- Department of Urology, Affiliated Hospital of Jiangsu University, Zhenjiang, China
The development of nanotechnology and nanomaterials has provided insights into the treatment of urinary system tumors. Nanoparticles can be used as sensitizers or carriers to transport drugs. Some nanoparticles have intrinsic therapeutic effects on tumor cells. Poor patient prognosis and highly drug-resistant malignant urinary tumors are worrisome to clinicians. The application of nanomaterials and the associated technology against urinary system tumors offers the possibility of improving treatment. At present, many achievements have been made in the application of nanomaterials against urinary system tumors. This review summarizes the latest research on nanomaterials in the diagnosis and treatment of urinary system tumors and provides novel ideas for future research on nanotechnologies in this field.
1 Introduction
The development of nanotechnology has brought new breakthroughs in the diagnosis and treatment of cancer (Wakaskar, 2018; Chaturvedi et al., 2019; Khan et al., 2020). Nanoparticles are usually loaded with therapeutic drugs, proteins, photothermal agents, imaging agents or immune molecules (Liu et al., 2018). Nanoparticles are modified on demand, and those with recognizable ligands are easily taken up by target cells after receptor‒ligand interactions (Song et al., 2017). Nanoparticles are powerful drug carriers and can increase drug uptake by tumors by prolonging drug circulation and reducing the risk of adverse toxic effects on nearby healthy tissue (Ahmed et al., 2012).
Urinary system tumors are common malignant tumors in humans with gradually increasing incidence (Siegel et al., 2019). The treatment of urinary system tumors is diverse. For example, chemotherapy and radiotherapy are the main treatment strategies for advanced renal cancer. However, drug resistance is very common (Penticuff and Kyprianou, 2015). Nanotechnology can be used to enhance the therapeutic effects of cancer drugs (Jaishree and Gupta, 2012). Although targeted therapy and immunotherapy are widely used, resistance to these modalities is inevitable, and the development of nanomaterials provides an alternative direction. Moreover, traditional treatment methods, such as surgery, radiotherapy and endocrine therapy, are facing great challenges. The development of nanomaterials provides novel ideas for the treatment of urinary tract tumors. Thus, this review summarizes the applications of several nanomaterials commonly used to treat urinary tract tumors.
2 Mesoporous silica nanoparticles (MSNs)
MSNs have well-defined mesoporous structures with diameters ranging from 2 to 10 nm, large pore volumes of 0.6–1 cm3/g and high surface areas of 700–1,000 m2/g (Iturrioz-Rodriguez et al., 2019). MSNs have certain characteristics, such as a uniform structure, large surface area, and modifiable pore size, and work as a suitable repository for loading therapeutic/diagnostic agents. MSNs protect their cargo from premature release and subsequent undesirable degradation in the stomach and intestine until they reach the target location (He and Shi, 2014; Nawaz et al., 2018). MSNs in the size range of 50–300 nm can promote endocytosis in living animal and plant cells without producing any significant cytotoxicity. In drug delivery and cancer treatment, the particle size of nanocarriers must be less than 100 nm to circulate in blood vessels for a long time and serve as a passive drug delivery carriers based on enhanced permeability and retention (EPR) effects. On the other hand, the pore sizes of MSNs can be adjusted from 2 to 6 nm, which allows the loading of different drug molecules. Therefore, MSNs have high drug loading and packaging efficiency (Porrang et al., 2022).
2.1 Application of MSNs in PCa
MSNs can be used in the treatment of PCa. Many researchers have used MSNs as carriers to load therapeutic drugs, and MSNs can deliver these drugs to tumor cells. For example, the study of Zanib Chaudhary et al. investigated resveratrol (RES), a polyphenol with antitumor properties. However, its poor pharmacokinetics and stability and low solubility limit its clinical application. Loading RES onto MSNs can significantly improve the efficacy of RES in PCa (Chaudhary et al., 2019). Fenbendazole (FBZ) is a potential anticancer drug whose application is limited by its low water solubility. The water solubility of FBZ can be increased when serialized on β-lactoglobulin-modified MSNs, which can increase the cytotoxicity of FBZ and inhibit the migration of PCa cells (Koohi et al., 2022). (Figures 1A,B) It is also possible to serialize FBZ on pegylated MSN (MCM-41) to improve its cytotoxicity and increase its delivery to PCa cells (Esfahani et al., 2021).
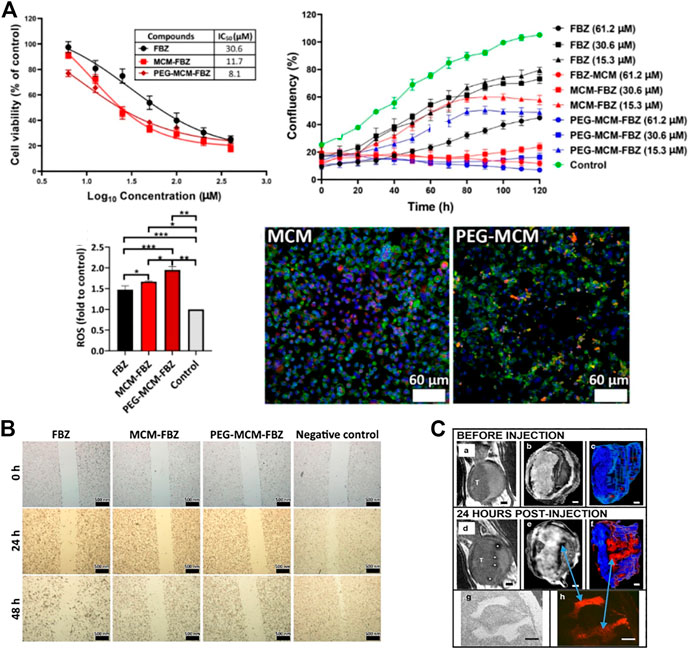
FIGURE 1. Synthesis and applications of mesoporous silica nanoparticles (MSNs). (A) The cytotoxicity of FBZ increased when it was bound the nanomaterial. Moreover, the inhibition of prostate cancer (PCa) cell proliferation was significantly increased. The intracellular ROS contents were also increased, thus inhibiting the proliferation of tumor cells. Fluorescence experiments showed that the FBZ complexes, especially the PEG-MCM-FBZ complex, increased the uptake of FBZ. (B) The complex effectively inhibits the migration of PCa cells. (C) Bladder tumor cells absorbed PEG-Gd2O3-TRITC-MSN particles with higher affinity than normal bladder epithelial cells and can be used for MRI T2 scanning.
Studies have found that functionalized MSNs themselves can also inhibit tumors. Walterinnesia aegyptia venom (WEV) serialized on MSNs is more effective than WEV alone. Researchers have also demonstrated that snake venom silica nanoparticles could alter the cell cycle in PCa cells and accelerate cell apoptosis. A study showed that the continuous delivery of nanoparticles carrying WEV is an effective treatment for PCa (Badr et al., 2013). pH-sensitive delivery systems can provide on-demand and selective drug release at acidic tumor sites. Polyacrylic acid (PAA)-functionalized MSNs (PAA-MSNs) can be used as an effective pH response template with great potential for effective controlled release in cancer therapy. PAA-MSNs also showed strong cellular uptake and a longer circulation time in vivo, and good results were obtained when they were linked to bicalutamide for the treatment of PCa (Saroj and Rajput, 2019). Researchers also linked etoposide (ETS) to PAA-MSNs, which greatly increased the cytotoxicity of ETS (Saroj and Rajput, 2018). Moreover, when calcium peroxide (CaO2) was loaded into PAA-MSNs, free CaO2 without antitumor effects can release reactive oxygen species (ROS) in response to the acidic microenvironment. This induces mitochondria-mediated apoptosis through significant oxidative stress, minimizes damage to normal tissues, and exerts excellent antitumor effects. This system represents a new way to treat PCa (Wu et al., 2020).
MSNs can also be used to diagnose PCa. Researchers developed an electrochemical immunosensor based on MSNs (Wang et al., 2013). Other researchers designed MSNs containing pH indicator molecules for PCa detection, which can improve the sensitivity of detecting prostate-specific antigen (PSA) (Shao et al., 2018). PSA-targeted manganese-oxide MSNs were found to be useful for detecting PCa, as they can accumulate in PCa cells but not in non-cancerous cells. PCa can be visualized using PSA-targeted fluorescence and Magnetic Resonance (MR) dual-function nanoparticles. They can be used as an MR contrast agent (Du et al., 2020). It is generally harder to detect low molecular weight biomarkers in vivo by traditional spectrometric methods, as they often exist in low concentrations and are covered by many other proteins. In fact, studies have shown that less than 25% of PCa cases with increased PSA levels were diagnosed appropriately.
In addition, a second biopsy can diagnose PCa in approximately 30% of patients with a previously benign biopsy result. Researchers have designed diagnostic systems for PCa. These use mercaptan MSN (MSN-SH) to detect low molecular weight proteins and distinguish between patients with benign prostatic hyperplasia and those with PCa with elevated PSA levels (Vidaurre-Agut et al., 2019).
2.2 Application of MSNs in bladder cancer
Researchers have linked targeted doxorubicin (DOX) to MSNs and the peptide conjugate CSNRDARRC (DOX-loaded MSNs@PDA-PEP) to form a complex. Further study suggested that the complex significantly improved cell uptake efficiency compared with DOX alone (Wei et al., 2017). Researchers invented a compound based on MSNs and found that 70% of bladder cancer cells were labeled by nanoparticles. The tumor contrast was enhanced by the nanoparticles, which could be used for tumor staging, treatment monitoring and drug delivery (Sweeney et al., 2016). At present, there are many studies on microRNAs, indicating that these RNAs have antitumor effects. Researchers have reported combinations of microRNA and MSNs as delivery agents. The uptake of these multifunctional nanoparticles by bladder cancer cells overexpressing EGFR was enhanced via receptor-mediated cellular internalization (Haddick et al., 2020). Moreover, modified MSNs were constructed and used as delivery carriers to deliver both miRNA-34a and si-PD-L1 to bladder cancer cells. Notably, the nanoparticles were biocompatible, which protects the compound from degradation. Therefore, this system could be used as a treatment for bladder cancers (Shahidi et al., 2022).
2.3 Application of MSNs in renal cancer
Lonidamine (LND), a heat-sensitive inhibitor of mitochondrial metabolism, was used in combination with photothermal-polydopamine (PDA) in the treatment of renal cell carcinoma (RCC). LND and PDA were loaded into MSNs with a star shape. The results indicated that the complex had excellent tumor targeting ability. LND and PDA combined with laser treatment enhanced the antiproliferation and anticancer abilities with good biocompatibility (Chen et al., 2021).
3 Gold nanoparticles (AuNPs)
Gold nanoparticles (AuNPs) have attracted great attention in biomedicine (Pissuwan et al., 2011; Dykman and Khlebtsov, 2012). AuNPs have large surfaces and different properties and are of great potential in diagnosis, delivery, photothermal and radiation therapy, enzyme fixation, and cell imaging (Zhang et al., 2010; Li et al., 2011; Nimesh et al., 2011; Pissuwan et al., 2011). AuNPs come in very diverse shapes, although spherical nanoparticles are thought to be the main type. Depending on the production method, AuNPs can be made in different forms: triangles, hexagons, octahedrons, cells, nanospheres, pores, stars, and nanorods (Ovais et al., 2017). The different form determines what the AuNP carries (Boisselier and Astruc, 2009). AuNPs can interact with biological fluids, and the nanoparticles acquire a biological component known as a protein corona (PC) (Maiorano et al., 2010; Corbo et al., 2016; Rampado et al., 2020). Thus, AuNPs can be widely used in the medical field.
3.1 Application of AuNPs in PCa
AuNPs can be used to detect PCa. Researchers designed a sensitive electrochemical immunosensor for the determination of PSA. AuNP/aminothiol-functionalized graphene-oxide composites were constructed and modified before they were used as an immunosensor platform to elevate the amount of PSA antibody 1 (Ab1) for PSA determination (Medetalibeyoglu et al., 2020). Studies have detected PCA3, a potential urinary biomarker, in urine using AuNPs (Htoo et al., 2019). Based on supramolecular hydrogel-AuNP spheres, an imaging biosensor with high sensitivity and specificity was developed for the determination of exosomes derived from PCa cells (Chen et al., 2020). The gold nanoprobes consisted of a specific peptide, and the AuNPs were used for the determination of PSA (Li et al., 2019a). Studies evaluated AuNPs in vitro and in vivo and found that these nanoparticles showed good potential features for cancer cell uptake and biodistribution and could also be used to detect PCa by fluorescence imaging (Pretze et al., 2018). (Figure 2A)
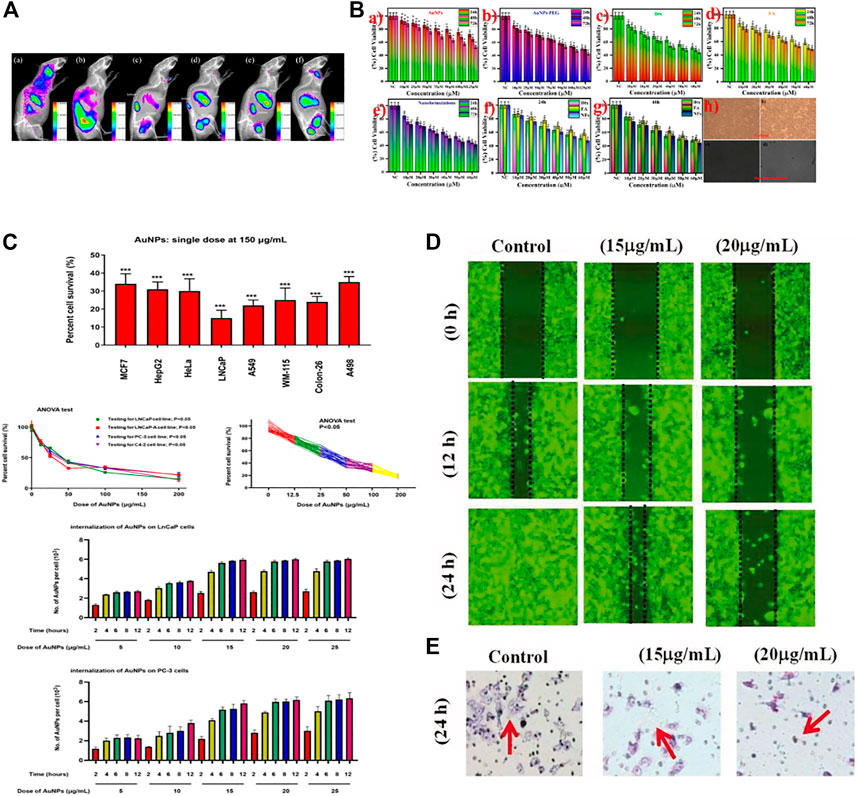
FIGURE 2. Applications of gold nanoparticles (AuNPs) in cancer. (A) PCA cells were treated with ultrafine AuNPs containing the gastrin-releasing peptide receptor. After 72 h, up to 6% of the AuNPs remained in the body, which could be used to detect PCa. (B) Docetaxel-coated AuNPs showed toxicity to PCa cells in vitro. (C) AuNPs were found to be toxic to eight types of tumor cells, most notably to PCa cells, showing toxic effects to all PCa cells tested. (D) AuNPs synthesized from abies fir plant extracts inhibited bladder cancer T24 cell migration in a concentration-dependent manner. (E) AuNPs mediated T24 cell death.
The application of AuNPs in the treatment of PCa is also very extensive. AuNPs can be used as carriers to deliver antitumor drugs to PCa cells. Researchers synthesized novel AuNPs conjugated with abiraterone (AuNPs-AB), which have shown good prospects for the treatment of PCa (Stolarczyk et al., 2020). Docetaxel (Dtx) was encapsulated by AuNPs for delivery to PCa cells (Thambiraj et al., 2021). A synthetic Gen-AuNP conjugate (Gen@AuNPs) selectively inhibited the proliferation of PCa cells. The authors also demonstrated the stability and bioactivity of the AuNPs along with their low toxicity to normal cells (Vodnik et al., 2021). Phosphatidylserine (PS) is an essential lipid that mediates macrophage exocytosis and is dysregulated in tumors. Biomimetic phosphatidylserine-coated AuNPs (PS-AuNPs) were synthesized, and their potential in PCa was investigated in vitro. After evaluating histone-associated DNA fragments as a marker of apoptosis, the researchers found that DNA fragmentation was significantly increased after PS-AuNP treatment compared with control treatment. Therefore, PS-AuNPs were indicated as a potential PCa treatment (Radaic et al., 2021).
AuNPs themselves can also be therapeutic for PCa. AuNPs have been shown to exert powerful cytotoxic effects, with synthetic AuNPs showing the most significant efficacy against PCa, whether or not the tumor cells were androgen-dependent. Further studies on the molecular mechanism showed that AuNPs can trigger the secretion of anticancer factors and myeloid cell-polarizing factors from tumor cells through MMP9 inhibition, thereby achieving antitumor effects (Hao et al., 2021). (Figure 2C) The same AuNPs decay at a high energy level and their radiation can be delivered to the tumor site without destroying the normal tissues or organs. Using AuNPs is a promising way to treat PCa (Al-Yasiri et al., 2019). AuNPs have also been widely used to treat PCa due to their unique optical properties. A silicon dioxide-coated AuNP cluster was proposed to address the therapeutic limitations of individual AuNPs and utilize their photothermal effects to treat typical PCa PC-3 cells. AuNP clusters have shown excellent therapeutic effects in photothermal tests under near-infrared radiation (Kim et al., 2020).
The combination of AuNPs and Gd(III) provides better cancer inhibition after radiotherapy. Precise tumor targeting by PSMA-1-modified AuNPs can achieve precise radiotherapy, reduce the irradiation dose, and minimize side effects (Luo et al., 2020). AuNPs are also effective radiosensitizers, and RALA (a short amphiphilic peptide)/AuNPs were synthesized, providing functional evidence of RALA-AuNP nuclear accumulation. RALA-AuNP produced meaningful radiosensitization using low microgram AuNP treatment concentrations (Bennie et al., 2021).
3.2 Application of AuNPs in RCC
Few studies of AuNPs in RCC have been reported. Researchers have found that 200 nm AuNPs have an antitumor effect in 786-O RCC cells by promoting cell apoptosis and inhibiting proliferation (Zhao et al., 2020). C. wenyujin is the main component of turmeric and has antioxidant, antiproliferative and antitumor properties. Researchers used C. wenyujin to synthesize AuNPs and found that the produced CW-AuNPs could activate the proapoptotic proteins caspase-3, caspase-9, Bid and Bad in renal cancer A498 cells in vitro. CW-AuNPs can reduce the level of Bcl-2 and thereby induce apoptosis of A498 cells (Liu et al., 2019). Other studies have found that AuNP treatment reduces the viability of cancer cells, suggesting a potential alternative to nephrectomy (Nikzad et al., 2017).
3.3 Application of AuNPs in bladder cancer
Hyaluronidase (HAase) is a bladder cancer biomarker found in urine. HAase can prevent the redshift observed when cationic gold nanoparticles (CTAB) aggregate with other substances. Therefore, this color change can be used for the diagnosis of bladder cancer (Nossier et al., 2014). Other researchers considered urine hepatocellular carcinoma upregulated RNA (HURP) as a biomarker of bladder cancer and developed a AuNP assay to directly detect unamplified HURP RNA for bladder cancer diagnosis (Eissa et al., 2014).
AuNPs can also be used in the treatment of bladder cancer. Researchers found that by combining fir extract with AuNPs, apoptosis of tumor cells could be promoted to play an anticancer role (Wu et al., 2019). (Figures 2D,E) Because AuNPs are effective enhancers of radiotherapy, some researchers improved the control of locally advanced bladder cancer. They used AuNPs to locate bladder tumors and found that the AuNPs were distributed throughout the bladder wall. However, most AuNPs are related to extracellular keratins, and their localization in the tumor stroma contributes to their specific radiotherapy enhancement for muscle-invasive bladder cancer (Smilowitz et al., 2017). AuNPs themselves also have therapeutic effects. Researchers have studied the cancer suppression effects of AuNPs in 5637 bladder cancer cells exposed to different concentrations of AuNPs for 24 h. The results showed that the AuNPs could reduce the survival of 5637 cells in a dose-dependent manner. In addition, ROS production was significantly increased in cells treated with 25 and 50 μg/mL AuNPs. These AuNPs could promote the apoptosis of tumor cells by inducing Bax overexpression and downregulating Bcl-2 (Daei et al., 2022).
4 Quantum dots (QDs)
Quantum dots (QDs) are nanomaterials with diameters of 2–10 nm (Sukhanova et al., 2018). QDs have unique physical and chemical properties, including high stability. Moreover, most QDs are considered non-toxic, so they are widely used in various fields of medicine (Rajendiran et al., 2019). QDs are potential smart drug delivery tools that can be used in cancer therapy, and their photostability, tunable emission, and wide excitation range make them more effective fluorescent markers than organic dyes in biological applications (Breus et al., 2015; Manshian et al., 2017; Hossen et al., 2019). (Figure 3A)
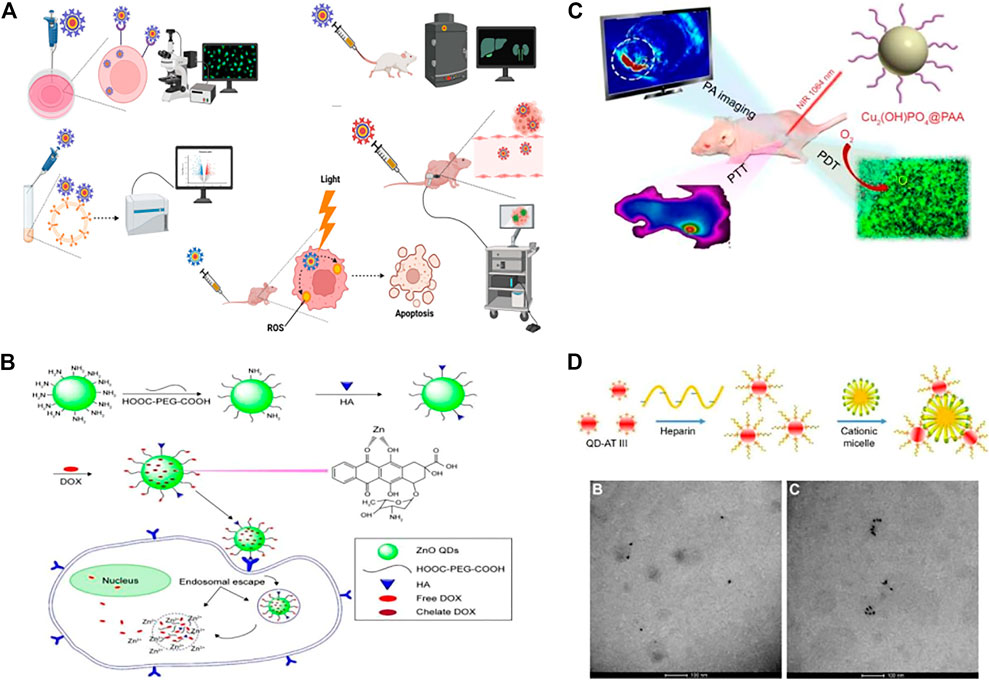
FIGURE 3. Biomedical applications of quantum dots (QDs). (A) QDs were used for intracellular imaging, in vivo imaging, fluorescence-activated cell sorting, photodynamic therapy, and traceable drug delivery. (B) QDs were used for drug delivery, such as the hyaluronic acid-ZnO QD-polyethylene glycol (HA-ZnO-PEG) material. This drug delivery system can release DOX (doxorubicin) in acidic cells. (C) QDs can be used for imaging and enable a combination of photodynamic therapy and photothermal therapy as well as photoacoustic imaging. (D) QDs have been used in sensors. TEM images of QDs-AT III-heparin without CTAB can be easily distinguished from TEM images of QD aggregates with CTAB after quantum dot synthesis and polymerization.
4.1 Application of QDs in PCa
Researchers synthesized highly stable AgInSe/ZnS QDs that can selectively target PCa and be taken up in large amounts. Therefore, AgInSe/ZnS QDs can be used as imaging probes to guide diagnosis and treatment (Ncapayi et al., 2021). (Figures 3B–D) Researchers used yeast cells and Saccharomyces cerevisiae to synthesize cadmium telluride (CdTe) QDs in modified Czapek medium through biological methods. In vitro experiments showed that the CdTe QDs induced a significant increase in ROS levels in PC-3 cells. Moreover, CdTe also arrested PC-3 cell growth in the G2/M phase of the cell cycle. Thus, CdTe QDs induced cell death and nuclear apoptosis in a dose-dependent manner (Jigyasu et al., 2020). Nanocomplexes containing QDs and β-cyclodextrin have been synthesized. This nanoconjugate has folate-targeting properties and has been used to deliver the anticancer Compound C-2028 to PCa cells. The QDs enter the cell through multiple endocytic pathways with various efficiencies to deliver the drug to cancer cells (Pilch et al., 2022). Researchers designed and synthesized a nanoparticle probe PSMA receptor-targeted QD (PSMA-QD655) by combining functionalized amino-PEG QDs with DUPA-targeted polypeptide constructs through heterobifunctional joints. PSMA-QD655 is a near-infrared imaging agent that can be used for navigation during surgery (Asha et al., 2021). Through the electrostatic adsorption of a large number of QDs on superparamagnetic Fe3O4, researchers prepared red and green magnetic QD nanobeads (MQBs) with excellent magnetic and high luminescence properties. MQBs can be used as multifunctional probes for the simultaneous determination of free and total PSA (Rong et al., 2019).
4.2 Application of QDs in bladder cancer
QDs have also been widely used in bladder cancer. Researchers prepared QD fluorescent probes conjured with prostate stem cell antigen (PSCA) monoclonal antibody (QD-PSCA). The probe specifically identifies PSCA expressed in bladder cancer cells. The stable fluorescence of the probe can be used as a specific marker (Yuan et al., 2018). A nanocarrier system was constructed with Mn:ZnS QDs. This delivery system can improve drug delivery efficacy in bladder cancer (Manan et al., 2021). The molecular detection of malignant nuclear matrix protein 22 (NMP22) in the urine has been introduced into clinical practice to identify bladder tumors. Researchers have also designed two-color QDs that can detect NMP22 with high sensitivity (Othman et al., 2020).
4.3 Application of QDs in RCC
Black phosphorus QDs (BP-QDs) can enhance the apoptosis of RCC cells after ionizing radiation (IR), indicating that BP-QDs have potential application value in the RCC radiosensitization therapy (Lang et al., 2022). Researchers have measured the expression of the proteins Tiam1 and Rac1 in RCC using immunohistochemistry (IHC) and QD labeling methods. It was found that the expression levels of Tiam1 and Rac1 are related to the differentiation, staging and lymphatic metastasis of RCC, suggesting that they are critical in RCC invasion and metastasis (Shan et al., 2017).
5 Carbon nanotubes (CNTs)
Carbon nanotubes (CNTs) are sheets of graphene 1 nm in diameter and a few microns in length. Single-walled CNTs (SWCNTs) and multiwalled CNTs (MWCNTs) (Elhissi et al., 2012) are the two common types of CNTs. Functionalized CNTs have the ability to cross the cell membrane, which allows functionalized CNTs to target specific tumor cells through endocytosis (Lacerda et al., 2012). (Figure 4A) Modified CNTs can be used to evaluate treatment, cell survival and apoptosis (Rastogi et al., 2014).
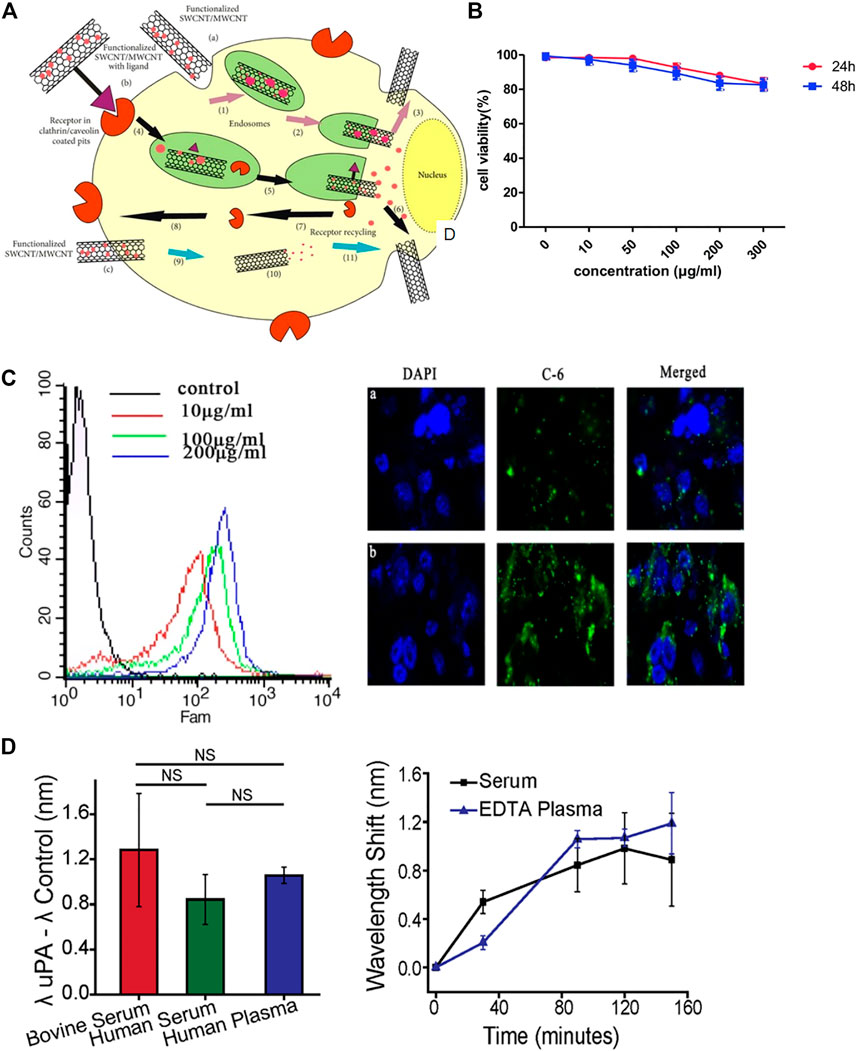
FIGURE 4. Biological applications of carbon nanotubes (CNTs). (A) CNTs penetrate into cells through various ways, including non-receptor-mediated endocytosis, receptor-mediated endocytosis and endocytosis-independent methods. (B) Flow cytometry and immunofluorescence assays indicated the uptake of CNT-PEG. (C) Cytotoxicity of CNT-PEG uPA at different concentrations. (D) Sensor detection of uPA in human blood products.
5.1 Application of CNTs in PCa
A nanoparticle delivery system including MWCNTs conjugated with the polypeptide H3R6 has been discovered for PCa immunotherapy in which the immunotherapeutic agents can be efficiently delivered to the prostate and lymph nodes (Xia et al., 2018). A novel nanoultrasound contrast agent based on MWCNTs was reported. Compared with traditional contrast materials, this contrast agent provides better visibility and accuracy and can target PCa cells more effectively (Gu et al., 2018). (Figures 4B,C) Researchers designed an optical sensor based on the fluorescence characteristics of SWCNTs. These sensors are expected to be used to distinguish between invasive PCa and inert prostate cells and reduce excessive treatment options for this disease (Williams et al., 2018). (Figures 4D,E)
5.2 Application of CNTs in bladder cancer
Bladder tumor-specific SWCNTs are delivered to the bladder at a low dose. Then, the nanotubes are heated with 360° NIR light for 30 s. NIR light can heat the tumor while protecting the healthy bladder wall (Virani et al., 2018). Epirubicin (EPI) was loaded on magnetic MWCNTs (MMWCNTs-EPI) for intravesical infusion. The MMWCNT-EPI system has high efficiency in enhancing cytotoxicity and inhibiting proliferation in vitro and in vivo, showing potential clinical application value (Suo et al., 2019).
6 Liposomes
Liposomes are spherical structures with sizes ranging from 25 nm to 2.5 μm (Akbarzadeh et al., 2013) Liposomes can be structured as monolayer (ULV) or multilayer (MLV). ULVs are suitable for the encapsulation of hydrophilic drugs or antigens. MLVs feature multiple lipid bilayers, which act as barriers to resist enzymes, certain pH conditions, and free radicals in vivo. Thus, liposomes prevent drug degradation until its release at target cells, organs, or systems. Due to their high biocompatibility, degradability, low toxicity, and ability to encapsulate hydrophilic and hydrophobic compounds, liposomes represent the most successful drug delivery system because of their high biocompatibility, degradability and low toxicity (Felice et al., 2014; Bozzuto and Molinari, 2015). (Figures 5A,B)
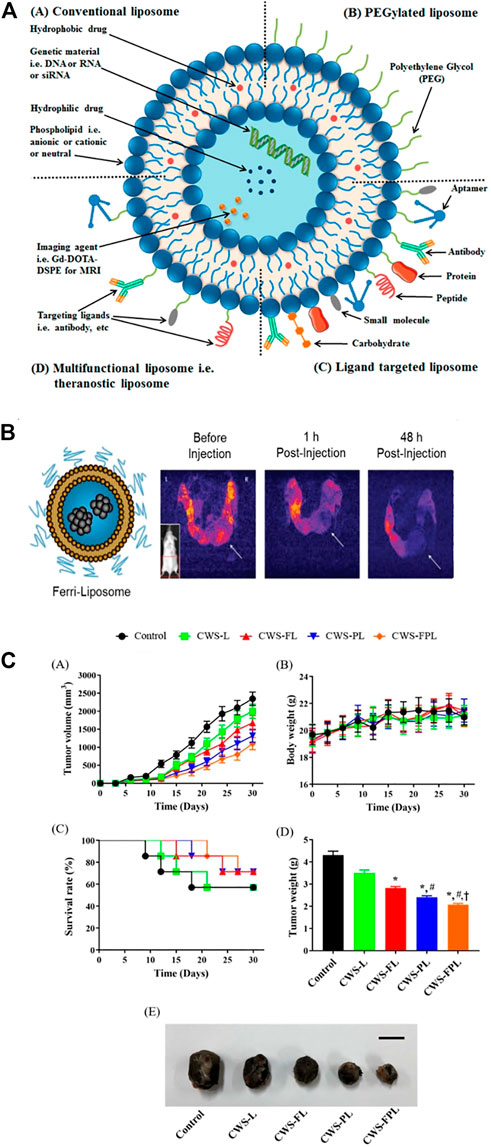
FIGURE 5. Liposomes have been used for cancer diagnosis and therapy. (A) A specific targeting ligand was incorporated to modify the surface of PEGylated liposomes. (B) T2-weighted MR images of a transgenic mouse before and after injection of Ferri-liposomes. (C) An in vivo study indicated that various liposomal formulations inhibited tumor growth.
6.1 Application of liposomes in PCa
The considerable side effects of the anthracycline doxorubicin (DOX) are major drawbacks. Simvastatin (Sim) can be used in combination with DOX. Researchers combined DOX and Sim on herceptin-conjugated liposomes. Studies have shown that liposomes can target PCa cells and exert an antitumor effect (Li et al., 2019b). Antisense oligonucleotides (ASOs) have a significant advantage due to their ability to provide virtually unlimited targeting of any gene. However, ASOs have the disadvantage of poor delivery in vivo. Researchers used the penetrating peptide iRGD to functionalize liposomes as anti-androgen receptor (AR) vectors. IRGD/liposomes significantly elevated the concentration of AR/ASO in target tissues and therefore inhibited tumor growth (Guan et al., 2021).
Liposomes increase the solubility the complexes, enhance the cytotoxicity of PCa drugs, and significantly reduce the growth rate of PCa cells, resulting in high radiotherapy efficacy in patients with locally advanced PCa (Silva et al., 2021). PSMA is a marker used for the diagnosis of advanced PCa and is recognized for targeted drug delivery. A liposome composed of a PSMA ligand and polyethylene glycol was synthesized. Liposomes with small PSMA binding motifs can be used to specifically identify PSMA + PCa cells (Yari et al., 2019).
6.2 Application of liposomes in bladder cancer
The BCG cell wall skeleton (BCG/CWS) was encapsulated in liposomes. Specific ligands were then conjugated on the surface of the liposomes to facilitate targeting and cell penetration. The modified liposomes significantly elevated the endocytosis of BCG/CWS by bladder cancer cells and strengthened the antitumor effect. Thus, BCG/CWS-modified liposomes could be a potential, highly efficacious therapeutic candidate in bladder cancer. (Figures 5C,D). The accumulation and distribution of DOX within liposomes in the bladder are significantly higher than those of intravenously administered DOX (Mikhail et al., 2017). Researchers developed a cationic liposome that combines three subclasses of mycolic acid (MA). This liposome can be effectively absorbed by MB49 bladder cancer cells and then induce antitumor immunity in vivo (Yoshino et al., 2019).
6.3 Application of liposomes in RCC
A liposome specifically targeting tumors was synthesized with phospholipids and cholesterol and showed excellent tumor-specific uptake as well as tumor inhibition (Pal et al., 2019).
7 Magnetic nanoparticles (MNPs)
Magnetic nanoparticles are an important class of nanoparticles that are usually made of pure metals (Fe, Co., and Ni) or mixtures of metals and polymers. The utilization of MNPs has increased in hyperthermic cancer treatment, controlled drug release, magnetic resonance imaging, and biosensing. A key advantage of MNPs is their ability to be magnetically manipulated by external magnetic fields (Farzin et al., 2020). MNPs can alter drug pharmacokinetics to reduce cytotoxicity and increase the drug release rate. In addition to the possibility of localizing MNPs to cancer cells by the application of magnetic fields, MNPs can be modified with high-affinity ligands. Additionally, MNPs can be applied to improve the MRI image contrast in the target tissue. MNPs can be localized to tissue sites to increase proton relaxation and improve visibility (Sun et al., 2008).
7.1 Application of MNPs in PCa
Magnetic hyperthermia (MHT) is a promising treatment for solid tumors. MHT generates heat in the presence of an alternating magnetic field (AMF). Researchers found new magnetic nanoparticles with enhanced heating efficiency that generate ideal intratumoral temperatures when exposed to an AMF. In vivo experiments confirmed that the nanoclusters significantly accumulate in tumor sites within hours and elevate the temperature to more than 42°C after exposure to an AMF. Finally, MHT application showed great inhibition of PCa cell growth with no side effects (Albarqi et al., 2020) (Figure 6). Galbanic acid, a natural sesquiterpenoid coumarin compound, causes significant toxicity to LNCaP PCa cells. Galbanic acid was loaded onto Fe3O4-coated MNPs and showed significant toxicity to all PCa cells (Mohtashami et al., 2019) Researchers also developed multifunctional MSNs that bind photosensitizers. These MSNs demonstrated significant anticancer effects by inducing apoptosis in PCa cells (Choi et al., 2018).
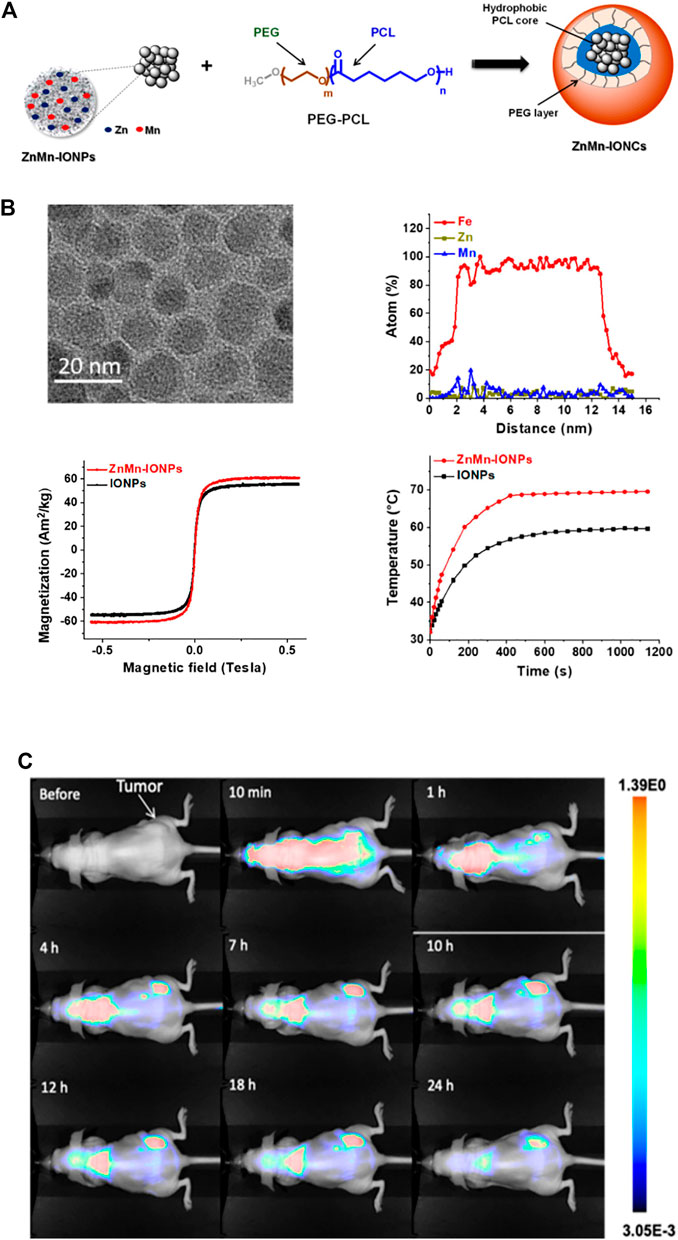
FIGURE 6. Biological applications of magnetic nanoparticles (MNPs). (A) Iron oxide nanoparticles with Zn and Mn were encapsulated into a nanocarrier. (B) Enhanced heating efficiency was achieved upon exposure to an alternating magnetic field. (C) In vivo evaluation of the nanoparticles suggested that ZnMn-IONCs started to accumulate in tumors. The fluorescence intensity in the tumors was higher than that the liver, kidneys, spleen and lungs.
8 Discussion
The development of nanotechnology and nanomaterials for the diagnosis and treatment of urinary system tumors has brought great achievements and opportunities (Table 1). Many of these studies are still in the experimental stage, and many of these concepts have not reached clinical application. There have been a few reports on nanotechnology and nanomaterials in bladder cancer and kidney cancer; however, we are far from fully understanding these technologies. We believe that through the efforts of researchers and clinicians, more diagnosis and treatment methods will be invented for patients with urinary tract tumors.
Despite the therapeutic benefits, problems of nanotechnology and nanomaterials in terms of biological safety as well as metabolism in vivo should be given sufficient attention. Nanomaterials vary in their distribution, which depends on their shape, size, porosity, surface functionalization, etc. (He et al., 2011). For instance, MSNs are mainly present in the liver and spleen (Fu et al., 2013). AuNPs are primarily concentrated in the liver, spleen and bone marrow (Blanco et al., 2015). CNTs are different from other nanomaterials. They enter the cells in two ways, through endocytosis and passive diffusion, while QDs are mainly distributed and accumulate in the reticuloendothelial system and kidney. These differences in distribution result in various metabolic fates, applications and toxicities. As far as metabolism is concerned, first, the clearance of nanomaterials, including MSNs and AuNPs, depends on clearance by the kidney and biliary tract. Second, nanomaterial metabolism depends on their size. Small AuNPs (less than 10 nm) are easier to metabolize than large AuNPs (Zhou et al., 2011). QDs with larger particle sizes remain unchanged in vivo for a longer time and are more difficult to remove. In addition, nanomaterials can combine with specific proteins and be found in systemic circulation. Thus, these nanomaterials can be absorbed by phagocytic cells in the liver and spleen, such as liver Kupffer cells and splenic B Cells. Finally, the nanomaterials can be excreted from the body after phagocytosis by the macrophages in the alveoli (Huang et al., 2011). In addition to their dosage, the particle size of nanomaterials has a great impact on their toxicity (Greish et al., 2012; Shao et al., 2017). Moreover, the nanoparticle surface properties are the most important factor that affects toxicity. Nanoparticles with positive charges are more likely to induce an immune response and cytotoxicity than neutral or negatively charged nanoparticles (Pisani et al., 2017; Li et al., 2019c). The cytotoxicity of nanoparticles is also considered to be shape-dependent (Lopez-Chaves et al., 2018) and depends on the biomolecules present on their surface (Xie et al., 2017).
Many problems with nanoparticles remain to be solved before these new developments can be applied in clinical settings. First, how can we improve the targeting efficiency of nanoparticles? Higher efficiency indicates that lower doses can be used with less toxicity. Second, we need to try to reduce particle loss in the targeting process, which is another way to increase efficiency and reduce toxicity. Third, and one of the most important issues, is how to reduce the side effects caused by nanoparticles, which usually lead to impairment of the kidney and liver. Fourth, we should also pay increasing attention to simplifying the manufacturing of nanoparticles so that their cost could be lower for wide use in the clinic. Finally, the waste from the production of these nanoparticles may pollute the environment, and we should thus optimize the production process to reduce this threat.
Furthermore, more clinical studies need to be conducted based on the issues mentioned above. For example, it is still unknown whether patient characteristics (e.g., age, sex, tumor type, tumor location, previous treatment) will alter the effects of some nanoparticles. In addition, it is also unknown whether nanoparticles can induce acute exacerbation of other diseases, such as asthma and diabetes. Third, nanoparticle application requires a complete set of platforms, which report the intake, distribution and metabolism of the drug in the body. Finally, most of the current research is focused on the design, production and targeting of nanoparticles to treat tumors. We believe that patients could benefit more from novel clinical research, including nanoparticle localization, which is also crucial in tumor therapy, for continuous anticancer effects.
At present, we are only beginning to study nanoparticles, most of which have not been put into clinical application. More clinical studies will have to be done to assure safety and effectiveness. Although many studies have shown the advantages of nanoparticles in the diagnosis and treatment of tumors, their potential biosafety and toxicity should also be given more attention. More critical questions should also be answered, including whether the age, weight, basic disease status, etc. of patients influence the effects of nanoparticles. Therefore, there is a long way to go before these nanoparticles can be widely accepted and used. Moreover, we could focus on modifying nanoparticles, which augments their application and minimizes cell and organ toxicity. Finally, nanoparticles have mainly focused on PCa in terms of urinary tumors, and research in bladder cancer and kidney cancer is still insufficient. Further studies in these patients are also important and worthy of investigation. Thus, more efforts should be made to investigate the applications of nanoparticles, which we believe that we could lead to increasingly applicable and creative treatments for urinary tumors.
Author contributions
ZQ and YZ drafted the manuscript. JY and SG contributed to the figures. BC provided specific academic guidance and improvement for the important intellectual content of the manuscript.
Funding
This work was supported by the grants No. 81402100 from National Natural Science Foundation of China, No.BK20211123 from Natural Science Foundation of Jiangsu Province and No. SH2021033 from Social Development Foundation of Zhenjiang.
Conflict of interest
The authors declare that the research was conducted in the absence of any commercial or financial relationships that could be construed as a potential conflict of interest.
The handling editor GP declared a shared parent affiliation with the authors at the time of review.
Publisher’s note
All claims expressed in this article are solely those of the authors and do not necessarily represent those of their affiliated organizations, or those of the publisher, the editors and the reviewers. Any product that may be evaluated in this article, or claim that may be made by its manufacturer, is not guaranteed or endorsed by the publisher.
References
Ahmed, N., Fessi, H., and Elaissari, A. (2012). Theranostic applications of nanoparticles in cancer. Drug Discov. Today 17 (17-18), 928–934. doi:10.1016/j.drudis.2012.03.010
Akbarzadeh, A., Rezaei-Sadabady, R., Davaran, S., Joo, S. W., Zarghami, N., Hanifehpour, Y., et al. (2013). Liposome: Classification, preparation, and applications. Nanoscale Res. Lett. 8 (1), 102. doi:10.1186/1556-276x-8-102
Al-Yasiri, A. Y., White, N. E., Katti, K. V., and Loyalka, S. K. (2019). Estimation of tumor and local tissue dose in gold nanoparticles radiotherapy for prostate cancer. Rep. Pract. Oncol. Radiother. 24 (3), 288–293. doi:10.1016/j.rpor.2019.02.006
Albarqi, H. A., Demessie, A. A., Sabei, F. Y., Moses, A. S., Hansen, M. N., Dhagat, P., et al. (2020). Systemically delivered magnetic hyperthermia for prostate cancer treatment. Pharmaceutics 12 (11), 1020. doi:10.3390/pharmaceutics12111020
Asha, K. M., Yadav, K., Roach, P., and Chelvam, V. (2021). A targeted near-infrared nanoprobe for deep-tissue penetration and imaging of prostate cancer. Biomater. Sci. 9 (6), 2295–2312. doi:10.1039/d0bm01970d
Badr, G., Al-Sadoon, M. K., Rabah, D. M., and Sayed, D. (2013). Snake (Walterinnesia aegyptia) venom-loaded silica nanoparticles induce apoptosis and growth arrest in human prostate cancer cells. Apoptosis 18 (3), 300–314. doi:10.1007/s10495-012-0787-1
Bennie, L. A., Feng, J., Emmerson, C., Hyland, W. B., Matchett, K. B., McCarthy, H. O., et al. (2021). Formulating RALA/Au nanocomplexes to enhance nanoparticle internalisation efficiency, sensitising prostate tumour models to radiation treatment. J. Nanobiotechnology 19 (1), 279. doi:10.1186/s12951-021-01019-8
Blanco, E., Shen, H., and Ferrari, M. (2015). Principles of nanoparticle design for overcoming biological barriers to drug delivery. Nat. Biotechnol. 33 (9), 941–951. doi:10.1038/nbt.3330
Boisselier, E., and Astruc, D. (2009). Gold nanoparticles in nanomedicine: Preparations, imaging, diagnostics, therapies and toxicity. Chem. Soc. Rev. 38 (6), 1759–1782. doi:10.1039/b806051g
Bozzuto, G., and Molinari, A. (2015). Liposomes as nanomedical devices. Int. J. Nanomedicine 10, 975–999. doi:10.2147/IJN.S68861
Breus, V. V., Pietuch, A., Tarantola, M., Basche, T., and Janshoff, A. (2015). The effect of surface charge on nonspecific uptake and cytotoxicity of CdSe/ZnS core/shell quantum dots. Beilstein J. Nanotechnol. 6, 281–292. doi:10.3762/bjnano.6.26
Chaturvedi, V. K., Singh, A., Singh, V. K., and Singh, M. P. (2019). Cancer nanotechnology: A new revolution for cancer diagnosis and therapy. Curr. Drug Metab. 20 (6), 416–429. doi:10.2174/1389200219666180918111528
Chaudhary, Z., Subramaniam, S., Khan, G. M., Abeer, M. M., Qu, Z., Janjua, T., et al. (2019). Encapsulation and controlled release of resveratrol within functionalized mesoporous silica nanoparticles for prostate cancer therapy. Front. Bioeng. Biotechnol. 7, 225. doi:10.3389/fbioe.2019.00225
Chen, J., Ren, F., Cao, W., Wu, Z., Ju, G., Xiao, C., et al. (2021). Photothermal therapy enhance the anti-mitochondrial metabolism effect of lonidamine to renal cell carcinoma in homologous-targeted nanosystem. Nanomedicine 34, 102370. doi:10.1016/j.nano.2021.102370
Chen, W., Li, J., Wei, X., Fan, Y., Qian, H., Li, S., et al. (2020). Surface plasmon resonance biosensor using hydrogel-AuNP supramolecular spheres for determination of prostate cancer-derived exosomes. Mikrochim. Acta 187 (11), 590. doi:10.1007/s00604-020-04573-4
Choi, K. H., Nam, K. C., Cho, G., Jung, J. S., and Park, B. (2018). Enhanced Photodynamic Anticancer Activities of Multifunctional Magnetic Nanoparticles (Fe3O4) Conjugated with Chlorin e6 and Folic Acid in Prostate and Breast Cancer Cells. Nanomater. (Basel) 8 (9), 722. doi:10.3390/nano8090722
Corbo, C., Molinaro, R., Parodi, A., Toledano Furman, N. E., Salvatore, F., and Tasciotti, E. (2016). The impact of nanoparticle protein corona on cytotoxicity, immunotoxicity and target drug delivery. Nanomedicine (Lond) 11 (1), 81–100. doi:10.2217/nnm.15.188
Daei, S., Ziamajidi, N., Abbasalipourkabir, R., Khanaki, K., and Bahreini, F. (2022). Anticancer effects of gold nanoparticles by inducing apoptosis in bladder cancer 5637 cells. Biol. Trace Elem. Res. 200 (6), 2673–2683. doi:10.1007/s12011-021-02895-9
Du, D., Fu, H. J., Ren, W. W., Li, X. L., and Guo, L. H. (2020). PSA targeted dual-modality manganese oxide–mesoporous silica nanoparticles for prostate cancer imaging. Biomed. Pharmacother. 121, 109614. doi:10.1016/j.biopha.2019.109614
Dykman, L., and Khlebtsov, N. (2012). Gold nanoparticles in biomedical applications: Recent advances and perspectives. Chem. Soc. Rev. 41 (6), 2256–2282. doi:10.1039/c1cs15166e
Eissa, S., Shawky, S. M., Matboli, M., Mohamed, S., and Azzazy, H. M. (2014). Direct detection of unamplified hepatoma upregulated protein RNA in urine using gold nanoparticles for bladder cancer diagnosis. Clin. Biochem. 47 (1-2), 104–110. doi:10.1016/j.clinbiochem.2013.10.022
Elhissi, A. M., Ahmed, W., Hassan, I. U., Dhanak, V. R., and D'Emanuele, A. (2012). Carbon nanotubes in cancer therapy and drug delivery. J. Drug Deliv. 2012, 837327. doi:10.1155/2012/837327
Esfahani, M., Alavi, S. E., Cabot, P. J., Islam, N., and Izake, E. L. (2021). PEGylated mesoporous silica nanoparticles (MCM-41): A promising carrier for the targeted delivery of fenbendazole into prostrate cancer cells. Pharmaceutics 13 (10), 1605. doi:10.3390/pharmaceutics13101605
Farzin, A., Etesami, S. A., Quint, J., Memic, A., and Tamayol, A. (2020). Magnetic nanoparticles in cancer therapy and diagnosis. Adv. Healthc. Mater 9 (9), e1901058. doi:10.1002/adhm.201901058
Felice, B., Prabhakaran, M. P., Rodriguez, A. P., and Ramakrishna, S. (2014). Drug delivery vehicles on a nano-engineering perspective. Mater Sci. Eng. C Mater Biol. Appl. 41, 178–195. doi:10.1016/j.msec.2014.04.049
Fu, C., Liu, T., Li, L., Liu, H., Chen, D., and Tang, F. (2013). The absorption, distribution, excretion and toxicity of mesoporous silica nanoparticles in mice following different exposure routes. Biomaterials 34 (10), 2565–2575. doi:10.1016/j.biomaterials.2012.12.043
Greish, K., Thiagarajan, G., Herd, H., Price, R., Bauer, H., Hubbard, D., et al. (2012). Size and surface charge significantly influence the toxicity of silica and dendritic nanoparticles. Nanotoxicology 6 (7), 713–723. doi:10.3109/17435390.2011.604442
Gu, F., Hu, C., Xia, Q., Gong, C., Gao, S., and Chen, Z. (2018). Aptamer-conjugated multi-walled carbon nanotubes as a new targeted ultrasound contrast agent for the diagnosis of prostate cancer. J. Nanopart Res. 20 (11), 303. doi:10.1007/s11051-018-4407-z
Guan, J., Guo, H., Tang, T., Wang, Y., Wei, Y., Seth, P., et al. (2021). iRGD-liposomes enhance tumor delivery and therapeutic efficacy of antisense oligonucleotide drugs against primary prostate cancer and bone metastasis. Adv. Funct. Mater 31 (24), 2100478. doi:10.1002/adfm.202100478
Haddick, L., Zhang, W., Reinhard, S., Moller, K., Engelke, H., Wagner, E., et al. (2020). Particle-size-dependent delivery of antitumoral miRNA using targeted mesoporous silica nanoparticles. Pharmaceutics 12 (6), 505. doi:10.3390/pharmaceutics12060505
Hao, Y., Hu, J., Wang, H., and Wang, C. (2021). Gold nanoparticles regulate the antitumor secretome and have potent cytotoxic effects against prostate cancer cells. J. Appl. Toxicol. 41 (8), 1286–1303. doi:10.1002/jat.4117
He, Q., and Shi, J. (2014). MSN anti-cancer nanomedicines: Chemotherapy enhancement, overcoming of drug resistance, and metastasis inhibition. Adv. Mater 26 (3), 391–411. doi:10.1002/adma.201303123
He, Q., Zhang, Z., Gao, F., Li, Y., and Shi, J. (2011). In vivo biodistribution and urinary excretion of mesoporous silica nanoparticles: Effects of particle size and PEGylation. Small 7 (2), 271–280. doi:10.1002/smll.201001459
Hossen, S., Hossain, M. K., Basher, M. K., Mia, M., Rahman, M., and Uddin, M. J. (2019). Smart nanocarrier-based drug delivery systems for cancer therapy and toxicity studies: A review. J. Adv. Res. 15, 1–18. doi:10.1016/j.jare.2018.06.005
Htoo, K., Yamkamon, V., Yainoy, S., Suksrichavalit, T., Viseshsindh, W., and Eiamphungporn, W. (2019). Colorimetric detection of PCA3 in urine for prostate cancer diagnosis using thiol-labeled PCR primer and unmodified gold nanoparticles. Clin. Chim. Acta 488, 40–49. doi:10.1016/j.cca.2018.10.036
Huang, X., Li, L., Liu, T., Hao, N., Liu, H., Chen, D., et al. (2011). The shape effect of mesoporous silica nanoparticles on biodistribution, clearance, and biocompatibility in vivo. ACS Nano 5 (7), 5390–5399. doi:10.1021/nn200365a
Iturrioz-Rodriguez, N., Correa-Duarte, M. A., and Fanarraga, M. L. (2019). <p>Controlled drug delivery systems for cancer based on mesoporous silica nanoparticles</p>. Int. J. Nanomedicine 14, 3389–3401. doi:10.2147/ijn.s198848
Jaishree, V., and Gupta, P. D. (2012). Nanotechnology: A revolution in cancer diagnosis. Indian J. Clin. Biochem. 27 (3), 214–220. doi:10.1007/s12291-012-0221-z
Jigyasu, A. K., Siddiqui, S., Jafri, A., Arshad, M., Lohani, M., and Khan, I. A. (2020). Biological synthesis of CdTe quantum dots and their anti-proliferative assessment against prostate cancer cell line. J. Nanosci. Nanotechnol. 20 (6), 3398–3403. doi:10.1166/jnn.2020.17316
Khan, A., Dias, F., Neekhra, S., Singh, B., and Srivastava, R. (2020). Designing and immunomodulating multiresponsive nanomaterial for cancer theranostics. Front. Chem. 8, 631351. doi:10.3389/fchem.2020.631351
Kim, J., Chun, S. H., Amornkitbamrung, L., Song, C., Yuk, J. S., Ahn, S. Y., et al. (2020). Gold nanoparticle clusters for the investigation of therapeutic efficiency against prostate cancer under near-infrared irradiation. Nano Converg. 7 (1), 5. doi:10.1186/s40580-019-0216-z
Koohi, M. E. M., Alavi, S. E., Cabot, P. J., Islam, N., Izake, E. L., et al. (2022). Beta-lactoglobulin-modified mesoporous silica nanoparticles: A promising carrier for the targeted delivery of fenbendazole into prostate cancer cells[J]. Pharmaceutics 14 (4).
Lacerda, L., Russier, J., Pastorin, G., Herrero, M. A., Venturelli, E., Dumortier, H., et al. (2012). Translocation mechanisms of chemically functionalised carbon nanotubes across plasma membranes. Biomaterials 33 (11), 3334–3343. doi:10.1016/j.biomaterials.2012.01.024
Lang, Y., Tian, X., Dong, H. Y., Zhang, X. X., Yu, L., Li, M., et al. (2022). Black phosphorus quantum dots enhance the radiosensitivity of human renal cell carcinoma cells through inhibition of DNA-PKcs kinase. Cells 11 (10), 1651. doi:10.3390/cells11101651
Li, H., Wu, X., Yang, B., Li, J., Xu, L., Liu, H., et al. (2019). Evaluation of biomimetically synthesized mesoporous silica nanoparticles as drug carriers: Structure, wettability, degradation, biocompatibility and brain distribution. Mater Sci. Eng. C Mater Biol. Appl. 94, 453–464. doi:10.1016/j.msec.2018.09.053
Li, N., Xie, X., Hu, Y., He, H., Fu, X., Fang, T., et al. (2019). Herceptin-conjugated liposomes co-loaded with doxorubicin and simvastatin in targeted prostate cancer therapy. Am. J. Transl. Res. 11 (3), 1255–1269.
Li, X., Zhou, H., Yang, L., Du, G., Pai-Panandiker, A. S., Huang, X., et al. (2011). Enhancement of cell recognition in vitro by dual-ligand cancer targeting gold nanoparticles. Biomaterials 32 (10), 2540–2545. doi:10.1016/j.biomaterials.2010.12.031
Li, Z., Wang, S., Fan, X., Cao, B., and Zhou, C. (2019). A novel gold nanoprobe for a simple electrochemiluminescence determination of a prostate-specific antigen based on a peptide cleavage reaction. Anal. Sci. 35 (2), 195–199. doi:10.2116/analsci.18p377
Liu, R., Pei, Q., Shou, T., Zhang, W., Hu, J., and Li, W. (2019). <p>Apoptotic effect of green synthesized gold nanoparticles from <em>Curcuma wenyujin</em> extract against human renal cell carcinoma A498 cells</p>. Int. J. Nanomedicine 14, 4091–4103. doi:10.2147/ijn.s203222
Liu, Y., Wang, X., Hussain, M., Lv, M., Dong, X., Wang, T., et al. (2018). Theranostics applications of nanoparticles in cancer immunotherapy. Med. Sci. (Basel) 6 (4), 100. doi:10.3390/medsci6040100
Lopez-Chaves, C., Soto-Alvaredo, J., Montes-Bayon, M., Bettmer, J., Llopis, J., and Sanchez-Gonzalez, C. (2018). Gold nanoparticles: Distribution, bioaccumulation and toxicity. in vitro and in vivo studies. Nanomedicine 14 (1), 1–12. doi:10.1016/j.nano.2017.08.011
Luo, D., Johnson, A., Wang, X., Li, H., Erokwu, B. O., Springer, S., et al. (2020). Targeted radiosensitizers for MR-guided radiation therapy of prostate cancer. Nano Lett. 20 (10), 7159–7167. doi:10.1021/acs.nanolett.0c02487
Maiorano, G., Sabella, S., Sorce, B., Brunetti, V., Malvindi, M. A., Cingolani, R., et al. (2010). Effects of cell culture media on the dynamic formation of Protein−Nanoparticle complexes and influence on the cellular response. ACS Nano 4 (12), 7481–7491. doi:10.1021/nn101557e
Manan, F., Yusof, N. A., Abdullah, J., Mohammad, F., Nurdin, A., Yazan, L. S., et al. (2021). Drug release profiles of mitomycin C encapsulated quantum dots-chitosan nanocarrier system for the possible treatment of non-muscle invasive bladder cancer. J. Pharm. 13 (9), 1379. doi:10.3390/pharmaceutics13091379
Manshian, B. B., Martens, T. F., Kantner, K., Braeckmans, K., De Smedt, S. C., Demeester, J., et al. (2017). The role of intracellular trafficking of CdSe/ZnS QDs on their consequent toxicity profile. J. Nanobiotechnology 15 (1), 45. doi:10.1186/s12951-017-0279-0
Medetalibeyoglu, H., Kotan, G., Atar, N., and Yola, M. L. (2020). A novel and ultrasensitive sandwich-type electrochemical immunosensor based on delaminated MXene@AuNPs as signal amplification for prostate specific antigen (PSA) detection and immunosensor validation. Talanta 220, 121403. doi:10.1016/j.talanta.2020.121403
Mikhail, A. S., Negussie, A. H., Pritchard, W. F., Haemmerich, D., Woods, D., Bakhutashvili, I., et al. (2017). Lyso-thermosensitive liposomal doxorubicin for treatment of bladder cancer. Int. J. Hyperth. 33 (7), 733–740. doi:10.1080/02656736.2017.1315459
Mohtashami, L., Ghows, N., Tayarani-Najaran, Z., and Iranshahi, M. (2019). Galbanic acid-coated Fe3O4 magnetic nanoparticles with enhanced cytotoxicity to prostate cancer cells. Planta Med. 85 (2), 169–178. doi:10.1055/a-0721-1886
Nawaz, Q., Rehman, M., Burkovski, A., Schmidt, J., Beltran, A. M., Shahid, A., et al. (2018). Synthesis and characterization of manganese containing mesoporous bioactive glass nanoparticles for biomedical applications. J. Mater Sci. Mater Med. 29 (5), 64. doi:10.1007/s10856-018-6070-4
Ncapayi, V., Ninan, N., Lebepe, T. C., Parani, S., Girija, A. R., Bright, R., et al. (2021). Diagnosis of prostate cancer and prostatitis using near infra-red fluorescent AgInSe/ZnS quantum dots. Int. J. Mol. Sci. 22 (22), 12514. doi:10.3390/ijms222212514
Nikzad, S., Mahmoudi, G., Amini, P., Baradaran-Ghahfarokhi, M., Vahdat-Moaddab, A., Sharafi, S. M., et al. (2017). Effects of radiofrequency radiation in the presence of gold nanoparticles for the treatment of renal cell carcinoma. J. Ren. Inj. Prev. 6 (2), 103–108. doi:10.15171/jrip.2017.20
Nimesh, S., Gupta, N., and Chandra, R. (2011). Cationic polymer based nanocarriers for delivery of therapeutic nucleic acids. J. Biomed. Nanotechnol. 7 (4), 504–520. doi:10.1166/jbn.2011.1313
Nossier, A. I., Eissa, S., Ismail, M. F., Hamdy, M. A., and Azzazy, H. M. E. S. (2014). Direct detection of hyaluronidase in urine using cationic gold nanoparticles: A potential diagnostic test for bladder cancer. Biosens. Bioelectron. 54, 7–14. doi:10.1016/j.bios.2013.10.024
Othman, H. O., Salehnia, F., Fakhri, N., Hassan, R., Hosseini, M., Faizullah, A., et al. (2020). A highly sensitive fluorescent immunosensor for sensitive detection of nuclear matrix protein 22 as biomarker for early stage diagnosis of bladder cancer. RSC Adv. 10 (48), 28865–28871. doi:10.1039/d0ra06191c
Ovais, M., Raza, A., Naz, S., Islam, N. U., Khalil, A. T., Ali, S., et al. (2017). Current state and prospects of the phytosynthesized colloidal gold nanoparticles and their applications in cancer theranostics. Appl. Microbiol. Biotechnol. 101 (9), 3551–3565. doi:10.1007/s00253-017-8250-4
Pal, K., Madamsetty, V. S., Dutta, S. K., and Mukhopadhyay, D. (2019). <p>Co-delivery of everolimus and vinorelbine via a tumor-targeted liposomal formulation inhibits tumor growth and metastasis in RCC</p>. Int. J. Nanomedicine 14, 5109–5123. doi:10.2147/ijn.s204221
Penticuff, J. C., and Kyprianou, N. (2015). Therapeutic challenges in renal cell carcinoma. Am. J. Clin. Exp. Urol. 3 (2), 77–90.
Pilch, J., Kowalik, P., Kowalczyk, A., Bujak, P., Kasprzak, A., Paluszkiewicz, E., et al. (2022). Foliate-targeting quantum dots-β-cyclodextrin nanocarrier for efficient delivery of unsymmetrical bisacridines to lung and prostate cancer cells. Int. J. Mol. Sci. 23 (3), 1261. doi:10.3390/ijms23031261
Pisani, C., Rascol, E., Dorandeu, C., Charnay, C., Guari, Y., Chopineau, J., et al. (2017). Biocompatibility assessment of functionalized magnetic mesoporous silica nanoparticles in human HepaRG cells. Nanotoxicology 11 (7), 871–890. doi:10.1080/17435390.2017.1378749
Pissuwan, D., Niidome, T., and Cortie, M. B. (2011). The forthcoming applications of gold nanoparticles in drug and gene delivery systems. J. Control Release 149 (1), 65–71. doi:10.1016/j.jconrel.2009.12.006
Porrang, S., Davaran, S., Rahemi, N., Allahyari, S., and Mostafavi, E. (2022). How advancing are mesoporous silica nanoparticles? A comprehensive review of the literature[J]. Int. J. Nanomedicine 17, 1803–1827. doi:10.2147/ijn.s353349
Pretze, M., Hien, A., Radle, M., Schirrmacher, R., Wangler, C., and Wangler, B. (2018). Gastrin-releasing peptide receptor- and prostate-specific membrane antigen-specific ultrasmall gold nanoparticles for characterization and diagnosis of prostate carcinoma via fluorescence imaging. Bioconjug Chem. 29 (5), 1525–1533. doi:10.1021/acs.bioconjchem.8b00067
Radaic, A., Joo, N. E., Jeong, S. H., Yoo, S. I., Kotov, N., and Kapila, Y. L. (2021). Phosphatidylserine-gold nanoparticles (PS-AuNP) induce prostate and breast cancer cell apoptosis. J. Pharm. 13 (7), 1094. doi:10.3390/pharmaceutics13071094
Rajendiran, K., Zhao, Z., Pei, D. S., and Fu, A. (2019). Antimicrobial activity and mechanism of functionalized quantum dots. J. Polym. (Basel) 11 (10), 1670. doi:10.3390/polym11101670
Rampado, R., Crotti, S., Caliceti, P., Pucciarelli, S., and Agostini, M. (2020). Recent advances in understanding the protein corona of nanoparticles and in the formulation of "stealthy" nanomaterials. Front. Bioeng. Biotechnol. 8, 166. doi:10.3389/fbioe.2020.00166
Rastogi, V., Yadav, P., Bhattacharya, S. S., Mishra, A. K., Verma, N., Verma, A., et al. (2014). Carbon nanotubes: An emerging drug carrier for targeting cancer cells. J. Drug Deliv. 2014, 670815. doi:10.1155/2014/670815
Rong, Z., Bai, Z., Li, J., Tang, H., Shen, T., Wang, Q., et al. (2019). Dual-color magnetic-quantum dot nanobeads as versatile fluorescent probes in test strip for simultaneous point-of-care detection of free and complexed prostate-specific antigen. Biosens. Bioelectron. 145, 111719. doi:10.1016/j.bios.2019.111719
Saroj, S., and Rajput, S. J. (2019). Facile development, characterization, and evaluation of novel bicalutamide loaded pH-sensitive mesoporous silica nanoparticles for enhanced prostate cancer therapy. Drug Dev. Ind. Pharm. 45 (4), 532–547. doi:10.1080/03639045.2018.1562463
Saroj, S., and Rajput, S. J. (2018). Tailor-made pH-sensitive polyacrylic acid functionalized mesoporous silica nanoparticles for efficient and controlled delivery of anti-cancer drug Etoposide. Drug Dev. Ind. Pharm. 44 (7), 1198–1211. doi:10.1080/03639045.2018.1438467
Shahidi, M., Abazari, O., Dayati, P., Bakhshi, A., Zavarreza, J., Modarresi, M. H., et al. (2022). Multicomponent siRNA/miRNA-loaded modified mesoporous silica nanoparticles targeted bladder cancer for a highly effective combination therapy. Front. Bioeng. Biotechnol. 10, 949704. doi:10.3389/fbioe.2022.949704
Shan, G., Tang, T., Qian, H., and Xia, Y. (2017). Expression of Tiam1 and Rac1 proteins in renal cell carcinoma and its clinical-pathological features. Int. J. Clin. Exp. Pathol. 10 (11), 11114–11121.
Shao, D., Lu, M. M., Zhao, Y. W., Zhang, F., Tan, Y. f., Zheng, X., et al. (2017). The shape effect of magnetic mesoporous silica nanoparticles on endocytosis, biocompatibility and biodistribution. Acta Biomater. 49, 531–540. doi:10.1016/j.actbio.2016.11.007
Shao, F., Zhang, L., Jiao, L., Wang, X., Miao, L., Li, H., et al. (2018). Enzyme-free immunosorbent assay of prostate specific antigen amplified by releasing pH indicator molecules entrapped in mesoporous silica nanoparticles. Anal. Chem. 90 (14), 8673–8679. doi:10.1021/acs.analchem.8b02019
Siegel, R. L., Miller, K. D., and Jemal, A. (2019). Cancer statistics, 2019. CA Cancer J. Clin. 69 (1), 7–34. doi:10.3322/caac.21551
Silva, V. L., Ruiz, A., Ali, A., Pereira, S., Seitsonen, J., Ruokolainen, J., et al. (2021). Hypoxia-targeted cupric-tirapazamine liposomes potentiate radiotherapy in prostate cancer spheroids. Int. J. Pharm. 607, 121018. doi:10.1016/j.ijpharm.2021.121018
Smilowitz, H. M., Tarmu, L. J., Sanders, M. M., Taylor III, J., Choudhary, D., Xue, C., et al. (2017). Biodistribution of gold nanoparticles in BBN-induced muscle-invasive bladder cancer in mice. Int. J. Nanomedicine 12, 7937–7946. doi:10.2147/ijn.s140977
Song, W., Musetti, S. N., and Huang, L. (2017). Nanomaterials for cancer immunotherapy. Biomaterials 148, 16–30. doi:10.1016/j.biomaterials.2017.09.017
Stolarczyk, E. U., Les, A., Laszcz, M., Kubiszewski, M., Strzempek, W., Menaszek, E., et al. (2020). The ligand exchange of citrates to thioabiraterone on gold nanoparticles for prostate cancer therapy. Int. J. Pharm. 583, 119319. doi:10.1016/j.ijpharm.2020.119319
Sukhanova, A., Bozrova, S., Sokolov, P., Berestovoy, M., Karaulov, A., and Nabiev, I. (2018). Dependence of nanoparticle toxicity on their physical and chemical properties. Nanoscale Res. Lett. 13 (1), 44. doi:10.1186/s11671-018-2457-x
Sun, C., Lee, J., and Zhang, M. (2008). Magnetic nanoparticles in MR imaging and drug delivery. Adv. Drug Deliv. Rev. 60 (11), 1252–1265. doi:10.1016/j.addr.2008.03.018
Suo, N., Wang, M., Jin, Y., Ding, J., Gao, X., Sun, X., et al. (2019). <p>Magnetic multiwalled carbon nanotubes with controlled release of epirubicin: An intravesical instillation system for bladder cancer</p>. Int. J. Nanomedicine 14, 1241–1254. doi:10.2147/ijn.s189688
Sweeney, S. K., Luo, Y., O'Donnell, M. A., and Assouline, J. (2016). Nanotechnology and cancer: Improving real-time monitoring and staging of bladder cancer with multimodal mesoporous silica nanoparticles. Cancer Nanotechnol. 7, 3. doi:10.1186/s12645-016-0015-8
Thambiraj, S., Vijayalakshmi, R., and Ravi, S. D. (2021). An effective strategy for development of docetaxel encapsulated gold nanoformulations for treatment of prostate cancer. Sci. Rep. 11 (1), 2808. doi:10.1038/s41598-020-80529-1
Vidaurre-Agut, C., Rivero-Buceta, E., Romani-Cubells, E., Clemments, A. M., Vera-Donoso, C. D., Landry, C. C., et al. (2019). Protein corona over mesoporous silica nanoparticles: Influence of the pore diameter on competitive adsorption and application to prostate cancer diagnostics. ACS Omega 4 (5), 8852–8861. doi:10.1021/acsomega.9b00460
Virani, N. A., Davis, C., McKernan, P., Hauser, P., Hurst, R. E., Slaton, J., et al. (2018). Phosphatidylserine targeted single-walled carbon nanotubes for photothermal ablation of bladder cancer. Nanotechnology 29 (3), 035101. doi:10.1088/1361-6528/aa9c0c
Vodnik, V. V., Mojic, M., Stamenovic, U., Otonicar, M., Ajdzanovic, V., Maksimovic-Ivanic, D., et al. (2021). Development of genistein-loaded gold nanoparticles and their antitumor potential against prostate cancer cell lines. Mater Sci. Eng. C Mater Biol. Appl. 124, 112078. doi:10.1016/j.msec.2021.112078
Wakaskar, R. R. (2018). Promising effects of nanomedicine in cancer drug delivery. J. Drug Target 26 (4), 319–324. doi:10.1080/1061186x.2017.1377207
Wang, H., Zhang, Y., Yu, H., Wu, D., Ma, H., Li, H., et al. (2013). Label-free electrochemical immunosensor for prostate-specific antigen based on silver hybridized mesoporous silica nanoparticles. Anal. Biochem. 434 (1), 123–127. doi:10.1016/j.ab.2012.11.012
Wei, Y., Gao, L., Wang, L., Shi, L., Wei, E., Zhou, B., et al. (2017). Polydopamine and peptide decorated doxorubicin-loaded mesoporous silica nanoparticles as a targeted drug delivery system for bladder cancer therapy. Drug Deliv. 24 (1), 681–691. doi:10.1080/10717544.2017.1309475
Williams, R. M., Lee, C., and Heller, D. A. (2018). A fluorescent carbon nanotube sensor detects the metastatic prostate cancer biomarker uPA. ACS Sens. 3 (9), 1838–1845. doi:10.1021/acssensors.8b00631
Wu, D., Zhu, Z. Q., Tang, H. X., Shi, Z. E., Kang, J., Liu, Q., et al. (2020). Efficacy-shaping nanomedicine by loading calcium peroxide into tumor microenvironment-responsive nanoparticles for the antitumor therapy of prostate cancer. Theranostics 10 (21), 9808–9829. doi:10.7150/thno.43631
Wu, T., Duan, X., Hu, C., Wu, C., Chen, X., Huang, J., et al. (2019). Synthesis and characterization of gold nanoparticles from Abies spectabilis extract and its anticancer activity on bladder cancer T24 cells. Artif. Cells Nanomed Biotechnol. 47 (1), 512–523. doi:10.1080/21691401.2018.1560305
Xia, Q., Gong, C., Gu, F., Wang, Z., Hu, C., Zhang, L., et al. (2018). Functionalized multi-walled carbon nanotubes for targeting delivery of immunostimulatory CpG oligonucleotides against prostate cancer. J. Biomed. Nanotechnol. 14 (9), 1613–1626. doi:10.1166/jbn.2018.2605
Xie, X., Liao, J., Shao, X., Li, Q., and Lin, Y. (2017). The effect of shape on cellular uptake of gold nanoparticles in the forms of stars, rods, and triangles. Sci. Rep. 7 (1), 3827. doi:10.1038/s41598-017-04229-z
Yari, H., Nkepang, G., and Awasthi, V. (2019). Surface modification of liposomes by a lipopolymer targeting prostate specific membrane antigen for theranostic delivery in prostate cancer. Mater. (Basel) 12 (5), 756. doi:10.3390/ma12050756
Yoshino, T., Miyazaki, J., Kojima, T., Kandori, S., Shiga, M., Kawahara, T., et al. (2019). Cationized liposomal keto-mycolic acids isolated from Mycobacterium bovis bacillus Calmette-Guérin induce antitumor immunity in a syngeneic murine bladder cancer model. PLoS One 14 (1), e0209196. doi:10.1371/journal.pone.0209196
Yuan, R., Rao, T., Cheng, F., Yu, W. M., Ruan, Y., Zhang, X. B., et al. (2018). Quantum dot-based fluorescent probes for targeted imaging of the EJ human bladder urothelial cancer cell line. Exp. Ther. Med. 16 (6), 4779–4783. doi:10.3892/etm.2018.6805
Zhang, X. D., Wu, H. Y., Wu, D., Wang, Y. Y., Chang, J. H., Zhai, Z. B., et al. (2010). Toxicologic effects of gold nanoparticles in vivo by different administration routes. Int. J. Nanomedicine 5, 771–781. doi:10.2147/IJN.S8428
Zhao, P., Chen, X., Wang, Q., Zou, H., Xie, Y., Liu, H., et al. (2020). Differential toxicity mechanism of gold nanoparticles in HK-2 renal proximal tubular cells and 786-0 carcinoma cells. Nanomedicine (Lond) 15 (11), 1079–1096. doi:10.2217/nnm-2019-0417
Keywords: mesoporous silica nanoparticles (MSN), gold nanoparticles (Au NPs), carbon nanotubes, magnetic nanoparticles (MNP), urinary tumors, quantum dots, liposome
Citation: Qian Z, Zhang Y, Yuan J, Gong S and Chen B (2023) Current applications of nanomaterials in urinary system tumors. Front. Bioeng. Biotechnol. 11:1111977. doi: 10.3389/fbioe.2023.1111977
Received: 30 November 2022; Accepted: 07 February 2023;
Published: 20 February 2023.
Edited by:
Guoqing Pan, Jiangsu University, ChinaReviewed by:
Chaoliang He, Changchun Institute of Applied Chemistry (CAS), ChinaQun Treen Huo, University of Central Florida, United States
Copyright © 2023 Qian, Zhang, Yuan, Gong and Chen. This is an open-access article distributed under the terms of the Creative Commons Attribution License (CC BY). The use, distribution or reproduction in other forums is permitted, provided the original author(s) and the copyright owner(s) are credited and that the original publication in this journal is cited, in accordance with accepted academic practice. No use, distribution or reproduction is permitted which does not comply with these terms.
*Correspondence: Binghai Chen, Y2hlbmJobnlAMTYzLmNvbQ==