- 1College of Life Sciences, Shihezi University, Shihezi, Xinjiang, China
- 2The Key Laboratory of Industrial Biotechnology, Ministry of Education, School of Biotechnology, Jiangnan University, Wuxi, China
In this study, a two-compartment biofilm reactor was designed for iturin A production. The biofilm reactor consists of a stirred-tank fermentor containing exclusively suspended cells and a packing column where the biofilm is attached. Polyester fiber with sphere shape and rough surfaces was chosen as the carrier of biofilm in packing column. Batch, fed-batch, and repeated-batch fermentation using Bacillus velezensis ND in the biofilm reactor were studied. Compared to conventional suspended cell fermentations, the productivity of iturin A in batch and fed-batch biofilm fermentation were increased by 66.7% and 63.3%, respectively. Maximum itutin A concentration of 6.8 ± 0.1 g/L and productivity of 46.9 ± 0.2 mg/L/h were obtained in fed-batch biofilm fermentation. Repeated-batch fermentation showed high stability, with almost same profile as batch fermentation. After a step-wise temperature control strategy was introduced in the biofilm reactor, productivity of iturin A was increased by 131.9% compared to suspended cell reactor. This superior performance of biofilm reactor confirms that it has great potential in industrial production of iturin A.
Highlights
• A two-compartment biofilm reactor was constructed to produce iturin A.
• The biofilm reactor exhibited high stability for repeated-batch fermentation.
• A maximum iturin A of 6.8 ± 0.1 g/L was produced in fed-batch fermentation.
• Step-wise temperature control fermentation improved the productivity of iturin A.
Introduction
Iturin A is a cyclic lipopeptide containing seven residues of α-amino acids and one residue of a β-amino acid produced by the Bacillus strains. (Ongena and Jacques, 2008). Because of its broad-spectrum antifungal activity, iturin A is considered a powerful biopesticide in biological control of plant diseases. Iturin A has great potential as alternatives to conventional chemical pesticides in agriculture due to its low toxicity for human health and the environment (Hsieh et al., 2008; Arrebola et al., 2010; Raaijmakers et al., 2010; Velho et al., 2011; Meena and Kanwar, 2015). In addition to antimicrobial activity, insecticidal and anticarcinogenic properties of iturin A have been reported (Wan et al., 2021). Iturin A has gained more attention among the researchers for its wide application prospect in food, pharmaceutical, and agriculture.
To date, there have been many attempts to improve iturin A yield by bioprocess optimization and strain engineering (Rahman et al., 2007; Jin et al., 2015; Liang et al., 2020; Yue et al., 2021). Most researchers have employed conventional fermentation mode, submerged liquid fermentation or solid-state fermentation, for iturin A production. The production of iturin A reached 4.4 g/L (Mizumoto et al., 2007), 2.01 g/L (Xu et al., 2020) and 1.14 g/L (Chen et al., 2019) by optimizing the medium and fermentation process parameters. However, the low yield and the high production cost of iturin A still impede its large-scale production and utilization. It is worthwhile to develop novel cost-effective fermentation process to address the problem. Many Bacillus species can form rough biofilms at the air-liquid interface due to the aerotaxis of the cells. Therefore, these strains are potentially suitable for biofilm based bioprocess. Rahman et al., 2007 studied the production of iturin A by Bacillus subtilis 168 recombinant strain which was cultivated in beakers and multidish plates for biofilm formation. Compared with submerged fermentation, biofilm fermentation enhances the amount of production of iturin A and the final yield of iturin A was nearly doubled. This result revealed the potential of producing iturin A by biofilm fermentation. To date, however, there are not works on iturin A production in biofilm reactor.
Biofilm reactor allow microorganisms to self-immobilized and form a thick cell layer known as “biofilm” by providing suitable support materials (carrier). Biofilms have complex structure and provide the benefit of a stable environment for the enclosed microbes. These embedded cells exhibit different growth and bioactivity such as production of biofilm specific metabolites compared with suspended cells (Cheng et al., 2010). Other advantages of biofilm include the strong resistance of the attached biomass to toxic compound, high biomass density, as well as stability. Thereby, biofilms have great potential as industrial workhorses for the production of valued products and biofilm reactor employed in the biofilm fermentation has attracted the interest of researchers (Rosche et al., 2009; Ercan and Demirci, 2013). A number of products, such as bioethanol, organic acids, enzymes, antibiotics, vitamin, and polysaccharides, have been targeted (Khiyami et al., 2006; Demirci et al., 2007; Izmirlioglu and Demirci, 2016). In particular, several studies have investigated the production of surfactin and fengycin, the two other types of lipopeptides, in different biofilm bioreactors (Coutte et al., 2010; Chtioui et al., 2012; Chtioui et al., 2014; Brück et al., 2020). Their results showed the efficiency of the biofilm bioreactor for lipopeptides production since they provide process stability through cell immobilization and avoid foam formation. Other studies also shown that the lipopeptide productivity could be increased through cell immobilization (Brück et al., 2019). Biofilm reactors have been considered as promising systems for lipopeptides production. Thus, our effort was paid to explore the possibility of producing iturin A by biofilm reactor.
For an improved production in biofilm reactors, reactor design and the support materials are needed to be improved. In this study, a novel two-compartment biofilm reactor was designed for iturin A production. In order to increase the attachment between microorganisms and carrier, several different types of carrier were tested and the most appropriate one was selected for biofilm reactor construction. Batch, fed-batch and repeated-batch fermentation were studied to achieve a high iturin A concentration and high reactor productivity. Additionally, a step-wise temperature control strategy was introduced to further enhance the production of iturin A. The advantages of biofilm fermentation in the biofilm reactor, as compared to suspended cell fermentation, were also discussed in this article.
Materials and methods
Microorganism and growth conditions
Bacillus velezensis ND (CCTCC M 2020983, China Center for Type Culture Collection, Wuhan, China), which was isolated from a soil sample (Zhao et al., 2021), was used in this study. Strain was routinely cultured overnight in Luria-Bertani (LB) agar (15 g/L agar) plates at 37°C. The inocula were scraped and transferred into 100 mL of seed medium (pH 7.0) containing 10 g/L tryptone, 5 g/L yeast extract, and 10 g/L NaCl and cultivated at 28°C, 200 rpm for 18–20 h. Then the resulted seeds (10%, v/v) were inoculated into 50 mL of fermentation medium containing 120 g/L Soybean meal powder, 16 g/L yeast extract, 1 g/L monosodium glutamate, 88.3 g/L glycerol, 0.5 g/L MgSO4, 7H2O, 1 g/L KH2PO4, 0.15 mg/L FeSO4, 7H2O, 5 mg/L MnSO4·H2O, and 0.16 mg/L CuSO4.5H2O, and adjust the pH to 8.0 using 1 mol/L NaOH solution. The broth was incubated at 28°C in a rotary shaker with 200 rpm speed for 5 days for shake flasks fermentation.
Selection of carrier for biofilm reactors
Six different porous carriers were tested, namely P (polyester fiber material), C (porous ceramic material), Q (Ceramic material), k1, k2, and k3 (polyethylene material). The particle size of carriers P, C, Q, k1, k2, and k3 was 4.0, 3.0, 2.6, 1.0, 1.5, 2.0 cm, respectively. The specific surface area was 3,000, 1800, 1,400, 600, 660, 750 m2/m3, respectively. Carrier (approximately 60% culture medium volume) was added into shaking flask fermentation to evaluate its effects on the cell weight and iturin A yield. These 250 mL flasks containing 50 mL of the fermentation medium were incubated at 28 °C with shaking at 200 rpm for 5 days.
Two-compartment biofilm reactor construction and operation
A schematic diagram of the biofilm reactor is given in Figure 1. The system consists of two parts: (i) a 3 L stirred-tank fermentor (Baoxing Co., China) and (ii) a glass column reactor (with a diameter of 60mm and a height of 340mm) packed with carriers for biofilm formation. The glass column reactor was connected to the stirred-tank fermentor through a recirculation line. The entire reactor system contained 1 L of the medium and the liquid medium was recirculated continuously between these two parts with a flow rate of 18 mL/min via peristaltic pump. Unless otherwise noted, the stirred-tank fermentor was controlled at 28°C with stirring at 500 rpm and aeration at 3 vvm (air volume/culture volume/min). During the fermentation process, the stirred-tank reactor offers aerobic growth conditions for suspended cells and ensures a mixing of the medium. On the other hand, the broth trickles from the top of the glass column and flow down over the surface of carriers where the biofilm is attached. Then, the broth collected at the bottom of the reactor flows into the stirred-tank reactor. To control foam production, 20 mL polyether defoamer (Xinjiang Fufeng Biotechnologies Co., Ltd. China.) was added to the culture medium before fermentation. When the defoaming electrode detects foam generation in the stirred-tank fermentor during fermentation, defoamer will be automatically fed into fermentor.
Batch and fed-batch fermentations in biofilm reactor
In batch fermentation, the reactor containing 1 L of medium was inoculated with seed culture of Bacillus velezensis ND (10%, v/v), and then the fermentation broth was circulated to the connected column reactor (4/5 volume of the reactor is filled with fiber ball carrier) by peristaltic pump for 8 days. Liquid samples were taken from the stirred-tank fermentor at regular intervals for the determination of suspended cell density, residual substrate and iturin A concentration. After each sampling procedure, sterile water was added to the fermentor to restore the fermentation volume to 1 L (High stirring rates and aeration can accelerate water evaporation in the fermentation broth, which will reduce the volume of the broth and cause viscosity). Fed-batch fermentation were carried out by adding steriled glycerol (carbon source) to the fermentor. The glycerol (18 g) was supplemented (one-time addition) at 48 h and 96 h respectively, at which point glycerol was less than 28 g/L.
Batch and fed-batch fermentations in suspended cell reactor
For comparison, batch and fed-batch fermentations in the suspended cell bioreactor were carried out. Specifically, a 3 L stirred-tank fermentor containing 1 L of medium was inoculated with seed culture of Bacillus velezensis ND (10%, v/v) and fermented at 28°C. The aeration rate was controlled at 3 vvm and the stirring speed was 500 rpm. Fed-batch fermentation were carried out by adding steriled glycerol (carbon source) to the fermentor. The glycerol (18 g) was supplemented (one-time addition) at 96 h and 144 h respectively, at which point glycerol was less than 28 g/L. Biomass, yield and productivity of iturin A, carbon source consumption was compared between suspended cell and biofilm reactor.
Repeated-batch fermentation in biofilm reactor
The repeated-batch fermentation in biofilm reactor was operated by replacing the fermented broth with 1 L of fresh medium and the culture immobilized on the carrier was used as the inocula for the next batch. Other fermentation conditions were the same as described in batch fermentation in biofilm reactor. Three repeated batch fermentation were carried out to assess the stability of iturin A production by the biofilm reactor.
Batch fermentation in biofilm reactor with a step-wise temperature control strategy
The operating procedures for this method were as follows: fermentation temperature was set at 37°C from 0–24 h, 34°C from 24–36 h, 31°C from 36–48 h, and 28°C after 48 h. This strategy was used during batch fermentations in biofilm reactor. The fermentation conditions except temperature were the same as described in batch fermentation in biofilm reactor.
For comparison, batch fermentations in the suspended cell bioreactor with step-wise temperature control strategy were carried out.
Analytical methods
For the determination of cell concentration in broth, 1 mL of fermentation broth mixed with 9 mL of sterile distilled water, and shaken by a vortex. Then, the mixture was serially diluted and spread onto LB-agar plates. After 12 h of incubation at 37°C, cell concentration was determined as colony-forming units per milliliter (CFU/mL).
For the determination of cell dry weight in broth, 10 mL sample was centrifuged at 5,000 rpm for 10 min. The cell were washed twice with 50 mL distilled water and dried to constant weight at 80°C.
For the determination of cell dry weight in biofilm, the carrier was taken out from the reactor to the gauze at the end of fermentation. The biofilm was stripped from the carrier with tweezers, then the biofilm with medium was washed with PBS buffer, and was dried to constant weight at 80°C.
The concentration of glycerol was determined with a Glycerol Assay Kit (APPLYGEN Co., China). The fermentation broth was centrifuged at 5,000 rpm for 10 min, and resulting supernatant was diluted 1,000 times. 5 μl of diluent was mixed with 195 ul detection buffer of the kit. After the mixture had been incubated at 37°C for 15 min, the glycerol concentration was determined by microplate reader at 550 nm.
For the determination of the concentration of iturin A, 0.3 mL of fermentation broth was added to 0.9 mL of methanol and the mixture was vigorously shaken using a vortex mixer for 10 min. The extract was centrifuged at 12,000 rpm for 15 min, and the supernatant was used for iturin A determination. The iturin A concentration was measured on an Agilent RP-HPLC (Agilent Technologies, United States), which equipped with Agilent Lichrospher C18 column (Agilent ZORBAX Eclipse XDB-C18 column, United States). The mobile phase was10 mM ammonium acetate/acetonitrile = (65:35, v/v), and flow rate was 1.0 mL/min. The injection volume was 20 μl and detection wavelength was 210 nm, and concentration of iturin A was calculated by the standard curve made by standard iturin A (purity 95%, Sigma-Aldrich United States).
For the determination of iturin A adsorbed on carrier, the carrier was taken out from the reactor at the end of fermentation and biofilm was rinsed thoroughly with 1,000 mL distilled water. Then, the mixture of biofilm and water was vigorously vortexed for 30 min to facilitate the disintegration of bacterial clumps and was used to extracted iturin A. The iturin A extraction and assay were performed as described above.
All assays were performed at least in triplicate, and data represent the mean of three or more experiments. All observations were repeated and the average values were obtained and demonstrated with standard errors of the repetitions as error bars. Using Origin 2018 ANOVA, any difference with p < 0.05 was considered significant.
Results
Selection of suitable carriers
Six different types of carrier were tested for their effects on the production of iturin A and cell growth so as to find a suitable carrier. As shown in Figure 2, P, C, k1, k2, and k3 demonstrated a significant promotion effect on cell growth and iturin A production (p < 0.05). This may be attributed to two factors: i) the carrier vibrated in a shake flask, promoting the oxygen and nutrient transfers in broth. ii) Biofilm formed gradually at the surface of these carriers, resulting in a higher amount of biomass. Unlike these carriers, Q appeared to have an inhibitory effect upon cell growth and biofilm was not observed at the surface. The reason of this unexpected observation was still unclear. It was probably due to unknown component of carrier Q which was made of natural raw materials. On the other hand, carrier P yielded the highest iturin A concentration (4.7 ± 0.1 g/L) and biomass (6.5 ± 0.1 g/L). These values were 10.6% and 22.3% greater than the control group (4.3 ± 0.1 and 5.3 ± 0.1 g/L, respectively). The better performance of P could be attributed to its larger specific area resulting from structural differences among the carriers. For these reasons, carrier P was selected as the most suitable packing for cell immobilization in biofilm reactor system and was used in the following experiments.
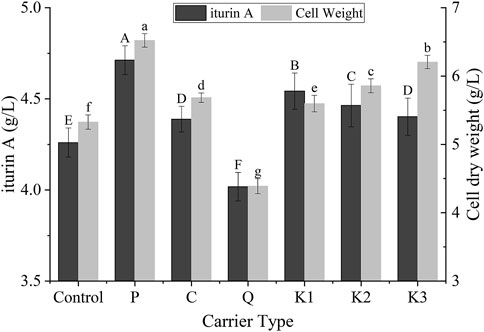
FIGURE 2. Effects of different carriers on the cell growth and the production of iturin A in B. velezensis ND in the shake-flask scale. Different letters meant there was significant difference among groups (p < 0.05).
Batch and fed-batch fermentations for iturin A production in the biofilm reactor
Batch fermentation was first performed to investigate the effectiveness of iturin A production by using the biofilm reactor. For comparison, batch and fed-batch fermentations in the suspended cell bioreactor were also carried out. Figure 3 shows the time courses of the biomass, residual glycerol and the concentration of iturin A in biofilm reactor and suspended cell reactor. As showing in Figure 3A, cell started growing rapidly both in suspended cell reactor and biofilm reactor during culture (0–72 h), and the maximum growth rate is almost the same. At 96 h of culture, suspended cell reactor achieved a highest biomass (184.0 ± 7.9 × 108 CFU/mL) which is higher than biofilm reactor (137.3 ± 2.9 × 108 CFU/mL). However, it should be noted that the biomass only represented growth in the broth and not in the biofilm. Actually, biofilm formed gradually in biofilm reactor and the total amount of biomass in broth and biofilm is higher than that in suspended cell reactor (Table 1). In both reactors, there was a rapid decrease in cell density after the culture reached its maximum concentration value, however, the iturin A production continued. This result indicated that iturin A is a non-growth-associated product. In biofilm reactor, faster glycerol consumption was observed and iturin A concentration reached maximum levels at the same time as the depletion of glycerol (Figure 3B). Figure 3C shows that iturin A concentration reached its maximum at 5.4 ± 0.1 g/L after 144 h in biofilm reactor and 4.6 ± 0.1 g/L after 192 h in suspended cell reactor. This implies that biofilm fermentation increases the yield by 17.3% and the productivity by 56.4% compared with suspended cell fermentation. In addition, 355.1 ± 31.7 mg iturin A was obtained from the biofilm on the carrier so that the total amount of iturin A in biofilm reactor was 5.7 ± 0.1 g/L which increased by 25.2% compared with the suspended cell fermentation.
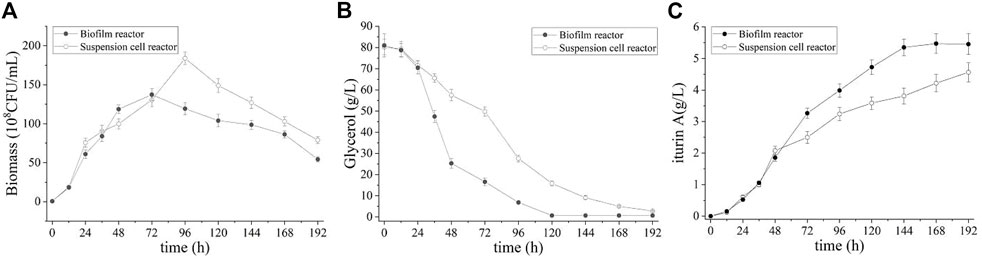
FIGURE 3. Time course of biomass (A), residual glycerol (B) and iturin A production (C) during the batch fermentation in suspension cell reactor and biofilm reactor.
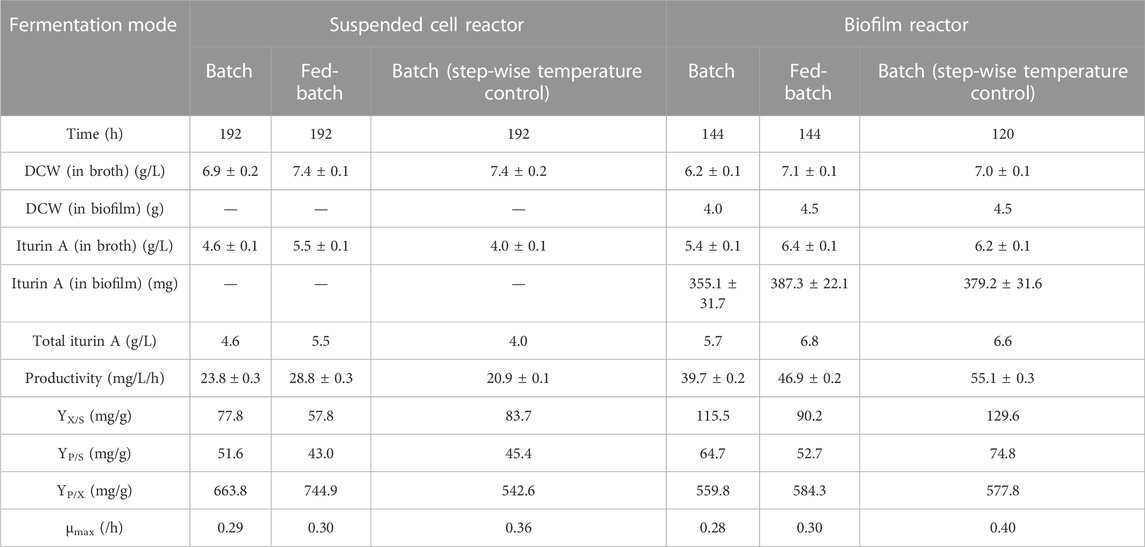
TABLE 1. Comparison of batch and fed-batch fermentation of Bacillus velezensis ND in suspended cell reactor and biofilm reactor. Productivity was calculated as total iturin A produced (mg/L) in a given time (h). DCW: Cell dry weight; YX/S:Yield of biomass per glycerol; YP/S:Yield of iturin A per carbon source; YP/X:Yield of iturin A per biomass; μmax (in broth): Maximum specific growth rate.
The evidence of substrate limitation occurred in batch fermentation and substrate inhibition present at high glycerol level (data not shown) indicated the appropriateness of fed-batch fermentation. Figure 4 shows the time course of fed-batch fermentation in biofilm reactor and suspended cell reactor. The glycerol was supplemented in biofilm reactor at 48 h and 96 h respectively, at which point glycerol was less than 28 g/L. For suspended cell reactor, the glycerol comsuption is slower and glycerol was supplemented at 96 h and 144 h respectively. As showing in Figure 4A, the feeding of glycerol prolonged the cell growth phase and achieved highest biomass at 144 h in both reactor. Accordingly, a higher cell yield than batch process in both biofilm and suspended cell reactor (166.7 ± 4.7×108 and 237.3 ± 5.8 × 108 CFU/mL, respectively) was achieved. As shown in Figure 4C, iturin A reached it maximum at 6.8 ± 0.1 g/L after 144 h in biofilm reactor while 5.5 ± 0.1 g/L after 192 h in suspended cell reactor. Compared with batch process, the yield of iturin A is further improved.
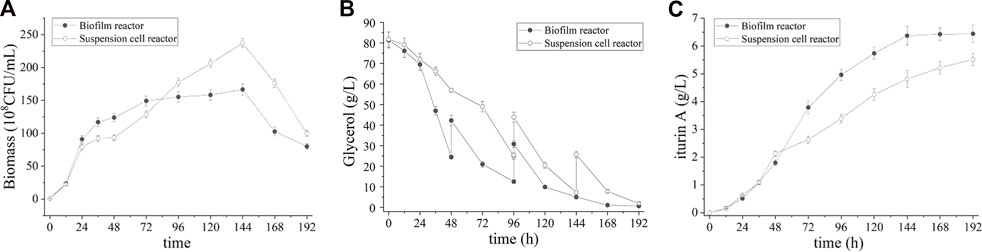
FIGURE 4. Time course of biomass (A), residual glycerol (B) and iturin A production (C) during the fed-batch fermentation in suspension cell reactor and biofilm reactor.
Repeated-batch fermentation in the biofilm reactor
Three cycles of repeated-batch fermentation were carried out in order to validate the stability of biofilm and long-term performance of the biofilm reactor. As shown in Figure 5, the profile of each fermentation cycle is nearly the same. Specific data of three cycles of repeated-batch fermentation are shown in Table 2. The yield of iturin A in three batches was 5.4 g/L, 5.3 g/L and 5.3 g/L, respectively, and the cells dry weight at the end of each batch increased gradually from 6.3 g/L to 6.9 g/L. Also, the trend of cell growth, substrate consumption and iturin A production in repeated-batch fermentation were nearly the same as single batch fermentation in biofilm reactor. These results indicated that the biofilm on the carrier could maintain their long-term stability, providing the seeds for the next batch. It should be noted that the repeated-batch process omitted inoculation requirements between fermentation cycles and saved costs for seed culture, but it did not showed a shorter period of fermentation and a higher productivity than single batch fermentation. However, long-term stability of biofilm in carrier implied a possibility of continuous fermentation process and the yield of iturin A could be further improved through continuous fermentation.
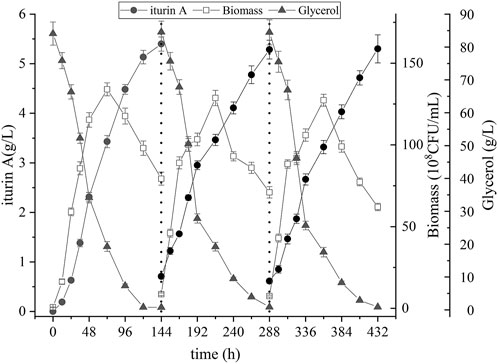
FIGURE 5. Time course of biomass, residual glycerol, and iturin A production during the repeated-batch fermentation in biofilm reactor.
Batch fermentations in the biofilm reactor with a step-wise temperature control strategy
According to our previous study of this strain, the optimal temperature for good cell growth is 37 °C while the optimal temperature for iturin A production is 28°C (data not shown). To address the inconsistent temperature in cell growth and iturin A production, a step-wise temperature control strategy was introduced in this study. During the first 24 h of cultivation, the temperature was set at 37°C for good cell growth. Subsequently, the temperature was decreased step-wise to 28°C over the next 24 h and maintained until the end of fermentation, favoring the production of iturin A. The result of batch fermentation was shown in Figure 6. The cell density in broth increased rapidly during the first 24 h and slow down as temperature decreased (24–48 h), reaching its highest value at 48 h (249.3 ± 8.5 × 108 CFU/mL) (Figure 6A). This value is 81.8% higher than that of batch process without step-wise temperature control strategy. Meanwhile, glycerol was also consumed at a rapid rate and nearly depleted at 72 h (Figure 6B). The highest level of iturin A was achieved (6.6 ± 0.1 g/L) at 120 h (Figure 6C). These results clearly indicated that the fermentation period was shortened and the productivity (55.1 ± 0.3 mg/L/h) was greatly improved by the step-wise temperature control strategy.
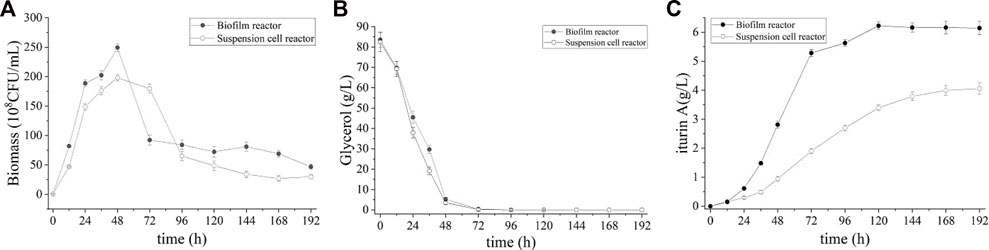
FIGURE 6. Time course of biomass (A), residual glycerol (B), and iturin A production (C) during the batch fermentation in suspension cell reactor and biofilm reactor with a step-wise temperature control strategy.
Interestingly, the step-wise temperature control strategy did not work in the suspended cell fermentation. During the culture (0–48 h), suspended cell reactor showed a faster cell growth than process without step-wise temperature control but the maximum biomass showed only a small increase (198.0 ± 8.1 × 108 CFU/mL vs. 184.0 ± 7.9 × 108 CFU/mL). At the same time, the consumption rate of glycerol was also increased, accompanied by the acceleration of cell growth. Glycerol was also depleted at 72 h and the cells entered decline phase prematurely in the suspended cell reactor. Although the same glycerol consumption rate was observed, the highest iturin A concentration in the suspended cell fermentation was only 4.0 ± 0.1 g/L after 196 h which is lower than process without step-wise temperature control.
Discussion
Biofilm growth is initiated by cell attachment to a surface of carrier, followed by surface adhesion by extracellular polymer matrix and colonization (Burmolle et al., 2006; Xu et al., 2011). Generally, porous materials with rough surface and a larger surface area work well for biofilm formation. In addition, surface properties of carrier, such as surface charge, hydrophobicity, particle diameter, and density, also play an essential role in initial cell attachment (Cheng et al., 2010). Therefore, it is necessary to choose the suitable carrier for different types of biofilm reactors and microorganisms, which can increase the attachment between microorganisms and carrier. A great variety of materials, such as stainless steel, lignocellulosic materials, polymeric materials, glass ceramic material, plastic composite, have been developed and designed for various types of biofilm reactors (Chu et al., 2014; Gu et al., 2014; Ercan and Demirci 2015; Jiang et al., 2016; Mahdinia et al., 2017; Morgan-Sagastume, 2018).
In this study, six carriers with different surface properties and structure were tested. It has been observed that not all the carriers are suitable for the Bacillus velezensis ND strain. Of all carriers, P (spherical filter material made of polyester fiber) showed the best performance. Thick cell layer was observed on the surface of P. It has been reported that the high degree of hydrophobicity strongly enhances adhesion of microorganisms (Silva-Dias et al., 2015; Elegbeleye and Buys, 2020). An additional aspect worthy of consideration is that iturin A secreted by Bacillus velezensis ND have relatively high amphiphilic (Meena and Kanwar, 2015). Therefore, it is reasonable to presume that the P with high degree of hydrophobicity is suitable for adhesion of Bacillus velezensis ND. The other characteristics of P are the roughness and large specific surface area (3,000 m2/m3). Moreover, P has the advantages of readily available, inexpensive, and reusable. For these reasons, P was chosen to construct the biofilm reactor after the pilot trials in shaking flasks. Its reusability was also validated in the biofilm reactor (detailed data not shown). The carrier were reused for the next batch after cleaned and dried, and the yield of iturin A was almost no change (5.4 ± 0.1 g/L). Reusability of the carrier lowers the cost of biofilm fermentation, which is important for industrial use.
The biofilm reactor we have constructed consists of a stirred-tank fermentor containing exclusively suspended cells and a packing column where the biofilm is attached. In this system, suspended cells and biofilm are co-existed. The packing column allow biofilm growth on static surfaces with low surface shear forces while the stirred-tank fermentor offers aerobic growth conditions for suspended cells. In the packing column, the liquid medium recirculated from the stirred bioreactor continuously flows down the carriers as a thin film to provide maximum gas-liquid contact, and nutrients supply for biofilm. In the stirred-tank fermentor, aerobic growth conditions support rapid proliferation of suspended cells. In brief, this system is designed to provide ideal growth conditions for suspended and biofilm population simultaneously so that higher biomass could be achieved.
During the biofilm fermentation, cell absorption to carrier was effective, and biofilm on the carrier was observed in 36–48 h. Maximum specific growth rate of both reactor were nearly the same. The total amount of biomass (in broth and biofilm) in biofilm reactor is considerably higher than suspended cell reactor (Table 1). The development of biofilm facilitated the utilization of glycerol as well as the production of iturin A. As a result, the fermentation period was shortened and volumetric productivity was greatly improved than suspended cell fermentation. Table 1 lists the important parameters of different fermentation process. The productivity and yield are most important parameters determining the economic feasibility of any bioprocess. The productivity of iturin A was increased by 66.7% and 63.3% in batch and fed-batch biofilm fermentation, respectively. The yield (YP/S and YX/S) in biofilm reactor also showed obvious increase, which indicated that biofilm reactor has more effective carbon source (glycerol) utilization than suspended cell reactor. However, suspended cell reactor achieved higher YP/X value than biofilm reactor, which implied that cells that were involved in iturin A production were only a fraction of the total cells in biofilm reactor. Usually biofilms contain multiple layers of cells. In order for the cells to be active and be taking part in the reaction, nutrients must diffuse to the inner layers of cells (Qureshi et al., 2005). Therefore, it is possible that he innermost layers of biofilm have no sufficient nutrients or oxygen and did not take part in the reaction. In order to increase substrates diffusion, further optimization for medium components and flow rate of fermentation broth is necessary.
The other advantage of biofilm reactor over suspended cell reactors is the operation stability, especially in repeated-batch fermentation. When medium is removed and fresh medium introduced, cells released from mature biofilms served as the seeds for the next batch. Repeated-batch fermentation has been shown to enhance the productivity of microbial fermentations, as it reduces the cost for seed culture, and omits inoculation requirements between batches (Qu et al., 2013; Jiang et al., 2016). The advantage have the potential to lead to significant savings in terms of both time and labour (Germec et al., 2015). As shown in this study, repeated-batch fermentation in the biofilm reactor was continuous, efficient, and stable, which is important for cost-saving and achieving industrial-scale production of iturin A.
Temperature is an important factor in fermentation. Each microbial species have their optimal growth temperature while the optimal temperature for the production of secondary metabolites may vary. In addition, temperature have an effect on the rheological properties of fermentation broth and biofilm formation. Temperatures at the high end of a culture’s growth range increase the rate of cell growth, EPS production, and surface adhesion, all of which enhance biofilm formation (Di Ciccio et al., 2015). Our results showed that higher temperature during the early time of fermentation lead to faster cell growth and the maximum specific growth rate (μmax) reached 0.4 h−1. The cell dry weight in both broth and biofilm increased and the fermentation period was shortened under the step-wise temperature control strategy. As a result, the productivity and yield (YP/S, YX/S and YP/X) were improved significantly (Table 1).
However, it is worth noting that the step-wise temperature control strategy did not work in the suspended cell reactor. The rate of cell growth and glycerol consumption increased but iturin A production decreased in the suspended cell fermentation. Biofilms are well known for their long-term activity and enhanced tolerance to toxic substances and other adverse conditions compared to suspended cells (Rosche et al., 2009). Therefore, this might be explained as follows: higher temperature result in faster growth and increased biomass so that the cells entered decline phase prematurely in the suspended cell reactor, while biofilm formation contribute to the maintenance of cell viability in the biofilm reactor. As a result, the step-wise temperature control strategy led to a 131.9% increase of iturin A productivity in batch biofilm fermentation than suspended cell fermentation.
No comparable value of iturin A production in biofilm reactor has been published in the literature. The parameters of iturin A was compared with other lipopeptides production in biofilm bioreactors (Table 3). The obtained iturin A productivity and yield (YP/S and YP/X) in this study was higher than that reported for rotating discs bioreactor and bubbleless membrane reactor. This may be attributed to better developed biofilm which resulted the higher biomass ratio of biofilm vs planktonic cells (0.64) in this study. Actually, the biomass ratio of biofilm vs planktonic cells in the rotating discs bioreactor and bubbleless membrane reactor was 0.12 and 0.04, respectively, which indicated that most of the cells were planktonic cells (ratio was calculated based on the data given in the references). The biomass ratio of biofilm vs planktonic cells in the biofilm reactor reported by Brück et al. reached 2.1 and this could be one reason for its relatively higher surfactin productivity and yield. However, it should be mentioned that the productivity and yield are affected by many factor, such as strain, substrate, oxygen supply and other conditions during fermentation process.
In conclusion, a novel two-compartment biofilm reactor is designed and constructed. Polyester fiber with sphere shape was chosen as the carrier of biofilm because of having high degree of hydrophobicity and rough surfaces. In this biofilm reactor, higher biomass could be achieved because of ideal growth conditions for suspended cell and biofilm population simultaneously. The fermentation period was shortened and volumetric productivity was greatly improved than suspended cell fermentation. Compared to suspended cell fermentations, the productivity of iturin A in batch and fed-batch biofilm fermentation were increased by 66.7% and 63.3%, respectively. The step-wise temperature control strategy resulted in 131.9% increase of iturin A productivity compared to suspended cell fermentation.
Data availability statement
The original contributions presented in the study are included in the article/Supplementary Material, further inquiries can be directed to the corresponding author.
Author contributions
ZT conducted experiments and wrote an original draft of the manuscript. HZ provided the funding and equipment and edited the manuscript. YL, JX, and WL provided comments, and edited and reviewed the final manuscript. All authors read and approved the manuscript.
Funding
This study was funded by the National Natural Science Foundation of China Regional Science Foundation project (21366028).
Conflict of interest
The authors declare that the research was conducted in the absence of any commercial or financial relationships that could be construed as a potential conflict of interest.
Publisher’s note
All claims expressed in this article are solely those of the authors and do not necessarily represent those of their affiliated organizations, or those of the publisher, the editors and the reviewers. Any product that may be evaluated in this article, or claim that may be made by its manufacturer, is not guaranteed or endorsed by the publisher.
Supplementary material
The Supplementary Material for this article can be found online at: https://www.frontiersin.org/articles/10.3389/fbioe.2023.1102786/full#supplementary-material
References
Arrebola, E., Jacobs, R., and Korsten, L. (2010). Iturin A is the principal inhibitor in the biocontrol activity of Bacillus amyloliquefaciens PPCB004 against postharvest fungal pathogens. J. Appl. Microbiol. 108 (2), 386–395. doi:10.1111/j.1365-2672.2009.04438.x
Brück, H. L., Coutte, F., Dhulster, P., Gofflot, S., Jacques, P., and Delvigne, F. (2020). Growth dynamics of bacterial populations in a two-compartment biofilm bioreactor designed for continuous surfactin biosynthesis. Microorganisms 8 (5), 679. doi:10.3390/microorganisms8050679
Brück, H. L., Delvigne, F., Dhulster, P., Jacques, P., and Coutte, F. (2019). Molecular strategies for adapting Bacillus subtilis 168 biosurfactant production to biofilm cultivation mode. Bioresour. Technol. 293, 122090. doi:10.1016/j.biortech.2019.122090
Burmolle, M., Webb, J. S., Rao, D., Hansen, L. H., Sorensen, S. J., and Kjelleberg, S. (2006). Enhanced biofilm formation and increased resistance to antimicrobial agents and bacterial invasion are caused by synergistic interactions in multispecies biofilms. Appl. Environ. Microb. 72 (6), 3916–3923. doi:10.1128/aem.03022-05
Chen, W., Li, X., Ma, X., Chen, S., Kang, Y., Yang, M., et al. (2019). Simultaneous hydrolysis with lipase and fermentation of rapeseed cake for iturin A production by Bacillus amyloliquefaciens CX-20. BMC Biotechnol. 19 (1), 98–10. doi:10.1186/s12896-019-0591-x
Chtioui, O., Dimitrov, K., Gancel, F., Dhulster, P., and Nikov, I. (2012). Rotating discs bioreactor, a new tool for lipopeptides production. Process Biochem. 47 (12), 2020–2024. doi:10.1016/j.procbio.2012.07.013
Chtioui, O., Dimitrov, K., Gancel, F., Dhulster, P., and Nikov, I. (2014). Selective fengycin production in a modified rotating discs bioreactor. Bioprocess Biosyst. Eng. 37 (2), 107–114. doi:10.1007/s00449-013-0964-9
Chu, L., Wang, J., Quan, F., Xing, X-H., Tang, L., and Zhang, C. (2014). Modification of polyurethane foam carriers and application in a moving bed biofilm reactor. Process Biochem. 49 (11), 1979–1982. doi:10.1016/j.procbio.2014.07.018
Coutte, F., Lecouturier, D., Ait Yahia, S., Leclère, V., Béchet, M., Jacques, P., et al. (2010). Production of surfactin and fengycin by Bacillus subtilis in a bubbleless membrane bioreactor. Appl. Microbiol. Biotechnol. 87 (2), 499–507. doi:10.1007/s00253-010-2504-8
Demirci, A., Pongtharangkul, T., and Pometto, A. (2007). Applications of biofilm reactors for production of value-added products by microbial fermentation. Biofilms Food Environ. 2, 167–190. doi:10.1002/9780470277782.ch8
Di Ciccio, P., Vergara, A., Festino, A. R., Paludi, D., Zanardi, E., Ghidini, S., et al. (2015). Biofilm formation by Staphylococcus aureus on food contact surfaces: Relationship with temperature and cell surface hydrophobicity. Food 50, 930–936. doi:10.1016/j.foodcont.2014.10.048
Elegbeleye, J. A., and Buys, E. M. (2020). Molecular characterization and biofilm formation potential of Bacillus subtilis and Bacillus velezensis in extended shelf-life milk processing line. J. dairy Sci. 103 (6), 4991–5002. doi:10.3168/jds.2019-17919
Ercan, D., and Demirci, A. (2015). Current and future trends for biofilm reactors for fermentation processes. Crit. Rev. Biotechnol. 35 (1), 1–14. doi:10.3109/07388551.2013.793170
Ercan, D., and Demirci, A. (2013). Production of human lysozyme in biofilm reactor and optimization of growth parameters of Kluyveromyces lactis K7. Appl. Microbiol. Biot. 97 (14), 6211–6221. doi:10.1007/s00253-013-4944-4
Germec, M., Turhan, I., Karhan, M., and Demirci, A. (2015). Ethanol production via repeated-batch fermentation from carob pod extract by using Saccharomyces cerevisiae in biofilm reactor. Fuel 161, 304–311. doi:10.1016/j.fuel.2015.08.060
Gu, Q., Sun, T., Wu, G., Li, M., and Qiu, W. (2014). Influence of carrier filling ratio on the performance of moving bed biofilm reactor in treating coking wastewater. Bioresour. Technol. 166, 72–78. doi:10.1016/j.biortech.2014.05.026
Hsieh, F. C., Lin, T. C., Meng, M., and Kao, S. S. (2008). Comparing methods for identifying Bacillus strains capable of producing the antifungal lipopeptide iturin A. Curr. Microbiol. 56 (1), 1–5. doi:10.1007/s00284-007-9003-x
Izmirlioglu, G., and Demirci, A. (2016). Ethanol production in biofilm reactors from potato waste hydrolysate and optimization of growth parameters for Saccharomyces cerevisiae. Fuel 181, 643–651. doi:10.1016/j.fuel.2016.05.047
Jiang, Y., Tang, B., Xu, Z., Liu, K., Xu, Z., Feng, X., et al. (2016). Improvement of poly-gamma-glutamic acid biosynthesis in a moving bed biofilm reactor by Bacillus subtilis NX-2. Bioresour. Technol. 218, 360–366. doi:10.1016/j.biortech.2016.06.103
Jin, H., Li, K., Niu, Y., Guo, M., Hu, C., Chen, S., et al. (2015). Continuous enhancement of iturin A production by Bacillus subtilis with a stepwise two-stage glucose feeding strategy. BMC Biotechnol. 15, 53. doi:10.1186/s12896-015-0172-6
Khiyami, M. A., Pometto, A. L., and Kennedy, W. J. (2006). Ligninolytic enzyme production by phanerochaete chrysosporium in plastic composite support biofilm stirred tank bioreactors. J. Agr. Food Chem. 54 (5), 1693–1698. doi:10.1021/jf051424l
Liang, C., Ding, S., Sun, W., Liu, L., Zhao, W., Zhang, D., et al. (2020). Biofilm-based fermentation: A novel immobilisation strategy for Saccharomyces cerevisiae cell cycle progression during ethanol production. Appl. Microbiol. Biot. 104 (17), 7495–7505. doi:10.1007/s00253-020-10770-1
Mahdinia, E., Demirci, A., and Berenjian, A. (2017). Strain and plastic composite support (PCS) selection for vitamin K (Menaquinone-7) production in biofilm reactors. Bioproc Biosyst. Eng. 40 (10), 1507–1517. doi:10.1007/s00449-017-1807-x
Meena, K. R., and Kanwar, S. S. (2015). Lipopeptides as the antifungal and antibacterial agents: Applications in food safety and therapeutics. Biomed. Res. Int. 2015, 1–9. doi:10.1155/2015/473050
Mizumoto, S., Hirai, M., and Shoda, M. (2007). Enhanced iturin A production by Bacillus subtilis and its effect on suppression of the plant pathogen Rhizoctonia solani. Appl. Microbiol. Biot. 75 (6), 1267–1274. doi:10.1007/s00253-007-0973-1
Morgan-Sagastume, F. (2018). Biofilm development, activity and the modification of carrier material surface properties in moving-bed biofilm reactors (MBBRs) for wastewater treatment. Crit. Rev. Env. Sci. Tec. 48 (5), 439–470. doi:10.1080/10643389.2018.1465759
Ongena, M., and Jacques, P. (2008). Bacillus lipopeptides: Versatile weapons for plant disease biocontrol. Trends Microbiol. 16, 115–125. doi:10.1016/j.tim.2007.12.009
Qu, L., Ren, L. J., Sun, G. N., Ji, X. J., Nie, Z. K., and Huang, H. (2013). Batch, fed-batch and repeated fed-batch fermentation processes of the marine thraustochytrid Schizochytrium sp. for producing docosahexaenoic acid. Bioproc Biosyst. Eng. 36 (12), 1905–1912. doi:10.1007/s00449-013-0966-7
Qureshi, N., Annous, B. A., Ezeji, T. C., Karcher, P., and Maddox, I. S. (2005). Biofilm reactors for industrial bioconversion processes: Employing potential of enhanced reaction rates. Microb. Cell factories 4 (1), 24–21. doi:10.1186/1475-2859-4-24
Raaijmakers, J. M., De Bruijn, I., Nybroe, O., and Ongena, M. (2010). Natural functions of lipopeptides from Bacillus and Pseudomonas: More than surfactants and antibiotics. Fems Microbiol. Rev34 (6), 1037–1062. doi:10.1111/j.1574-6976.2010.00221.x
Rahman, M. S., Ano, T., and Shoda, M. (2007). Biofilm fermentation of iturin A by a recombinant strain of Bacillus subtilis 168. J. Biotechnol. 127 (3), 503–507. doi:10.1016/j.jbiotec.2006.07.013
Rosche, B., Li, X. Z., Hauer, B., Schmid, A., and Buehler, K. (2009). Microbial biofilms: A concept for industrial catalysis? Trends Biotechnol. 27 (11), 636–643. doi:10.1016/j.tibtech.2009.08.001
Shin, I. L., Lin, C. Y., Wu, J. Y., and Hsieh, C. (2009). Production of antifungal lipopeptide from Bacillus subtilis in submerged fermentation using shake flask and fermentor. Korean J. Chem. Eng. 26 (6), 1652–1661. doi:10.1007/s11814-009-0237-0
Silva-Dias, A., Miranda, I. M., Branco, J., Monteiro-Soares, M., Pina-Vaz, C., and Rodrigues, A. G. (2015). Adhesion, biofilm formation, cell surface hydrophobicity, and antifungal planktonic susceptibility: Relationship among Candida spp. Front. Microbiol. 6, 205. doi:10.3389/fmicb.2015.00205
Velho, R. V., Medina, L. F. C., Segalin, J., and Brandelli, A. (2011). Production of lipopeptides among Bacillus strains showing growth inhibition of phytopathogenic fungi. Folia Microbiol. 56 (4), 297–303. doi:10.1007/s12223-011-0056-7
Wan, C., Fan, X., Lou, Z., Wang, H., Olatunde, A., and Rengasamy, K. R. R. (2021). Iturin: Cyclic lipopeptide with multifunction biological potential. Crit. Rev. Food Sci. Nutr. 62, 7976–7988. doi:10.1080/10408398.2021.1922355
Xu, H., Lee, H. Y., and Ahn, J. (2011). Characteristics of biofilm formation by selected foodborne pathogens. J. Food Saf. 31 (1), 91–97. doi:10.1111/j.1745-4565.2010.00271.x
Xu, Y., Cai, D., Zhang, H., Gao, L., Yang, Y., Gao, J., et al. (2020). Enhanced production of iturin A in Bacillus amyloliquefaciens by genetic engineering and medium optimization. Process Biochem. 90, 50–57. doi:10.1016/j.procbio.2019.11.017
Yue, H., Zhong, J., Li, Z., Zhou, J., Yang, J., Wei, H., et al. (2021). Optimization of iturin A production from Bacillus subtilis ZK-H2 in submerge fermentation by response surface methodology. 3 Biotech. 11 (2), 36. doi:10.1007/s13205-020-02540-7
Zhao, C., Wan, Y., Tang, G., Jin, Q., Zhang, H., and Xu, Z. (2021). Comparison of different fermentation processes for the vitamin K2 (Menaquinone-7) production by a novel Bacillus velezensis ND strain. Process Biochem. 102, 33–41. doi:10.1016/j.procbio.2020.11.029
Keywords: biofilm reactor, iturin A, Bacillus velezensis ND, batch fermentation, step-wise temperature control
Citation: Tang Z, Zhang H, Xiong J, Li Y and Luo W (2023) Enhanced iturin a production in a two-compartment biofilm reactor by Bacillus velezensis ND. Front. Bioeng. Biotechnol. 11:1102786. doi: 10.3389/fbioe.2023.1102786
Received: 19 November 2022; Accepted: 09 January 2023;
Published: 19 January 2023.
Edited by:
Eric D. van Hullebusch, UMR7154 Institut de Physique du Globe de Paris (IPGP), FranceReviewed by:
François Coutte, Université de Lille, FranceJianguo Zhang, University of Shanghai for Science and Technology, China
Copyright © 2023 Tang, Zhang, Xiong, Li and Luo. This is an open-access article distributed under the terms of the Creative Commons Attribution License (CC BY). The use, distribution or reproduction in other forums is permitted, provided the original author(s) and the copyright owner(s) are credited and that the original publication in this journal is cited, in accordance with accepted academic practice. No use, distribution or reproduction is permitted which does not comply with these terms.
*Correspondence: Huili Zhang, emhhbmdoY2gwMkAxMjYuY29t
†ORCID:Zhongmin Tang, 0000-0003-3977-6167