- 1College of Civil Engineering, Huaqiao University, Xiamen, China
- 2Fujian Water Conservancy and Hydropower Engineering Bureau Company Limited, Quanzhou, China
With societal development, the growing scale of engineering construction, and the increase in environmental protection requirements, the necessity of engineering waste mud disposal is becoming increasingly prominent. In this study, microbially induced struvite precipitation (MISP) was introduced to treat engineering waste mud. The study mainly focused on: i) the optimal mineralization scheme for microbially induced struvite precipitation, ii) the feasibility of the process and the effect of reaction parameters on treating engineering waste mud with microbially induced struvite precipitation, and iii) the mechanism of microbially induced struvite precipitation in treating engineering waste mud. The results showed that the waste mud could be well treated with
1 Introduction
With increasing societal development, the scale of engineering construction is continuing to expand, resulting in the production of greater amounts of construction mud (e.g., shield soil). According to a previous study, 300 million
Flocculation is an important process in treating engineering waste mud. To date, there are various flocculants, including inorganic flocculants, organic flocculants, and microbial flocculants (Lee et al., 2014; Dotto et al., 2019). Inorganic flocculants, such as aluminium and ferric polymers, are economically friendly and easy to obtain, but they have negative effects on water quality. Organic flocculants essentially refer to organic polymer flocculants, and polyacrylamide and its derivatives are the primary organic polymer flocculants. Specifically, polyacrylamide and its derivatives can be split into non-ionic, cationic, anionic, and amphoteric types. They can significantly accelerate the flocculation process at a low dosage and are convenient to separate from water (Wei et al., 2018). Although possessing a better pH in application than inorganic flocculants (Faouzi et al., 2018), organic polymer flocculants are difficult to degrade and could further cause secondary environmental pollution. Previous studies had found that chemical methods using calcium and sodium salts had adverse effects on the microstructure evolution and macroscopic mechanical properties of silt soils (Wang et al., 2022a).
Microbial flocculants are safe, non-toxic and environmentally friendly and are considered ideal substitutes for inorganic and organic polymer coagulants (Zhao et al., 2017). Therefore, as a product with centuries of history, microbial flocculants may have extensive application potential in treating engineering waste mud. Generally, non-toxic biopolymers formed by microorganisms or their metabolites are the active components of microbial flocculants. The flocculation efficiency is positively correlated with the length of the biopolymer flocculant molecular chain; that is, the longer the molecular chain is, the better the flocculation efficiency (Li et al., 2020). With advances in science and technology, various microbial flocculants have been investigated and developed. Traditional microbial flocculants are metabolites produced by microorganisms and do not participate directly in the flocculation process. Thus, it is necessary to strip the active ingredient from the microbial medium to explore the mechanisms of flocculation. For example, flocculants can be separated and purified from microbial media through organic solvent precipitation and the Sevage method (Wei, 2017). However, these methods are complicated and difficult to perform.
Phosphate (P) and ammonia (N) are often present in actual water bodies and cause eutrophication at high concentrations (Mavhungu et al., 2021). Therefore, the recovery of P and N is an important process in water treatment. In this study, microbially induced struvite precipitation (MISP) was introduced to treat engineering waste mud. In contrast to traditional microbial flocculants, MISP technology can realize the direct participation of bacteria in the flocculation process. The MISP technique is one of the biomineralization techniques, along with microbially induced calcite precipitation (MICP) and enzyme-induced carbonate precipitation (EICP). Previous studies had explored reinforcing rocks and soils with MICP technology (Tang et al., 2020; Xiao et al., 2021). EICP technology was used to increase soil strength, and the addition of Mg2+ could enhance the improvement effect (Hu et al., 2021). In addition, Electrokinetic treatment (EKT) and Biopile treatment, excellent soil remediation techniques, effectively degraded heavy metals and organic matter in the soil (Gandhi et al., 2022; Mohammad et al., 2022). In previous studies, straw fiber could be used to increase the strength of soil (Xue et al., 2021).
Microorganisms, equipped with negative charges, can transform nitrogen-containing compounds to
This study introduced MISP to treat engineering waste mud and mainly focused on: i) the optimal mineralization scheme for MISP, ii) the feasibility and the effect of reaction parameters on treating engineering waste mud with MISP, and iii) the mechanism of MISP in treating engineering waste mud. The microbial and MISP mechanisms were studied by X-ray diffraction (XRD), scanning electron microscopy (SEM), and other characterization methods.
2 Materials and methods
2.1 Materials
All initial inorganic reagents were of analytical grade and purchased from Sinopharm Chemical Reagent Co., Ltd. The yeast extract was of biotechnology grade and was purchased from Sangon Biotech (Shanghai) Co., Ltd. All chemicals were used as purchased, without further purification. Bacteria were purchased from the German Biological Collection. Deionized water was used in all experiments.
2.2 Bacterial strain, culture medium, and cultivation
Sporosarcina pasteurii is a facultative anaerobic Gram-positive bacterium with a high urease secretion capacity (Yang, 2013), good adaptability in the environment, and high urease activity and mineralization efficiency, which is often used to reinforce rocks and soils via MICP (Mountassir et al., 2018; Liu and Gao, 2020). This bacterium was selected for the experiments in this study.
ATCC 1376 NH4-YE medium (Wang et al., 2019) was used to culture S. pasteurii. For the study, 100 mL of the culture medium was placed in a 250 mL conical flask and autoclaved at 121°C for 40 min. Then, the strain was inoculated into the culture medium, covered with breathable film, and cultured at 30°C and 180 rpm for 48 h to prepare a stable liquid as the seed liquid for subsequent experiments.
In this study, the concentration of bacteria was calculated by using the optical density of the bacterial solution at 600 nm (OD600) (Zhao, 2014). When OD600 = 1.5, the number of microorganisms was considered to be stable (Sarda et al., 2009).
2.3 Experimental procedures
To explore the optimal mineralization parameters, feasibility, and mechanisms of MISP during flocculation, the concentration of the added bacterial solution was first determined. Then, we explored the optimal scheme and feasibility for the treatment of engineering waste mud with MISP, as well as the influence of pH and heavy metal ions on the treatment efficiency. Finally, the mechanisms were analyzed through microscopic characterization.
2.3.1 Determination of bacterial concentration
To obtain the optimal bacterial concentration conducive to mineralization, mineralization tests were designed with different bacterial concentrations using a direct ammonium source (
Due to their ability to convert urea in the environment to
In addition, a sterile culture medium was used as the control group to exclude the influence of different volumes of culture medium on MAP mineralization. Therefore, an experiment to explore the influence of the culture medium on MAP precipitation was designed in the sterile state, and the conditions are shown in A2, B2, and C2 as noted in Table 1. The bacterial solution was filtered through a 0.22
The appropriate microbial concentration for MAP was determined through the above experiments for use in subsequent experiments. The apparent rate constant (
where C is the concentration of the sample and t is the reaction time at the sample collection time point. The concentration of
2.3.2 Optimization of the MISP mineralization scheme
A 5 g/L kaolin suspension was used as simulated mud to optimize the scheme and analyze the effect of MISP mud treatment (Ajao et al., 2018). The experimental group was divided into six groups according to the concentration of the added reactants. The control group was divided into control group I and control group II, and bacteria were replaced with culture medium and ultrapure water, respectively. The system components of each group are shown in Table 2. Kaolin powder was added to the mixed solution composed of
Finally, the precipitation samples obtained from the experiment were washed and dried. XRD and SEM were used to analyze the mechanisms involved in the treatment of engineering waste mud with MISP. The obtained liquid was filtered and tested in mice to analyze toxicity. The experimental method and process are shown in Figure 2.
2.3.3 Effect of initial pH
The precipitation of MAP can be affected by pH. To understand the influence of pH on the flocculation effect of the treatment of engineering waste mud with MISP, the mineralization flocculation experiments under 6, 7, 8, 9, 10, and 11 pH values (adjusted by the prepared PBS (
2.3.4 Effect of
Heavy metal ions that enter surrounding environments can threaten human health, such as by causing kidney failure and neurological and digestive disorders in humans upon exposure (Xue et al., 2022). A previous study reported that in Hangzhou shield waste mud, heavy metal ions were prevalent, and the concentration of
2.4 Analytical methods
2.4.1 Concentrations of phosphate ( -P) and ammonia ( -N)
Since the precipitation capacity in the experiment was difficult to quantitatively analyze, the ratio of the
2.4.2 Flocculation rate
The flocculation rate is a dimensionless ratio and was used to denote the flocculation efficiency. At the end of the reaction, the suspension was moved to a settling tube and left undisturbed for 10 min. The absorbance value of the supernatant at 550 nm was measured by a UV‒vis spectrophotometer and used in the calculation in Eq. 6.
A1 is the absorbance value of the Control II supernatant at 550 nm, and B1 is the absorbance value of the sample supernatant at 550 nm.
2.4.3 Sample analysis
Sediment was collected through a vacuum filter, washed three times with anhydrous ethanol, and dried in a drying box at 50°C. The material composition of the samples was analyzed by XRD. All XRD analyses were performed on a Miniflex 600 (Neiji Co., LTD., Japan). The scanning angle was 4°–90°, and the scanning speed was 10°/min. MDI Jade 6 Pattern Processing software was used to identify the qualitative phases. The morphology and elemental composition of the samples were studied by SEM and transmission electron microscopy (TEM). An SU 1510 SEM system (Hitachi High-Tech Co., LTD., Japan) and a Talso F200X TEM system (Thermo Fisher Scientific Co., LTD., United States) were used.
3 Results and discussion
3.1 Determination of bacterial concentration
3.1.1 Direct ammonium source
White precipitates were observed at 20 min and significantly increased at 3 h in A0, B0, and C0. The precipitates of A0 were less than those of the other two treatments (Figure 3). This result indicated that the bacterial solution had the ability to induce precipitation in the MISP system, and the concentration of bacteria had a positive effect on the reduction in precipitation.
Figures 4A, B shows the effect of various bacterial solution concentrations (A0, B0, and C0) on
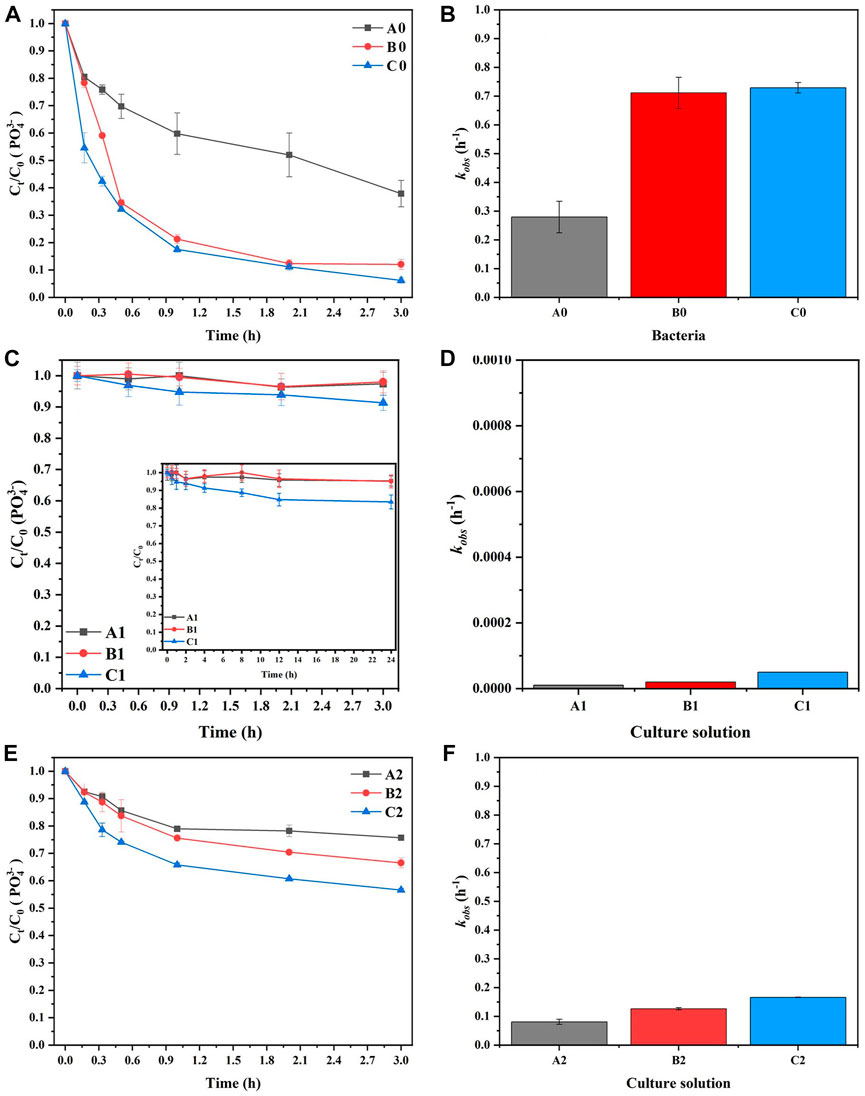
FIGURE 4. The effect of bacterial concentration on (A) the elimination of
Figures 4C, D shows the elimination of
The medium also had the ability to precipitate MAP due to the presence of
In addition, in the presence of microorganisms, the mineralization rate of MAP and the removal of
3.1.2 Indirect ammonium source
Figure 5 shows the effect of various bacterial solution concentrations (A3, B3, and C3) on
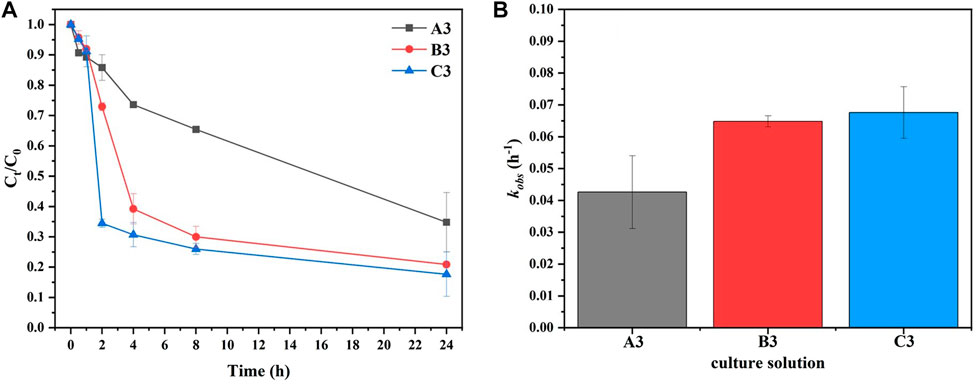
FIGURE 5. (A) The elimination of
Interestingly, the concentrations of
Previous studies reported that bacterially induced MAP crystallization took more than 72 h to consume most of the
3.2 Optimization of the MISP mineralization scheme
Figure 6 shows the effect of different groups (Table 2) on
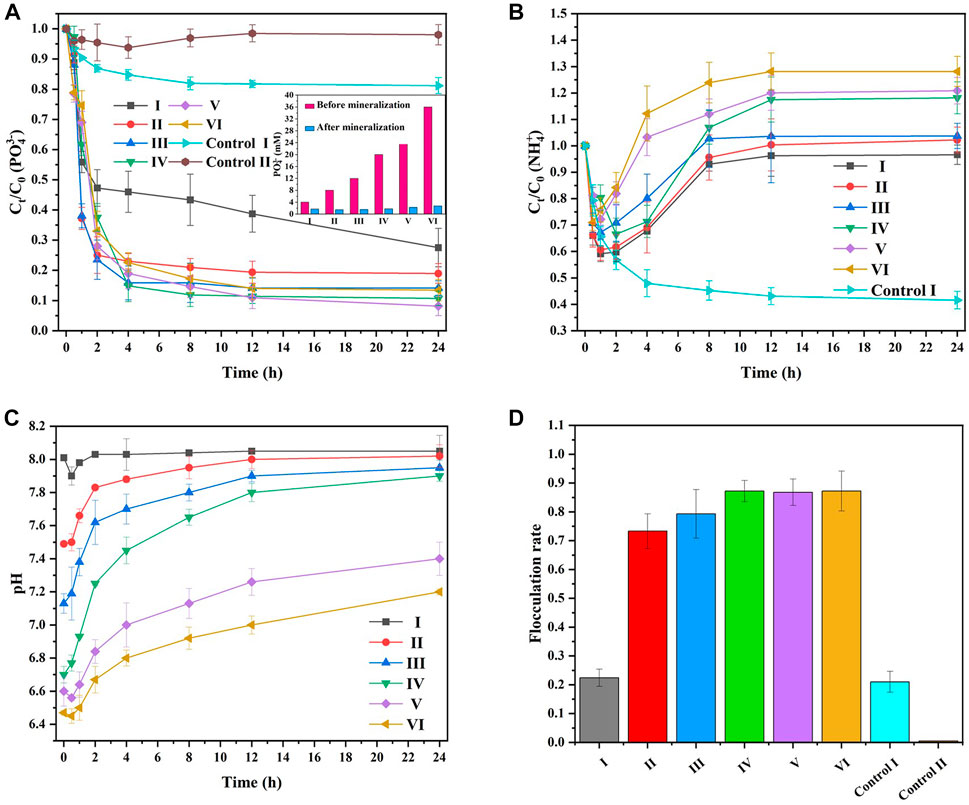
FIGURE 6. The elimination of (A)
The concentration of
Generally, the decrease in
Hydrolysis of urea can increase surrounding pH, and the formation of struvite can reduce surrounding pH. As shown in Figure 6C, the pH of all groups decreased at 0–0.5 h. And then increased and approached pH 8 after 0.5 h. This is because
As shown in Figure 6D, from Group I to Group IV, the flocculation rates were 22.4%, 73.3%, 85.3%, and 87.2%, respectively, suggesting that the flocculation rate improved with increasing reactant concentration. Group I exhibited a 22.4% flocculation rate, and Control I exhibited a 21.0% flocculation rate, indicating that the flocculation effect could also be observed in the presence of the culture medium. This result revealed that MAP could be mineralized and precipitated between kaolin particles in the MISP system. Therefore, there existed a synergistic effect between the decrease in
In summary, 10 mM urea, 20 mM PBS, and 25 mM
3.3 Effect of initial pH
Generally, pH 9 is the optimum condition for MAP mineralization and precipitation with non-microbial processes (Jaffer et al., 2002), while MAP precipitation induced by bacteria has an excellent effect at pH values between 7.3 and 8.3 (Tansel et al., 2018). Therefore, it is necessary to explore the influence of pH on MAP mineralization and precipitation in MISP technology. Figure 7 shows the effect of pH on the
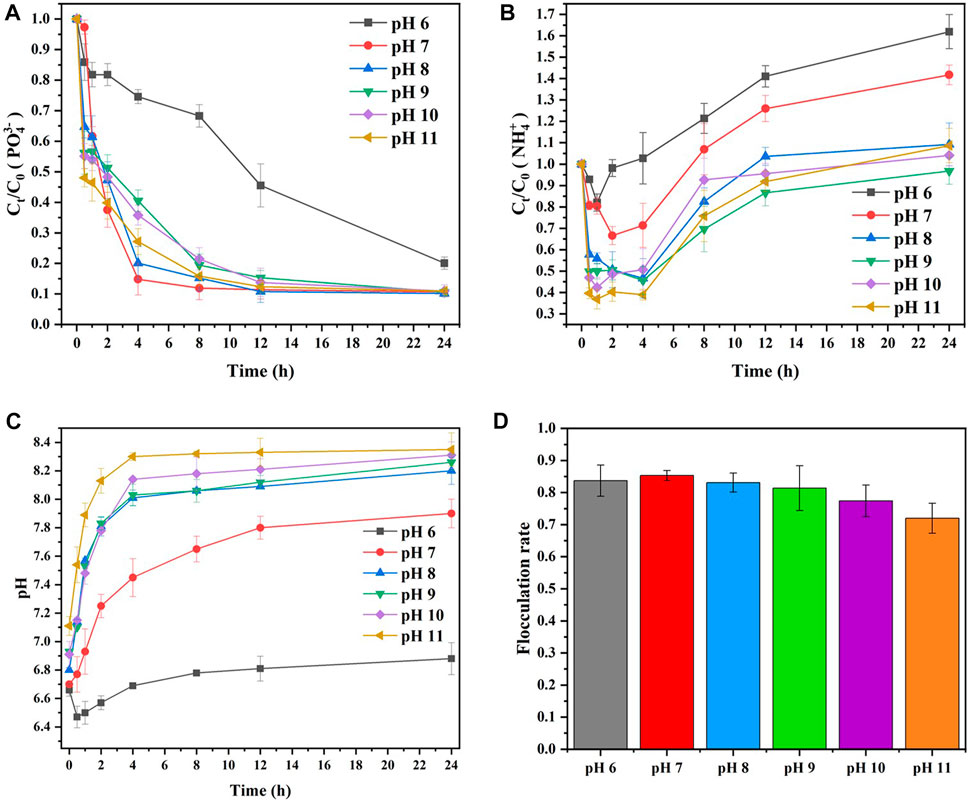
FIGURE 7. Effect of initial pH on the elimination of (A)
As displayed in Figure 7A, the elimination efficiency of
The
As shown in Figure 7C, the reaction solution pH could not be well buffered by PBS because of the added acidic MgCl2. The initial pH of the reaction is between 6.6 and 7.1. Then, the pH increased gradually as the reaction progressed. It was because the
Therefore, the flocculation rate was related to the utilization rate of
3.4 Effect of
The waste mud contained a certain concentration of
Figure 8 shows the effect of
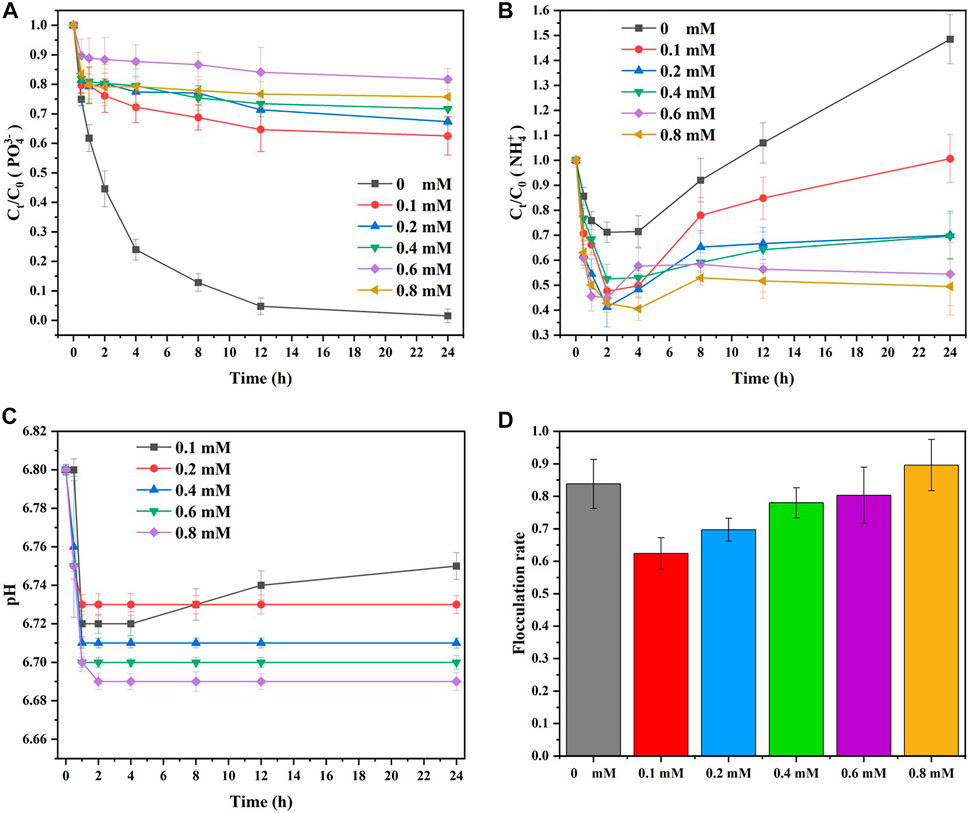
FIGURE 8. Effect of Cu2+ concentration on the elimination of (A)
As shown in Figure 8B, the concentration of
As shown in Figure 8C, the reaction solution pH was not alkaline because the added solutions of MgCl2 and CuCl2 were acid and could not be well buffered by PBS. Mg2+ and Cu2+ could not coexist with
Owing to the inhibition effect of the added
In summary,
3.5 Characteristics of precipitates
To verify their specific composition, the precipitates were collected, washed, and dried according to the method described in Section 2.4.4, and then the material composition, morphology, and elemental composition were analyzed by XRD, SEM, and TEM-EDS.
As shown in Figure 9A, the XRD patterns of the precipitates of B3 showed strong typical MAP diffraction peaks at 16°, 21°, and 33° (Thamaphat et al., 2008), suggesting that MAP was the main component of the precipitates. Figure 9B shows the XRD patterns of the different groups in Section 3.2. The kaolin from Control I and Control II showed strong diffraction peaks at 29°, 33°, and 43°, while the patterns at other angles were flat. The XRD patterns of the flocculated products in the experimental group showed that in addition to the original diffraction peaks for kaolin, stronger MAP diffraction peaks at 16°, 21°, and 33° could be detected. These results further indicated that MAP was the main component of the flocculation products obtained from the above experiments. With increasing reactant concentration, the diffraction peaks of the XRD patterns at 16°, 21°, and 33° became stronger (Figure 9B), suggesting that the amount of MAP in the mineralized products accumulated with increasing reactant concentration. Interestingly, from Group I to Group III, XRD peaks could also be detected at angles near 16°, 21°, and 33°, and the diffraction peaks became stronger with increasing reactant concentration. However, from Group IV to Group VI, diffraction peaks only appeared at 16°, 21°, and 33° and were stronger than those in Group I to Group III. These results indicated that increasing the concentration of the reactants was conducive to the generation of MAP crystals with a single shape in the flocculated products.
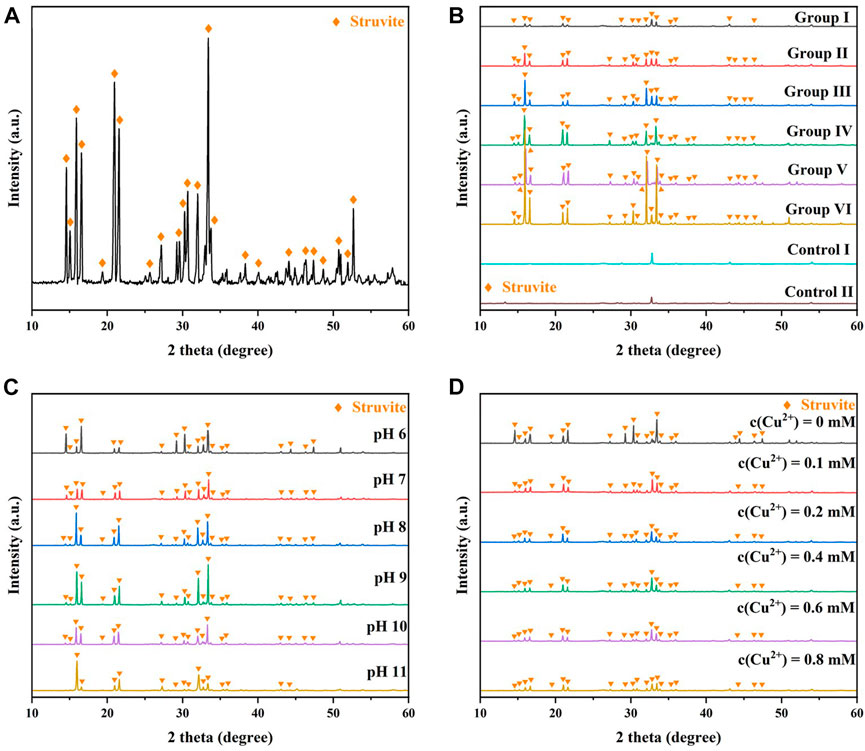
FIGURE 9. (A) XRD patterns of MAP and XRD patterns of precipitates for (B) different groups, (C) different
Figure 9C shows the effect of initial pH on the XRD patterns of the reaction precipitates. MAP crystallites are more disorganized in the initial pH 6. The initial pH from 7 to 9, the higher the initial pH, the more monotonous the crystal of the mineralized MAP. Figure 9D shows the effect of
MAP obtained from different methods or microorganisms exhibits different crystal shapes, usually needle-like (Chauhan and Joshi, 2013) or rod-like shapes (Stolzenburg et al., 2015). Therefore, SEM was carried out to detect the MAP morphology. Figure 10A shows SEM images of the MAP precipitates in B3 under the microbial mineralization described in Section 3.1.2. The MAP crystals had a length of 60–130
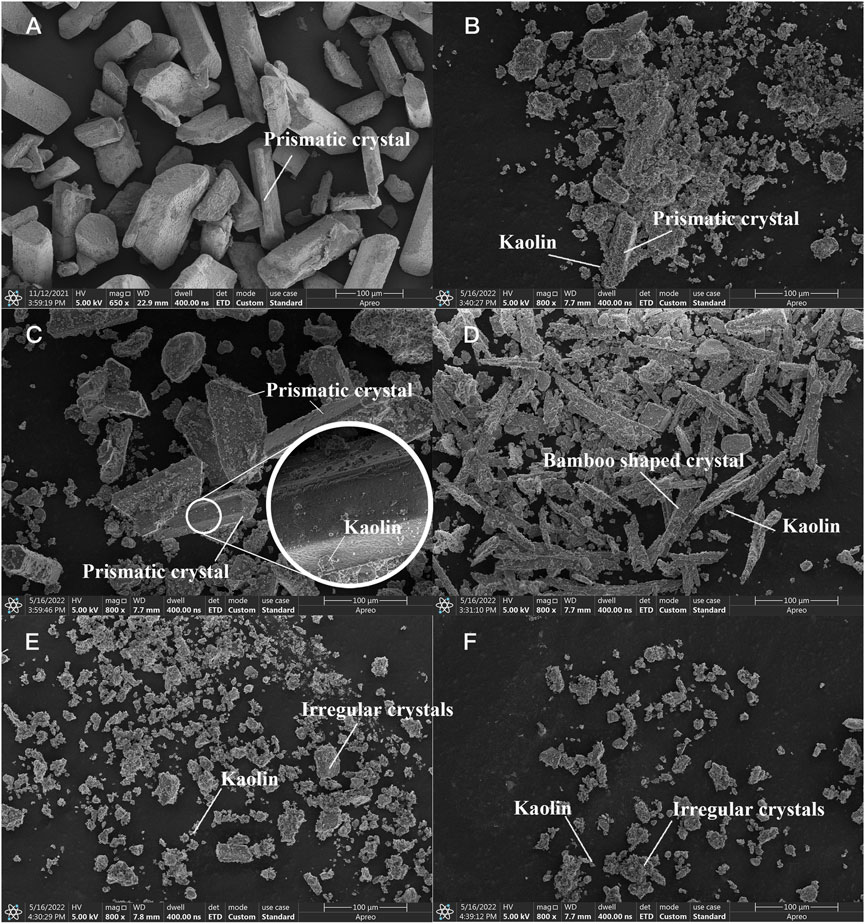
FIGURE 10. SEM images of precipitates from (A) B3, (B) Group I, (C) Group IV, (D) pH 11, (E)
Figure 10B show SEM photos of the flocculation products in Group I and Group IV. Although similar to the crystal shape shown in Figure 10A, the crystals observed in Group I and Group IV contained some extra matter on the surface (Figure 10B). This result indicated that the kaolin particles suspended in the MISP system can also serve as nucleation sites for MAP crystals, settle under gravity, and finally achieve flocculation. The mineralized crystals were small in volume and mass, prismatic in shape, and different in type (Figure 10B) because of the low concentration of reactants in Group I. As the reactant (i.e.,
According to a previous study, the shape of crystals changes under strongly alkaline pH conditions (Prywer et al., 2012). Figure 10D shows SEM imagines of the flocculated products at pH 11. The crystals were in the form of elongated rods with floccules attached to the crystal surface under strongly alkaline pH conditions. However, this was not a normal crystal shape for MAP and might have been caused by the high pH.
Figures 10E,F show SEM images of precipitates formed with 0.1 and 0.2 mM
Figure 11 shows the EDS analysis results and TEM elemental mapping images of precipitates formed with 0.8 mM
Therefore, with suitable mineralization conditions,
3.6 Costs
The costs of common inorganic flocculants and organic flocculants were compared to assess the cost of the MISP mud treatment. Different flocculants need different dosages to achieve the best flocculation efficiency. A previous study reported that mixing different inorganic flocculants with 20 mL acrylamide solution (52%) could achieve the best flocculation efficiency for mud treatment.
The cost of the MISP technology mainly consists of the costs of the culture medium and
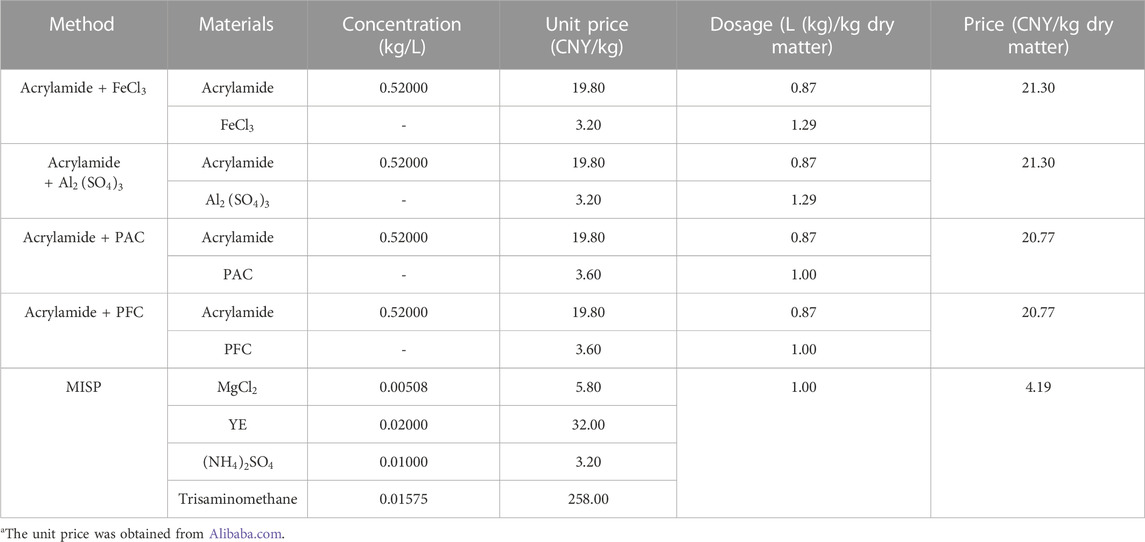
TABLE 3. Cost at current market valuea and dosage of flocculant.
In summary, the MISP technology possesses a relatively low environmental impact and costs, and the process would have good applications in the treatment of engineering waste mud.
4 Conclusion
In this study, MISP technology was introduced into the field of mud treatment to induce solid settling. The mechanism of MISP technology for mud treatment was explored, and the scheme for mineralization during the treatment of mud was optimized. The main conclusions are as follows:
(1) The flocculation rate could reach about 87.2% under the optimum condition of mud treatment with MISP technology (
(2) XRD and SEM analysis showed that the MAP crystals, with a length of 60–130 μm, were prismatic and surrounded by kaolin particles under the optimum mineralization parameters.
(3) MISP technology could improve the fertility of the flocculated product and should prioritize the removal of heavy metals for soil applications.
(4) MISP technology was more advantageous in cost and environmentally friendly than other methods to treat waste mud.
(5) In the real application, the depth of the mud pool and the stillness of the pool water would be the challenges with MISP. The injection of air into the mud pool and the stirring of the mud pool could improve MISP technology to treat waste mud.
Data availability statement
The original contributions presented in the study are included in the article/supplementary material, further inquiries can be directed to the corresponding author.
Author contributions
YH performed the research, analyzed most of the data, and wrote the paper; SL contributed the central idea and reviewed the paper; GS reviewed the paper; MP reviewed the paper; YC reviewed the paper; and JY reviewed the paper. All authors discussed the results and revised the manuscript.
Funding
This work was supported by the National Natural Science Foundation of China (Nos. 52178334 and 51978292).
Acknowledgments
Special thanks are extended to the Xiamen Institute of Rare Earth Materials, Haixi Institutes, University of Chinese Academy of Sciences, and to all the scholars mentioned in the references for data support at this study site.
Conflict of interest
Author MP was employed by Fujian Water Conservancy and Hydropower Engineering Bureau Company Limited.
The remaining authors declare that the research was conducted in the absence of any commercial or financial relationships that could be construed as a potential conflict of interest.
Publisher’s note
All claims expressed in this article are solely those of the authors and do not necessarily represent those of their affiliated organizations, or those of the publisher, the editors and the reviewers. Any product that may be evaluated in this article, or claim that may be made by its manufacturer, is not guaranteed or endorsed by the publisher.
References
Ajao, V., Bruning, H., Rijnaarts, H., and Temmink, H. (2018). Natural flocculants from fresh and saline wastewater: Comparative properties and flocculation performances. Chem. Eng. J. 349, 622–632. doi:10.1016/j.cej.2018.05.123
Chaouf, S., Barkany, S. E., Jilal, I., Ouardi, Y. E., Abou-salama, M., Loutou, M., et al. (2019). Anionic reverse microemulsion grafting of acrylamide (AM) on HydroxyEthylCellulose (HEC): Synthesis, characterization and application as new ecofriendly low-cost flocculant. J. Water Process Eng. 31, 100807. doi:10.1016/j.jwpe.2019.100807
Chauhan, C. K., and Joshi, M. J. (2013). In vitro crystallization, characterization and growth-inhibition study of urinary type struvite crystals. J. Cryst. Growth 362, 330–337. doi:10.1016/j.jcrysgro.2011.11.008
Ding, Z., Liu, T., Zhang, Y., Su, X., and Zheng, J. (2022). The curing and strength properties of highly moist waste mud from slurry shield tunnel construction. Appl. Sci. 12, 3762. doi:10.3390/app12083762
Dong, B., Liu, S., Gao, X., and Wang, R. (2020). Evaluation of microbial-induced phosphate precipitation strengthening calcareous sand in seawater environment. J. Civ. Environ. Eng. Chin. Engl. 42, 205–206. doi:10.11835/j.issn.2096-6717.2020.096
Dotto, J., Fagundes-Klen, M. R., Veit, M. T., Palácio, S. M., and Bergamasco, R. (2019). Performance of different coagulants in the coagulation/flocculation process of textile wastewater. J. Clean. Prod. 208, 656–665. doi:10.1016/j.jclepro.2018.10.112
Faouzi, B. R., Wissem, M., and Saifeldin, M. S. (2018). Microbial flocculants as an alternative to synthetic polymers for wastewater treatment: A review. Symmetry 10, 556. doi:10.3390/sym10110556
Gandhi, M., Moghal, A. A. B., Rasheed, R. M., and Almajed, A. (2022). State-of-the-art review on geoenvironmental benign applicability of biopiles. Innov. Infrastruct. Solut. 7, 166. doi:10.1007/s41062-022-00774-3
Hu, W., Cheng, W.-C., Wen, S., and Yuan, K. (2021). Revealing the enhancement and degradation mechanisms affecting the performance of carbonate precipitation in eICP Process. Front. Bioeng. Biotechnol. 9, 750258. doi:10.3389/fbioe.2021.750258
Jaffer, Y., Clark, T. A., Pearce, P., and Parsons, S. A. (2002). Potential phosphorus recovery by struvite formation. Water Res. 36, 1834–1842. doi:10.1016/S0043-1354(01)00391-8
Lee, C. S., Robinson, J., and Chong, M. F. (2014). A review on application of flocculants in wastewater treatment. Process Saf. Environ. Prot. 92, 489–508. doi:10.1016/j.psep.2014.04.010
Leng, Y., and Soares, A. (2021). The mechanisms of struvite biomineralization in municipal wastewater. Sci. Total Environ. 799, 149261. doi:10.1016/j.scitotenv.2021.149261
Li, H., Wu, S., Du, C., Zhong, Y., and Yang, C. (2020). Preparation, performances, and mechanisms of microbial flocculants for wastewater treatment. Int. J. Environ. Res. Public Health 17, 1360. doi:10.3390/ijerph17041360
Liu, S., and Gao, X. (2020). Evaluation of the anti-erosion characteristics of an MICP coating on the surface of tabia. J. Mater. Civ. Eng. 32, 04020304. doi:10.1061/(ASCE)MT.1943-5533.0003408
Lu, X., Huang, Z., Liang, Z., Li, Z., Yang, J., Wang, Y., et al. (2021). Co-precipitation of Cu and Zn in precipitation of struvite. Sci. Total Environ. 764, 144269. doi:10.1016/j.scitotenv.2020.144269
Luo, Y., Li, H., Huang, Y., Zhao, T., Yao, Q., Fu, S., et al. (2018). Bacterial mineralization of struvite using MgO as magnesium source and its potential for nutrient recovery. Chem. Eng. J. 351, 195–202. doi:10.1016/j.cej.2018.06.106
Mavhungu, A., Foteinis, S., Mbaya, R., Masindi, V., Kortidis, I., Mpenyana-Monyatsi, L., et al. (2021). Environmental sustainability of municipal wastewater treatment through struvite precipitation: Influence of operational parameters. J. Clean. Prod. 285, 124856. doi:10.1016/j.jclepro.2020.124856
Mohammad, N., Moghal, A. A. B., Rasheed, R. M., and Almajed, A. (2022). Critical review on the efficacy of electrokinetic techniques in geotechnical and geoenvironmental applications. Arab. J. Geosci. 15, 781. doi:10.1007/s12517-022-10037-1
Moulessehoul, A., Gallart-Mateu, D., Harrache, D., Djaroud, S., Guardia, M. d. l., and Kameche, M. (2017). Conductimetric study of struvite crystallization in water as a function of pH. J. Cryst. Growth 471, 42–52. doi:10.1016/j.jcrysgro.2017.05.011
Mouni, L., Belkhiri, L., Bollinger, J.-C., Bouzaza, A., Assadi, A., Tirri, A., et al. (2018). Removal of methylene blue from aqueous solutions by adsorption on kaolin: Kinetic and equilibrium studies. Appl. Clay Sci. 153, 38–45. doi:10.1016/j.clay.2017.11.034
Mountassir, G. E., Minto, J. M., Paassen, L. A. v., Salifu, E., and Lunn, R. J. (2018). “Chapter two - applications of microbial processes in geotechnical engineering,” in Adv. Appl. Microbiol. 104. Editors G. M. Gadd, and S. Sariaslani (Academic Press), 39–91.
Paris, D. F., and Rogers, J. E. (1986). Kinetic concepts for measuring microbial rate constants: Effects of nutrients on rate constants. Appl. Environ. Microbiol. 51, 221–225. doi:10.1128/aem.51.2.221-225.1986
Peng, C., Chai, L., Song, Y., Min, X., and Tang, C. (2018). Thermodynamics, kinetics and mechanism analysis of Cu(II) adsorption by in-situ synthesized struvite crystal. J. Cent. South Univ. 25, 1033–1042. doi:10.1007/s11771-018-3803-y
Prywer, J., Torzewska, A., and Płociński, T. (2012). Unique surface and internal structure of struvite crystals formed by Proteus mirabilis. Urol. Res. 40, 699–707. doi:10.1007/s00240-012-0501-3
Qi, L., Rui, C., Kai, Y., Wei, X., Dehong, G., and Fumin, R. (2022). Analysis of waste drilling fresh water mud pollutant treatment technology Chemical Safety and Environment. Environ. Eng. 3538, 1222–1526. doi:10.13205/j.hjgc.202003004
Sarda, D., Choonia, H. S., Sarode, D. D., and Lele, S. S. (2009). Biocalcification by Bacillus pasteurii urease: A novel application. J. Ind. Microbiol. Biotechnol. 36, 1111–1115. doi:10.1007/s10295-009-0581-4
Sathiasivan, K., Ramaswamy, J., and Rajesh, M. (2021). Struvite recovery from human urine in inverse fluidized bed reactor and evaluation of its fertilizing potential on the growth of Arachis hypogaea. J. Environ. Chem. Eng. 9, 104965. doi:10.1016/j.jece.2020.104965
Sinha, A., Singh, A., Kumar, S., Khare, S. K., and Ramanan, A. (2014). Microbial mineralization of struvite: A promising process to overcome phosphate sequestering crisis. Water Res. 54, 33–43. doi:10.1016/j.watres.2014.01.039
Stolzenburg, P., Capdevielle, A., Teychené, S., and Biscans, B. (2015). Struvite precipitation with MgO as a precursor: Application to wastewater treatment. Chem. Eng. Sci. 133, 9–15. doi:10.1016/j.ces.2015.03.008
Tang, C., Yin, L., Jiang, N., Zhu, C., Zeng, H., Li, H., et al. (2020). Factors affecting the performance of microbial-induced carbonate precipitation (MICP) treated soil: A review. Environ. Earth Sci. 79, 94. doi:10.1007/s12665-020-8840-9
Tansel, B., Lunn, G., and Monje, O. (2018). Struvite formation and decomposition characteristics for ammonia and phosphorus recovery: A review of magnesium-ammonia-phosphate interactions. Chemosphere 194, 504–514. doi:10.1016/j.chemosphere.2017.12.004
Thamaphat, K., Limsuwan, P., and Ngotawornchai, B. (2008). Phase characterization of TiO2 powder by XRD. TEM. Kasetsart J. (Nat. Sci.) 42, 357–361.
Thant Zin, M. M., and Kim, D. J. (2021). Simultaneous recovery of phosphorus and nitrogen from sewage sludge ash and food wastewater as struvite by Mg-biochar. J. Hazard. Mater. 403, 123704. doi:10.1016/j.jhazmat.2020.123704
Wang, L., Cheng, W.-C., and Xue, Z.-F. (2022a). Investigating microscale structural characteristics and resultant macroscale mechanical properties of loess exposed to alkaline and saline environments. Bull. Eng. Geol. Environ. 81, 146. doi:10.1007/s10064-022-02640-z
Wang, L., Cheng, W., and Xue, Z. (2022b). The effect of calcium source on Pb and Cu remediation using enzyme-induced carbonate precipitation. Front. Bioeng. Biotechnol. 10, 849631. doi:10.3389/fbioe.2022.849631
Wang, Y., Soga, K., DeJong, J. T., and Kabla, A. J. (2019). Microscale visualization of microbial-induced calcium carbonate precipitation processes. J. Geotech. Geoenviron. Eng. 145, 04019045. doi:10.1061/(ASCE)GT.1943-5606.0002079
Wei, H., Gao, B., Ren, J., Li, A., and Yang, H. (2018). Coagulation/flocculation in dewatering of sludge: A review. Water Res. 143, 608–631. doi:10.1016/j.watres.2018.07.029
Wei, W. (2017). Selective flocculation of fine-grained coal and minerals in slime water by microbial flocculants and its mechanism. Taiyuan, China: Taiyuan University of Technology.
Wu, L., Zhang, X., Chen, L., Zhang, H., Li, C., Lv, Y., et al. (2018). Amphoteric starch derivatives as reusable flocculant for heavy-metal removal. RSC Adv. 8, 1274–1280. doi:10.1039/C7RA12798G
Xiao, Y., Zhao, C., Sun, Y., Wang, S., Wu, H., Chen, H., et al. (2021). Compression behavior of MICP-treated sand with various gradations. Acta Geotech. 16, 1391–1400. doi:10.1007/s11440-020-01116-2
Xue, Z.-F., Cheng, W.-C., Wang, L., and Song, G. (2021). Improvement of the shearing behaviour of Loess using recycled straw fiber reinforcement. KSCE J. Civ. Eng. 25, 3319–3335. doi:10.1007/s12205-021-2263-3
Xue, Z., Cheng, W., Wang, L., Qin, P., and Zhang, B. (2022). Revealing degradation and enhancement mechanisms affecting copper (Cu) immobilization using microbial-induced carbonate precipitation (MICP). J. Environ. Chem. Eng. 10, 108479. doi:10.1016/j.jece.2022.108479
Yang, Z. (2013). Study on mechanism and working performance of high strength microbial mortar. Beijing, China: Tsinghua University.
Yao, C. (2006). Study on simultaneous recovery of ammonia nitrogen and phosphorus from wastewater treatment plant in the Form of struvite. Changsha, China: Hunan University.
Yu, X., Chu, J., Yang, Y., and Qian, C. (2021). Reduction of ammonia production in the biocementation process for sand using a new biocement. J. Clean. Prod. 286, 124928. doi:10.1016/j.jclepro.2020.124928
Yu, X., Qian, C., and Xue, B. (2016). Loose sand particles cemented by different bio-phosphate and carbonate composite cement. Constr. Build. Mater. 113, 571–578. doi:10.1016/j.conbuildmat.2016.03.105
Zhang, C., Liu, X., Fu, J., Yang, J., Li, L., and Xie, Y. (2022). Evaluating the feasibility of muck from slurry shield tunnels as a growth medium for landscaping. J. Air Waste Manage. Assoc. 72, 455–462. doi:10.1080/10962247.2022.2051775
Zhao, C., Yang, Q., and Zhang, H. (2017). Optimization of microbial flocculant-producing medium for bacillus subtilis. Indian J. Microbiol. 57, 83–91. doi:10.1007/s12088-016-0631-3
Zhao, Q. (2014). Experimental study on soil stabilization by microorganism induced calcium carbonate precipitation (MICP). Beijing): China University of Geosciences.
Keywords: MISP, flocculation, waste mud, Sporosarcina pasteurii, struvite
Citation: He Y, Liu S, Shen G, Pan M, Cai Y and Yu J (2023) Treatment of engineering waste slurries by microbially induced struvite precipitation mechanisms. Front. Bioeng. Biotechnol. 11:1109265. doi: 10.3389/fbioe.2023.1109265
Received: 27 November 2022; Accepted: 06 January 2023;
Published: 20 January 2023.
Edited by:
Wen-Chieh Cheng, Xi’an University of Architecture and Technology, ChinaReviewed by:
Zhong-Fei Xue, Xi’an University of Architecture and Technology, ChinaArif Ali Baig Moghal, National Institute of Technology Warangal, India
Copyright © 2023 He, Liu, Shen, Pan, Cai and Yu. This is an open-access article distributed under the terms of the Creative Commons Attribution License (CC BY). The use, distribution or reproduction in other forums is permitted, provided the original author(s) and the copyright owner(s) are credited and that the original publication in this journal is cited, in accordance with accepted academic practice. No use, distribution or reproduction is permitted which does not comply with these terms.
*Correspondence: Shiyu Liu, c2Nob2xhcnJhaW5AMTYzLmNvbQ==