaded solid lipid nanoparticles on renal ischemia‒reperfusion injury (IRI)-induced AKI. This nanosystem reduced the levels of superoxide dismutase (SOD) and glutathione (GSH), increased the activity of malondialdehyde enzyme, and decreased the level of oxidative stress of AKI. At the same time, it can also reduce the side effect of osteopenia caused by DXA during treatment (Hu et al., 2017c).
4.2 Polymeric nanosystems
Polymeric carriers are made of natural macromolecular materials or synthetic polymer materials through simple processes and are the most widely used drug carriers (Kang et al., 2016). Therapeutic drugs are covalently linked on polymeric materials or embedded in nanoparticles assembled by polymeric materials and alteration of the solubility and metabolism process occurs in vivo. Polymer nanocarriers have advantages of high drug encapsulation efficiency, good stability, controllable drug release, and protection of drugs from body fluids and enzymes (Yin et al., 2020).
4.2.1 Natural polymeric nanosystems
4.2.1.1 Natural polysaccharide-based nanosystems
Natural polysaccharide polymers are considered as ideal carriers and scaffolds in the field of drug delivery and tissue engineering because of their good biocompatibility and biodegradability (Yang et al., 2022). Low molecular weight chitosan (LMWC) is a natural linear polysaccharide generated from the deacetylation of chitin. Its main components are D-glucosamine and N-acetyl-D-glucamine. LMWC, as a drug carrier loaded with the antioxidant curcumin (CUR), can target the transport of CUR to renal tubular epithelial cells, which is conducive to drug aggregation in the kidneys. Compared with free drugs, chitosan-loaded drugs can prolong the circulation time of drugs in vivo, improve the distribution and accumulation of CUR in tissues, and improve the bioavailability of drugs through aggregation in target epitopes (Wang D.-W. et al., 2021). C. X. Yang designed chitosan nanoparticles to deliver siRNA to treat renal injury caused by urinary obstruction (UUO). Chitosan/siRNA nanoparticles can eliminate cyclooxygenase-2 (COX-2) in macrophages, and thereby reduce renal tubule injury, inflammation, cell apoptosis and oxidative stress to alleviate UUO-induced renal injury. This study targets the phagocytic activity of macrophages and at the same time avoids the serum instability of polymers. Through the homing property of macrophages, siRNA can be specifically gathered into the damaged kidney. Only a low dose of siRNA can achieve a therapeutic effect, and this is also a new treatment method for AKI (Yang et al., 2015). Hyaluronic acid (HA) and chitosan were prepared in nanoparticles by electrostatic composite action, which is used to load mitochondria-targeting antioxidant peptide SS-31 for AKI treatment. As HA is a natural polysaccharide with good biocompatibility and biodegradability, it can accumulate in tissues overexpressing CD44, such as renal tubular epithelial cells repaired after injury and vascular endothelial cells. This nanosystem increases the biological distribution of SS-31 in kidney tissue overexpressing the CD44 receptor and achieves effective delivery. Therefore, in the AKI model, it can reduce the degree of oxidative stress and inflammatory damage in renal tubule tissue and prevent diseases caused by apoptosis and necrosis of renal tubules (Liu D. et al., 2019).
Additionally, because of the specific interaction between the biomarker molecule of kidney injury (Kim-1) and serine, injured renal tubular epithelial cells targeting L-serine-modified chitosan (SC) was synthesized. The mitochondrial targeted antioxidant SS31was conjugated with SC through ROS cleavable thioketal bonds to prepare a polysaccharide drug precursor (SC-TK-SS31) that can be accurately targeted step by step, combine specific kidney distribution with ROS-responsive drug release behavior, endow the carrier with the ability to target AKI damaged organs (kidneys), cells (renal tubular epithelial cells) and organelles (mitochondria), and multiple combinations enhance the therapeutic effect of SS31 on AKI (Figure 3) (Liu D. et al., 2020).
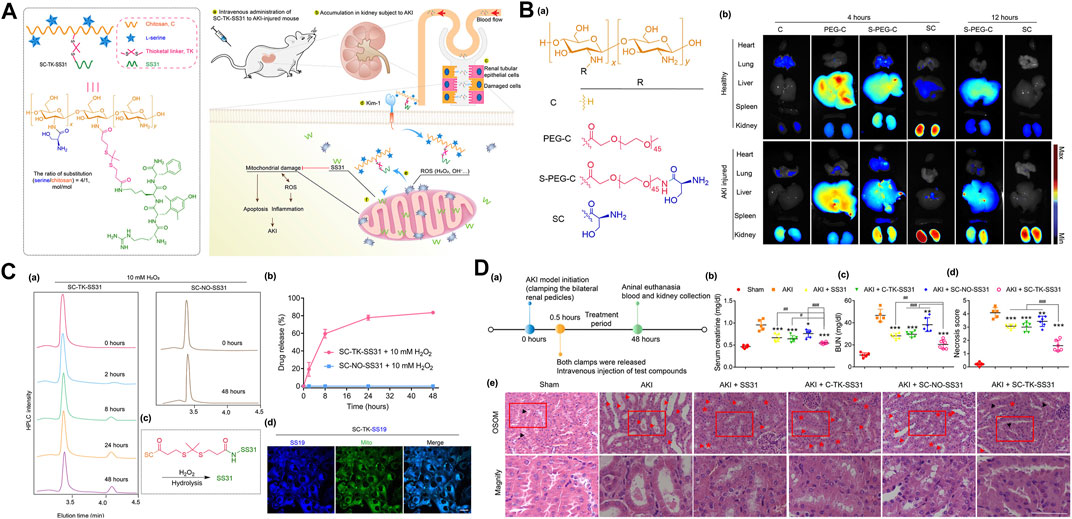
FIGURE 3. (A)Schematic illustration of the design and structural of ROS cleavable polysaccharide drug precursor SC-TK-SS31, and the principle for targeted AKI treatment; (B) (a) the design and structural of L-serine-modified chitosan (SC) and its analogues, (b) Fluorescent images of heart, lung, liver, spleen and kidney of mice at 4 or 12 h after intravenous injection of various of Cy5-labeled SC and its analogues; (C) (a) The absorption peak (220 nm) of SC-TK-SS31 monitored by HPLC in the presence of 10 mM H2O2, (b) the release curve of SC-TK-SS31 and SC-NO-SS31 dissolved in H2O2 (detected by HPLC), (c) Schematic diagram of SS-31 synthesis (d) Confocal image of HK-2 cells induced by H2O2 incubated with SC-TK-SS19 for 12 h. (D) (a) the schedule for the blood and kidney collection 48 h after intravenous injection of different drugs for AKI mice, (b) Determination of serum creatinine, (c) blood urea nitrogen (BUN) and (d) necrosis score in AKI mice after different treatments, (e) hematoxylin eosin (H&E) staining of renal tissue after different treatment. Adapted with permission from (Sci. Adv. 2020, 6 (41), eabb7422.) (Liu D. et al., 2020).
4.2.1.2 Protein and peptide-based nanosystems
Proteins/peptides have characteristics of low immunogenicity, low toxicity and good biocompatibility and have unique advantages as drug delivery systems. The protein carrier can also reduce the aggregation of nanoparticles. Bovine serum albumin (BSA) is used to improve the stability of selenium nanoparticles (SeNPs); the good biocompatibility will not cause toxic biological reactions and is also a simple and accessible material. Studies have proven that newly synthesized nanomaterial selenoprotein nanoparticles (Se@BSANPs) can reduce the activity of caspase-1 and the proinflammatory factor IL-1 by upregulating the level of GPx-1 and inhibiting the activation of NLRP3 inflammatory bodies in renal tubular epithelial cells after IRI β and IL-18. Se@BSANPs were enriched in the kidney by intravenous injection because this energetic drug regulates the GPx-1/NLRP3/Caspase-1 pathway, improves the renal injury of AKI mice induced by post ischemia perfusion in a dose-dependent manner, and significantly inhibits the subsequent renal fibrosis transformation caused by AKI. It is also a promising drug for treating AKI (Figure 4A) (Wang et al., 2022).
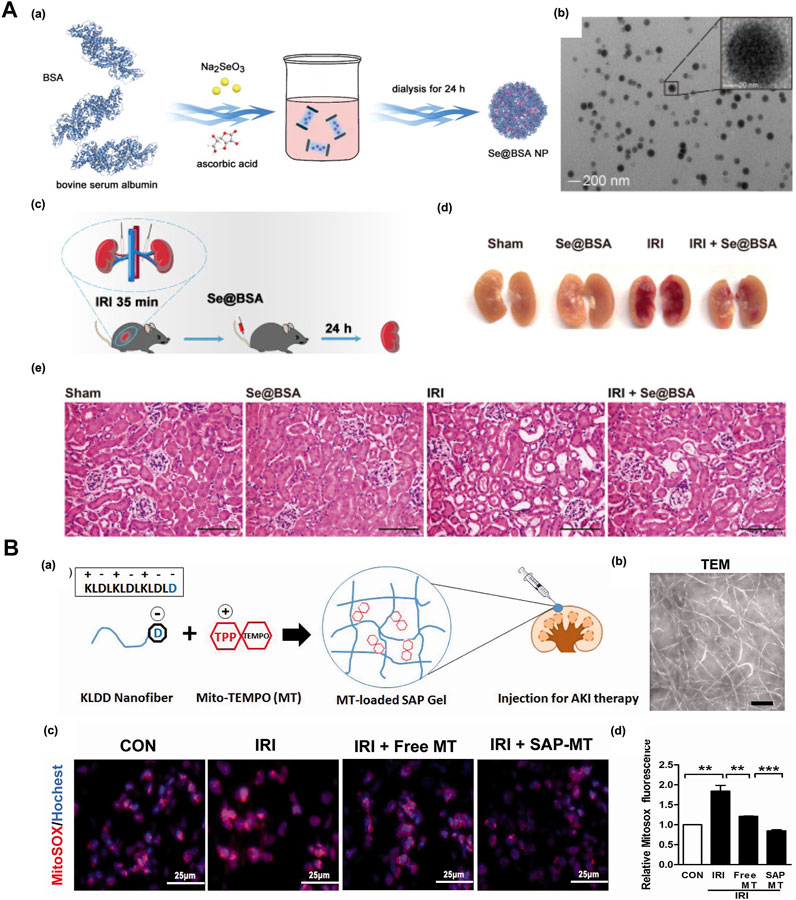
FIGURE 4. (A) (a) The scheme for synthesis of Se@BSA NPs, (b)Se@BSA NPs transmission electron microscope image. The region of interest is enlarged in the illustration, where the bare scale indicates 20 nm, (c) Schematic diagram of A)IRI-AKI mouse model establishment, (d) Selenium content in kidney of IRI-Akiaki mice. The results are shown as mean standard deviation, (e) HE staining of the kidneys of AKI mice with Se@BSA NPs treatment. Adapted with permission from (Theranostics. 2022, 12 (8), 3882–3895) (Wang et al., 2022); (B) (a) Schematic illustration of Mito TEMPO-loaded KLDD peptide hydrogel for AKI treatment, (b) TEM images of KLDD nanofibers, (c)The production of renal mtROS was detected by MitoSOX staining after frozen sections, (d) Evaluation of renal mtROS level staining. Adapted with permission from (Drug Delivery. 2018, 25 (1), 546–554) (Zhao et al., 2018).
The G3-C12 (ANTPCGPYTHDCPVKR) polypeptide was designed and found to have a renal targeting function after intravenous injection. Captopril (CAP), an angiotensin converting enzyme inhibitor (ACEI), is connected to the G3-C12 peptide through disulfide bonds to obtain a G3-C12-CAP conjugate with kidney-targeting ability. In vivo animal experiments show drug enrichment in the kidney and the inhibition of kidney ACE produced by the combination of the drug and peptide carrier (Geng et al., 2012).
Self-assembling peptide (SAP) can form hydrogels under physiological conditions without additional chemical initiators or UV light-induced cross-linking, and thus it can reduce the toxicity and immune reaction of the carrier during treatment. The anionic polypeptide KLDD is prepared by inserting the C-terminus of the self-assembling KLD-12 polypeptide (n-KLDLKLDLKLDL-c) into aspartic acid. Thee negatively charged KLDD nanofiber hydrogel can interact with positive mitochondria-targeting antioxidant Mito-2,6,6-tetramethylpiperidine-N-oxyl (Mito-TEMPO, MT) to regulate its release profiles in vitro. The slow release of MT provided continuous protection for IRI-induced mitochondrial dysfunction mice, which alleviated injury of renal tubular and inflammation, indicating the potential of SAP hydrogel as the antioxidant drugs carrier for renal repair after AKI (Figure 4B) (Zhao et al., 2018).
4.2.1.3 DNA origami nanostructure system
DNA nanotechnology constructs predictable and programmable nanostructures by using Watson Crick base pairs within and between molecules to design complex structures with different sizes (Linko et al., 2015; Zhang et al., 2015). DNA nanostructures have excellent in vivo properties, such as low toxicity, low immunogenicity and good biological stability (Lacroix and Sleiman, 2021; Ma et al., 2021). D. W. Jiang used the single step annealing program to construct three different forms of DNA origami nanostructures (DON): rectangular DON (90 nm × 60 nm), triangular DON (∼120 nm) and tubular DON (∼400 nm). Specifically, rectangular DON has renal protective properties and its efficacy is similar to that of the antioxidant N-acetylcysteine, which can improve AKI and protect renal function from the effects of nephrotoxic drugs (Jiang et al., 2018). Among the DNA nanostructures, the high stability and high accessibility in mammalian cells of tetrahedral skeleton nucleic acid (tFNA) enabled them as the potential therapeutic agent for AKI induced by rhabdomyolysis (RM) (Figure 5A). tFNA is the self-assembly of four single stranded DNA through the principle of base complementary pairing. As shown in the report, tFNA displayed selective targeting and accumulation to the lesion site in RM-AKI mice. The study proved that tFNA can inhibit the apoptosis of renal tubule cell, thereby reducing intracellular oxidative stress and mitochondrial dysfunction. In addition, tFNA displayed the therapeutic effect to alleviate the AKI without any other drug carrying (Zhang Q. et al., 2021).
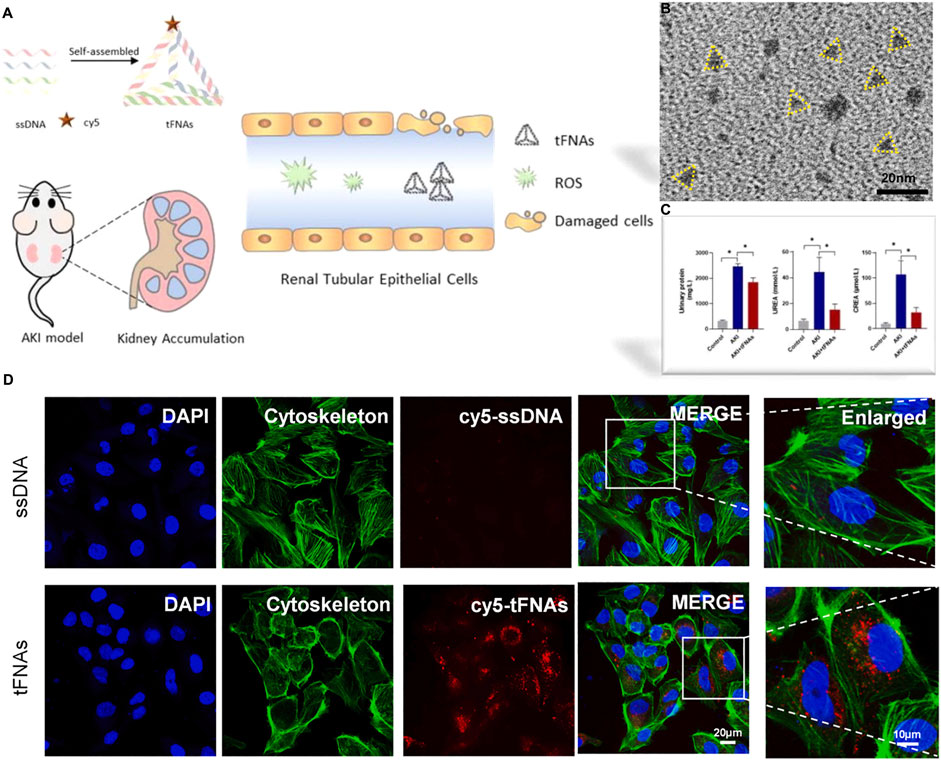
FIGURE 5. (A)Schematic illustration of tFNAs treating RM-induced AKI. TFNAs can reduce the oxidative stress and inhibit apoptosis by targeting renal tubular epithelial cells, showing kidney accumulation and alleviating kidney injury; (B) Representative TEM images of tFNAs; (C) Analysis of various factors and levels of AKI model mice before and after administration; (D) The uptake of Cy5-tFNAs and Cy5-ssDNA by HK-2 cells. Adapted with permission from (Chem. Eng. J., 2021, 413, 127426.) (Zhang Q. et al., 2021).
4.2.2 Synthetic polymeric nanosystems
In addition to natural polymers, synthetic polymers are widely used to prepare nanocarriers. Synthetic polymers can be formed by copolymerization or polycondensation of different monomers or preformed polymers and can precisely control the characteristics of nanoparticles through the modification of various monomer structures so that they can be widely used in drug delivery systems for AKI treatment. Mesoscale nanoparticle (MNPs) prepared from intravenous polyethylene glycol polylactic acid glycolic acid copolymer (PEG-PLGA), which are approved by the FDA, have a particle size of approximately 400 nm. In vivo distribution studies show that MNPs can be selectively locate in proximal renal tubular epithelial cells. The study also found that the selective localization of MNPs in renal tubules does not have a negative impact on the kidney (Williams et al., 2015). Furthermore, the nanoparticles are used to deliver a class of NEMO (NF-κB regulator) to the proximal tubule of the kidney. This peptide can block the binding of NEMO and the IKK complex and prevent NF-κB activation and reduce proinflammatory NF by inhibiting NEMO-κB signal generation to prevent ischemic AKI (Han et al., 2020). This MNP is also used to load the B2 adrenergic agonist formoterol and prepare the mesoscale nanoparticles (FNPs) that range between 400 and 500 nm. The nanoparticles can target renal tubular necrosis and fibrosis in mouse models that accumulate in renal tubules and can significantly reduce I/R damage, restore mitochondria and renal function, and avoid cardiovascular toxicity (Vallorz et al., 2022).
Polycation is an effective gene carrier, and the positive charge carried by the polycation can form a complex with gene drugs (pDNA or RNA) through electrostatic interactions and promote the endocytosis of the nanosystem by the effector cells. The linear polymeric chemokine receptor CXCR4 antagonist (PCX) is synthesized by the Michael addition copolymerization of phenyl cyclic derivatives and hexamethylene bisacrylamide. The polymer can effectively deliver p53 siRNA (sip53) to the kidney of AKI models with cisplatin and bilateral ischemia‒reperfusion injury and treat AKI by inhibiting CXCR4 and combining p53 gene silencing to improve kidney function and reduce kidney damag (Tang et al., 2022).
Reactive oxygen-responsive organic polymers (mPEG-TK-PLGA) were prepared by copolymerization of thioacetalinker with PEG and PLGA, which were used to load triphenylphosphine (TPP)-modified cerium nanoparticles and atorvastatin (ATV/PTP-TCeria NPs). This nanoparticle can be passively targeted into the mouse model of acute renal injury caused by sepsis with enhanced vascular permeability, and can passively target renal inflammatory injury to the vascular endothelium, protect the mitochondrial structure, and reduce the apoptosis and necrosis of renal tubular cells by reducing the oxidative stress and inflammatory reaction (Yu et al., 2020).
Perfluorocarbon nanoparticles (PFC NP) are a blood substitute approved by the FDA. The Proline-Phenylalanine-Arginine-Chloromethyl-Ketone (PPACK) polypeptide-conjugated PFC nanosystem formed by chemically coupling the thrombin inhibitor PPACK polypeptide can prevent thrombin signal transmission and microvascular thrombosis, limit inflammatory signal amplification, protect the renal tubule microstructure and reduce damage in atherosclerosis and AKI models (Vargas et al., 2021).
4.3 Inorganic nanosystems
Inorganic nanomaterials mainly refer to nanosystems that are constructed of inorganic materials (such as carbon, mesoporous silicon, calcium, gold, and iron), which have advantages of simple preparation, easy surface modification, a high drug-loading rate, small particle size, adjustable size, a large specific surface area, and good biocompatibility (Yang et al., 2019; Wang X. et al., 2021). Nanoenzyme systems based on inorganic nanomaterials reveal the inherent biological effects and new characteristics of nanomaterials and expand their scope of application. Nanozymes are artificial enzymes that are more stable, have a lower cost, have adjustable catalytic activity, are easy to modify and have other unique advantages over natural enzymes or traditional analog enzymes (Jiang et al., 2019; Cai et al., 2021). Additionally, nanomaterials have excellent physical and chemical properties, such as optics, magnetic fields, thermodynamics and large specific surface areas, which can endow nanozymes with multiple functions and regulate the catalytic activity and surface modification of nanoenzyme surfaces (Yang et al., 2018b; Wang et al., 2020b).
Research shows that the Prussian blue (PB) nanoenzyme system based on Prussian blue can effectively eliminate ROS in vivo and in vitro, and the ultrasmall PB nanoenzyme prepared with natural chitosan as a template can accumulate in the kidney tissue. Because of their excellent enrichment in the kidney and unique multienzyme simulation ability, ultrasmall PB nanoenzyme have shown better therapeutic effects than amifostine in rhabdomyolysis- or cisplatin-induced AKI mouse models (Zhang et al., 2021c). As a broad-spectrum ROS scavenger, self-assembled ultrasmall nanoparticles (FGP nanoparticles) composed of iron ions, gallic acid and polyvinylpyrrolidone can alleviate mouse AKI induced by glycerol and cisplatin (Zhang et al., 2021b). After the combination of Mn2+-chelated melanin (MMP) nanoparticles and polyethylene glycol, the obtained coordination assembled MMPP nanoparticles have the ability to eliminate a variety of reactive oxygen free radicals and inhibit the oxidative stress induced by reactive oxygen species (Sun et al., 2019). Ultrasmall Cu5.4O nanozymes simulate various biological enzymes and have a broad spectrum and efficient active oxygen scavenging capacity. They can reduce oxidative stress and inhibit NF by eliminating excessive ROS-κB signaling and downregulating proinflammatory factors such as TNF-α and IL-1β. The secretion of IL-6 reduces damage to kidney tissue (Figure 6A) (Liu S. et al., 2020b). Some studies have used the unique redox cycle characteristics of nanoceria, that is, it can cycle between Ce3+ (reduction) and Ce4+ (oxidation) states, which can improve its ability to scavenge active oxygen. As an antioxidant, citric acid-modified ceria nanoenzymes can effectively protect renal tubular epithelial cells against H2O2 stimulation in vitro. In addition, because of their very small size, cerium dioxide nanoparticles can effectively accumulate in the kidney, clear ROS in the kidneys, and protect kidney cells. Ultrasmall cerium dioxide nanoenzymes can be used as antioxidants to alleviate AKI caused by rhabdomyolysis and show great clinical potential (Zhang D.-Y. et al., 2020). Activation of the Nrf2/Keap1 signaling pathway regulates ROS-related genes by regulating the catalytic activity of cerium oxide nanoenzymes (CNPs) so that they can effectively eliminate ROS in the kidneys (a neutral microenvironment). In the acidic tumor microenvironment, excessive H+ interferes with the re-exposure of active catalytic sites, and inert CNPs no longer interfere with the generation of ROS mediated by chemotherapy for cancer treatment. Catalytic activity of the microenvironment response and adjustable CNP regulation of ROS can open up a new way for patients (especially patients receiving chemotherapy) to prevent AKI (Weng et al., 2021).
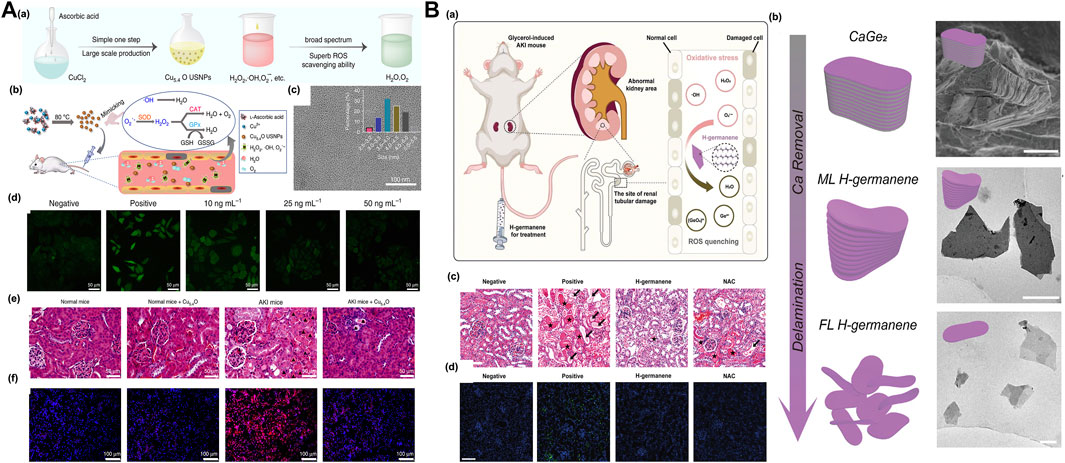
FIGURE 6. (A) (a) Schematic of synthetic process of Cu5.4O USNPs (ultrasmall nanoparticles) with broad ROS scavenging property, (b) Cu5.4O USNPs exhibited SOD, CAT and GPx-mimicking activity present the potentiality for treatment of oxidative stress related injury, (c) TEM image of Cu5.4O USNPs, (d) Representative ROS staining of HEK293 cells with different Cu5.4O USNPs treatment, the therapeutic efficacy of Cu5.4O USNPs in vivo: (e) H&E staining of kidney tissues (f) Dihydroethidium staining of kidney tissues for each group. Adapted with permission from (Nat. Commun. 2020, 11, 2788.) (Liu S. et al., 2020b); (B) (a) The schematic illustration of H-germanene as an ROS-quenching agent accumulated at the damaged renal tubular for AKI treatment, (b) characterization of precursor CaGe2 and H-germanene nanosheets: synthetic process of the H-germanene (left) and SEM image of the precursor CaGe2, TEM images of ML H-germanene and FL H-germanene (right), (c) H&E staining of the kidney tissues, (d) TUNEL staining of the kidney tissues. Adapted with permission from (Adv. Sci. 2022, 9, 2202933.) (Chen et al., 2022).
The 2D-H germanium nanosheet with flaky DNA geometry has a broad-spectrum free radical scavenging effect, which can effectively remove hydrogen peroxide (H2O2), superoxide anions (O2•-) and hydroxyl radicals (•OH). Because of their geometric structure that is similar to DNA, such new 2D nanomaterials can effectively accumulate in the kidneys, improve the side effects of conventional antioxidant drugs, such as N-acetylcysteine and amifostine, and avoid rapid metabolism, low utilization and low efficacy while having no significant toxicity for treating AKI (Figure 6B) (Chen et al., 2022). A new nanoenzyme antioxidant system based on ultrathin Ti3C2-PVP nanosheets (TPNSs) also has biodegradability triggered by enzymes/ROS. The nanoenzyme system shows a broad-spectrum ROS-scavenging ability, can inhibit the NF-κB signaling pathway, is used to inhibit the inflammatory reaction induced by oxidative stress and can treat related diseases caused by AKI and other ROS (Zhao X. et al., 2021).
4.4 Cell-derived extracellular vesicles and exosomes
Cell-derived extracellular vesicles and exosomes are extracellular membranous lipid vesicles that are secreted by cells. They play critical roles in cell signaling transportation by transferring proteins, RNA, DNA, and other substances for intercellular communication and become increasingly popular topics in disease diagnosis, treatment and prevention (Richter et al., 2021). Currently, exosomes are considered disease biomarkers and prognostic factors and exhibit further potential as delivery vehicles for gene and chemotherapy drugs with important clinical significance (Li et al., 2020).
A recent study found that IL-10-loaded extracellular vesicles (IL-10+ EVs) were produced from RAW macrophages by plasmid transfection and dexamethasone intervention. This IL-10+ EV, as part of the IL-10 delivery platform, could accumulate in tubular epithelial cells and macrophages after IRI. The therapeutic efficacy of IL-10 could be beneficial for maintaining mitochondrial homeostasis by suppressing mTOR signaling, significantly reducing inflammatory cell infiltration and alleviating pathological damage in the kidney, which displayed the potential for targeted therapy of IRI-AKI (Figure 7A) (Tang et al., 2020).
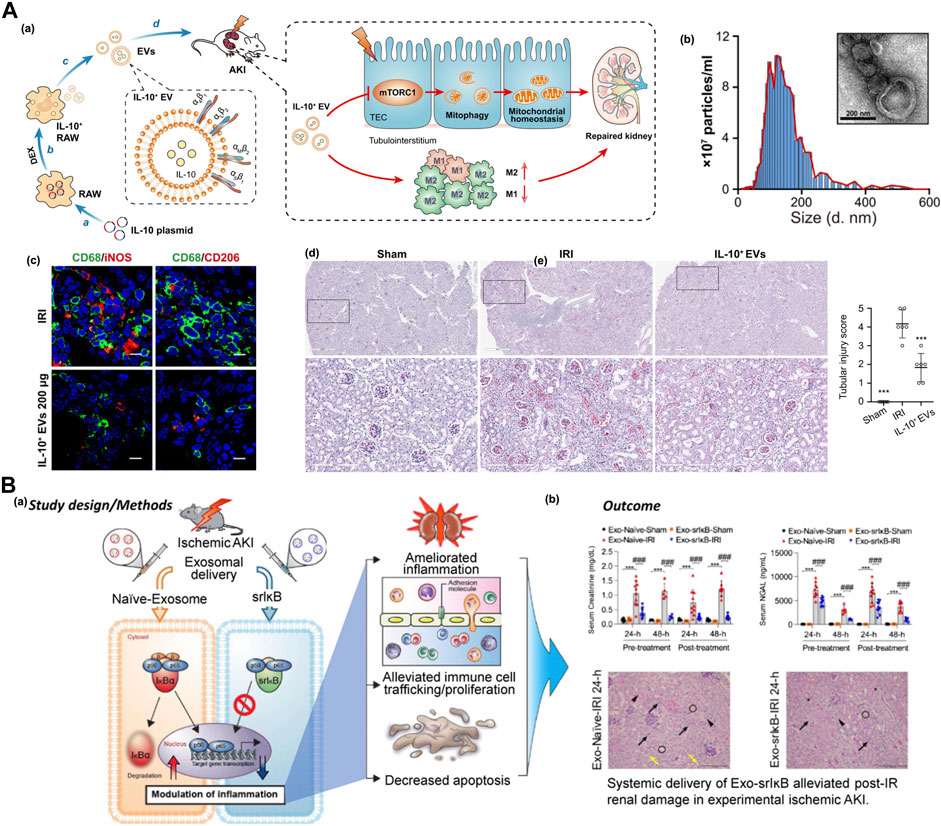
FIGURE 7. (A) (a) Schematic of the IL-10-loaded extracellular vesicles (IL-10 + EVs) with property of reducing inflammatory cell infiltration by mTOR inhition for targeted therapy of IRI-AKI, (b) the nano-/micro-morphology of IL-10+ EV and the size distribution, Scale bars, 200 nm, (c) the immunofluorescence images of M1 (CD68+iNOS+) phenotypes and M2 (CD68+CD206+) phenotype macrophages in kidney section with different treatment, Scale bars, 10 μm, (d) periodic acid–Schiff (PAS) staining of renal tissue after different treatment to evaluate tubular injury score. Adapted with permission from (Sci. Adv. 2020, 6, eaaz0748) (Tang et al., 2020); (B) (a) The schematic illustration of exosome-based anti-inflammatory super-repressor IκBα protein (SrIκB) reduce the off-target effects by targeting the cytoplasmic inflammatory signaling directly, which is differ from the conventional inhibition of NF-κB upstream signaling; (b) the efficacy of the anti-inflammatory exosomes for the treatment of IRI-AKI in vivo. In a preclinical IRI-AKI murine model, systemic administration of ILB-202 significantly reduced the AKI-associated serum biomarkers, BUN (blood urea nitrogen), creatine, and NGAL (neutrophil gelatinase-associated lipocalin). Adapted with permission from (Kidney Int. 2021, 100 (3), 570–584.) (Kim et al., 2021).
The kidney injury molecule-1 (Kim-1) is a transmembrane glycoprotein on renal proximal tubule epithelial cells whose expression is low in normal kidney tissue. However, the expression of Kim-1 is significantly upregulated in proximal tubular epithelial cells after IRI, which could serve as a novel biomarker for AKI (Rees and Kain, 2008; Vaidya et al., 2010; Boyacıoğlu et al., 2022). LTH peptide, a Kim-1–specific binding peptide, was designed and applied for the engineered modification of red blood cell-derived extracellular vesicles. This biomimetic nanosystem has been utilized to deliver siRNAs of P65 and Snai1 transcription factors to injured tubular epithelial cells. The inhibition of P65 and Snai1 simultaneously attenuated renal inflammation and fibrosis in a murine model of IRI and UUO, and slowed the progression of ischemic AKI (Tang et al., 2021).
ILB-202 (Exo-SrIκB) is an exosome-based therapeutic agent containing the anti-inflammatory superrepressor IκBα protein (SrIκB), which was developed for acute and chronic inflammatory diseases. SrIκB, the main active form of IκBα, was proven to attenuate inflammatory responses in various diseases by inhibiting NF-κB nuclear translocation. ILB-202 reduces off-target effects by directly targeting cytoplasmic inflammatory signaling, which differs from the conventional inhibition of NF-κB upstream signaling (Choi et al., 2020). The ILIAS company demonstrated the efficacy of anti-inflammatory exosomes for the treatment of IRI-AKI in vivo. In a preclinical IRI-AKI murine model, systemic administration of ILB-202 significantly reduced the AKI-associated serum biomarkers BUN (blood urea nitrogen), creatine, and NGAL (neutrophil gelatinase-associated lipocalin) (Figure 7B) (Kim et al., 2021).
4.5 Modification of nanosystems for active targeting in TKI therapy
In addition to their own specific enrichment effect, nanosystems can also achieve the active targeting drug delivery by surface modification, and thereby enhance the therapeutic effect of various antioxidant damage drugs on AKI (Yang et al., 2018a; Liu et al., 2021).
Renal tubular epithelial cells (RTECs), as the main undertaker of the renal reabsorption function, are one of the main sites of AKI. These are based on ε Physicochemical properties of polylysine and design and synthesis of natural peptide bond linked kidney-targeting peptide (Lysine-Lysine-Glutamic acid-Glutamic acid-Glutamic acid)3-Lysine, named (KKEEE)3K), which shows ideal pharmacokinetic characteristics and a high affinity for the kidneys (Wischnjow et al., 2016). This optimized polypeptide can bind to the receptor giant protein on the surface renal tubule cells to achieve targeted delivery. The amphiphilic micelles self-assembled by (KKEEE)3K peptide conjugated DSPE-PEG(2000)-amine with excellent renal proximal tubule cells targeting property, can principally reduce the off-targeting aggregation. (Wang et al., 2018). Although ligand-modified nanoparticles are used in the RTEC targeting strategy after systemic administration, NPs easily bind non-specific plasma proteins, which greatly weaken the targeting efficiency and stability of NPs. ShuoQin et al. buried tripterine (CLT) in D-α-tocopherol polyethylene glycol 1,000 succinate (TPGS) to form a nanocomposite core (CT) loaded with CLT, and then BSA was attached to the CT surface to afford a complete albumin corona without obvious denaturation (CTB). The experiment proved that the particle size distribution of CTB was uniform and stable both in vivo and in vitro, and CTB could be actively internalized in RTEC cells through macroprotein receptor-mediated endocytosis. CTB showed enhanced tubular-specific distribution and targeting in mice. In addition, pharmacodynamic studies in vivo further support the conclusion that CTB can reduce the IRI-AKI effect without obvious systemic side effects (Figure 8A) (Qin et al., 2022).
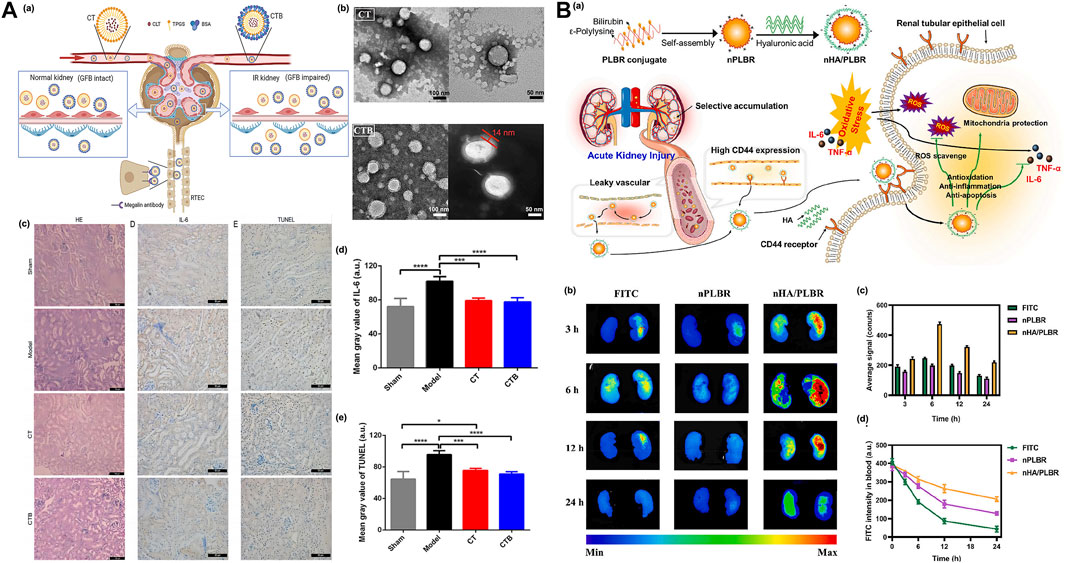
FIGURE 8. (A) (a) Schematic diagram of the action of CLT-TPGS nanocomposite (CT) in IR-AKI mice. GFB, glomerular filtration barrier; RTEC, renal tubular epithelial cells, (b) Transmission electron microscope (TEM) images of CT or CTB, (c) The results of H-E staining, representative images of IL-6 expression, and TUNEL method were used to detect the apoptosis of renal cells in different groups, (d) Semi-quantitative results of IL-6 expression, (e) Semi-quantitative results of renal cell apoptosis. Adapted with permission from (J. Control. Release 2022, 349, 401–412.) (Qin et al., 2022); (B) (a) nHA/PLBR can accumulate in damaged renal tubular epithelial cells by targeting CD44, (b) Renal fluorescence intensity of AKI rat models treated with three different drugs, (c) Fluorescence intensity analysis, (d) Comparison of FITC fluorescence intensity in blood of mice in each group after administration. Adapted with permission from (J. Control. Release 2021, 334, 275–289.) (Huang Z.-W. et al., 2021).
Vascular endothelial cells (VEC) are lined in the entire circulatory system from the heart to the capillaries and have unique vascular biological functions. In acute renal injury, glomerular vascular endothelial cells are damaged. Therefore, targeting anti-inflammatory drugs to endothelial cells with damaged glomerular blood vessels also reduces apoptosis and necrosis induced by oxidative stress/inflammation and can be used for the treatment of AKI. Selectin is a glycoprotein located in the membrane of endothelial cells (ECs) and is mainly used to participate in the recognition and adhesion of leukocytes and endothelial cells. After endothelial cells are activated by proinflammatory factors, the expression levels of P-selectin and E-selectin are highly upregulated. Blocking these two targets may have significant renoprotective effects. Therefore, selectin is considered an ideal target for drug delivery and the effective treatment of inflammatory, injury-related diseases such as AKI. Fucoidan is composed of L-fucose and sulfate, which can specifically recognize and adhere to inflammatory injured vascular endothelial cells that highly express P-selectin. Using fucoidan as the target, the kidneys target octyl succinic anhydride fucoidan/curcumin micelles (OSA Fucoidan/CUR) (Shu et al., 2021). Q. Kang prepared sialic acid polyethylene glycol dexamethasone (SA-PEG-DXM) coupling micelles. Because SA can specifically bind E-selectin, SA-modified drugs are more easily absorbed by damaged vascular ECs so more drug-loaded micelles accumulate in the kidneys of AKI model mice, which is also a new treatment strategy for AKI (Hu et al., 2017b). Hyaluronic acid (HA)-wrapped ε-polylysine bilirubin conjugate nanoparticles (nHA/PLBR) was designed and prepared by self-assembling with PLBR and followed by coating with HA though electrostatic interactions. The nanoparticles can accumulate in the injured renal tubular epithelial cells and vascular endothelial cells through the CD44 targeting effect mediated by HA. Bilirubin can regulate the polarization of macrophages to M2 (anti-inflammatory type), reduce oxidative stress/inflammatory induced damage, effectively protect the kidney from serious damage and maintain renal function (Figure 8B) (Huang Z.-W. et al., 2021).
In addition, some nanomaterials with properties of regulating oxidative stress are utilized for AKI management, for instance, ammonium functionalized carbon nanotubes (fCNT) for siRNA delivering siRNA, lecithin emulsion with F6H8 (perfluorohexane), and TNF-α neutralizing antibody and hepatocyte growth factor assembled hydrogel are reported to repair of damaged kidney tissue, which can inhibit the production of proinflammatory cytokines and the infiltration of macrophages in kidney tissue (Alidori et al., 2016; Tsagogiorgas et al., 2019; Liu S. et al., 2020b).
5 Conclusion and perspectives
In conclusion, individualized strategies for the prevention, diagnosis and management of AKI based on different causes and conditions are still a major challenge for clinical research. The incidence of AKI involves hemodynamic alterations, inflammation, and damage to the vascular endothelium and tubular epithelial cells. The dedifferentiation and proliferation of surviving epithelial cells gradually restores the functional integrity of the kidney tubular epithelium, which indicates the inherent self-repair capability of the epithelium, while maladaptive and abnormal repair leads to chronic kidney disease. The design of targeted treatment strategies to alleviate damage and accelerate repair based on a comprehensive understanding of the underlying pathophysiological process of acute kidney injury and repair is urgent.
Therefore, the alleviation of oxidative stress, inflammation and apoptosis in injured kidneys is crucial for AKI management. However, the application of antioxidant ingredients still faces challenges for AKI therapy. Conventional antioxidation treatment seldom delivers antioxidants to renal tubular epithelial cells accurately and fails to combat the cascade reaction of oxidative stress within damaged mitochondria since the kidney is an important excretory organ of the human body. Whether nanodrugs for antioxidant ingredient delivery or nanobiomaterials with free radical scavenging capability are used, nanosystems will still need to optimize their renal targeting efficacy and continuously adjust their metabolism processes in vivo to effectively improve drug efficacy and the therapeutic index in the management of AKI. In addition, promoting the stability of highly effective antioxidant-loaded nanosystems and their biosafety within physiological conditions in vivo has become an important cornerstone for future nanodrugs with clinical transformation in the personalized treatment of AKI. With a comprehensive understanding of the pathogenesis and molecular biology of AKI, innovative research on molecular pharmacology and nanodrug systems based on nanotechnology will benefit the personalized treatment of AKI with individual causes and conditions. In the future, an intensive investigation of the therapeutic action, pharmacokinetics, toxicology and biological mechanisms of antioxidant nanodrugs would promote relevant clinical trials and the application of clinical AKI management.
Author contributions
YW and HJ wrote the draft; YW and QY conceived and structured this review; LZ and PY did literature search for this work; QY and SW revised the draft. The manuscript has been read and approved by all named authors. All authors have read and agreed to the published version of the manuscript.
Funding
National Natural Science Foundation of China (grant number: NSFC31600811); the Science and Technology Program for Distinguished Young Scholar of Sichuan Province (grant number: 2020JDJQ0048); the CNNC 2021 Key Project of “Nuclear Medicine Science and Technology Innovation” (grant number: ZHYLZD2021005); the Zhong guancun Nephropathy Blood Purification Innovation Alliance CKD Youth Research Fund (grant number: NBPIA20QC0202); the Chengdu Medical Research Project form Chengdu Municipal Health Commission (grant numbers: 2022324 and 2021056).
Conflict of interest
The authors declare that the research was conducted in the absence of any commercial or financial relationships that could be construed as a potential conflict of interest.
Publisher’s note
All claims expressed in this article are solely those of the authors and do not necessarily represent those of their affiliated organizations, or those of the publisher, the editors and the reviewers. Any product that may be evaluated in this article, or claim that may be made by its manufacturer, is not guaranteed or endorsed by the publisher.
References
Akilesh, S., Huber, T. B., Wu, H., Wang, G., Hartleben, B., Kopp, J. B., et al. (2008). Podocytes use FcRn to clear IgG from the glomerular basement membrane. Proc. Natl. Acad. Sci. 105, 967–972. doi:10.1073/pnas.0711515105
Alge, J. L., and Arthur, J. M. (2015). Biomarkers of AKI: A review of mechanistic relevance and potential therapeutic implications. Clin. J. Am. Soc. Nephrol. 10, 147–155. doi:10.2215/cjn.12191213
Alidori, S., Akhavein, N., Thorek, D. L., Behling, K., Romin, Y., Queen, D., et al. (2016). Targeted fibrillar nanocarbon RNAi treatment of acute kidney injury. Sci. Transl. Med. 8, 331ra39–331ra339. doi:10.1126/scitranslmed.aac9647
Andrade-Oliveira, V., Amano, M. T., Correa-Costa, M., Castoldi, A., Felizardo, R. J. F., De Almeida, D. C., et al. (2015). Gut bacteria products prevent AKI induced by ischemia-reperfusion. J. Am. Soc. Nephrol. 26, 1877–1888. doi:10.1681/asn.2014030288
Asgeirsdóttir, S. A., Kamps, J. A., Bakker, H. I., Zwiers, P. J., Heeringa, P., Van Der Weide, K., et al. (2007). Site-specific inhibition of glomerulonephritis progression by targeted delivery of dexamethasone to glomerular endothelium. Mol. Pharm. 72, 121–131. doi:10.1124/mol.107.034140
Aymanns, C., Keller, F., Maus, S., Hartmann, B., and Czock, D. (2010). Review on pharmacokinetics and pharmacodynamics and the aging kidney. Clin. J. Am. Soc. Nephrol. 5, 314–327. doi:10.2215/cjn.03960609
Birk, A. V., Liu, S., Soong, Y., Mills, W., Singh, P., Warren, J. D., et al. (2013). The mitochondrial-targeted compound SS-31 Re-energizes ischemic mitochondria by interacting with cardiolipin. J. Am. Soc. Nephrol. 24, 1250–1261. doi:10.1681/asn.2012121216
Bonventre, J. V., and Yang, L. (2011). Cellular pathophysiology of ischemic acute kidney injury. J. Clin. Invest. 121, 4210–4221. doi:10.1172/jci45161
Boyacıoğlu, H., Yola, B. B., Karaman, C., Karaman, O., Atar, N., and Yola, M. L. (2022). A novel electrochemical kidney injury molecule-1 (KIM-1) immunosensor based covalent organic frameworks-gold nanoparticles composite and porous NiCo2S4@CeO2 microspheres: The monitoring of acute kidney injury. Appl. Surf. Sci. 578, 152093. doi:10.1016/j.apsusc.2021.152093
Brown, J. R., Block, C. A., Malenka, D. J., O'connor, G. T., Schoolwerth, A. C., and Thompson, C. A. (2009). Sodium bicarbonate plus N-acetylcysteine prophylaxis. JACC Cardiovasc. Interv. 2, 1116–1124. doi:10.1016/j.jcin.2009.07.015
Cai, X., Jiao, L., Yan, H., Wu, Y., Gu, W., Du, D., et al. (2021). Nanozyme-involved biomimetic cascade catalysis for biomedical applications. Mat. Today 44, 211–228. doi:10.1016/j.mattod.2020.12.005
Cai, Y., Huang, C., Zhou, M., Xu, S., Xie, Y., Gao, S., et al. (2022). Role of curcumin in the treatment of acute kidney injury: Research challenges and opportunities. Phytomedicine 104, 154306. doi:10.1016/j.phymed.2022.154306
Chen, H., and Busse, L. W. (2017). Novel therapies for acute kidney injury. Kidney Int. Rep. 2, 785–799. doi:10.1016/j.ekir.2017.06.020
Chen, Q., Nan, Y., Yang, Y., Xiao, Z., Liu, M., Huang, J., et al. (2023). Nanodrugs alleviate acute kidney injury: Manipulate RONS at kidney. Bioact. Mat. 22, 141–167. doi:10.1016/j.bioactmat.2022.09.021
Chen, Z., Qi, F., Qiu, W., Wu, C., Zong, M., Ge, M., et al. (2022). Hydrogenated germanene nanosheets as an antioxidative defense agent for acute kidney injury treatment. Adv. Sci. 9, e2202933. doi:10.1002/advs.202202933
Cheng, X., Yan, H., Pang, S., Ya, M., Qiu, F., Qin, P., et al. (2022). Liposomes as multifunctional nano-carriers for medicinal natural products. Front. Chem. 10, 963004. doi:10.3389/fchem.2022.963004
Chew, S. T. H., and Hwang, N. C. (2019). Acute kidney injury after cardiac surgery: A narrative review of the literature. J. Cardiothorac. Vasc. Anesth. 33, 1122–1138. doi:10.1053/j.jvca.2018.08.003
Chini, C. C. S., Zeidler, J. D., Kashyap, S., Warner, G., and Chini, E. N. (2021). Evolving concepts in NAD+ metabolism. Cell Metab. 33, 1076–1087. doi:10.1016/j.cmet.2021.04.003
Choi, H., Kim, Y., Mirzaaghasi, A., Heo, J., Kim, Y. N., Shin, J. H., et al. (2020). Exosome-based delivery of super-repressor IκBα relieves sepsis-associated organ damage and mortality. Sci. Adv. 6, eaaz6980. doi:10.1126/sciadv.aaz6980
Dai, Y., Ding, Y., and Li, L. (2021). Nanozymes for regulation of reactive oxygen species and disease therapy. Chin. Chem. Lett. 32, 2715–2728. doi:10.1016/j.cclet.2021.03.036
De Seigneux, S., and Martin, P.-Y. (2017). Preventing the progression of AKI to CKD: The role of mitochondria. Am. Soc. Nephrol. 28 (5), 1327–1329. doi:10.1681/asn.2017020146
Fan, H., Yang, H.-C., You, L., Wang, Y.-Y., He, W.-J., and Hao, C.-M. (2013). The histone deacetylase, SIRT1, contributes to the resistance of young mice to ischemia/reperfusion-induced acute kidney injury. Kidney Int. 83, 404–413. doi:10.1038/ki.2012.394
Geng, Q., Sun, X., Gong, T., and Zhang, Z.-R. (2012). Peptide–drug conjugate linked via a disulfide bond for kidney targeted drug delivery. Bioconjug. Chem. 23, 1200–1210. doi:10.1021/bc300020f
Geo, H. N., Murugan, D. D., Chik, Z., Norazit, A., Foo, Y. Y., Leo, B. F., et al. (2022). Renal Nano-drug delivery for acute kidney Injury: Current status and future perspectives. J. Control. Release 343, 237–254. doi:10.1016/j.jconrel.2022.01.033
Gonsalez, S. R., Cortês, A. L., Silva, R. C. D., Lowe, J., Prieto, M. C., and Silva Lara, L. D. (2019). Acute kidney injury overview: From basic findings to new prevention and therapy strategies. Pharmacol. Ther. 200, 1–12. doi:10.1016/j.pharmthera.2019.04.001
Guan, Y., Wang, S.-R., Huang, X.-Z., Xie, Q.-H., Xu, Y.-Y., Shang, D., et al. (2017). Nicotinamide mononucleotide, an NAD+ precursor, rescues age-associated susceptibility to AKI in a Sirtuin 1–dependent manner. J. Am. Soc. Nephrol. 28, 2337–2352. doi:10.1681/asn.2016040385
Guo, C., Dong, G., Liang, X., and Dong, Z. (2019). Epigenetic regulation in AKI and kidney repair: Mechanisms and therapeutic implications. Nat. Rev. Nephrol. 15, 220–239. doi:10.1038/s41581-018-0103-6
Guo, L., Luo, S., Du, Z., Zhou, M., Li, P., Fu, Y., et al. (2017). Targeted delivery of celastrol to mesangial cells is effective against mesangioproliferative glomerulonephritis. Nat. Commun. 8, 878. doi:10.1038/s41467-017-00834-8
Han, S. J., and Lee, H. T. (2019). Mechanisms and therapeutic targets of ischemic acute kidney injury. Kidney Res. Clin. Pract. 38, 427–440. doi:10.23876/j.krcp.19.062
Han, S. J., Williams, R. M., Kim, M., Heller, D. A., D’agati, V., Schmidt-Supprian, M., et al. (2020). Renal proximal tubular NEMO plays a critical role in ischemic acute kidney injury. JCI Insight 5, e139246. doi:10.1172/jci.insight.139246
Hou, Y., Li, S., Wu, M., Wei, J., Ren, Y., Du, C., et al. (2016). Mitochondria-targeted peptide SS-31 attenuates renal injury via an antioxidant effect in diabetic nephropathy. Am. J. Physiol. Ren. Physiol. 310, F547–F559. doi:10.1152/ajprenal.00574.2014
Hu, J. B., Kang, X. Q., Liang, J., Wang, X. J., Xu, X. L., Yang, P., et al. (2017c). E-selectin-targeted sialic acid-PEG-dexamethasone micelles for enhanced anti-inflammatory efficacy for acute kidney injury. Theranostics 7, 2204–2219. doi:10.7150/thno.19571
Hu, J. B., Song, G. L., Liu, D., Li, S. J., Wu, J. H., Kang, X. Q., et al. (2017b). Sialic acid-modified solid lipid nanoparticles as vascular endothelium-targeting carriers for ischemia-reperfusion-induced acute renal injury. Drug Deliv. 24, 1856–1867. doi:10.1080/10717544.2017.1410258
Hu, J., Chen, R., Jia, P., Fang, Y., Liu, T., Song, N., et al. (2017a). Augmented O-GlcNAc signaling via glucosamine attenuates oxidative stress and apoptosis following contrast-induced acute kidney injury in rats. Free Radic.Biol. Med. 103, 121–132. doi:10.1016/j.freeradbiomed.2016.12.032
Huang, X., He, D., Pan, Z., Luo, G., and Deng, J. (2021a). Reactive-oxygen-species-scavenging nanomaterials for resolving inflammation. Mat. Today Bio 11, 100124. doi:10.1016/j.mtbio.2021.100124
Huang, Y., Wang, J., Jiang, K., and Chung, E. J. (2021b). Improving kidney targeting: The influence of nanoparticle physicochemical properties on kidney interactions. J. Control. Release 334, 127–137. doi:10.1016/j.jconrel.2021.04.016
Huang, Z.-W., Shi, Y., Zhai, Y.-Y., Du, C.-C., Zhai, J., Yu, R.-J., et al. (2021c). Hyaluronic acid coated bilirubin nanoparticles attenuate ischemia reperfusion-induced acute kidney injury. J. Control. Release 334, 275–289. doi:10.1016/j.jconrel.2021.04.033
Iglesias, P., Selgas, R., Romero, S., and Díez, J. J. (2013). Selenium and kidney disease. J. Nephrol. 26, 266–272. doi:10.5301/jn.5000213
Ishimoto, Y., and Inagi, R. (2015). Mitochondria: A therapeutic target in acute kidney injury. Nephrol. Dial. Transpl. 31, 1062–1069. doi:10.1093/ndt/gfv317
Jang, H. R., and Rabb, H. (2015). Immune cells in experimental acute kidney injury. Nat. Rev. Nephrol. 11, 88–101. doi:10.1038/nrneph.2014.180
Jiang, D., Ge, Z., Im, H.-J., England, C. G., Ni, D., Hou, J., et al. (2018). DNA origami nanostructures can exhibit preferential renal uptake and alleviate acute kidney injury. Nat. Biomed. Eng. 2, 865–877. doi:10.1038/s41551-018-0317-8
Jiang, D., Ni, D., Rosenkrans, Z. T., Huang, P., Yan, X., and Cai, W. (2019). Nanozyme: New horizons for responsive biomedical applications. Chem. Soc. Rev. 48, 3683–3704. doi:10.1039/c8cs00718g
Jiang, S., Zhu, W., Li, C., Zhang, X., Lu, T., Ding, Z., et al. (2013). α-Lipoic acid attenuates LPS-induced cardiac dysfunction through a PI3K/Akt-dependent mechanism. Int. Immunopharmacol. 16, 100–107. doi:10.1016/j.intimp.2013.03.024
Kaballo, M. A., Elsayed, M. E., and Stack, A. G. (2017). Linking acute kidney injury to chronic kidney disease: The missing links. J. Nephrol. 30, 461–475. doi:10.1007/s40620-016-0359-5
Kang, H., Gravier, J., Bao, K., Wada, H., Lee, J. H., Baek, Y., et al. (2016). Renal clearable organic nanocarriers for bioimaging and drug delivery. Adv. Mat. 28, 8162–8168. doi:10.1002/adma.201601101
Kellum, J. A., Romagnani, P., Ashuntantang, G., Ronco, C., Zarbock, A., and Anders, H.-J. (2021). Acute kidney injury. Nat. Rev. Dis. Prim. 7, 52. doi:10.1038/s41572-021-00284-z
Kim, S., Lee, S. A., Yoon, H., Kim, M. Y., Yoo, J.-K., Ahn, S.-H., et al. (2021). Exosome-based delivery of super-repressor IκBα ameliorates kidney ischemia-reperfusion injury. Kidney Int. 100, 570–584. doi:10.1016/j.kint.2021.04.039
Lacroix, A., and Sleiman, H. F. (2021). DNA nanostructures: Current challenges and opportunities for cellular delivery. ACS Nano 15, 3631–3645. doi:10.1021/acsnano.0c06136
Lazzareschi, D., Mehta, R. L., Dember, L. M., Bernholz, J., Turan, A., Sharma, A., et al. (2022). Overcoming barriers in the design and implementation of clinical trials for acute kidney injury: A report from the 2020 kidney disease clinical trialists meeting. Nephrol. Dial. Transpl., gfac003. doi:10.1093/ndt/gfac003
Li, J., and Kataoka, K. (2021). Chemo-physical strategies to advance the in vivo functionality of targeted nanomedicine: The next generation. J. Am. Chem. Soc. 143, 538–559. doi:10.1021/jacs.0c09029
Li, M., Li, S., Du, C., Zhang, Y., Li, Y., Chu, L., et al. (2020). Exosomes from different cells: Characteristics, modifications, and therapeutic applications. Eur. J.Med. Chem. 207, 112784. doi:10.1016/j.ejmech.2020.112784
Liang, X., Wang, H., Zhu, Y., Zhang, R., Cogger, V. C., Liu, X., et al. (2016). Short-and long-term tracking of anionic ultrasmall nanoparticles in kidney. ACS Nano 10, 387–395. doi:10.1021/acsnano.5b05066
Linko, V., Ora, A., and Kostiainen, M. A. (2015). DNA nanostructures as smart drug-delivery vehicles and molecular devices. Trends Biotechnol. 33, 586–594. doi:10.1016/j.tibtech.2015.08.001
Liu, C.-P., Hu, Y., Lin, J.-C., Fu, H.-L., Lim, L. Y., and Yuan, Z.-X. (2019a). Targeting strategies for drug delivery to the kidney: From renal glomeruli to tubules. Med. Res. Rev. 39, 561–578. doi:10.1002/med.21532
Liu, D., Du, Y., Jin, F.-Y., Xu, X.-L., and Du, Y.-Z. (2021). Renal cell-targeted drug delivery strategy for acute kidney injury and chronic kidney disease: A mini-review. Mol. Pharm. 18, 3206–3222. doi:10.1021/acs.molpharmaceut.1c00511
Liu, D., Jin, F., Shu, G., Xu, X., Qi, J., Kang, X., et al. (2019b). Enhanced efficiency of mitochondria-targeted peptide SS-31 for acute kidney injury by pH-responsive and AKI-kidney targeted nanopolyplexes. Biomaterials 211, 57–67. doi:10.1016/j.biomaterials.2019.04.034
Liu, D., Shu, G., Jin, F., Qi, J., Xu, X., Du, Y., et al. (2020a). ROS-responsive chitosan-SS31 prodrug for AKI therapy via rapid distribution in the kidney and long-term retention in the renal tubule. Sci. Adv. 6, eabb7422. doi:10.1126/sciadv.abb7422
Liu, R., Luo, C., Pang, Z., Zhang, J., Ruan, S., Wu, M., et al. (2023). Advances of nanoparticles as drug delivery systems for disease diagnosis and treatment. Chin. Chem. Lett. 34, 107518. doi:10.1016/j.cclet.2022.05.032
Liu, S., Zhao, M., Zhou, Y., Li, L., Wang, C., Yuan, Y., et al. (2020b). A self-assembling peptide hydrogel-based drug co-delivery platform to improve tissue repair after ischemia-reperfusion injury. Acta Biomater. 103, 102–114. doi:10.1016/j.actbio.2019.12.011
Liu, T., Xiao, B., Xiang, F., Tan, J., Chen, Z., Zhang, X., et al. (2020b). Ultrasmall copper-based nanoparticles for reactive oxygen species scavenging and alleviation of inflammation related diseases. Nat. Commun. 11, 2788. doi:10.1038/s41467-020-16544-7
Ma, W., Zhan, Y., Zhang, Y., Mao, C., Xie, X., and Lin, Y. (2021). The biological applications of DNA nanomaterials: Current challenges and future directions. Signal Transduct. Target Ther. 6, 351. doi:10.1038/s41392-021-00727-9
Magner, K., Ilin, J. V., Clark, E. G., Kong, J. W. Y., Davis, A., and Hiremath, S. (2022). Meta-analytic techniques to assess the association between N-acetylcysteine and acute kidney injury after contrast administration: A systematic review and meta-analysis. JAMA Netw. Open 5, e2220671. doi:10.1001/jamanetworkopen.2022.20671
Murphy, M. P., and Hartley, R. C. (2018). Mitochondria as a therapeutic target for common pathologies. Nat. Rev. Drug Discov. 17, 865–886. doi:10.1038/nrd.2018.174
Okamura, D. M., and Pennathur, S. (2015). The balance of powers: Redox regulation of fibrogenic pathways in kidney injury. Redox. Biol. 6, 495–504. doi:10.1016/j.redox.2015.09.039
Pei, J., Cai, S., Song, S., Xu, Y., Feng, M., Luo, G., et al. (2020). Normobaric hyperoxia plays a protective role against renal ischemia-reperfusion injury by activating the Nrf2/HO-1 signaling pathway. Biochem. Biophys. Res. Commun. 532, 151–158. doi:10.1016/j.bbrc.2020.07.004
Pickkers, P., Murray, P. T., and Ostermann, M. (2022). New drugs for acute kidney injury. Intensive Care Med. 48, 1796–1798. doi:10.1007/s00134-022-06859-y
Qin, S., Wu, B., Gong, T., Zhang, Z.-R., and Fu, Y. (2022). Targeted delivery via albumin corona nanocomplex to renal tubules to alleviate acute kidney injury. J. Control. Release 349, 401–412. doi:10.1016/j.jconrel.2022.07.013
Qiu, W., Chen, N., Zhang, Q., Zhuo, L., Wang, X., Wang, D., et al. (2014). Resistin increases platelet P-selectin levels via p38 MAPK signal pathway. Diab. Vasc. Dis. Res. 11, 121–124. doi:10.1177/1479164113513912
Rees, A. J., and Kain, R. (2008). Kim-1/Tim-1: From biomarker to therapeutic target? Nephrol. Dial. Transpl. 23, 3394–3396. doi:10.1093/ndt/gfn480
Richter, M., Vader, P., and Fuhrmann, G. (2021). Approaches to surface engineering of extracellular vesicles. Adv. Drug Deliv. Rev. 173, 416–426. doi:10.1016/j.addr.2021.03.020
Sancho-Martínez, S. M., López-Novoa, J. M., and López-Hernández, F. J. (2015). Pathophysiological role of different tubular epithelial cell death modes in acute kidney injury. Clin. Kidney J. 8, 548–559. doi:10.1093/ckj/sfv069
Sato, Y., and Yanagita, M. (2018). Immune cells and inflammation in AKI to CKD progression. Am. J. Physiol. Ren. Physiol. 315, F1501–F1512. doi:10.1152/ajprenal.00195.2018
Schnaper, H. W. (2017). The tubulointerstitial pathophysiology of progressive kidney disease. Adv. Chronic. Kidney Dis. 24, 107–116. doi:10.1053/j.ackd.2016.11.011
Shanu, A., Kim, H. B., Wood, S., Weekley, C. M., Jade, B. A., Harris, H. H., et al. (2013). Selenium inhibits renal oxidation and inflammation but not acute kidney injury in an animal model of rhabdomyolysis. Antioxid. Redox Signal. 18, 756–769. doi:10.1089/ars.2012.4591
Sharfuddin, A. A., and Molitoris, B. A. (2011). Pathophysiology of ischemic acute kidney injury. Nat. Rev. Nephrol. 7, 189–200. doi:10.1038/nrneph.2011.16
Shu, G., Lu, C., Wang, Z., Du, Y., Xu, X., Xu, M., et al. (2021). Fucoidan-based micelles as P-selectin targeted carriers for synergistic treatment of acute kidney injury. Nanomed-Nanotechnol. 32, 102342. doi:10.1016/j.nano.2020.102342
Sun, T., Jiang, D., Rosenkrans, Z. T., Ehlerding, E. B., Ni, D., Qi, C., et al. (2019). A melanin-based natural antioxidant defense nanosystem for theranostic application in acute kidney injury. Adv. Funct. 29, 1904833. doi:10.1002/adfm.201904833
Szeto, H. H., Liu, S., Soong, Y., Wu, D., Darrah, S. F., Cheng, F.-Y., et al. (2011). Mitochondria-targeted peptide accelerates ATP recovery and reduces ischemic kidney injury. J. Am. Soc. Nephrol. 22, 1041–1052. doi:10.1681/asn.2010080808
Tang, T.-T., Wang, B., Li, Z.-L., Wen, Y., Feng, S.-T., Wu, M., et al. (2021). Kim-1 targeted extracellular vesicles: A new therapeutic platform for RNAi to treat AKI. J. Am. Soc. Nephrol. 32, 2467–2483. doi:10.1681/asn.2020111561
Tang, T.-T., Wang, B., Wu, M., Li, Z.-L., Feng, Y., Cao, J.-Y., et al. (2020). Extracellular vesicle-encapsulated IL-10 as novel nanotherapeutics against ischemic AKI. Sci. Adv. 6, eaaz0748. doi:10.1126/sciadv.aaz0748
Tang, W., Chen, Y., Jang, H.-S., Hang, Y., Jogdeo, C. M., Li, J., et al. (2022). Preferential siRNA delivery to injured kidneys for combination treatment of acute kidney injury. J. Control. Release 341, 300–313. doi:10.1016/j.jconrel.2021.11.029
Trujillo, J., Chirino, Y. I., Molina-Jijón, E., Andérica-Romero, A. C., Tapia, E., and Pedraza-Chaverrí, J. (2013). Renoprotective effect of the antioxidant curcumin: Recent findings. Redox. Biol. 1, 448–456. doi:10.1016/j.redox.2013.09.003
Tsagogiorgas, C., Anger, F., Beck, G., Breedijk, A., Yard, B., and Hoeger, S. (2019). <p>Impact of different emulsifiers on biocompatibility and inflammatory potential of Perfluorohexyloctane (F6H8) emulsions for new intravenous drug delivery systems</p>. Drug Des. Dev. Ther. 13, 2097–2110. doi:10.2147/dddt.s195954
Vaidya, V. S., Ozer, J. S., Dieterle, F., Collings, F. B., Ramirez, V., Troth, S., et al. (2010). Kidney injury molecule-1 outperforms traditional biomarkers of kidney injury in preclinical biomarker qualification studies. Nat. Biotechnol. 28, 478–485. doi:10.1038/nbt.1623
Vallorz, E. L., Janda, J., Mansour, H. M., and Schnellmann, R. G. (2022). Kidney targeting of formoterol containing polymeric nanoparticles improves recovery from ischemia reperfusion-induced acute kidney injury in mice. Kidney Int. 102, 1073–1089. doi:10.1016/j.kint.2022.05.032
Vargas, I., Stephenson, D. J., Baldwin, M., Gaut, J. P., Chalfant, C. E., Pan, H., et al. (2021). Sustained local inhibition of thrombin preserves renal microarchitecture and function after onset of acute kidney injury. Nanomed-Nanotechnol. 38, 102449. doi:10.1016/j.nano.2021.102449
Visweswaran, G. R. R., Gholizadeh, S., Ruiters, M. H., Molema, G., Kok, R. J., and Kamps, J. A. (2015). Targeting rapamycin to podocytes using a vascular cell adhesion molecule-1 (VCAM-1)-harnessed SAINT-based lipid carrier system. PLoS One 10, e0138870. doi:10.1371/journal.pone.0138870
Wang, D.-W., Li, S.-J., Tan, X.-Y., Wang, J.-H., Hu, Y., Tan, Z., et al. (2021a). Engineering of stepwise-targeting chitosan oligosaccharide conjugate for the treatment of acute kidney injury. Carbohydr. Polym. 256, 117556. doi:10.1016/j.carbpol.2020.117556
Wang, H., Li, D., Hu, Z., Zhao, S., Zheng, Z., and Li, W. (2016). Protective effects of green tea polyphenol against renal injury through ROS-mediated JNK-MAPK pathway in lead exposed rats. Mol. Cells 39, 508–513. doi:10.14348/molcells.2016.2170
Wang, J., Poon, C., Chin, D., Milkowski, S., Lu, V., Hallows, K. R., et al. (2018). Design and in vivo characterization of kidney-targeting multimodal micelles for renal drug delivery. Nano Res. 11, 5584–5595. doi:10.1007/s12274-018-2100-2
Wang, S., Chen, Y., Han, S., Liu, Y., Gao, J., Huang, Y., et al. (2022). Selenium nanoparticles alleviate ischemia reperfusion injury-induced acute kidney injury by modulating GPx-1/NLRP3/Caspase-1 pathway. Theranostics 12, 3882–3895. doi:10.7150/thno.70830
Wang, X., Zhong, X., Li, J., Liu, Z., and Cheng, L. (2021b). Inorganic nanomaterials with rapid clearance for biomedical applications. Chem. Soc. Rev. 50, 8669–8742. doi:10.1039/d0cs00461h
Wang, Z., Wu, J., Hu, Z., Luo, C., Wang, P., Zhang, Y., et al. (2020a). Dexmedetomidine alleviates lipopolysaccharide-induced acute kidney injury by inhibiting p75NTR-mediated oxidative stress and apoptosis. Oxid. Med. Cell. Longev. 2020, 1–13. doi:10.1155/2020/5454210
Wang, Z., Zhang, R., Yan, X., and Fan, K. (2020b). Structure and activity of nanozymes: Inspirations for de novo design of nanozymes. Mat. Today 41, 81–119. doi:10.1016/j.mattod.2020.08.020
Weng, Q., Sun, H., Fang, C., Xia, F., Liao, H., Lee, J., et al. (2021). Catalytic activity tunable ceria nanoparticles prevent chemotherapy-induced acute kidney injury without interference with chemotherapeutics. Nat. Commun. 12, 1436. doi:10.1038/s41467-021-21714-2
Williams, R. M., Shah, J., Ng, B. D., Minton, D. R., Gudas, L. J., Park, C. Y., et al. (2015). Mesoscale nanoparticles selectively target the renal proximal tubule epithelium. Nano Lett. 15, 2358–2364. doi:10.1021/nl504610d
Wischnjow, A., Sarko, D., Janzer, M., Kaufman, C., Beijer, B., Brings, S., et al. (2016). Renal targeting: Peptide-based drug delivery to proximal tubule cells. Bioconjug. Chem. 27, 1050–1057. doi:10.1021/acs.bioconjchem.6b00057
Xing, H., Hwang, K., and Lu, Y. (2016). Recent developments of liposomes as nanocarriers for theranostic applications. Theranostics 6, 1336–1352. doi:10.7150/thno.15464
Yang, C., Nilsson, L., Cheema, M. U., Wang, Y., Frøkiær, J., Gao, S., et al. (2015). Chitosan/siRNA nanoparticles targeting cyclooxygenase type 2 attenuate unilateral ureteral obstruction-induced kidney injury in mice. Theranostics 5, 110–123. doi:10.7150/thno.9717
Yang, G., Phua, S. Z. F., Bindra, A. K., and Zhao, Y. (2019). Degradability and clearance of inorganic nanoparticles for biomedical applications. Adv. Mat. 31, 1805730. doi:10.1002/adma.201805730
Yang, Q., Peng, J., Chen, C., Xiao, Y., Tan, L., Xie, X., et al. (2018a). Targeting delivery of rapamycin with anti-collagen IV peptide conjugated Fe3O4@ nanogels system for vascular restenosis therapy. J. Biomed. Nanotechnol. 14, 1208–1224. doi:10.1166/jbn.2018.2588
Yang, Q., Peng, J., Xiao, H., Xu, X., and Qian, Z. (2022). Polysaccharide hydrogels: Functionalization, construction and served as scaffold for tissue engineering. Carbohydr. Polym. 278, 118952. doi:10.1016/j.carbpol.2021.118952
Yang, Q., Peng, J., Xiao, Y., Li, W., Tan, L., Xu, X., et al. (2018b). Porous Au@Pt nanoparticles: Therapeutic platform for tumor chemo-photothermal Co-therapy and alleviating doxorubicin-induced oxidative damage. ACS Appl. Mat. Interfaces 10, 150–164. doi:10.1021/acsami.7b14705
Yifan, Z., Benxiang, N., Zheng, X., Luwei, X., Liuhua, Z., Yuzheng, G., et al. (2020). Ceftriaxone calcium crystals induce acute kidney injury by NLRP3-mediated inflammation and oxidative stress injury. Oxid. Med. Cell. Longev. 2020, 1–13. doi:10.1155/2020/6428498
Yin, Y., Hu, B., Yuan, X., Cai, L., Gao, H., and Yang, Q. (2020). Nanogel: A versatile nano-delivery system for biomedical applications. Pharmaceutics 12, 290. doi:10.3390/pharmaceutics12030290
Yu, H., Jin, F., Liu, D., Shu, G., Wang, X., Qi, J., et al. (2020). ROS-responsive nano-drug delivery system combining mitochondria-targeting ceria nanoparticles with atorvastatin for acute kidney injury. Theranostics 10, 2342–2357. doi:10.7150/thno.40395
Yu, H., Liu, D., Shu, G., Jin, F., and Du, Y. (2021). Recent advances in nanotherapeutics for the treatment and prevention of acute kidney injury. Asian J. Pharm. Sci. 16, 432–443. doi:10.1016/j.ajps.2020.11.001
Zhang, C., Wang, X., Du, J., Gu, Z., and Zhao, Y. (2021a). Reactive oxygen species-regulating strategies based on nanomaterials for disease treatment. Adv. Sci. 8, 2002797. doi:10.1002/advs.202002797
Zhang, D.-Y., Liu, H., He, T., Younis, M. R., Tu, T., Yang, C., et al. (2021b). Biodegradable nanodots: Biodegradable self-assembled ultrasmall nanodots as reactive oxygen/nitrogen species scavengers for theranostic application in acute kidney injury (small 8/2021). Small 17, 2170033. doi:10.1002/smll.202170033
Zhang, D.-Y., Liu, H., Li, C., Younis, M. R., Lei, S., Yang, C., et al. (2020a). Ceria nanozymes with preferential renal uptake for acute kidney injury alleviation. ACS Appl. Mat. Interfaces 12, 56830–56838. doi:10.1021/acsami.0c17579
Zhang, D.-Y., Liu, H., Zhu, K. S., He, T., Younis, M. R., Yang, C., et al. (2021c). Prussian blue-based theranostics for ameliorating acute kidney injury. J. Nanobiotechnol. 19, 266. doi:10.1186/s12951-021-01006-z
Zhang, F., Jiang, S., Wu, S., Li, Y., Mao, C., Liu, Y., et al. (2015). Complex wireframe DNA origami nanostructures with multi-arm junction vertices. Nat. Nanotechnol. 10, 779–784. doi:10.1038/nnano.2015.162
Zhang, J., Luan, Z.-L., Huo, X.-K., Zhang, M., Morisseau, C., Sun, C.-P., et al. (2023a). Direct targeting of sEH with alisol B alleviated the apoptosis, inflammation, and oxidative stress in cisplatin-induced acute kidney injury. Int. J. Biol. Sci. 19, 294–310. doi:10.7150/ijbs.78097
Zhang, P., Zhang, X., Zhang, J., Song, Y., Liu, T., Zeng, Z., et al. (2021d). Novel nanoliposomes alleviate contrast-induced nephropathy by mediating apoptosis response in New Zealand rabbits. Front. Mol. Biosci. 8, 681849. doi:10.3389/fmolb.2021.681849
Zhang, Q., Lin, S., Wang, L., Peng, S., Tian, T., Li, S., et al. (2021e). Tetrahedral framework nucleic acids act as antioxidants in acute kidney injury treatment. Chem. Eng. J. 413, 127426. doi:10.1016/j.cej.2020.127426
Zhang, S., Sun, H., Kong, W., and Zhang, B. (2020b). Functional role of microRNA-500a-3P-loaded liposomes in the treatment of cisplatin-induced AKI. IET Nanobiotechnol 14, 465–469. doi:10.1049/iet-nbt.2019.0247
Zhang, W., Dai, X., Jin, X., Huang, M., Shan, J., Chen, X., et al. (2023b). Promotion of wound healing by a thermosensitive and sprayable hydrogel with nanozyme activity and anti-inflammatory properties. Smart Mat. Med. 4, 134–145. doi:10.1016/j.smaim.2022.08.004
Zhao, M., Wang, Y., Li, L., Liu, S., Wang, C., Yuan, Y., et al. (2021a). Mitochondrial ROS promote mitochondrial dysfunction and inflammation in ischemic acute kidney injury by disrupting TFAM-mediated mtDNA maintenance. Theranostics 11, 1845–1863. doi:10.7150/thno.50905
Zhao, M., Zhou, Y., Liu, S., Li, L., Chen, Y., Cheng, J., et al. (2018). Control release of mitochondria-targeted antioxidant by injectable self-assembling peptide hydrogel ameliorated persistent mitochondrial dysfunction and inflammation after acute kidney injury. Drug Deliv. 25, 546–554. doi:10.1080/10717544.2018.1440445
Keywords: acute kideny injury, anti-inflammtory, oxidative stress, ROS scavenging, nanosystem
Citation: Wang Y, Jiang H, Zhang L, Yao P, Wang S and Yang Q (2023) Nanosystems for oxidative stress regulation in the anti-inflammatory therapy of acute kidney injury. Front. Bioeng. Biotechnol. 11:1120148. doi: 10.3389/fbioe.2023.1120148
Received: 09 December 2022; Accepted: 30 January 2023;
Published: 09 February 2023.
Edited by:
Junjie Li, Kyushu University, JapanReviewed by:
Panyue Wen, University of Science and Technology of China, ChinaXianwen Wang, Anhui Medical University, China
Copyright © 2023 Wang, Jiang, Zhang, Yao, Wang and Yang. This is an open-access article distributed under the terms of the Creative Commons Attribution License (CC BY). The use, distribution or reproduction in other forums is permitted, provided the original author(s) and the copyright owner(s) are credited and that the original publication in this journal is cited, in accordance with accepted academic practice. No use, distribution or reproduction is permitted which does not comply with these terms.
*Correspondence: Shaoqing Wang, d293b3Nhc2EyMDAzQDE2My5jb20=; Qian Yang, eW95b3lvdW5nODI5M0BnbWFpbC5jb20=
†These authors have contributed equally to this work.