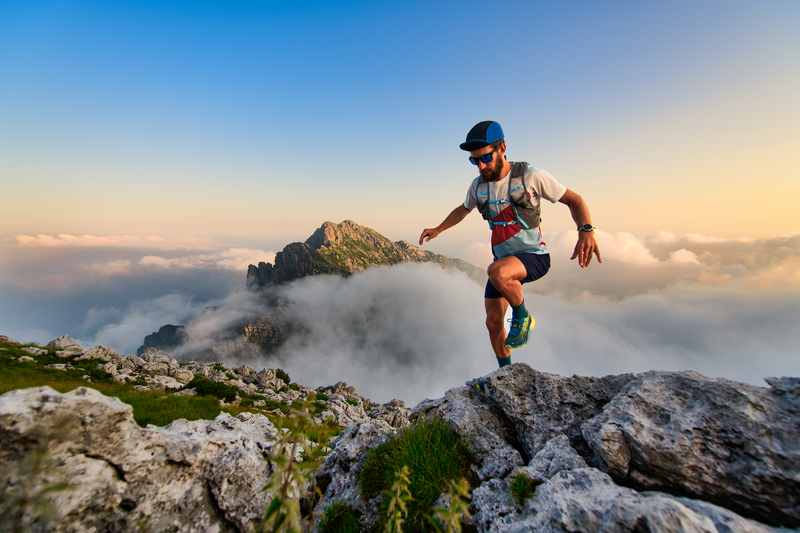
95% of researchers rate our articles as excellent or good
Learn more about the work of our research integrity team to safeguard the quality of each article we publish.
Find out more
ORIGINAL RESEARCH article
Front. Bioeng. Biotechnol. , 02 September 2022
Sec. Industrial Biotechnology
Volume 10 - 2022 | https://doi.org/10.3389/fbioe.2022.987796
This article is part of the Research Topic Advances and Trends in Microbial Production of Biopolymers and Their Building Blocks View all 11 articles
Polysaccharides are important natural biomacromolecules. In particular, microbial exopolysaccharides have received much attention. They are produced by a variety of microorganisms, and they are widely used in the food, pharmaceutical, and chemical industries. The Candida glabrata mutant 4-C10, which has the capacity to produce exopolysaccharide, was previously obtained by random mutagenesis. In this study we aimed to further enhance exopolysaccharide production by systemic fermentation optimization. By single factor optimization and orthogonal design optimization in shaking flasks, an optimal fermentation medium composition was obtained. By optimizing agitation speed, aeration rate, and fed-batch fermentation mode, 118.6 g L−1 of exopolysaccharide was obtained by a constant rate feeding fermentation mode, with a glucose yield of 0.62 g g−1 and a productivity of 1.24 g L−1 h−1. Scaling up the established fermentation mode to a 15-L fermenter led to an exopolysaccharide yield of 113.8 g L−1, with a glucose yield of 0.60 g g−1 and a productivity of 1.29 g L−1 h−1.
Polysaccharides are important natural biomacromolecules. They are widely present in microorganisms, plants, and animals (Liao et al., 2022; Rajoka et al., 2022). Because of the unique physical and chemical characteristics, such as degradability, bioactivity, nontoxicity, and biocompatibility, polysaccharides are widely applied in the food, pharmaceutical, and chemical industries, where they serve as texture enhancers, stabilizers, gelatinizing agents, emulsifiers, drug carriers, etc. (Kokoulin et al., 2021; Yang et al., 2021; Zhang et al., 2022). Recently, microbial polysaccharides have attracted extensive attention from researchers for their anti-tumor, anti-oxidation, hypoglycemic, and immune-enhancing activities (Saadat et al., 2019; Zeng Y. J. et al., 2019). Microbial polysaccharides are traditionally divided into three types: intracellular polysaccharides, cell wall polysaccharides, and secreted exopolysaccharide (Nachtigall et al., 2020; Tian et al., 2021). In particular, exopolysaccharides have broad application prospects and have attracted increasing attention because of advantages such as relatively high titer, simple separation, and easy large-scale production (Zeng Y. J. et al., 2019).
Exopolysaccharide could be synthesized by various microorganisms, including bacteria, archaea, yeast, fungi, and microalgae (Nambiar et al., 2018; Schmid, 2018). Generally, exopolysaccharides with various structures and high molecular weights are produced and released into the external environment during the process of cell growth or in response to changes in the surrounding environments, such as pH, temperature, ionic strength, and nutritional content (Ayyash et al., 2020; Gan et al., 2020). With the increasing demand for natural biopolymers for diverse industrial applications, various types of exopolysaccharides from different microbial species were investigated and applied (Becker, 2015; Schmid, 2018); for example, hyaluronic acid produced by Streptococcus zooepidemicus (Mohan et al., 2022), xanthan gum produced by Xanthomonas campestris (Wang Z. C. et al., 2016), and scleroglucan produced by Sclerotium rolfsii (Tan et al., 2019). Some kinds of exopolysaccharides have also been isolated from different yeast species, such as Rhodotorula mucilaginosa (Ma et al., 2018) and Zygosaccharomyces rouxii (Zhang et al., 2022).
Candida glabrata is a classical nonconventional yeast and also a multi-vitamin auxotrophic yeast (Raschmanova et al., 2018). Due to the easy cultivation process, the simple culture medium, and the possibility to use renewable resources, C. glabrata has become the dominant producer for the important chemical compound pyruvic acid with the microbiological fermentation route (Luo et al., 2019; Guo et al., 2020a). To enhance the production of pyruvic acid, several strategies have been tried, including selecting high-producing strains combined with high-throughput screening and mutagenesis approaches, metabolic engineering to strengthen the synthesis pathway and extracellular transport, and systematic optimization of the fermentation process (Luo et al., 2018; Guo et al., 2020a; Luo et al., 2020). Interestingly, the C. glabrata mutant strain 4-C10, which has the capacity to accumulate exopolysaccharide, was obtained by screening for high pyruvic acid production with random mutagenesis in our previous work. The monosaccharide components and formation mechanism of the exopolysaccharide were also analyzed. By knocking out the genes involved in exopolysaccharide synthesis, the pyruvic acid production was enhanced, while the exopolysaccharide accumulation was decreased (Luo et al., 2017a; Luo et al., 2017b).
To further enhance the production of exopolysaccharide with the obtained mutant, C. glabrata 4-C10, a systematic optimization of the fermentation process should be conducted. In the present study, based on the employed fermentation medium compositions for pyruvic acid production, suitable fermentation medium compositions for exopolysaccharide production were first obtained with single factor optimization and orthogonal design optimization at the shaking flask level. Then, the effects of agitation speed and aeration rate on exopolysaccharide accumulation were investigated in a 1-L fermenter, and a constant rate feeding fermentation mode was established. The production of exopolysaccharide reached 118.6 g L−1. Finally, the established constant rate feeding fermentation mode was scaled up to a 15-L fermenter. The exopolysaccharide production and the product yield were basically stable, laying a foundation for industrial production.
The producer, C. glabrata 4-C10 (CCTCC M2017047), used in this research is a four-vitamin auxotrophic strain (thiamine, biotin, niacin, and pyridoxine), which was previously obtained by random mutagenesis in C. glabrata (CCTCC M202019) (Luo et al., 2017a; Luo et al., 2017b).
The medium for slant and seeds were consisted of (gL−1): glucose 30, plant protein extract 10, KH2PO4 1.0 and MgSO4·7H2O 0.5. Additionally, 20 g L−1 of agar was needed to add in the slant medium. The initial fermentation medium consisted of (gL−1): glucose 120, urea 3.84, KH2PO4 2.0, MgSO4·7H2O 0.8, CH3COONa 3, CaCO3 40. The trace element mixture (10 ml L−1) and vitamin mixture (6 ml L−1) were added separately. The optimized fermentation medium contained (gL−1): glucose 150, urea 5.0, KH2PO4 3.0, MgSO4·7H2O 0.9, CH3COONa 3, CaCO3 40. The trace element mixture (10 ml L−1) and vitamin mixture (6 ml L−1) were also added separately. The trace element mixture was dissolved with 2 M of HCl, consisting of (gL−1): MnCl2·4H2O 12, FeSO4·7H2O 2, CaCl2·2H2O 2, CuSO4·5H2O 0.05, ZnCl2 0.5. The vitamin mixture was dissolved with 2 M of HCl, consisting of (gL−1): biotin 0.004, thiamine 0.00075, pyridoxine 0.04, niacin 0.8 (Luo et al., 2017a).
C. glabrata 4-C10 was incubated in seed culture medium at 30°C for 16 h. The seed cultures and fermentation cultivation were implemented in 500 ml shaking flasks consisting of 50 ml of culture medium at 30°C on a reciprocal shaker at 220 rmin−1 (Zhichu, Shanghai, China). Batch fermentation and fed-batch fermentation were performed in a 1-L parallel bioreactor (T&J Bio-engineering, Shanghai, China) with a 0.6-L working volume. The agitation speed, aeration rate, and fed-batch mode were adjusted (see Results and discussion section). Fermentation was conducted in a 15-L bioreactor (T&J Bio-engineering, Shanghai, China) containing a 12-L working volume with an agitation speed of 500 rmin−1, 1.0 vvm, and a pressure of 0.035 MPa. The pH was automatically kept at 5.5 by adding 8 M of NaOH. The inoculation size was 10% (v/v) and all cultivations were carried out at 30°C (Luo et al., 2017a; Guo et al., 2020b).
Determination of biomass: The different samples taken in the fermentation process were all diluted to a certain concentration with 2 M HCl. The optical density (OD) of the diluted fermentation broth was detected using a Biospe-1601 spectrophotometer (Shimadzu Co., Kyoto, Japan) at 660 nm. The dry cell weight (DCW) was calculated with the relationship DCW = 0.23×OD660. (Luo et al., 2020).
Determination of glucose and pyruvic acid: The glucose was detected with a glucose-lactate biosensor (Sieman Technology, Shenzhen, China), and the pyruvic acid were detected by high-performance liquid chromatography (HPLC, Agilent 1260, CA, United States) with a UV detector at 210 nm with an Aminex HPX-87H column (Bio-Rad, CA, United States). The specific detection conditions were as follows: an injection volume of 10 μl, a mobile phase of 5 mM H2SO4, a flow rate of 0.5 ml min−1, and a column temperature of 40°C (Zhou et al., 2009).
Determination of the concentration of exopolysaccharide: The different samples of fermentation broths were centrifuged at 5000 × g for 15 min. Then, two volumes of precooled ethanol (4°C) were added to the obtained supernatant. After 1 h, the precipitate was centrifuged at 5000 × g for 15 min. The exopolysaccharide concentration was determined after the precipitate was freeze-dried to a constant weight (Luo et al., 2017a).
All fermentation processes were carried out in triplicate and the results were presented as mean values. The orthogonal experiment was designed by Design Expert and the experimental data were analyzed by Origin 2019b.
For the biosynthesis of specific target metabolites by microbial fermentation, the composition of fermentation medium is usually the preferred optimization factor to enhance the production during the fermentation process (Xin et al., 2017; Wagh et al., 2022). For pyruvic acid production, the optimization of fermentation medium composition and process control was systematically conducted, and significant enhancements in titer and productivity was obtained (Xu et al., 2009; Guo et al., 2020b). For exopolysaccharide production, the optimal addition of vitamin mixture has also been determined (Luo et al., 2017a). To further enhance the exopolysaccharide accumulation, the concentrations of some important components of the medium could be investigated.
Based on the previously obtained initial fermentation medium, the influence of different medium components (glucose, urea, MgSO4·7H2O, and KH2PO4) on exopolysaccharide accumulation is shown in Figure 1. Increasing glucose concentrations result in enhanced exopolysaccharide production. When the concentration was 140 g L−1, the exopolysaccharide production was highest, reaching 74.4 g L−1. The effect of urea addition on exopolysaccharide production was not significant; the production was highest when the concentration was 2 g L−1. A relatively high exopolysaccharide production was obtained when the MgSO4·7H2O concentration was 0.6 g L−1. A high exopolysaccharide production could be obtained when the KH2PO4 concentration was 1 g L−1 or 3 g L−1, while the exopolysaccharide production decreased when the KH2PO4 concentration was further increased. To obtain the optimal combination, an orthogonal experiment for addition of glucose, urea, MgSO4·7H2O, and KH2PO4 was designed. Results showed that 89.7 g L−1 of exopolysaccharide was harvested with a composition of 150 g L−1 of glucose, 5 g L−1 of urea, 0.9 g L−1 of MgSO4·7H2O, and 3 g L−1 of KH2PO4 (Table S1). Statistical analysis showed that the effect of the initial glucose concentration on exopolysaccharide accumulation is extremely significant (Table S2).
FIGURE 1. Effects of medium components on exopolysaccharide accumulation in flasks. (A) Effects of glucose concentration on exopolysaccharide accumulation. (B) Effects of urea concentration on exopolysaccharide accumulation. (C) Effects of MgSO4·7H2O concentration on exopolysaccharide accumulation. (D) Effects of KH2PO4 concentration on exopolysaccharide accumulation.
To enhance the exopolysaccharide production, the cultivation condition was further optimized based on obtaining the optimal fermentation medium compositions. The influences of different agitation speeds (300 rmin−1, 400 rmin−1, 500 rmin−1, and 600 rmin−1) on exopolysaccharide production in a 1-L fermenter are shown in Figure 2. With the increase of agitation speed, the biomass and the exopolysaccharide production improved. The highest exopolysaccharide production was obtained when the agitation speed was 600 rmin−1, reaching 97.9 g L−1 with a glucose yield of 0.65 g g−1. In addition, the time profiles of the by-product pyruvic acid accumulation showed the same trend under different agitation speeds. The accumulation gradually increased and then decreased, but the fermentation time for pyruvic acid to peak value gradually shortened.
FIGURE 2. Time courses of exopolysaccharide production at different agitation speeds in a 1-L fermenter. (A) 1.0 vvm and 300 rmin−1. (B) 1.0 vvm and 400 rmin−1. (C) 1.0 vvm and 500 rmin−1. (D) 1.0 vvm and 600 rmin−1. Black down triangles, glucose; orange up triangles, pyruvic acid; red circles, DCW; blue squares, exopolysaccharide.
Agitation speed is an important parameter affecting the dissolved oxygen level during the fermentation process (Huang et al., 2020; Fang et al., 2021). Considering the cultivation mode without pressure on the glass fermenter, it is generally preferred to optimize the agitation speed. There have been many examples of improving the accumulation of specific target products by systematically optimizing the agitation speed. By establishing a two-stage agitation speed controlling strategy, the production and molecular weight of pullulan with Aureobasidium pullulans were simultaneously enhanced (Wang D. H. et al., 2016). By studying the influence of the agitation speed, an optimal agitation speed was obtained for 1,3-propanediol production with Shimwellia blattae (Rodriguez et al., 2016). C. glabrata has been proved to require a relatively high agitation speed for pyruvic acid production (Luo et al., 2018; Guo et al., 2020b). The results obtained in this study indicated that a higher stirring speed was also beneficial to the accumulation of polysaccharides.
To investigate the influence of aeration rate on exopolysaccharide accumulation, three different aeration rates (0.5 vvm, 1.0 vvm, and 1.5 vvm) were selected. The results are shown in Figure 3. When the aeration rate was controlled at a relatively low value (0.5 vvm), the growth rate and the glucose consumption rate were obviously lower than under the other two conditions (1.0 vvm and 1.5 vvm). When the aeration rate was 1.5 vvm, the highest exopolysaccharide production was just 65.8 g L−1, which was significantly lower than at 0.5 vvm and 1.0 vvm. Exopolysaccharide yields of 87.2 g L−1 and 97.9 g L−1 were obtained under 0.5 vvm and 1.0 vvm, respectively.
FIGURE 3. Time courses of exopolysaccharide production at different aeration rates in a 1-L fermenter. (A) 600 rmin−1 and 0.5 vvm. (B) 600 min−1 and 1.0 vvm. (C) 600 rmin−1 and 1.5 vvm. Black down triangles, glucose; orange up triangles, pyruvic acid; red circles, DCW; blue squares, exopolysaccharide.
Aeration rate is another important parameter affecting the dissolved oxygen level during the fermentation process. In many fermentation processes, the supply of oxygen plays a crucial role in the growth of cells and the synthesis of specific target compounds (Alonso et al., 2012; Fang et al., 2021). Generally, the optimization of aeration rate is combined with the agitation speed for co-regulating dissolved oxygen levels. A series of combinatorial optimization strategies have been applied for the synthesis of some important products (Wang et al., 2010; Song et al., 2018). A high dissolved oxygen level could promote cell growth and pyruvic acid production at the expense of glucose consumption in C. glabrata, and a strategy has been used to reduce the dependence of cells on oxygen (Luo et al., 2019; Luo et al., 2020). Notably, a high aeration rate was detrimental to exopolysaccharide production, as shown in Figure 3.
After the optimization of agitation speed and aeration rate in the 1-L fermenter, feeding strategies were tried to further improve the accumulation of exopolysaccharide, such as single-dose fed-batch mode and constant rate feeding mode. The initial concentration of glucose was 150 g L−1 and the total concentration was 190 g L−1. When the residual glucose concentration was reduced to 15–20 g L−1, an additional 40 g L−1 of glucose was fed by a single dose at 56 h and by a constant rate of 1.43 g L−1 h−1 during 56–84 h, respectively (Figure 4). It was shown that the constant rate feeding mode yielded a better result, with a highest exopolysaccharide production of 118.6 g L−1, a glucose yield of 0.62 g g−1, and a productivity of 1.24 g L−1 h−1, which were all higher than those of by the single-dose fed-batch mode (with a highest exopolysaccharide production of 97.7 g L−1, a glucose yield of 0.51 g g−1, and a productivity of 1.02 g L−1 h−1).
FIGURE 4. Effects of different feeding strategies on exopolysaccharide production in a 1-L fermenter. The initial concentration of glucose was 150 g L−1, and then 40 g L−1 of glucose was fed with different feeding strategies. (A) One-dose feeding fermentation mode of 40 g L−1 glucose at 56 h. (B) Constant rate feeding fermentation mode of 40 g L−1 glucose during 56–84 h. Black down triangles, glucose; orange up triangles, pyruvic acid; red circles, DCW; blue squares, exopolysaccharide.
The fed-batch fermentation mode is a common fermentation strategy in the field of microbial industrial fermentation. It can be divided into a variety of feeding modes, including single-dose fed-batch mode, intermittent fed-batch mode, constant rate feeding mode, exponential feeding mode, etc. (Zeng W. Z. et al., 2019; Wang et al., 2020; de Oliveira et al., 2021). These established feeding modes have been widely applied to improve the production, yield, and productivity of some specific target products, such as 2-phenylethanol, scleroglucan, and keto acids (Zeng et al., 2017; Tan et al., 2019; Tian et al., 2020). However, it should be noted that the optimal feeding mode for industrial microorganisms to produce diverse metabolites is different. In this study, the constant rate feeding mode was proved more suitable for exopolysaccharide production in C. glabrata, but the fed-batch strategy could be optimized more detailedly in the future, for example by comparing other feeding modes or combining with other strategies in the fed-batch process.
In order to verify the constant rate feeding mode under the conditions that were closest to industrial production, the established process was further scaled up to a 15-L fermenter. As in the 1-L fermenter, the aeration rate was controlled at 1.0 vvm and 40 g L−1 was fed with a constant rate of 1.43 g L−1 h−1 during 56–84 h. The agitation speed was maintained at 500 rmin−1 under a fermenter pressure of 0.035 MPa. The result is shown in Figure 5. The highest exopolysaccharide titer was obtained at 88 h, reaching 113.8 g L−1 with a glucose yield of 0.60 g g−1 and a productivity of 1.29 g L−1 h−1. Compared with the constant rate feeding mode in the 1-L fermenter, the exopolysaccharide production was slightly decreased. Additionally, during the fermentation process in the 15-L fermenter, the highest and the final accumulation of by-product pyruvic acid were also obviously less than in the 1-L fermenter. The specific fermentation characteristics in 1-L and 15-L fermenters are presented in Table 1.
FIGURE 5. Time course of exopolysaccharide accumulation in a 15-L fermenter. The initial concentration of glucose was 150 g L−1, and then 40 g L−1 glucose was fed constantly during 56–84 h. Fermentation was conducted at 30°C, 500 rmin−1, and 1.0 vvm for 96 h. Black down triangles, glucose; orange up triangles, pyruvic acid; red circles, DCW; blue squares, exopolysaccharide.
The development of commercial production methods of a specific product with microorganism fermentation is a long amplification process, from microtiter plates, to shaking flasks, to bench-scale fermenters, to pilot-scale fermenters, and ultimately to commercial fermenters (Wehrs et al., 2019; Ganeshan et al., 2021). The scaling up of the fermentation process for a target product is a systematic project, but not simply a matter of increasing cultivation and vessel volume (Hewitt & Nienow, 2007; Krajang et al., 2021). A reliable scaling up process at the lab scale should reasonably solve the problem from shaking flasks to bench-scale fermenters, particularly the fermenters equipped with pressure control. A series of scaling up processes has been published for some exopolysaccharides, such as glucan, xanthan gum, levan, and pullulan (Freitas et al., 2017; Bzducha-Wrobel et al., 2018; Vaishnav et al., 2022). In this study, the exopolysaccharide production after scaling up to a 15-L fermenter was slightly decreased. But the time to reach the maximum was shortened, thereby achieved a relatively higher space time yield. To further enhance the production, the feeding rate and the glucose concentration could be appropriately increased in the fed-batch process.
In this study, the fermentation medium components were optimized in shaking flasks to enhance exopolysaccharide production with C. glabrata strain 4-C10, which was obtained by random mutagenesis. By optimizing the agitation speed and aeration rate and establishing a constant rate feeding fermentation mode in a 1-L fermenter, the exopolysaccharide titer was enhanced to 118.6 g L−1, with a glucose yield of 0.62 g g−1 and a productivity of 1.24 g L−1 h−1. After scaling up to a 15-L fermenter, the production was slightly decreased, reaching 113.8 g L−1. The obtained results could provide references for industrial exopolysaccharide production.
The original contributions presented in the study are included in the article/Supplementary Material, further inquiries can be directed to the corresponding author.
SX: methodology, investigation, formal analysis, writing—original draft. JX: investigation. WZ: supervision, writing—review and editing, validation. XS: methodology, investigation. JZ: supervision, funding acquisition, writing—review and editing.
This work was supported by the National Key Research and Development Program of China (2021YFC2101400), and the Foundation for Innovative Research Groups of the National Natural Science Foundation of China (32021005).
The authors declare that the research was conducted in the absence of any commercial or financial relationships that could be construed as a potential conflict of interest.
All claims expressed in this article are solely those of the authors and do not necessarily represent those of their affiliated organizations, or those of the publisher, the editors and the reviewers. Any product that may be evaluated in this article, or claim that may be made by its manufacturer, is not guaranteed or endorsed by the publisher.
The Supplementary Material for this article can be found online at: https://www.frontiersin.org/articles/10.3389/fbioe.2022.987796/full#supplementary-material
Alonso, S., Rendueles, M., and Diaz, M. (2012). Role of dissolved oxygen availability on lactobionic acid production from whey by Pseudomonas taetrolens. Bioresour. Technol. 109, 140–147. doi:10.1016/j.biortech.2012.01.045
Ayyash, M., Abu-Jdayil, B., Itsaranuwat, P., Almazrouei, N., Galiwango, E., Esposito, G., et al. (2020). Exopolysaccharide produced by the potential probiotic Lactococcus garvieae C47: Structural characteristics, rheological properties, bioactivities and impact on fermented camel milk. Food Chem. x. 333, 127418. doi:10.1016/j.foodchem.2020.127418
Becker, A. (2015). Challenges and perspectives in combinatorial assembly of novel exopolysaccharide biosynthesis pathways. Front. Microbiol. 6, 687. doi:10.3389/fmicb.2015.00687
Bzducha-Wrobel, A., Pobiega, K., Blazejak, S., and Kieliszek, M. (2018). The scale-up cultivation of Candida utilis in waste potato juice water with glycerol affects biomass and beta(1, 3)/(1, 6)-glucan characteristic and yield. Appl. Microbiol. Biotechnol. 102 (21), 9131–9145. doi:10.1007/s00253-018-9357-y
de Oliveira, P. M., Santos, L. P., Coelho, L. F., Neto, P. M. A., Sass, D. C., and Contiero, J. (2021). Production of L (+) lactic acid by Lactobacillus casei Ke11: Fed batch fermentation strategies. Ferment. (Basel). 7 (3), 151. doi:10.3390/fermentation7030151
Fang, J., Zeng, W. Z., and Zhou, J. W. (2021). Breeding and process optimization of Yarrowia lipolytica for high-yield α-ketoglutaric acid production. Food Fermen Ind. 47 (2), 137–144. doi:10.13995/j.cnki.11-1802/ts.024665
Freitas, F., Torres, C. A. V., and Reis, M. A. M. (2017). Engineering aspects of microbial exopolysaccharide production. Bioresour. Technol. 245, 1674–1683. doi:10.1016/j.biortech.2017.05.092
Gan, L. Z., Li, X. G., Wang, H. B., Peng, B. Y., and Tian, Y. Q. (2020). Structural characterization and functional evaluation of a novel exopolysaccharide from the moderate halophile Gracilibacillus sp. SCU50. Int. J. Biol. Macromol. 154, 1140–1148. doi:10.1016/j.ijbiomac.2019.11.143
Ganeshan, S., Kim, S. H., and Vujanovic, V. (2021). Scaling-up production of plant endophytes in bioreactors: Concepts, challenges and perspectives. Bioresour. Bioprocess. 8 (1), 63. doi:10.1186/s40643-021-00417-y
Guo, L. K., Zeng, W. Z., Xu, S., and Zhou, J. W. (2020a). Fluorescence-activated droplet sorting for enhanced pyruvic acid accumulation by Candida glabrata. Bioresour. Technol. 318, 124258. doi:10.1016/j.biortech.2020.124258
Guo, L. K., Zeng, W. Z., and Zhou, J. W. (2020b). Process optimization of fed-batch fermentation for pyruvic acid production with Candida glabrata. Food Fermen Ind. 46 (7), 10–16. doi:10.13995/j.cnki.11-1802/ts.022872
Hewitt, C. J., and Nienow, A. W. (2007). The scale-up of microbial batch and fed-batch fermentation processes. Adv. Appl. Microbiol. 62, 105–135. doi:10.1016/S0065-2164(07)62005-X
Huang, M. W., Chen, H. Q., Tang, X., Lu, H. Q., Zhao, J. X., Zhang, H., et al. (2020). Two-stage pH control combined with oxygen-enriched air strategies for the highly efficient production of EPA by Mortierella alpina CCFM698 with fed-batch fermentation. Bioprocess Biosyst. Eng. 43 (9), 1725–1733. doi:10.1007/s00449-020-02367-9
Kokoulin, M. S., Kuzmich, A. S., Romanenko, L. A., and Chikalovets, I. V. (2021). Structure and in vitro antiproliferative activity of the acidic capsular polysaccharide from the deep-sea bacterium Psychrobacter submarinus KMM 225T. Carbohydr. Polym. 262, 117941. doi:10.1016/j.carbpol.2021.117941
Krajang, M., Malairuang, K., Sukna, J., Rattanapradit, K., and Chamsart, S. (2021). Single-step ethanol production from raw cassava starch using a combination of raw starch hydrolysis and fermentation, scale-up from 5-L laboratory and 200-L pilot plant to 3000-L industrial fermenters. Biotechnol. Biofuels 14 (1), 68. doi:10.1186/s13068-021-01903-3
Liao, Y. T., Gao, M., Wang, Y. T., Liu, X. Z., Zhong, C., and Jia, S. R. (2022). Structural characterization and immunomodulatory activity of exopolysaccharide from Aureobasidium pullulans CGMCC 23063. Carbohydr. Polym. 288, 119366. doi:10.1016/j.carbpol.2022.119366
Luo, Z. S., Liu, S., Du, G. C., Xu, S., Zhou, J. W., and Chen, J. (2018). Enhanced pyruvate production in Candida glabrata by carrier engineering. Biotechnol. Bioeng. 115 (2), 473–482. doi:10.1002/bit.26477
Luo, Z. S., Liu, S., Du, G. C., Zhou, J. W., and Chen, J. (2017a). Identification of a polysaccharide produced by the pyruvate overproducer Candida glabrata CCTCC M202019. Appl. Microbiol. Biotechnol. 101 (11), 4447–4458. doi:10.1007/s00253-017-8245-1
Luo, Z. S., Zeng, W. Z., Du, G. C., Chen, J., and Zhou, J. (2019). Enhanced pyruvate production in Candida glabrata by engineering ATP futile cycle system. ACS Synth. Biol. 8 (4), 787–795. doi:10.1021/acssynbio.8b00479
Luo, Z. S., Zeng, W. Z., Du, G. C., Chen, J., and Zhou, J. W. (2020). Enhancement of pyruvic acid production in Candida glabrata by engineering hypoxia-inducible factor 1. Bioresour. Technol. 295, 122248. doi:10.1016/j.biortech.2019.122248
Luo, Z. S., Zeng, W. Z., Du, G. C., Liu, S., Fang, F., Zhou, J. W., et al. (2017b). A high-throughput screening procedure for enhancing pyruvate production in Candida glabrata by random mutagenesis. Bioprocess Biosyst. Eng. 40 (5), 693–701. doi:10.1007/s00449-017-1734-x
Ma, W. J., Chen, X. F., Wang, B., Lou, W. J., Chen, X., Hua, J. L., et al. (2018). Characterization, antioxidativity, and anti-carcinoma activity of exopolysaccharide extract from Rhodotorula mucilaginosa CICC 33013. Carbohydr. Polym. 181, 768–777. doi:10.1016/j.carbpol.2017.11.080
Mohan, N., Pavan, S. S., Jayakumar, A., Rathinavelu, S., and Sivaprakasam, S. (2022). Real-time metabolic heat-based specific growth rate soft sensor for monitoring and control of high molecular weight hyaluronic acid production by Streptococcus zooepidemicus. Appl. Microbiol. Biotechnol. 106 (3), 1079–1095. doi:10.1007/s00253-022-11760-1
Nachtigall, C., Surber, G., Herbi, F., Wefers, D., Jaros, D., and Rohm, H. (2020). Production and molecular structure of heteropolysaccharides from two lactic acid bacteria. Carbohydr. Polym. 236, 116019. doi:10.1016/j.carbpol.2020.116019
Nambiar, R. B., Sellamuthu, P. S., Perumal, A. B., Sadiku, E. R., Phiri, G., and Jayaramudu, J. (2018). Characterization of an exopolysaccharide produced by Lactobacillus plantarum HM47 isolated from human breast milk. Process Biochem. 73, 15–22. doi:10.1016/j.procbio.2018.07.018
Rajoka, M. S. R., Mehwish, H. M., Kitazawa, H., Barba, F. J., Berthelot, L., Umair, M., et al. (2022). Techno-functional properties and immunomodulatory potential of exopolysaccharide from Lactiplantibacillus plantarum MM89 isolated from human breast milk. Food Chem. x. 377, 131954. doi:10.1016/j.foodchem.2021.131954
Raschmanova, H., Weninger, A., Glieder, A., Kovar, K., and Vogl, T. (2018). Implementing CRISPR-Cas technologies in conventional and non-conventional yeasts: Current state and future prospects. Biotechnol. Adv. 36 (3), 641–665. doi:10.1016/j.biotechadv.2018.01.006
Rodriguez, A., Wojtusik, M., Ripoll, V., Santos, V. E., and Garcia-Ochoa, F. (2016). 1, 3-Propanediol production from glycerol with a novel biocatalyst Shimwellia blattae ATCC 33430: Operational conditions and kinetics in batch cultivations. Bioresour. Technol. 200, 830–837. doi:10.1016/j.biortech.2015.10.061
Saadat, Y. R., Khosroushahi, A. Y., and Gargari, B. P. (2019). A comprehensive review of anticancer, immunomodulatory and health beneficial effects of the lactic acid bacteria exopolysaccharides. Carbohydr. Polym. 217, 79–89. doi:10.1016/j.carbpol.2019.04.025
Schmid, J. (2018). Recent insights in microbial exopolysaccharide biosynthesis and engineering strategies. Curr. Opin. Biotechnol. 53, 130–136. doi:10.1016/j.copbio.2018.01.005
Song, X. Q., Zhang, Y., Xue, J. Y., Li, C., Wang, Z. J., and Wang, Y. H. (2018). Enhancing nemadectin production by Streptomyces cyaneogriseus ssp noncyanogenus through quantitative evaluation and optimization of dissolved oxygen and shear force. Bioresour. Technol. 255, 180–188. doi:10.1016/j.biortech.2017.09.033
Tan, R. Q., Lyu, Y. B., Zeng, W. Z., and Zhou, J. W. (2019). Enhancing scleroglucan production by Sclerotium rolfsii WSH-G01 through a pH-shift strategy based on kinetic analysis. Bioresour. Technol. 293, 122098. doi:10.1016/j.biortech.2019.122098
Tian, J. J., Zhang, C. P., Wang, X. M., Rui, X., Zhang, Q. Q., Chen, X. H., et al. (2021). Structural characterization and immunomodulatory activity of intracellular polysaccharide from the mycelium of Paecilomyces cicadae TJJ1213. Food Res. Int. 147, 110515. doi:10.1016/j.foodres.2021.110515
Tian, S. F., Liang, X. L., Chen, J., Zeng, W. Z., Zhou, J. W., and Du, G. C. (2020). Enhancement of 2-phenylethanol production by a wild-type Wickerhamomyces anomalus strain isolated from rice wine. Bioresour. Technol. 318, 124257. doi:10.1016/j.biortech.2020.124257
Vaishnav, A., Upadhyay, K., Koradiya, M., Tipre, D., and Dave, S. (2022). Valorisation of fruit waste for enhanced exopolysaccharide production by Xanthomonas campestries using statistical optimisation of medium and process. Food Biosci. 46, 101608. doi:10.1016/j.fbio.2022.101608
Wagh, V. S., Said, M. S., Bennale, J. S., and Dastager, S. G. (2022). Isolation and structural characterization of exopolysaccharide from marine Bacillus sp. and its optimization by Microbioreactor. Carbohydr. Polym. 285, 119241. doi:10.1016/j.carbpol.2022.119241
Wang, D. H., Bian, J. J., Wei, G. Y., Jiang, M., and Dong, M. S. (2016). Simultaneously enhanced production and molecular weight of pullulan using a two-stage agitation speed control strategy. J. Chem. Technol. Biotechnol. 91 (2), 467–475. doi:10.1002/jctb.4600
Wang, X. L., Zhou, J. J., Shen, J. T., Zheng, Y. F., Sun, Y. Q., and Xiu, Z. L. (2020). Sequential fed-batch fermentation of 1, 3-propanediol from glycerol by Clostridium butyricum DL07. Appl. Microbiol. Biotechnol. 104 (21), 9179–9191. doi:10.1007/s00253-020-10931-2
Wang, Y. H., Fang, X. L., Li, Y. P., and Zhang, X. (2010). Effects of constant and shifting dissolved oxygen concentration on the growth and antibiotic activity of Xenorhabdus nematophila. Bioresour. Technol. 101 (19), 7529–7536. doi:10.1016/j.biortech.2010.04.070
Wang, Z. C., Wu, J. R., Zhu, L., and Zhan, X. B. (2016). Activation of glycerol metabolism in Xanthomonas campestris by adaptive evolution to produce a high-transparency and low-viscosity xanthan gum from glycerol. Bioresour. Technol. 211, 390–397. doi:10.1016/j.biortech.2016.03.096
Wehrs, M., Tanjore, D., Eng, T., Lievense, J., Pray, T. R., and Mukhopadhyay, A. (2019). Engineering robust production microbes for large-scale cultivation. Trends Microbiol. 27 (6), 524–537. doi:10.1016/j.tim.2019.01.006
Xin, F. X., Chen, T. P., Jiang, Y. J., Lu, J. S., Dong, W. L., Zhang, W. M., et al. (2017). Enhanced biobutanol production with high yield from crude glycerol by acetone uncoupled Clostridium sp strain CT7. Bioresour. Technol. 244, 575–581. doi:10.1016/j.biortech.2017.08.002
Xu, Q. L., Xu, X. P., Liu, L. M., Shi, Z. P., Du, G. C., and Chen, J. (2009). Effects of environmental conditions on pyruvate production. Chin. J. Bioproc Eng. 7 (1), 13–18.
Yang, X. L., Wei, S. Q., Lu, X. M., Qiao, X. G., Simal-Gandara, J., Capanoglu, E., et al. (2021). A neutral polysaccharide with a triple helix structure from ginger: Characterization and immunomodulatory activity. Food Chem. x. 350, 129261. doi:10.1016/j.foodchem.2021.129261
Zeng, W. Z., Cai, W., Liu, L., Du, G. C., Chen, J., and Zhou, J. W. (2019). Efficient biosynthesis of 2-keto-D-gluconic acid by fed-batch culture of metabolically engineered Gluconobacter japonicus. Synth. Syst. Biotechnol. 4 (3), 134–141. doi:10.1016/j.synbio.2019.07.001
Zeng, W., Zhang, H., Xu, S., Fang, F., and Zhou, J. (2017). Biosynthesis of keto acids by fed-batch culture of Yarrowia lipolytica WSH-Z06. Bioresour. Technol. 243, 1037–1043. doi:10.1016/j.biortech.2017.07.063
Zeng, Y. J., Yang, H. R., Zong, M. H., Yang, J. G., and Lou, W. Y. (2019). Novel antibacterial polysaccharides produced by endophyte Fusarium solani DO7. Bioresour. Technol. 288, 121596. doi:10.1016/j.biortech.2019.121596
Zhang, M., Che, Y. L., and Wu, C. D. (2022). A novel exopolysaccharide produced by Zygosaccharomyces rouxii with cryoprotective and freeze-drying protective activities. Food Chem. x. 392, 133304. doi:10.1016/j.foodchem.2022.133304
Keywords: Candida glabrata, exopolysaccharide, medium composition, fed-batch fermentation, overproduction
Citation: Xu S, Xu J, Zeng W, Shan X and Zhou J (2022) Efficient biosynthesis of exopolysaccharide in Candida glabrata by a fed-batch culture. Front. Bioeng. Biotechnol. 10:987796. doi: 10.3389/fbioe.2022.987796
Received: 06 July 2022; Accepted: 08 August 2022;
Published: 02 September 2022.
Edited by:
Guang Zhao, Shandong University, ChinaCopyright © 2022 Xu, Xu, Zeng, Shan and Zhou. This is an open-access article distributed under the terms of the Creative Commons Attribution License (CC BY). The use, distribution or reproduction in other forums is permitted, provided the original author(s) and the copyright owner(s) are credited and that the original publication in this journal is cited, in accordance with accepted academic practice. No use, distribution or reproduction is permitted which does not comply with these terms.
*Correspondence: Jingwen Zhou, emhvdWp3MTk4MkBqaWFuZ25hbi5lZHUuY24=
Disclaimer: All claims expressed in this article are solely those of the authors and do not necessarily represent those of their affiliated organizations, or those of the publisher, the editors and the reviewers. Any product that may be evaluated in this article or claim that may be made by its manufacturer is not guaranteed or endorsed by the publisher.
Research integrity at Frontiers
Learn more about the work of our research integrity team to safeguard the quality of each article we publish.