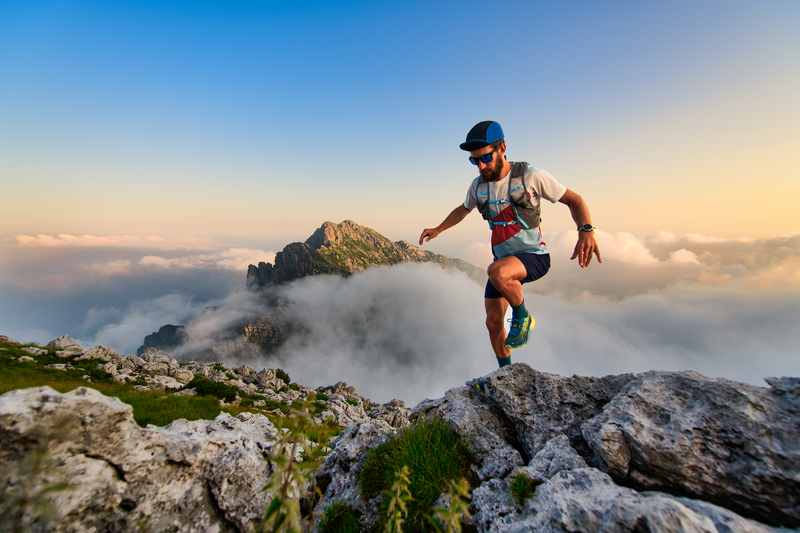
95% of researchers rate our articles as excellent or good
Learn more about the work of our research integrity team to safeguard the quality of each article we publish.
Find out more
ORIGINAL RESEARCH article
Front. Bioeng. Biotechnol. , 16 January 2023
Sec. Synthetic Biology
Volume 11 - 2023 | https://doi.org/10.3389/fbioe.2023.1091899
This article is part of the Research Topic Synthetic Metabolism for the Third-Generation (3G) Biorefineries View all 5 articles
Microbial C1 fixation has a vast potential to support a sustainable circular economy. Hence, several biotechnologically important microorganisms have been recently engineered for fixing C1 substrates. However, reports about C1-based bioproduction with these organisms are scarce. Here, we describe the optimization of a previously engineered formatotrophic Escherichia coli strain. Short-term adaptive laboratory evolution enhanced biomass yield and accelerated growth of formatotrophic E. coli to 3.3 g-CDW/mol-formate and 6 h doubling time, respectively. Genome sequence analysis revealed that manipulation of acetate metabolism is the reason for better growth performance, verified by subsequent reverse engineering of the parental E. coli strain. Moreover, the improved strain is capable of growing to an OD600 of 22 in bioreactor fed-batch experiments, highlighting its potential use for industrial bioprocesses. Finally, demonstrating the strain’s potential to support a sustainable, formate-based bioeconomy, lactate production from formate was engineered. The optimized strain generated 1.2 mM lactate —10% of the theoretical maximum— providing the first proof-of-concept application of the reductive glycine pathway for bioproduction.
The valorization of carbon dioxide is a major challenge in our society and is subject to intense research and investment. Naturally, the biological transformation of carbon dioxide takes place in plants and algae. However, photosynthesis greatly suffers from low-energy conversion efficiencies in the range of 3%–5% (Janssen et al., 2003; Zhu et al., 2010). Pure chemical transformation, where various chemicals such as urea, methanol, and salicylic acid can be derived directly from carbon dioxide, might be another option (He et al., 2013; Wong, 2014). However, the processes require extreme conditions and their product spectrum and product selectivity are limited. A promising solution is to combine the individual strengths of biological and chemical processes to circumvent their weaknesses. In such an approach, carbon dioxide can be reduced electrochemically, using renewable energy sources, to various C1 compounds. Among them is formic acid, which can be produced with a very high faradaic efficiency and can be utilized as a feedstock for microorganisms (Li et al., 2012; Liu et al., 2016; Yishai et al., 2016). Formic acid is one of the most suitable C1 compounds for the bioindustry, especially because of its solubility and low toxicity (Claassens et al., 2019).
However, engineering natural C1-assimilating microorganisms to produce value-added biochemicals from single-carbon compounds is often limited by their poor growth characteristics and recalcitrance to genetic modification. Various natural C1 assimilation routes have been identified, including the reductive pentose phosphate cycle, the reductive acetyl-CoA pathway, and the reductive citric acid cycle from various domains of life (Berg et al., 2010; Bar-Even et al., 2012a; Bar-Even et al., 2012b). Nature has optimized these microorganisms, enzymes, and metabolic fluxes over billions of years, rendering attempts to improve energy consumption and carbon-fixation efficiency very challenging. Implementing natural or new-to-nature synthetic pathways for C1 assimilation into biotechnologically important microbes such as E. coli, which do not naturally grow on C1 compounds, can solve these problems. Assimilation of C1 compounds such as CO2, formate, CO, or methanol via their respective assimilation pathways has been receiving increased attention (Schwander et al., 2016; Meyer et al., 2018; Gleizer et al., 2019; Bang et al., 2020; Chen et al., 2020; Gassler et al., 2020). Besides the reductive acetyl-CoA pathway, the synthetic and oxygen-tolerant reductive glycine (rGly) pathway is the most efficient formate assimilation pathway (Bar-Even et al., 2013; Claassens et al., 2022). This pathway was recently successfully engineered in E. coli for generation of all biomass from formate and CO2 (Figure 1), reaching a doubling time of 9 h and a biomass yield of 2.3 g cell dry weight/mol (CDW/mol) formate (Kim et al., 2020). However, so far bioproduction via synthetic C1-assimilation pathways has not been reported.
FIGURE 1. Reductive glycine pathway as operating in the formatotrophic E. coli strain. Displayed is formate and CO2 conversion to lactate as a final bioproduct.
In this work, growth performance of the formatotrophic E. coli strain was improved using an adaptive laboratory evolution approach on formate under 10% CO2 atmosphere. Genome sequencing identified mutations that apparently increased the growth performance on formate, and their effects were verified through reverse engineering. Finally, we demonstrate high biomass production in a fed-batch experiment and engineer lactate production from formate by an optimized E. coli strain.
Primers were ordered from IDT (Leuven, Belgium). PCR reactions were performed using either Phusion High-Fidelity DNA Polymerase for gene amplification or DreamTaq DNA Polymerase for colony PCR (Thermo Fisher Scientific, Dreieich, Germany). Restriction digestions were carried out using FastDigest restriction enzymes and for ligation reactions T4 DNA ligase was used (all from Thermo Fisher Scientific). Sodium formate was ordered from Sigma-Aldrich (Steinheim, Germany).
The formatotrophic Escherichia coli strain [based on E. coli MG1655 (F− λ− ilvG− rfb-50 rph-1)] equipped with reductive glycine pathway (K4e) (Kim et al., 2020) was used as base strain for adaptive evolution and reverse engineering. E. coli DH5α (F− λ− Φ80lacZΔM15 Δ(lacZYA-argF)U169 deoR, recA1 endA1, hsdR17(rK− mK+) phoA supE44 thi-1 gyrA96 relA1) was used for cloning. E. coli ST18 (pro thi hsdR+ Tpr Smr; chromosome::RP4-2 Tc::Mu-Kan::Tn7λpirΔhemA) was used for conjugation. All strains are listed in Table 1.
Genes were knocked out by P1 phage transduction (Thomason et al., 2007). Lysates for knockouts were generated from Keio collection strains (Baba et al., 2006). For selection marker removal (all flanked by FRT (flippase recognition target) sites), cells were transformed with a flippase recombinase plasmid (FLPe; temperature sensitive ORI, Gene Bridges, Heidelberg, Germany). Temperature increase to 37°C was used for flippase expression and to cure the cells from the FLPe plasmid.
A gene native to E. coli, lactate dehydrogenase (ldhA), was prepared via PCR amplification from the E. coli MG1655 genome. Using the previously described method (Zelcbuch et al., 2013), PCR product was cloned into pNiv, a high-copy-number cloning vector. Afterwards the gene was cloned into a pZ vector (15A origin of replication, streptomycin marker) by ligating the insert restricted with EcoRI and PstI from pNiv into similarly restricted pZ backbone. The promoters and RBS used were described previously (Braatsch et al., 2008; Zelcbuch et al., 2013).
LB medium (1% NaCl, 0.5% yeast extract, 1% tryptone) was used for strain maintenance and molecular biology work. Growth experiments were carried out in M9 minimal media (50 mM Na2HPO4, 20 mM KH2PO4, 1 mM NaCl, 20 mM NH4Cl, 2 mM MgSO4, and 100 μM CaCl2) with trace elements (134 μM EDTA, 13 μM FeCl3·6 H2O, 6.2 μM ZnCl2, 0.76 μM CuCl2·2 H2O, 0.42 μM CoCl2·2 H2O, 1.62 μM H3BO3, 0.081 μM MnCl2·4 H2O). For growth experiments, LB overnight cultures were used to inoculate 4 mL M9 preculture containing 10 mM glucose, 1 mM glycine, and 30 mM formate in 10 mL glass tubes to an optical density (600 nm, OD600) of 0.02. All glass tube cultures were incubated at 37°C with shaking at 240 rpm. To inoculate growth experiments cells from precultures were centrifuged (18,407 × g, 3 min, 4°C), washed two times in M9 medium, and used to inoculate M9 containing the indicated carbon sources. Experiments were carried out in aerobic conditions either in 10 mL glass tubes or in 96-well microplates (Nunc, Thermo Fisher Scientific). In both conditions, an atmosphere of 90% air and 10% CO2 was supplied in incubator and platereader, respectively. When following growth in platereader experiments, microtiter plates contained 150 μL culture and (to avoid evaporation) the culture was covered with 50 μL mineral oil (Sigma-Aldrich). Growth experiments were conducted at 90% air/10% CO2 in BioTek Epoch 2 plate readers (Agilent, Santa Clara, CA, United States) at 37°C. OD600 was measured at the end of 12 shaking steps, that were alternating between linear and orbital (1 mm amplitude) shaking for 60 s. Plate reader measured OD600 values were converted to cuvette OD600 values according to ODcuvette = ODplate/0.23. When experiments were carried out in glass-tubes, volume loss by evaporation was corrected by adding sterile water. Growth data shown represents averages of growth experiments performed in triplicate.
Colonies from LB plates were used to start test tube LB over-night precultures. Strains were washed 3 times with M9 medium. Each strain was then inoculated in test tubes with starting OD600 of ∼ 0.05. All tubes were incubated in an orbital shaker at 37°C with 10% CO2 until the stationary phase in each treatment was reached. Selected cultures were fed with formic acid when each culture reached the stationary phase (OD stopped increasing). Formic acid was added to each tube to increase its concentration by either 30 mM or 60 mM. For each strain, control tubes were left without feeding. Expression of ldhA was induced by adding 1 mM isopropyl β-d-1-thiogalactopyranoside (IPTG) together with additional formic acid after 120 h of cultivation. Periodic sampling was performed for measuring the extracellular ions dissolved in the medium by ion chromatography (Dionex ICS 6000 HPAEC, IonPac AS11-HC-4µm Analytical/Capillary Column; Thermo Fisher Scientific). At each sampling point, 200 µL were taken from the cultures and centrifuged at 15,000 rpm for 3 min. The supernatant was then diluted 20 times with ddH2O. The diluted sample was centrifuged again and transferred to a chromatography vial for the ion chromatography analysis.
Biomass yield was determined by dry cell weight (CDW) measurement of triplicate cultures. E. coli cells were inoculated to a starting OD600 of 0.01 into M9 medium containing 90 mM of formate in 125 mL flasks. Cultures were incubated in the presence of 10% CO2 at 37°C with shaking at 240 rpm. Cells were harvested in exponential growth phase (OD600 of 0.6–0.8) on 90 mM formate. Samples of up to 50 mL were harvested by centrifugation (3,220 × g, 20 min) and washed three times in H2O to remove residual medium compounds (7,000 × g, 5 min). Finally, the cells were resuspended in 2 mL H2O, transferred into pre-dried aluminum dishes, and dried for 16 h at 90°C. CDW was determined and subtracted by the dish weight.
We previously developed a formatotrophic E. coli strain named K4e (Kim et al., 2020). Engineering of this strain was achieved following a modular strategy that included four different modules: i) the C1 module for the conversion of formate into methylene-THF (Methylobacterium extorquens formate THF ligase, methenyl-THF cyclohydrolase, and methylene-THF dehydrogenase); ii) the C2 module condensing methylene-THF, CO2, and ammonia to glycine (endogenous enzymes of the glycine cleavage system; GCS, GcvT, GcvH, and GcvP); iii) the C3 module, condensing glycine and methylene-THF to serine, and finally deaminating serine to pyruvate (endogenous serine hydroxymethyltransferase (SHMT) and serine deaminase); and iv) the energy module generating NADH from formate [formate dehydrogenase (FDH) from Pseudomonas sp. (strain 101)] (Kim et al., 2020). After initial growth was observed, the strain’s growth was optimized, reaching performance characteristics of isolated mutants (K4e) of 9 h doubling time and a biomass yield of 2.3 g CDW/mol formate. Subsequent genome sequence analysis of K4e and reverse engineering revealed that upregulation mutations in the energy module and the membrane-bound transhydrogenase (a gene product of pntAB) supported enhanced growth on formate.
To improve K4e′s growth performance further, we used this strain in an adaptive laboratory evolution (ALE) experiment, selecting for faster growth on formate and CO2. The cells were grown in M9 minimal medium containing formate and CO2 as the sole carbon sources. We cultivated the K4e strain in test tubes with a formate concentration of 90 mM in a CO2 atmosphere set to 10%. Once the turbidity reached an OD600 of 1.0 (corresponding to late exponential phase), the culture was diluted 1:100 into fresh medium of the same composition to start a new cultivation cycle. While the doubling time gradually decreased over 30 cycles, the final OD600 was stagnant for the first 14 cycles (≤90 generations). From cycle 15 onwards it appeared that a new mutant became dominant and a stairway-like enhancement in OD600 was observed (Figure 2A). To confirm the growth improvement of individuals from the ALE culture, growth of four independent isolates (originating from cycle 26) was analyzed in the plate reader. These independent growth tests conducted with the newly isolated strains, named as ‘K4e2’, confirmed > 40% faster growth of the isolates, reflected by a decrease in doubling time from 9 to 6.3 h. Strikingly, the isolates also showed a 40% increase in biomass yield, from 2.3 to 3.3 g-CDW/mol formate. Furthermore, increased tolerance toward formate was observed for the newly isolated strain K4e2, which showed accelerated growth as expressed by a reduced doubling time from 8.9 to 6 h and an increase of the final OD600 from 0.9 to 1.53 at 150 mM formate (Figure 2C). Moreover, even with 200 mM of formate, the strain grows without any significant growth-rate reduction (Figure 2D, 6.5 h doubling time). Compared to K4e, which showed optimal growth at < 90 mM formate and poor growth at > 150 mM (Kim et al., 2020), K4e2 represents a clear improvement. Thus, the isolated strain not only improved in biomass productivity, but also in formate tolerance, a feature, that is especially beneficial in terms of bioprocess design, since the system can be more robust with strains that exhibit higher formate tolerance.
FIGURE 2. Evolution approaches for enhancing growth on formate. (A) Evolution from K4e to K4e2 via laboratory evolution was conducted in test tubes in M9 minimal medium with 90 mM formate in the presence of 10% CO2. Final OD600 (orange circle) and doubling times (blue circle) were normalized to K4e. (B) All identified mutated genes obtained after 30 cycles of re-inoculation. Only newly identified mutations are shown compared with parental strain K4e. (C) Growth profile comparison between evolved strains. (D) Formate tolerance test of K4e2. (E) Fed-batch cultivation of strain K4e2 in a bioreactor with pH control. Feeding and pH control were achieved by pumping 10 M of formic acid. List of genes: pdhR, pyruvate dehydrogenase complex regulator; ymdE, uncharacterized protein; ackA, acetate kinase; yqhO, biofilm formation related gene; rpoC, RNA polymerase subunit beta; glpK, glycerol kinase; Experiments (C,D) were carried out at 10% CO2 in 96-well plates in triplicates, displayed results are averages with errors < 5%. The corresponding doubling times (Dt) are shown in the figure.
To reveal the genetic changes underlying the growth improvements of the K4e2 isolates, the genomes of all four isolates were sequenced (see Supplementary Table S1 for all mutations identified). The analysis revealed that all four isolates share three common mutations. The first mutation is a mobile element insertion in the promoter region of ackA, which encodes an acetate kinase, responsible for acetate uptake or acetate overflow metabolism in the presence of oxygen (Wolfe, 2005; Szenk et al., 2017). The second mutation is a non-sense mutation (E239X) in pdhR, encoding a DNA-binding transcriptional dual regulator. The gene product of pdhR represses genes of the pyruvate dehydrogenase complex (PDH) and in the terminal electron transport systems (Ogasawara et al., 2007). As PDH converts pyruvate—a product of formate assimilation via the rGly pathway—to acetyl-CoA, a change in gene expression brought about by a pdhR mutation might positively influence growth by decreasing oxidative flux via the TCA cycle. Moreover, the enzyme complexes of PDH and GCS, the key enzyme of the rGly pathway, both contain lipoamide dehydrogenase, the expression of the corresponding gene (lpd) is repressed by PdhR (Quail and Guest, 1995). Lastly, a point mutation (A919V) occurs at rpoC, which encodes RNA polymerase subunit β’. Here, a direct relevance to carbon and energy metabolism is not obvious (Figure 2B).
Among the mutations found in the K4e2 isolates from the evolution experiment, the mobile element (ME) insertion into the upstream region of ackA (acetate kinase) provides important information regarding the formatotrophic growth mode of E. coli via the rGly pathway. The ME insertion occurred at the −35 element in the promoter region of ackA, which we assume would decrease the level of ackA expression. It is not intuitive to consider the occurrence of acetate overflow metabolism in E. coli while growing on formate and reaching only very limited final OD600 (Basan et al., 2015; Bernal et al., 2016). However, the observed ME insertion suggests that by-product generation might be a limiting factor while growing on formate. Hence, we measured accumulation of metabolites, including acetate, succinate, lactate, and pyruvate during formatotrophic growth. Acetate accumulation was indeed observed from an early growth stage and reached up to 0.2 mM in K4e (Figure 3A). Besides acetate, no other organic acid was measurable in the analyzed samples. We found that the excreted acetate is re-assimilated as the cell enters the mid-exponential phase. This can be facilitated by either acetate kinase (ackA) and phosphotransacetylase (pta), consuming one ATP, or acetyl-CoA synthetase (acs), which converts acetate to acetyl-CoA while consuming two ATP equivalents (Kumari et al., 1995). In order to prevent K4e from synthesizing acetate, causing loss of carbon and to mimic the ME insertion found in the K4e2 isolates, both ackA and pta were deleted, resulting in the K4eΔackA-pta strain. When K4eΔackA-pta was cultured using the same conditions, acetate excretion was strongly reduced. To compare the growth performance of K4e and K4eΔackA-pta, we used two different formate concentrations. With 80 mM formate, both strains show similar growth patterns with almost identical doubling times. However, with 150 mM formate, the K4eΔackA-pta strain displays not only a reduced doubling time of 7.2 h (as compared to 8.5 h of K4e), but also exhibits a 30% increase in the final OD600 (Figure 3B). Indeed, we determined the observed biomass yield of K4eΔackA-pta to be 3.1 g CDW/mol formate, close to that of K4e2 (3.3 g CDW/mol formate). Moreover, the apparent lag phase of K4e on 150 mM formate was not present in K4eΔackA-pta, thus the K4eΔackA-pta strain grew within 80 h to the stationary phase, while the parental strain required more than 140 h to reach the stationary phase. As we expected, avoiding acetate excretion by deleting acetate biosynthesis in K4e2 led to increased biomass yield on formate. Thus, abolishing acetate biosynthesis in K4e is apparently helpful for growth on formate. The inactivity of acetate synthesis removes the option of ATP generation via acetate kinase, to compensate for this ATP generation option, an increased TCA cycle flux to generate additional NADH and ATP could be the consequence. Additionally, as the K4e strain carries a deletion in aceA, encoding isocitrate lyase, the strain lacks a functional glyoxylate shunt, which is essential for acetate assimilation as the sole carbon source. Thus, acetate, when assimilated into the TCA-cycle, mainly generates reducing power via the TCA-cycle and contributes only to a small fraction of some biomass building blocks (e.g. fatty acids, leucine, and glutamate-family amino acids). This might be one reason for the better growth of the ackA-pta deletion strain. A further explanation for the growth optimization is, that upon deletion of ackA-pta RNA polymerase sigma factor (RpoS) is upregulated. RpoS is a major regulator of the general stress response in E. coli and positively affects resistance to acid stress (Kirkpatrick et al., 2001). Since, the deletion of ackA-pta in the naïve strain already improved growth performance to the level of the K4e2 strain, reverse engineering of rpoC and pdhR, that cannot directly be connected to carbon and energy metabolism, was not pursued.
FIGURE 3. Effect of acetate overflow metabolism on the growth of K4e. (A) Acetate accumulation of K4e in test tubes with 90 mM initial formate. Only acetate was excreted in K4e and re-assimilated as the cell growth enters exponential phase. Strongly reduced acetate production was observed with K4e ∆ackA-pta. (B) Growth profile of K4e and K4e∆ackA-pta on 80 and 150 mM formate in 96-well plate experiments performed in triplicates, which displayed identical growth curves (<5% errors). Deletion of ack-pta resulted in increasing final OD600 and high formate tolerance. Dt, doubling time.
To further investigate if a complete deletion of ackA and pta in the evolved K4e2 strain would positively influence the strain’s growth performance, we deleted both genes in the K4e2 strain, yielding K4e2ΔackA-pta. A direct comparison of growth of K4e2 to K4e2ΔackA-pta revealed no difference in terms of doubling times and final OD600 when strains grew with 150 or 240 mM formate (Figure 4). However, K4e2ΔackA-pta clearly displays a reduced lag phase before the onset of exponential growth, suggesting that the complete deletions allow the strain to use the formate more efficiently. However, this is not reflected in the observed biomass yield of 3.4 g CDW/mol formate, which is virtually identical to the biomass yield of the parental strain K4e2. However, the biomass yields achieved by K4e2 exceeds the reported average biomass yields of microorganisms naturally growing on formate via the Calvin–Benson–Basham cycle (3.2 g CDW/mol formate) (Claassens et al., 2019).
FIGURE 4. Deletion of ack-pta in K4e2 further optimizes formatotrophic growth. Experiments were carried out at 10% CO2 in 96-well plates in triplicates, displayed results are averages with errors < 5%.
In order to characterize the strain’s potential for large-scale production we conducted fed-batch cultivation in a 1-L stirred-tank bioreactor, growing strain K4e2 in M9 medium with 30 mM formate at pH 7. For controlling pH and feeding of formate we used 10 M formic acid only. Starting from an inoculation OD600 of 0.34, the strain reached a final OD600 of 22 within 116 h (corresponding to six doublings) with a maximal growth rate of 0.048 h−1, corresponding to a doubling time of 14.4 h. The total biomass produced corresponded to 8 g CDW/l (Figure 2E). With a total consumption of 2.289 mol/L formate, the observed biomass yield was determined to be 3.5 g CDW/mol formate, which is consistent with the values derived from batch cultivations. The achieved cell density and growth velocity largely exceeds those previously reported for engineered formatotrophic E. coli (Bang et al., 2020). This highlights the potential of the engineered strain for use in industrial bioprocesses, where high cell densities are often required to achieve economic feasibility. However, some improvement with respect to biomass yield and doubling time is still possible, especially when comparing to the reported maximal theoretical biomass yields of ∼ 5 g CDW/mol formate (Bar-Even et al., 2013; Cotton et al., 2020). We thus set out to further improve our strains by analyzing and making use of mutations that accumulated during the adaptive evolution.
Lactate was selected as a proxy chemical to show the potential of formatotrophic bioproduction. Lactate is an important chemical used in the food and chemical industry. It contains a hydroxyl as well as a carboxyl group and can form poly-lactic acid by self-esterification, a polymer for producing bio-plastic (Maki-Arvela et al., 2014). Lactate can be generated by a reaction catalyzed by lactate dehydrogenase (ldhA), which oxidizes NADH using pyruvate as an electron acceptor. In order to prevent E. coli from re-assimilating the lactate, quinone dependent D-lactate dehydrogenase (dld) was deleted, generating K4eΔackA-ptaΔdld, named KS44. To achieve formatotrophic lactate production, the KS44 strain was transformed with the ldhA gene cloned into an IPTG-inducible expression cassette in plasmid pSStac. The final strain with ldhA overexpression was named KS46. To test for IPTG-inducible lactate production from formate, we applied a two-phased strategy. The growth phase was started in 90 mM formate and continued until the cells entered the stationary phase. The production phase was initiated by adding 1 mM IPTG and 60 mM of formic acid to the culture (Figure 5A). During the growth phase, the pH of the culture increases due to formate uptake into the cell, either via a proton symport mechanism or in the form of free formic acid (Wang et al., 2009; Lü et al., 2011; Wiechert and Beitz, 2017). Assimilation of 90 mM formate increased the culture pH from 6.9 to 7.8 (Figure 5B). Addition of 60 mM formic acid decreased the culture’s pH back from 7.8 to 6.9. Along with the two-phased strategy, a normal batch culture was also cultivated for comparison. When formate was completely consumed in the culture, lactate concentrations in the supernatant was analyzed. Here, we were able to detect 1.2 mM of lactate in the two-phase cultivation (Figure 5C), corresponding to almost 10% of the maximal theoretical yield (Cotton et al., 2020).
FIGURE 5. Lactate production from formate with formatotrophic E. coli. (A) Engineered E. coli strain was cultured in minimal medium using 90 mM formate and 10% CO2 as carbon sources. Two different cultivation methods were tested: normal batch mode and fed-batch mode with the addition of 60 mM formic acid (FA) at the indicated time point. (B) pH profile during growth on formate. Cell growth on formate directly correlates with increased medium pH due to the accumulation of OH−. (C) Lactate production was observed only with the strain in the fed-batch mode along with ldhA overexpression (n = 4, lactate measurement was conducted at the end of cultivation). Experiments were carried out at 10% CO2 in incubator shaker. Displayed results are averages with errors < 5%.
This study demonstrates that a previously engineered formatotrophic E. coli strain growing via the rGly pathway can be optimized for the production of value-added chemicals such as lactate. Adaptive laboratory evolution conducted with CO2 and formate as carbon sources yielded a strain with increased biomass yield, shorter doubling time, and the ability to grow to high cellular densities. Interestingly, formate tolerance was increased as well, allowing growth with 200 mM formate, while the parent formatotrophic strain K4e did not grow at such formate concentrations. Subsequent genome sequencing revealed that avoidance of acetate production is one of the key factors for improved growth. By-product analysis of K4e showed that this strain indeed generates acetate during growth on formate and re-assimilates excreted acetate at the late stage of the growth phase. When acetate kinase and phosphate acetyltransferase were deleted from K4e, a similar growth-profile compared to K4e2 was observed, especially with high formate concentration. While the maximal cell density previously reported was 3.5 g CDW/l and the biomass yield 2.5 g CDW/mol formate (Bang et al., 2020), the newly evolved K4e2 strain exceeded those by reaching a cell density of 8 g CDW/l and a biomass yield of 3.4 g CDW/mol formate. This finding not only exemplifies the utility of adaptive laboratory evolution but also constitutes a further step towards the industrial use of the synthetic rGly pathway. This sustainable approach to bacterial biomass production can directly find application in areas like single-cell protein or feed production. However, even more urgent but more challenging is the development of sustainable processes for value-added chemicals to provide alternatives to petroleum-based sources.
In order to achieve biological transformation of formate to lactate, inducible lactate dehydrogenase was implemented and the lactate assimilating quinone-dependent D-lactate dehydrogenase was deleted. When formate/formic-acid fed-batch cultivation was carried out with this strain, production of lactate was observed. We thus showed that our formatotrophic E. coli strain, which utilizes formate as energy and carbon source through the rGly pathway, can be further optimized, in this case by prevention of the wasteful acetate formation, and can be applied for the microbial conversion to a chemical of interest. Finally, further strain engineering to increase flux towards lactate and the establishment of an optimized bioprocess will unlock the full potential of the rGly pathway and hence help paving the way towards a C1-bioeconomy.
In the presented study, we used ALE experiments to achieve faster growth via the rGly pathway. However, some additions to the genomic preset might be helpful to further optimize pathway efficiency and hence growth performance. E.g., changing the cofactor of the GCS to NADPH can provide a thermodynamic push, which might allow growth at lower CO2 concentrations. Additionally, improved ATP efficiency of the rGly pathway can increase growth efficiency. One way to achieve this is to replace the iron-sulfur-cluster enzyme serine deaminase, which provides limiting kinetic properties, with a transaminase converting serine to hydroxypyruvate which is entering glycolysis at the level of 3-phosphoglycerate.
The original contributions presented in the study are included in the article/Supplementary Material, further inquiries can be directed to the corresponding author.
SK: Investigation, formal analysis, visualization, supervision, and writing—original draft. ND: Investigation and formal analysis. VR: Investigation and formal analysis. FM: Investigation, formal analysis, and visualization. FC: Investigation, formal analysis, and writing—review and editing. AK: Formal analysis and writing—review and editing. FK: Supervision. AB-E: Conceptualization, funding acquisition, and supervision. SL: Conceptualization, supervision, visualization, writing—original draft, and writing—review and editing.
This work was funded by the Max Planck Society and by the European Union’s Horizon 2020 research and innovation programme under grant agreement No. 763911 (Project eForFuel).
The authors thank Enrico Orsi and Hezi Tenenboim for critically reading the manuscript. This work was funded by the Max Planck Society and by the European Union’s Horizon 2020 research and innovation programme under grant agreement No. 763911 (Project eForFuel).
Authors FC, AK, and FK are employed by b.fab GmbH.
The remaining authors declare that the research was conducted in the absence of any commercial or financial relationships that could be construed as a potential conflict of interest.
All claims expressed in this article are solely those of the authors and do not necessarily represent those of their affiliated organizations, or those of the publisher, the editors and the reviewers. Any product that may be evaluated in this article, or claim that may be made by its manufacturer, is not guaranteed or endorsed by the publisher.
The Supplementary Material for this article can be found online at: https://www.frontiersin.org/articles/10.3389/fbioe.2023.1091899/full#supplementary-material
Baba, T., Ara, T., Hasegawa, M., Takai, Y., Okumura, Y., Baba, M., et al. (2006). Construction of Escherichia coli K-12 in-frame, single-gene knockout mutants: The Keio collection. Mol. Syst. Biol. 2, 2006.0008. doi:10.1038/msb4100050
Bang, J., Hwang, C. H., Ahn, J. H., Lee, J. A., and Lee, S. Y. (2020). Escherichia coli is engineered to grow on CO2 and formic acid. Nat. Microbiol. 5, 1459–1463. doi:10.1038/s41564-020-00793-9
Bar-Even, A., Flamholz, A., Noor, E., and Milo, R. (2012a). Thermodynamic constraints shape the structure of carbon fixation pathways. Biochim. Biophys. Acta - Bioenerg. 1817, 1646–1659. doi:10.1016/j.bbabio.2012.05.002
Bar-Even, A., Noor, E., Flamholz, A., and Milo, R. (2013). Design and analysis of metabolic pathways supporting formatotrophic growth for electricity-dependent cultivation of microbes. Biochim. Biophys. Acta - Bioenerg. 1827, 1039–1047. doi:10.1016/j.bbabio.2012.10.013
Bar-Even, A., Noor, E., and Milo, R. (2012b). A survey of carbon fixation pathways through a quantitative lens. J. Exp. Bot. 63, 2325–2342. doi:10.1093/jxb/err417
Basan, M., Hui, S., Okano, H., Zhang, Z., Shen, Y., Williamson, J. R., et al. (2015). Overflow metabolism in Escherichia coli results from efficient proteome allocation. Nature 528, 99–104. doi:10.1038/nature15765
Berg, I. A., Kockelkorn, D., Ramos-Vera, W. H., Say, R. F., Zarzycki, J., Hügler, M., et al. (2010). Autotrophic carbon fixation in archaea. Nat. Rev. Microbiol. 8, 447–460. doi:10.1038/nrmicro2365
Bernal, V., Castaño-Cerezo, S., and Cánovas, M. (2016). Acetate metabolism regulation in Escherichia coli: Carbon overflow, pathogenicity, and beyond. Appl. Microbiol. Biotechnol. 8985, 8985–9001. doi:10.1007/s00253-016-7832-x
Blattner, F. R., Goeden, M. A., Glasner, J. D., Rode, C. K., Collado-Vides, J., Mau, B., et al. (1997). The complete genome sequence of Escherichia coli K-12, Science 277, 1453–1462. doi:10.1126/science.277.5331.1453
Braatsch, S., Helmark, S., Kranz, H., Koebmann, B., and Jensen, P. R. (2008). Escherichia coli strains with promoter libraries constructed by Red/ET recombination pave the way for transcriptional fine-tuning. Biotechniques 45, 335–337. doi:10.2144/000112907
Chen, F. Y.-H., Jung, H.-W., Tsuei, C.-Y., and Liao, J. C. (2020). Converting Escherichia coli to a synthetic methylotroph growing solely on methanol. Cell 182, 933–946.e14. doi:10.1016/j.cell.2020.07.010
Claassens, N. J., Cotton, C. A. R., Kopljar, D., and Bar-Even, A. (2019). Making quantitative sense of electromicrobial production. Nat. Catal. 2, 437–447. doi:10.1038/s41929-019-0272-0
Claassens, N. J., Satanowski, A., Bysani, V. R., Dronsella, B., Orsi, E., Rainaldi, V., et al. (2022). Engineering the reductive Glycine pathway: A promising synthetic metabolism approach for C1-assimilation BT - one-carbon feedstocks for sustainable bioproduction. Editors A.-P. Zeng, and N. J. Claassens (Cham: Springer International Publishing), 299–350. doi:10.1007/10_2021_181
Cotton, C. A., Claassens, N. J., Benito-Vaquerizo, S., and Bar-Even, A. (2020). Renewable methanol and formate as microbial feedstocks. Curr. Opin. Biotechnol. 62, 168–180. doi:10.1016/j.copbio.2019.10.002
Gassler, T., Sauer, M., Gasser, B., Egermeier, M., Troyer, C., Causon, T., et al. (2020). The industrial yeast Pichia pastoris is converted from a heterotroph into an autotroph capable of growth on CO2. Nat. Biotechnol. 38, 210–216. doi:10.1038/s41587-019-0363-0
Gleizer, S., Ben-Nissan, R., Bar-On, Y. M., Antonovsky, N., Noor, E., Zohar, Y., et al. (2019). Conversion of Escherichia coli to generate all biomass carbon from CO2. Cell 179, 1255–1263.e12. doi:10.1016/j.cell.2019.11.009
He, M., Sun, Y., and Han, B., 2013. Green carbon science: Scientific basis for integrating carbon resource processing, utilization, and recycling. Angew. Chem. Int. Ed. 52, 9620–9633. doi:10.1002/anie.201209384
Janssen, M., Tramper, J., Mur, L. R., and Wijffels, R. H., 2003. Enclosed outdoor photobioreactors: Light regime, photosynthetic efficiency, scale-up, and future prospects. Biotechnol. Bioeng. 81, 193–210. doi:10.1002/bit.10468
Kim, S., Lindner, S. N., Aslan, S., Yishai, O., Wenk, S., Schann, K., et al. (2020). Growth of E. coli on formate and methanol via the reductive glycine pathway. Nat. Chem. Biol. 16, 538–545. doi:10.1038/s41589-020-0473-5
Kirkpatrick, C., Maurer, L. M., Oyelakin, N. E., Yoncheva, Y. N., Maurer, R., and Slonczewski, J. L. (2001). Acetate and formate stress: Opposite responses in the proteome of Escherichia coli. J. Bacteriol. 183, 6466–6477. doi:10.1128/JB.183.21.6466-6477.2001
Kumari, S., Tishel, R., Eisenbach, M., and Wolfe, A. J. (1995). Cloning, characterization, and functional expression of acs, the gene which encodes acetyl coenzyme A synthetase in Escherichia coli. J. Bacteriol. 177, 2878–2886. doi:10.1128/jb.177.10.2878-2886.1995
Li, H., Opgenorth, P. H., Wernick, D. G., Rogers, S., Wu, T. Y., Higashide, W., et al. (2012). Integrated electromicrobial conversion of CO2 to higher alcohols. Science 335, 1596. doi:10.1126/science.1217643
Liu, C., Colón, B. C., Ziesack, M., Silver, P. A., and Nocera, D. G. (2016). Water splitting–biosynthetic system with CO2 reduction efficiencies exceeding photosynthesis. Science 352, 1210–1213. doi:10.1126/science.aaf5039
Lü, W., Du, J., Wacker, T., Gerbig-Smentek, E., Andrade, S. L., and Einsle, O. (2011). pH-dependent gating in a FocA formate channel. Science 332, 352–354. doi:10.1126/science.1199098
Maki-Arvela, P., Simakova, I. L., Salmi, T., and Murzin, D. Y. (2014). Production of lactic acid/lactates from biomass and their catalytic transformations to commodities. Chem. Rev. 114, 1909–1971. doi:10.1021/cr400203v
Meselson, M., and Yuan, R. (1968). DNA restriction enzyme from E. coli. Nature 217, 1110–1114. doi:10.1038/2171110a0
Meyer, F., Keller, P., Hartl, J., Gröninger, O. G., Kiefer, P., and Vorholt, J. A. (2018). Methanol-essential growth of Escherichia coli. Escherichia coli Nat. Commun. 9, 1508. doi:10.1038/s41467-018-03937-y
Ogasawara, H., Ishida, Y., Yamada, K., Yamamoto, K., and Ishihama, A. (2007). PdhR (pyruvate dehydrogenase complex regulator) controls the respiratory electron transport system in Escherichia coli. J. Bacteriol. 189, 5534–5541. doi:10.1128/JB.00229-07
Quail, M. A., and Guest, J. R., 1995. Purification, characterization and mode of action of PdhR, the transcriptional repressor of the pdhR–aceEF–Ipd operon of Escherichia coli. Mol. Microbiol. 15, 519–529. doi:10.1111/j.1365-2958.1995.tb02265.x
Schwander, T., Schada von Borzyskowski, L., Burgener, S., Cortina, N. S., and Erb, T. J. (2016). A synthetic pathway for the fixation of carbon dioxide in vitro. Science 80, 900–904. doi:10.1126/science.aah5237
Szenk, M., Dill, K. A., and de Graff, A. M. R. (2017). Why do fast-growing bacteria enter overflow metabolism? Testing the membrane real estate hypothesis. Cell Syst. 5, 95–104. doi:10.1016/j.cels.2017.06.005
Thoma, S., and Schobert, M. (2009). An improved Escherichia coli donor strain for diparental mating. FEMS Microbiol. Lett. 294, 127–132. doi:10.1111/j.1574-6968.2009.01556.x
Thomason, L. C., Costantino, N., and Court, D. L. (2007). E. coli genome manipulation by P1 transduction. Curr. Protoc. Mol. Biol. 79, Unit 1.17. doi:10.1002/0471142727.mb0117s79
Wang, Y., Huang, Y., Wang, J., Cheng, C., Huang, W., Lu, P., et al. (2009). Structure of the formate transporter FocA reveals a pentameric aquaporin-like channel. Nature 462, 467–472. doi:10.1038/nature08610
Wiechert, M., and Beitz, E. (2017). Mechanism of formate–nitrite transporters by dielectric shift of substrate acidity. EMBO J. 36, 949–958. doi:10.15252/embj.201695776
Wolfe, A. J. (2005). The acetate switch. Microbiol. Mol. Biol. Rev. 69, 12–50. doi:10.1128/mmbr.69.1.12-50.2005
Wong, T. S. (2014). Carbon dioxide capture and utilization using biological systems: Opportunities and challenges. J. Bioprocess. Biotech. 04. doi:10.4172/2155-9821.1000155
Yishai, O., Bouzon, M., Döring, V., and Bar-Even, A. (2018). In vivo assimilation of one-carbon via a synthetic reductive glycine pathway in Escherichia coli. ACS Synth. Biol. 7, 2023–2028. doi:10.1021/acssynbio.8b00131
Yishai, O., Lindner, S. N., Gonzalez de la Cruz, J., Tenenboim, H., and Bar-Even, A. (2016). The formate bio-economy. Curr. Opin. Chem. Biol. 35, 1–9. doi:10.1016/j.cbpa.2016.07.005
Zelcbuch, L., Antonovsky, N., Bar-Even, A., Levin-Karp, A., Barenholz, U., Dayagi, M., et al. (2013). Spanning high-dimensional expression space using ribosome-binding site combinatorics. Nucleic Acids Res. 41, e98. doi:10.1093/nar/gkt151
Keywords: formate (compound CID: 283), E. coli—Escherichia coli, C1-assimilation, bioeconomy and circular economy, bioproduction, synthetic biology (synbio), metabolic rewiring
Citation: Kim S, Giraldo N, Rainaldi V, Machens F, Collas F, Kubis A, Kensy F, Bar-Even A and Lindner SN (2023) Optimizing E. coli as a formatotrophic platform for bioproduction via the reductive glycine pathway. Front. Bioeng. Biotechnol. 11:1091899. doi: 10.3389/fbioe.2023.1091899
Received: 07 November 2022; Accepted: 06 January 2023;
Published: 16 January 2023.
Edited by:
Lei Zhao, Tianjin Institute of Industrial Biotechnology (CAS), ChinaReviewed by:
Jian-Ming Liu, Technical University of Denmark, DenmarkCopyright © 2023 Kim, Giraldo, Rainaldi, Machens, Collas, Kubis, Kensy, Bar-Even and Lindner. This is an open-access article distributed under the terms of the Creative Commons Attribution License (CC BY). The use, distribution or reproduction in other forums is permitted, provided the original author(s) and the copyright owner(s) are credited and that the original publication in this journal is cited, in accordance with accepted academic practice. No use, distribution or reproduction is permitted which does not comply with these terms.
*Correspondence: Steffen N. Lindner, c3RlZmZlbi5saW5kbmVyQGNoYXJpdGUuZGU=
†Deceased
Disclaimer: All claims expressed in this article are solely those of the authors and do not necessarily represent those of their affiliated organizations, or those of the publisher, the editors and the reviewers. Any product that may be evaluated in this article or claim that may be made by its manufacturer is not guaranteed or endorsed by the publisher.
Research integrity at Frontiers
Learn more about the work of our research integrity team to safeguard the quality of each article we publish.