- 1Boehringer Ingelheim International GmbH, Ingelheim, Germany
- 2ViraTherapeutics GmbH, Rum, Austria
- 3Boehringer Ingelheim Pharmaceutical, Inc., Ridgefield, CT, United States
Purification of viruses, especially for therapeutic purposes, is a tedious and challenging task. The challenges arise due to the size and surface complexity of the virus particles. VSV-GP is a promising oncolytic virus, which has been approved for phase I clinical trials by the Food and Drug Administration (FDA) of United States and Paul Ehrlich Institute (PEI) of Germany. The virus particles of VSV-GP are larger in size than vectors commonly used for gene therapy (e.g., adenovirus, adeno-associated virus, etc.). The current established proprietary clinical-grade manufacturing process for the purification of VSV-GP encompasses several chromatographic and non-chromatographic steps. In this study, we describe a new single-step purification process for the purification of VSV-GP virus, using cation exchange convective flow column with relatively higher yields. The purified virus was characterized for its quality attributes using TCID50 assay (for viral infectivity), host cell protein contaminant ELISA, SDS-PAGE, size exclusion chromatography (SEC), and cryo-electron microscopy. Furthermore, the purified viral therapeutic material was tested in vivo for its efficacy and safety. All these characterization methods demonstrated a therapeutic virus preparation of high purity and yield, which can be readily used for various studies.
Introduction
Advanced therapeutic and medicinal products (ATMPs), often touted as the “golden bullet,” are a class of new and innovative therapeutics developed as breakthrough therapies for the treatment of “un-treatable or extraordinary diseases” such as against various types of cancer (Schneider et al., 2010). Oncolytic viruses are a class of ATMPs used to treat cancer by killing malignant cells, while sparing normal cells. Further discussion about oncolytic viruses can be read in these excellent publications (Muik et al., 2012; Kaufman et al., 2015; Macedo et al., 2020; Apolonio et al., 2021). Currently, there are only a limited number of oncolytic viral (OV) therapies being registered for commercial use in various international markets, of which IMLYGIC™ or Talimogene Laherparepvec (T-VEC) was the first OV to be approved both by FDA and EMA (EMA, 2018; Burke et al., 2021).
Vesicular stomatitis virus (VSV) is a negative-strand RNA virus that belongs to the family of rhabdoviridae (Barber, 2005). It can induce potent tumor cytotoxic effects, in a number of preclinical tumor models (Barber, 2004). VSV is also an important viral vector that has already been approved for human usage as the vaccine against the deadly Ebola viral infection (Hoenen et al., 2012; Sridhar, 2015). In this case, VSV was pseudotyped with Ebola surface glycoprotein thereby successfully demonstrating the principle of pseudotyping VSV with glycoprotein from a different virus.
Recently, a recombinant version (VSV-GP) has been developed as an immune-oncology platform that can induce both direct tumor cell killing as well as therapeutic cancer vaccination (Muik et al., 2012; Muik et al., 2014; Tober et al., 2014; Kimpel et al., 2018; Schreiber et al., 2019). Herein, VSV was pseudotyped with glycoprotein of lymphocytic choriomeningitis virus (LCMV), the pseudotyped virus being called as VSV-GP (Muik et al., 2012). This modification reduces its ability to induce anti-surface antibodies resulting in reduced sensitivity to complement-mediated neutralization and mitigates the inherent neurotropism of wildtype VSV (Muik et al., 2014; Kimpel et al., 2018). However, it must be noted that the obstacles arise both in early research as well as manufacturing of such innovative and revolutionary therapies.
One of the major challenges when developing a complex therapy, such as oncolytic viral therapy, lies in developing an efficient manufacturing process (Working et al., 2005; Ungerechts et al., 2016). This challenge is amplified by the need for a high drug concentration to allow for therapeutic dosing of oncolytic viruses as compared to vaccines (Heo et al., 2013; Ungerechts et al., 2016), wherein the dose requirement is several magnitude lower. Therefore, simple downstream processes involving 1–2 steps, used for viral vaccines (Kis et al., 2019), are generally not sufficient to achieve the quality and concentration. Consequently, an extensive downstream process is generally required to further concentrate the oncolytic virus and at the same time reducing the impurity (e.g., host cell protein and DNA) levels below the regulatory guidelines. This is a fine balance and generally difficult to achieve with simplified downstream processes used for vaccine applications.
There are various types of chromatography columns commercially available for downstream processing and purification of various therapeutic molecules, especially with ion exchangers (Jungbauer et al., 2009). However, it is important to understand that large virus-like particles or viruses have specific requirements when it comes to their purification (Cramer and Holstein, 2011; Bandeira et al., 2012; Kramberger et al., 2015; Singh and Heldt, 2022). Moreover, the largest challenges arise not only due to their size, but also due to the shape and complexity of surface charges on the viruses (Singh and Heldt, 2022). Furthermore, the surface charges on the virus particles are further modulated by incorporation of host cell proteins on the surface of virions (Burnie, 2022).
The challenges due to such complexities include but not limited to, the fact that virus size can be greater than the pore size of circular chromatography beads in the column (Singh and Heldt, 2022). Also, the shear stress generated, during the movement through the void volume, can be detrimental to the integrity of the virus particles (Gagnon, 2008; Loewe et al., 2019; Boi et al., 2020; Pereira Aguilar et al., 2020). Furthermore, porous bead-based columns are also not economical (Ghosh, 2002; Jacquemart et al., 2016) and rely on diffusion-based slower flow rates, thereby increasing the process time significantly (Ghosh, 2002).
In this study, we describe a novel, quick and cost-effective bioprocess for the purification of the oncolytic virus, VSV-GP using membrane adsorber based cation exchange chromatography. This process produces, not just a purer form of VSV-GP, but also a more concentrated drug substance. This is achieved without the need for an extensive concentration step, usually involving ultrafiltration resulting in the high concentration of oncolytic viruses, often being a pre-requisite enabling intra-tumor application of the virus. The latter is known to damage the virus particles due to shear stress (Loewe et al., 2019). Furthermore, we have characterized the purified virus using in vitro and in vivo assays to substantiate the critical quality attributes.
Materials and methods
Cells and viruses
BHK-21 (C13) cells used for the infectivity assay (TCID50) were obtained from ATCC (ATCC, 2022). 293-F suspension cells were obtained from Thermo Fisher Scientific (ThermoFisher, 2022). CT26. Cl25-IFNAR1−/− cells were generated as described previously (Schreiber et al., 2019).
VSV-GP, which is VSV (Indiana strain) pseudotyped with glycoprotein from LCMV (LCMV WE HPI-GP) and has been described previously (Muik et al., 2012; Muik et al., 2014; Tober et al., 2014). A VSV-GP variant expressing firefly luciferase (VSV-GP-Luc) was also described previously (Dold et al., 2016). Viruses were amplified in 293-F cells, virus containing supernatant harvested using centrifugation at 2000 g for 5 min. Subsequently, 0.2 M NaCl is added to the suspension before filtering the material using a 0.22 µm bottle-top filter (Corning, Cat. #431098). Filtered material is then treated with SAN-HQ Nuclease (Arcticzymes, Cat. #70921-150) to reduce the host-cell DNA content. This is performed by adding 30 U/ml of SAN-HQ and incubating for 30 min at room temperature. Finally, the virus material is diluted 1:1 with 100 mM Tris-HCl, pH 7.5 to bring NaCl concentration to 0.1 M before proceeding for downstream processing.
Cation exchange chromatography and final virus formulation
Sartobind S Nano® membrane adsorbers were used for virus capture in CEX. These membranes are cellulose-based cation exchange membrane adsorber capsules with 3 ml membrane volume (MV) and a bed height of 8 mm (Cat. Reference: 96IEXS42EUC11—A, Sartorius). The nominal pore size for these membrane adsorbers is > 3 µm (Sartorius, product datasheet). For all CEX experiments, an Äkta™ Pure 150 liquid chromatography system (Cytiva) with a 280 nm UV, pH and conductivity monitoring was operated at room temperature. Prior to virus application, membranes were equilibrated with 50 mM Tris-HCl, 50 mM Arginine, pH 7.5 at a flow rate of 15 ml/min. Sample application was conducted with the same flow rate. The elution of the virus particles was carried out with a 300 mM NaCl, 50 mM Tris-HCl, 50 mM Arginine, pH 7.5 buffer at a flow rate of 3 ml/min. Pooled elution fractions from CEX were diluted 6x in order to reach the final buffer composition with 50 mM NaCl instead of 300 mM NaCl in the elution fraction. Afterwards, virus is sterile-filtered using a 0.22 µm syringe-filter (Sarstedt, Cat. #831826001). Virus aliquots were stored at −80°C before use.
Median tissue culture infectious dose assay
To determine the quantity of infectious virus present in our samples, TCID50 assay was performed as described previously (Hochdorfer et al., 2022). Briefly, BHK-21 (C13) cells were plated in 96-well plates. Post-seeding (24 h), 0.5 log serial dilutions of the virus are added into the cell wells. After 3 days, cell confluence was used to determine the cytopathic effect (CPE) for each well. Cell confluencies >95% are regarded as CPE negative. Final titers (TCID50/ml) were obtained by the formula of Spearman Kärber (Spearman, 1908; Kärber, 1931).
Host-cell protein ELISA assay
The quantification of host-cell protein (HCP) impurities was determined by performing the Human Embryonic Kidney 293 Host Cell Proteins ELISA kit from Cygnus Technologies (Cat. Reference: F650S) according to the manufacturer´s instructions. The amount of hydrolyzed substrate, which gives a colorimetric signal directly proportional to the HCPs concentration, was measured with a Spark 20 M Tecan Plate Reader® using Nunclon® Flat bottom 96-well plates (Thermo Fisher).
Sodium dodecyl sulfate polyacrylamide gel electrophoresis and silver staining
In order to test purity and identify the viral and host-cell proteins, SDS-PAGE followed by silver staining were carried out (Chevallet et al., 2006). Precast 4%–20% gradient gels (Bio-Rad, Cat. #4561095) were chosen due to their ability of separating broad ranges of molecular weights. Virus samples were diluted with 4x Laemmli Sample Buffer (Bio-Rad, Cat. #1610747) containing freshly added 200 mM Dithiothreitol (DTT) as a reducing agent (Bio-Rad, Cat. #1610610). Afterwards, samples were heat-denatured at 95°C for 5 min. As a molecular weight ladder, Precision Plus Protein Dual Color Standard (Bio-Rad, Cat. #1610374) was used.
For revealing the gel protein bands, the commercial kit “Pierce™ Silver Stain” (Thermo Fisher Scientific, Cat. #24612) was employed. Silver staining is an ultrasensitive method which can effectively reveal protein bands that are in sub-nanogram ranges. Silver staining was performed as detailed by the manufacturer´s instructions. Gel images were acquired using VisionWorks LS software (Version 8) with ChemStudio gel imaging instrument (AnalytikJena, Jena, Germany).
Cryo-transmission electron microscopy
Cryo-TEM imaging was performed by NanoImaging Services, Inc (San Diego, CA, United States). Each sample was prepared by applying a 3 μl drop of undiluted sample suspension to a cleaned grid (holey carbon films on 400-mesh copper grid) and immediately proceeding with vitrification in liquid ethane. Electron microscopy was performed using a Thermo Fisher Scientific Glacios Cryo-TEM operated at 200 kV and equipped with a Falcon three direct electron detector. Automated data-collection is carried out using Leginon software (Suloway et al., 2005; Cheng et al., 2021). High magnification images were acquired at nominal magnifications of 73,000 × (0.201 nm/pixel) and 28,000 × (0.519 nm/pixel) after identifying potentially suitable target areas for imaging at lower magnifications. The images were acquired at a nominal underfocus of −5.6 μm to −2.5 μm and electron doses of ∼10–25 e−/Å2.
Analytical size exclusion chromatography
In SEC analysis, 40 µl samples were injected through a 1,290 Infinity II HPLC system (Agilent Technologies, Inc., CA, United States) with UV detection at 280 nm wavelength. Contaminant analyses were performed using TSKgel 3000 PWXL SEC column (13 μm, 30 cm × 7.8 mm, TOSOH Bioscience, Tokyo, Japan) operated at room temperature with an isocratic flow at 0.5 ml/min flow rate using a mobile phase composed of phosphate buffered saline (PBS).
In vivo studies
Six to eight-week-old female BALB/c (BALB/cByJ) mice, were obtained from Charles River Laboratories (Wilmington, MA, Unites States). CT26. Cl25-IFNAR1−/− tumors were implanted by subcutaneous injection of 100 μl containing 1 × 106 cells in the right flank (Mueller et al., 2019). Tumor size was measured three times a week with a caliper and volume was calculated using the formula: length × width2 × 0.5. Treatment commenced when the mean tumor volume reached a size of 80–150 mm3. Virus solutions with 109 TCID50 of virus were used for intravenous (100 μl) injection. Mice were sacrificed when their tumor volume reached 1,500 mm3 or tumors showed signs of ulcerations (Workman et al., 2010). Animals were euthanized by overdose on gas anesthesia (isoflurane) followed by cervical dislocation or exsanguination.
Bioluminescence imaging
For Bioluminescence imaging, a charge–coupled device camera (PhotonIMAGER RT, Biospace Lab, France) was used. Mice were injected intraperitoneally with 50 mg/kg D-Luciferin (D-Luciferin potassium salt, in vivo imaging solution at 15 mg/ml) in DPBS, and imaged 15 min after injection upon transient narcosis. Exposure times were adjusted to bioluminescence intensity to optimize signal levels and avoid saturation of the light sensors. For quantification of bioluminescent signal, regions of interest (ROI) were manually defined and the average intensity within the ROI was measured as photons/sec/cm2/sr. The obtained images were analyzed using M3Vision image analysis software (Biospace Lab, France).
Results
In the current study, specific binding, and elution of oncolytic virus VSV-GP with the cationic exchange membrane adsorber, Sartobind S, was tested along with the concentration of VSV-GP from diluted crude feed material obtained after digesting nucleic acids with SAN-HQ nuclease (methods and materials). Moreover, it is important to mention that the treatment of feed with nuclease resulted in a host cell DNA concentration of only 4.16 ng/ml, which is already below any regulatory requirements (Yang, 2013). This cation exchange membrane adsorber was selected based on the virus surface properties [recombinantly modified to have LCMV GP instead of WT VSV glycoprotein (Muik et al., 2012; Kimpel et al., 2018)] having a positive net charge at physiological pH (Uniprot: P09991).
The current Sartobind S based chromatography method was developed to facilitate the binding of VSV-GP to the membrane adsorber column in the presence of low concentration of sodium chloride (0.1 M NaCl) thereby eliminating non-specific binding of molecules such proteins present in the crude feed (Figure 1A). A wash step, involving washing with a mild salt concentration (0.05 M NaCl), was also incorporated to further remove non-specific binders and maximize the elimination of process-related impurities, while still binding most of the quantity of the target molecule i.e., VSV-GP. Indeed, it was discovered that >95% of the virus particles bound to the column from the feed material (Figure 1). The bound material was then eluted from the column using a linear gradient of 1 M NaCl, resulting in an efficient elution (Figure 1B). The main peak observed in the elution fraction with the linear gradient was calculated to be at around 300 mM NaCl with >60% of total loaded VSV-GP particles as calculated using TCID50 (Figure 1).
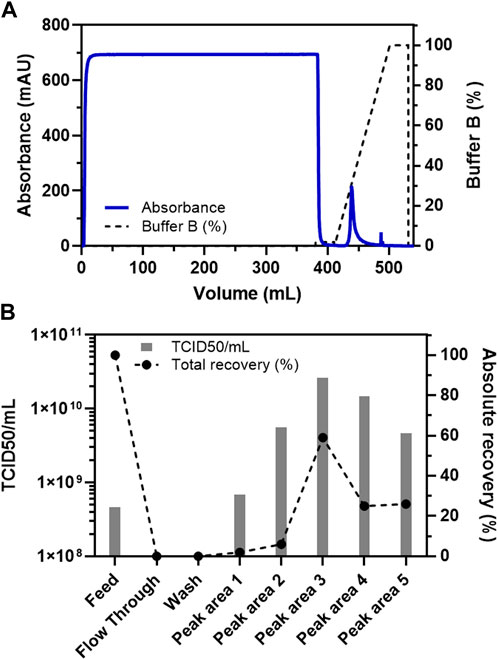
FIGURE 1. Binding and elution (using a linear gradient) of VSV-GP from the crude feed on Sartobind S membrane adsorber. (A) Chromatogram for the purification of VSV-GP using Sartobind S membrane adsorber column. (B) TCID50-based infectivity assay for various fractions obtained during purification of VSV-GP in (A).
Furthermore, we wanted to analyze the dynamic binding capacity of the column with a load of 5,800 ml of the feed material on a 3 ml Sartobind S column (Supplementary Figure S1). Despite such a high load in terms of feed volume, the number of particles detected in the flow through fractions remained relatively less, not reaching a threshold of >10% breakthrough (Supplementary Figure S1B). Observation during the linear step gradient that the main virus peak was observed at around 300 mM NaCl (Figure 1), we also incorporated a step elution gradient to pinpoint the elution profile of the VSV-GP from the cation-exchange membrane adsorber (Supplementary Figure S1). The earlier observation with the linear gradient (Figure 1A) was confirmed, showing efficient virus elution with 300 mM NaCl. Only non-significant elution occurred at higher (>300 mM) NaCl concentrations (Supplementary Figure S1).
Apart from factors such as a higher yield, speed, and cost-effectiveness, it is also imperative to consider the purification efficiency of the process. Therefore, a consolidation run with a feed of 2,300 ml (766.66 membrane volumes) and a fixed step elution with 300 mM NaCl was carried out (Figure 2). This consolidation run was used to incorporate all previous optimizations, establish the final process, and analyze the purified virus product for its quality attributes. The consolidated process resulted in a single peak during the chromatography (Figure 2A) and a very high concentration of the virus of interest with a volume of 8 ml (Figure 2B). Generally, the requirement for oncolytic viruses is also to be administered intra-tumorally. Therefore, a higher concentration of virus in the drug product is needed, which was achieved with this step. However, the peak was further diluted 6x to have a working stock appropriate for further experiments. The purified virus material, using the Sartobind S column, was further analyzed for the removal of host cell protein impurities. The purification of crude feed material using the cation-exchange membrane adsorber resulted in a >99% removal of host cell protein contaminants (Figure 3A). Contaminant analysis using analytical SEC method (manuscript under preparation) also revealed a prominent single peak as compared to multiple peaks for the same virus purified using density gradient centrifugation (Figure 3B). SDS-PAGE analysis also revealed that the virus material was purified to a similar extent as the GMP process being used for manufacturing clinical-grade VSV-GP (Figure 3C).
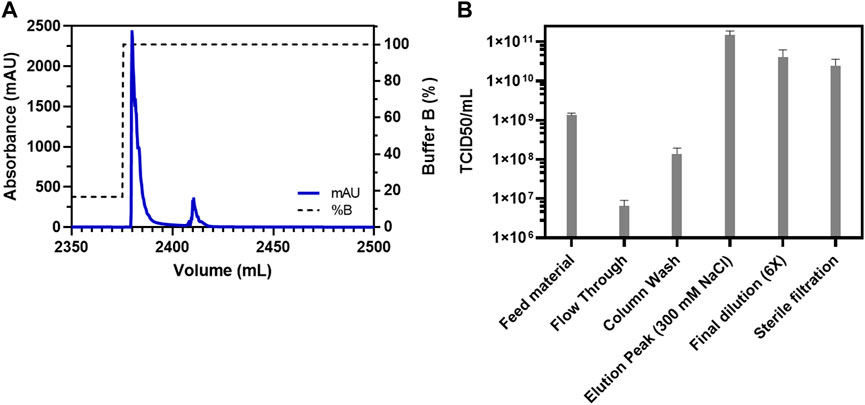
FIGURE 2. Consolidation run for the purification of VSV-GP from crude feed on Sartobind S membrane adsorber. (A) Chromatogram for the purification of VSV-GP using Sartobind S membrane adsorber column. The first peak contained the virus with a volume of 8 ml and (B) TCID50-based infectivity assay for various fractions obtained during purification of VSV-GP in (A) with final a recovery of about 30% in the elution fraction.
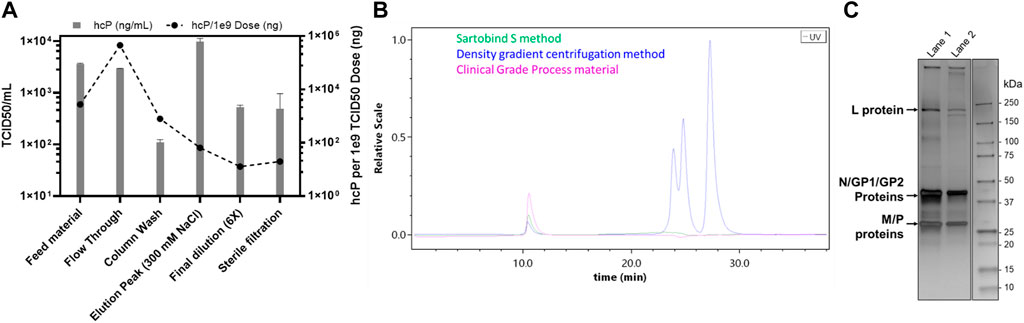
FIGURE 3. Host contaminant analysis for the purification of VSV-GP using Sartobind S membrane adsorber. (A) Host-cell protein ELISA for the various fractions collected during the purification of VSV-GP, (B) analytical SEC for the final VSV-GP preparation as compared to that of purified using a density gradient centrifugation and clinical-grade process, and (C) SDS-PAGE analysis, comparing the VSV-GP purified, using: density gradient centrifugation (lane 1); and Sartobind S method (lane 2). The arrows show the respective viral proteins, L: large polymerase protein; N: nucleoprotein; GP1 and GP2: processed glycoprotein complex proteins pseudotyped from LCMV; and M: matrix protein.
To further substantiate the virus quality, the purified virus was analyzed using cryo-TEM (Figure 4A). Bullet shaped particles at an average size of about 190 nm × 70 nm with interior striated pattern and intact lipid bilayer membrane decorated with surface spikes were observed, consistent with features of intact VSV virus (Ge et al., 2010). VSV-GP purified using the current method correlated well with the expected bullet shaped morphology of VSV-GP virus particles, yielding native bullet shaped particles (Figure 4B).
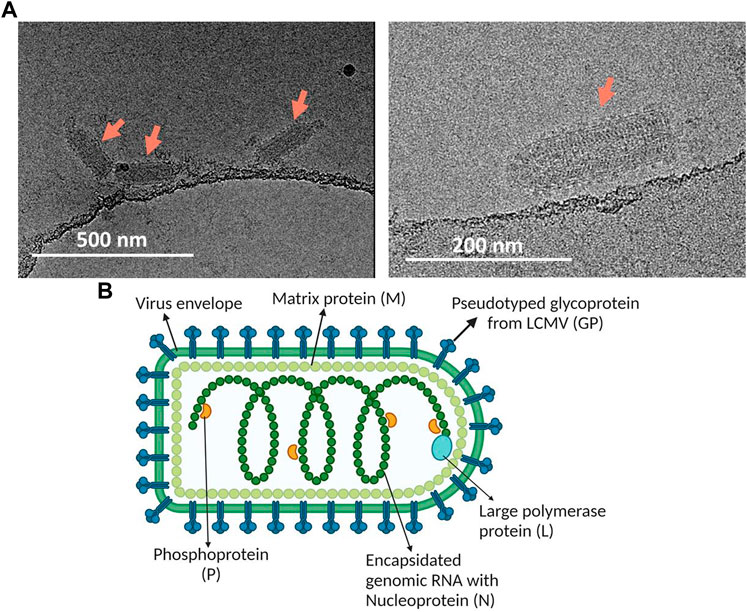
FIGURE 4. (A) Cryo-TEM for morphological characterization of purified VSV-GP using Sartobind S. Arrows point towards these bullet shaped VSV-GP virus particles. (B) Image depicting the structure of VSV-GP along with its essential structural elements. The figure was generated using BioRender.
Finally, we wanted to ascertain that the therapeutic virus was effective in animal models in vivo. Therefore, we tested and compared it with the virus material purified using a comprehensive downstream process (clinical-grade process) currently used for manufacturing at large scale for clinical trials. To address whether the different downstream processes have an impact on the efficacy, tolerability, or activity of the virus, we compared relevant read-outs in BALB/c mice bearing CT26. cl25-IFNAR1−/− tumors (Figure 5). A single systemic VSV-GP-luc injection of 109 TCID50 induced a delay in tumor growth with no difference between our new and clinical-grade process (Figure 5A). This resulted in an identical mean survival benefit of 8 days compared to vehicle-treated animals (Figure 5D). Both test substances were also well tolerated with only a transient weight drop being reported from which all animals recovered within in few days (Figure 5B). After treatment, virus activity was monitored over time via luciferase signal. The respective treatments resulted in comparable tumor-selective bioluminescence signals. These signals increased over several days, peaking at day 4, indicating similar active viral replication (Figures 5C,E).
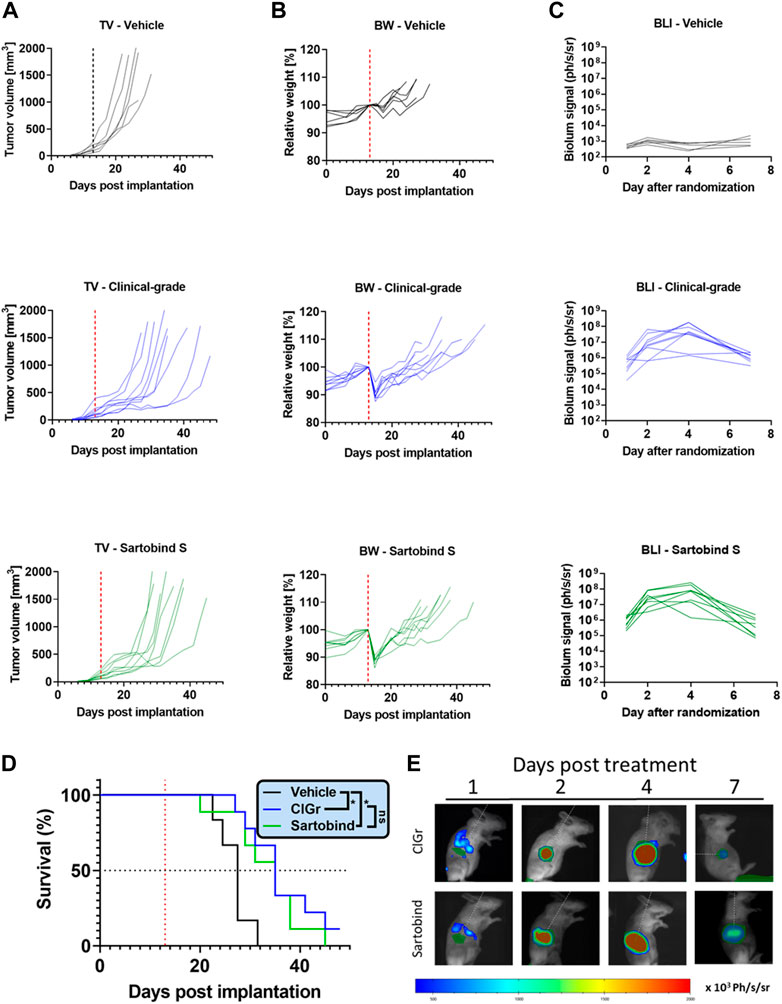
FIGURE 5. In vivo study compares the efficacy, tolerability, and activity of the virus purified using Sartobind S or the clinical-grade process. Tumors were implanted in BALB/c mice by subcutaneously injecting 106 CT26.Cl25-IFNAR1−/− cells. Established CT26.Cl25-IFNAR1−/− tumors were treated intravenously with a single dose of 109 TCID50 VSV-GP-Luc (red dotted line), produced either by the clinical-grade process (ClGr) or Sartobind S-based process (Sartobind). (A) Individual tumor volume (TV) graphs are shown (B) Individual body weight (BW) graphs are shown. (C) Tumors were monitored on day 1, 2, 4, and 7 post treatment using the in vivo bioluminescence imaging (BLI) system IVIS. Quantification of the average radiance in the individual tumor areas are shown. (D) Kaplan–Meier survival curves are shown. Virus treated animals showed a significant survival benefit. Log-rank (Mantel-Cox) test was performed (*p < 0.05) (E) Representative BLI pictures of treated mice are shown. A region of interest (ROI) was drawn on bioluminescent images over the subcutaneous tumors. Luminescence is displayed as photons/second/steradian (p/s/sr).
Discussion
Cation exchange chromatography is a purification platform used frequently for purification of not only proteins and antibodies (Brämer et al., 2019; Boi et al., 2020), but can also be efficiently utilized for the purification of complex viruses, as demonstrated in this study and previously by other authors (Eckhardt et al., 2022; Mueller et al., 2022). For example, Eckhardt et al. (Eckhardt et al., 2022) have used resin based cation exchange chromatography for the purification of measles virus. However, apart from dis-advantages such as lower flow rates limited by diffusion, resin based chromatography media also have drawbacks in terms of disrupting the integrity and infectivity of virus particles due to increased shear stress, especially if process times are rather long (Gagnon, 2008). Moreover, adopting a protocol from one virus to another is extremely challenging and cannot be easily adopted. As it depends on multiple factors such as surface glycoproteins, cell lines used to generate the virus particles, enveloped vs. non-enveloped virus particles, size and shape of the virus particles, etc. (Nestola et al., 2015; Zhou et al., 2020; Wright et al., 2022). Mueller et al. (2022) in their patent application have described a process for the purification of VSV-GP however the current study demonstrates a single step (vs multi step) process using membrane adsorber (vs monolithic) chromatography columns.
There are other chromatographic methods for the purification of certain viruses, such as affinity chromatography which can be highly specific for the target particles with very good removal of contaminants without additional polishing steps (Zhao et al., 2019). However, the disadvantages still outweigh the advantages especially when it comes to the purification of new modalities such as large virus particles, i.e., VSV-GP. The drawbacks include slow and long process due to the time required to develop the affinity molecules or antibodies, scale up challenges, non-optimal reproducibility due to lack of streamlined chromatographic matrix-affinity ligand production, lack of elution due to strong multivalent interaction, etc. Emergence of pseudo-affinity ligands and chromatographic media in the market might tackle some of these challenges but that is something still to be validated (Opitz et al., 2009). Another type of chromatographic technique has emerged for the purification of viruses called steric exclusion chromatography (Lothert et al., 2020). However, this technique also lacks due to the absence of a streamlined raw material supply, scale up and industrial applications, as well as requirement of high viscosity materials during chromatography such as polyethylene glycol (PEG). Ion exchange chromatography coupled with convective flow chromatographic matrix (i.e., membrane adsorber) overcomes these challenges, as it is a well-established technique not just for lab scale but also for industrial scales.
In this study we utilized the capabilities of cation exchange chromatography coupled with convective flow media, i.e., membrane adsorber. This is a very important single use technology along with large pore sizes/channels (Hagemann et al., 2022), allowing an efficient capture/binding of the virus of interest, as well as their efficient purification. The yields obtained for the purified virus (VSV-GP) were also impressive using the method in this study, as compared to other processes (Mueller et al., 2022), while retaining critical quality attributes. Additionally, there was a good reproducibility of the method even with different working volumes of feed when applied to the column along with any observed absence of membrane clogging or fouling (Figures 1, 2). Therefore, this study demonstrates that a simple downstream process can be developed and implemented for the purification and concentration of ATMPs, such as oncolytic viruses. Complex multi-step processes can easily result in reduced infectious virus yields and quality. However, both the yield and quality are extremely important for applications such as oncolytic viruses. Therefore, the current process provides the benefits of a lean downstream process, combined with the higher product quality and yield.
Data availability statement
The original contributions presented in the study are included in the article/Supplementary Material, further inquiries can be directed to the corresponding author.
Ethics statement
Animal housing and experimental procedures were in compliance with French and European regulations and the NRC guide for the care and use of laboratory animals. The animal facility is authorized by the French authorities (Agreement No. C 21 231 011 EA). All animal procedures were submitted to the Institutional Animal Care and Use Committee of Oncodesign (Oncomet) approved by French authorities (CNREEA agreement N° 91).
Author contributions
SG, DX, and BS designed the study. SG, AG, BS, and DX performed the experiments, provided the materials and methods, and analyzed the data. SG, BS, and DX wrote the manuscript. All authors discussed the results and commented on the manuscript.
Acknowledgments
We thank Jara Ballestin and Manuel Garbe for their assistance with cell culture and virus harvesting. We are thankful to Knut Elbers for a review of the manuscript and useful discussions and are also grateful to Patrik Erlmann, Judy Ng, and Tobias Nolden for technical discussions. We also thank Mandy Janssen and Camille Hennessy from Nanoimaging Services for acquiring cryo-TEM data.
Conflict of interest
SG, AG, and BS are employees of ViraTherapeutics GmbH, which is a subsidiary of Boehringer Ingelheim International GmbH. DX is an employee of Boehringer Ingelheim Pharmaceutical, Inc.
The handling editor (MW) declared a past collaboration with the author (SG).
Publisher’s note
All claims expressed in this article are solely those of the authors and do not necessarily represent those of their affiliated organizations, or those of the publisher, the editors and the reviewers. Any product that may be evaluated in this article, or claim that may be made by its manufacturer, is not guaranteed or endorsed by the publisher.
Supplementary material
The Supplementary Material for this article can be found online at: https://www.frontiersin.org/articles/10.3389/fbioe.2022.992069/full#supplementary-material
References
Apolonio, J. S., Gonçalves, V. L. de S., Santos, M. L. C., Luz, M. S., Souza, J. V. S., Pinheiro, S. L. R., et al. (2021). Oncolytic virus therapy in cancer: A current review. World J. Virol. 10 (5), 229–255. doi:10.5501/wjv.v10.i5.229
Atcc, (2022) BHK-21 [C-13]. Available from https://www.atcc.org/products/ccl-10 (Accessed May 25, 2022).
Bandeira, V., Peixoto, C., Rodrigues, A. F., Cruz, P. E., Alves, P. M., Coroadinha, A. S., et al. (2012). Downstream processing of lentiviral vectors: Releasing bottlenecks. Hum. Gene Ther. Methods 23 (4), 255–263. doi:10.1089/hgtb.2012.059
Barber, G. N. (2004). Vesicular stomatitis virus as an oncolytic vector. Viral Immunol. 17 (4), 516–527. doi:10.1089/vim.2004.17.516
Barber, G. N. (2005). VSV-tumor selective replication and protein translation. Oncogene 24 (52), 7710–7719. doi:10.1038/sj.onc.1209042
Boi, C., Malavasi, A., Carbonell, R. G., and Gilleskie, G. (2020). A direct comparison between membrane adsorber and packed column chromatography performance. J. Chromatogr. A 1612, 460629. doi:10.1016/j.chroma.2019.460629
Brämer, C., Tünnermann, L., Gonzalez Salcedo, A., Reif, O. W., Solle, D., Scheper, T., et al. (2019). Membrane adsorber for the fast purification of a monoclonal antibody using protein A chromatography. Membranes 9 (12), 159. doi:10.3390/membranes9120159
Burke, L. M. B., Yu, H., Burke, K., Gwynn, M., Louie, R. J., Ollila, D. W., et al. (2021). Ultrasound-guided intralesional injection of Talimogene laherparepvec (imlygic) for advanced melanoma: Technical note on a preliminary experience. Cardiovasc. Interv. Radiol. 44 (5), 801–806. doi:10.1007/s00270-020-02748-3
Burnie, J. (2022). P-selectin glycoprotein ligand-1 (PSGL-1/CD162) is incorporated into clinical HIV-1 isolates and can mediate virus capture and subsequent transfer to permissive cells. Retrovirology 19, 9. doi:10.1186/s12977-022-00593-5
Cheng, A., Negro, C., Bruhn, J. F., Rice, W. J., Dallakyan, S., Eng, E. T., et al. (2021). Leginon: New features and applications. Protein Sci. 30 (1), 136–150. doi:10.1002/pro.3967
Chevallet, M., Luche, S., and Rabilloud, T. (2006). Silver staining of proteins in polyacrylamide gels. Nat. Protoc. 1 (4), 1852–1858. doi:10.1038/nprot.2006.288
Cramer, S. M., and Holstein, M. A. (2011). Downstream bioprocessing: Recent advances and future promise. Curr. Opin. Chem. Eng. 1 (1), 27–37. doi:10.1016/j.coche.2011.08.008
Dold, C., Rodriguez Urbiola, C., Wollmann, G., Egerer, L., Muik, A., Bellmann, L., et al. (2016). Application of interferon modulators to overcome partial resistance of human ovarian cancers to VSV-GP oncolytic viral therapy. Mol. Ther. - Oncolytics 3, 16021. doi:10.1038/mto.2016.21
Eckhardt, D., Dieken, H., Loewe, D., Grein, T. A., Salzig, D., and Czermak, P. (2022). Purification of oncolytic measles virus by cation-exchange chromatography using resin-based stationary phases. Sep. Sci. Technol. 57 (6), 886–896. doi:10.1080/01496395.2021.1955267
Ema, (2018). Imlygic. European medicines agency. Available from https://www.ema.europa.eu/en/medicines/human/EPAR/imlygic (accessed May 9, 2022).
Gagnon, P. (2008). The emerging generation of chromatography tools for virus purification. BioProcess Int., 24–30.
Ge, P., Tsao, J., Schein, S., Green, T. J., Luo, M., and Zhou, Z. H. (2010). Cryo-EM model of the bullet-shaped vesicular stomatitis virus. Science 327 (5966), 689–693. doi:10.1126/science.1181766
Ghosh, R. (2002). Protein separation using membrane chromatography: Opportunities and challenges. J. Chromatogr. A 952 (1), 13–27. doi:10.1016/s0021-9673(02)00057-2
Hagemann, F., Wypysek, D., Baitalow, K., Adametz, P., Thom, V., and Wessling, M. (2022). Why device design is crucial for membrane adsorbers. J. Chromatogr. Open 2, 100029. doi:10.1016/j.jcoa.2021.100029
Heo, J., Reid, T., Ruo, L., Breitbach, C. J., Rose, S., Bloomston, M., et al. (2013). Randomized dose-finding clinical trial of oncolytic immunotherapeutic vaccinia JX-594 in liver cancer. Nat. Med. 19 (3), 329–336. doi:10.1038/nm.3089
Hochdorfer, D., Businger, R., Hotter, D., Seifried, C., and Solzin, J. (2022). Automated, label-free TCID50 assay to determine the infectious titer of virus-based therapeutics. J. Virol. Methods 299, 114318. doi:10.1016/j.jviromet.2021.114318
Hoenen, T., Groseth, A., and Feldmann, H. (2012). Current ebola vaccines. Expert Opin. Biol. Ther. 12 (7), 859–872. doi:10.1517/14712598.2012.685152
Jacquemart, R., Vandersluis, M., Zhao, M., Sukhija, K., Sidhu, N., and Stout, J. (2016). A single-use strategy to enable manufacturing of affordable biologics. Comput. Struct. Biotechnol. J. 14, 309–318. doi:10.1016/j.csbj.2016.06.007
Jungbauer, A., and Hahn, R. (2009). “Chapter 22 ion-exchange chromatography,” in Methods in enzymology. Editors R. R. Burgess, and M. P. Deutscher. 2nd Edition (Massachusetts, United States: Academic Press), 349–371.
Kärber, G. (1931). Beitrag zur kollektiven Behandlung pharmakologischer Reihenversuche. Arch. F. Exp. Pathol. U. Pharmakol. 162 (4), 480–483. doi:10.1007/bf01863914
Kaufman, H. L., Kohlhapp, F. J., and Zloza, A. (2015). Oncolytic viruses: A new class of immunotherapy drugs. Nat. Rev. Drug Discov. 14 (9), 642–662. doi:10.1038/nrd4663
Kimpel, J., Urbiola, C., Koske, I., Tober, R., Banki, Z., Wollmann, G., et al. (2018). The oncolytic virus VSV-GP is effective against malignant melanoma. Viruses 10 (3), 108. doi:10.3390/v10030108
Kis, Z., Shattock, R., Shah, N., and Kontoravdi, C. (2019). Emerging Technologies for low-cost, rapid vaccine manufacture. Biotechnol. J. 14 (1), 1800376. doi:10.1002/biot.201800376
Kramberger, P., Urbas, L., and Štrancar, A. (2015). Downstream processing and chromatography based analytical methods for production of vaccines, gene therapy vectors, and bacteriophages. Hum. Vaccin. Immunother. 11 (4), 1010–1021. doi:10.1080/21645515.2015.1009817
Loewe, D., Häussler, J., Grein, T. A., Dieken, H., Weidner, T., Salzig, D., et al. (2019). Forced degradation studies to identify critical process parameters for the purification of infectious measles virus. Viruses 11 (8), 725. doi:10.3390/v11080725
Lothert, K., Sprick, G., Beyer, F., Lauria, G., Czermak, P., and Wolff, M. W. (2020). Membrane-based steric exclusion chromatography for the purification of a recombinant baculovirus and its application for cell therapy. J. Virol. Methods 275, 113756. doi:10.1016/j.jviromet.2019.113756
Macedo, N., Miller, D. M., Haq, R., and Kaufman, H. L. (2020). Clinical landscape of oncolytic virus research in 2020. J. Immunother. Cancer 8 (2), e001486. doi:10.1136/jitc-2020-001486
Mueller, D., Garcia, A. P., Grein, T. A., Kaess, F., Ng, J., Pesta, K., et al. (2022). Process for producing a purified rhabdovirus from cell culture. Available from: https://patents.google.com/patent/US20220010286A1/en?oq=20220010286 (Accessed Sep 26, 2022).
Mueller, P., Erlmann, P., Petersson, M., Nolden, T., Urbiola, C., Schreiber, L. M., et al. (2019). Abstract 2267: Therapeutic synergy of the chimeric, oncolytic virus VSV-GP with immune modulators. Cancer Res. 79, 2267. doi:10.1158/1538-7445.am2019-2267
Muik, A., Dold, C., Geiß, Y., Volk, A., Werbizki, M., Dietrich, U., et al. (2012). Semireplication-competent vesicular stomatitis virus as a novel platform for oncolytic virotherapy. J. Mol. Med. 90 (8), 959–970. doi:10.1007/s00109-012-0863-6
Muik, A., Stubbert, L. J., Jahedi, R. Z., Geiβ, Y., Kimpel, J., Dold, C., et al. (2014). Re-Engineering vesicular stomatitis virus to abrogate neurotoxicity, circumvent humoral immunity, and enhance oncolytic potency. Cancer Res. 74 (13), 3567–3578. doi:10.1158/0008-5472.can-13-3306
Nestola, P., Peixoto, C., Silva, R. R. J. S., Alves, P. M., Mota, J. P. B., and Mjt, C. (2015). Improved virus purification processes for vaccines and gene therapy. Biotechnol. Bioeng. 112 (5), 843–857. doi:10.1002/bit.25545
Opitz, L., Lehmann, S., Reichl, U., and Wolff, M. W. (2009). Sulfated membrane adsorbers for economic pseudo-affinity capture of influenza virus particles. Biotechnol. Bioeng. 103 (6), 1144–1154. doi:10.1002/bit.22345
Pereira Aguilar, P., Reiter, K., Wetter, V., Steppert, P., Maresch, D., Ling, W. L., et al. (2020). Capture and purification of Human Immunodeficiency Virus-1 virus-like particles: Convective media vs porous beads. J. Chromatogr. A 1627, 461378. doi:10.1016/j.chroma.2020.461378
Schneider, C. K., Salmikangas, P., Jilma, B., Flamion, B., Todorova, L. R., Paphitou, A., et al. (2010). Challenges with advanced therapy medicinal products and how to meet them. Nat. Rev. Drug Discov. 9 (3), 195–201. doi:10.1038/nrd3052
Schreiber, L. M., Urbiola, C., Das, K., Spiesschaert, B., Kimpel, J., Heinemann, F., et al. (2019). The lytic activity of VSV-GP treatment dominates the therapeutic effects in a syngeneic model of lung cancer. Br. J. Cancer 121 (8), 647–658. doi:10.1038/s41416-019-0574-7
Singh, N., and Heldt, C. L. (2022). Challenges in downstream purification of gene therapy viral vectors. Curr. Opin. Chem. Eng. 35, 100780. doi:10.1016/j.coche.2021.100780
Spearman, C. (1908). The method of ‘right and wrong cases’ (‘constant stimuli’) without gauss’s formulae. Br. J. Psychol. 1904-1920 2 (3), 227–242. doi:10.1111/j.2044-8295.1908.tb00176.x
Sridhar, S. (2015). Clinical development of Ebola vaccines. Ther. Adv. Vaccines 3 (5–6), 125–138. doi:10.1177/2051013615611017
Suloway, C., Pulokas, J., Fellmann, D., Cheng, A., Guerra, F., Quispe, J., et al. (2005). Automated molecular microscopy: The new Leginon system. J. Struct. Biol. X. 151 (1), 41–60. doi:10.1016/j.jsb.2005.03.010
ThermoFisher, (2022) 293-F cells. Available from: https://www.thermofisher.com/uk/en/home/technical-resources/cell-lines/2/cell-lines-detail-29.html (Accessed May 25, 2022).
Tober, R., Banki, Z., Egerer, L., Muik, A., Behmüller, S., Kreppel, F., et al. (2014). VSV-GP: A potent viral vaccine vector that boosts the immune response upon repeated applications. J. Virol. 88 (9), 4897–4907. doi:10.1128/jvi.03276-13
Ungerechts, G., Bossow, S., Leuchs, B., Holm, P. S., Rommelaere, J., Coffey, M., et al. (2016). Moving oncolytic viruses into the clinic: Clinical-grade production, purification, and characterization of diverse oncolytic viruses. Mol. Ther. - Methods & Clin. Dev. 3, 16018. doi:10.1038/mtm.2016.18
Working, P. K., Lin, A., and Borellini, F. (2005). Meeting product development challenges in manufacturing clinical grade oncolytic adenoviruses. Oncogene 24 (52), 7792–7801. doi:10.1038/sj.onc.1209045
Workman, P., Aboagye, E. O., Balkwill, F., Balmain, A., Bruder, G., Chaplin, D. J., et al. (2010). Guidelines for the welfare and use of animals in cancer research. Br. J. Cancer 102 (11), 1555–1577. doi:10.1038/sj.bjc.6605642
Wright, E., Kawka, K., Medina, M. F. C., and Latulippe, D. R. (2022). Evaluation of host cell impurity effects on the performance of sterile filtration processes for therapeutic viruses. Membranes 12 (4), 359. doi:10.3390/membranes12040359
Yang, H. (2013). Establishing acceptable limits of residual DNA. PDA J. Pharm. Sci. Technol. 67 (2), 155–163. doi:10.5731/pdajpst.2013.00910
Zhao, M., Vandersluis, M., Stout, J., Haupts, U., Sanders, M., and Jacquemart, R. (2019). Affinity chromatography for vaccines manufacturing: Finally ready for prime time? Vaccine 37 (36), 5491–5503. doi:10.1016/j.vaccine.2018.02.090
Keywords: oncolytic viruses, cation-exchange chromatography, membrane adsorbers, sartobind S, downstream processing, VSV-GP, cryo-EM of viruses, virus analytics and characterization
Citation: Gautam S, Xin D, Garcia AP and Spiesschaert B (2022) Single-step rapid chromatographic purification and characterization of clinical stage oncolytic VSV-GP. Front. Bioeng. Biotechnol. 10:992069. doi: 10.3389/fbioe.2022.992069
Received: 12 July 2022; Accepted: 13 October 2022;
Published: 28 October 2022.
Edited by:
Michael Wolff, Technische Hochschule Mittelhessen, GermanyReviewed by:
Ricardo J. S. Silva, Instituto de Biologia e Tecnologia Experimental (iBET), PortugalRoland Pach, Roche, Switzerland
Egbert Müller, Tosoh Bioscience gmbh, Germany
Copyright © 2022 Gautam, Xin, Garcia and Spiesschaert. This is an open-access article distributed under the terms of the Creative Commons Attribution License (CC BY). The use, distribution or reproduction in other forums is permitted, provided the original author(s) and the copyright owner(s) are credited and that the original publication in this journal is cited, in accordance with accepted academic practice. No use, distribution or reproduction is permitted which does not comply with these terms.
*Correspondence: Saurabh Gautam, Z2F1dGFtc2F1cmFiaDEyQGdtYWlsLmNvbQ==