- 1Research Center for Prevention of Oral and Dental Diseases, Baqiyatallah University of Medical Sciences, Tehran, Iran
- 2Department of Oral and Maxillofacial Surgery, School of Dentistry, Shahid Beheshti University of Medical Sciences, Tehran, Iran
- 3Department of Prosthodontics, School of Dentistry, Shahid Beheshti University of Medical Sciences, Tehran, Iran
- 4Department of Restorative Dentistry, School of Dentistry, Ardabil University of Medical Sciences, Ardabil, Iran
- 5Orthodontic Department, Dental School, Bushehr University of Medical Sciences, Bushehr, Iran
- 6Department of Science and Research, Islimic Azade University, Tehran, Iran
- 7Dental Research Center, Zahedan University of Medical Sciences, Zahedan, Iran
The state-of-the-art approach to regenerating different tissues and organs is tissue engineering which includes the three parts of stem cells (SCs), scaffolds, and growth factors. Cellular behaviors such as propagation, differentiation, and assembling the extracellular matrix (ECM) are influenced by the cell’s microenvironment. Imitating the cell’s natural environment, such as scaffolds, is vital to create appropriate tissue. Craniofacial tissue engineering refers to regenerating tissues found in the brain and the face parts such as bone, muscle, and artery. More biocompatible and biodegradable scaffolds are more commensurate with tissue remodeling and more appropriate for cell culture, signaling, and adhesion. Synthetic materials play significant roles and have become more prevalent in medical applications. They have also been used in different forms for producing a microenvironment as ECM for cells. Synthetic scaffolds may be comprised of polymers, bioceramics, or hybrids of natural/synthetic materials. Synthetic scaffolds have produced ECM-like materials that can properly mimic and regulate the tissue microenvironment’s physical, mechanical, chemical, and biological properties, manage adherence of biomolecules and adjust the material’s degradability. The present review article is focused on synthetic materials used in craniofacial tissue engineering in recent decades.
Introduction
Over the last 30–40 years, synthetic materials have become more prevalent in medical and dental repair applications (Zaszczynska et al., 2020). Generally, biocompatibility, composition, and products released after the decomposition of the scaffolds are highly dependent on the primary materials used for their fabrication (Eltom et al., 2019). Developing biodegradable synthetic scaffolds has omitted the need for additional surgery to remove the guidance conduit (Marti et al., 2013). Hydrogels are porous hydrophilic polymer constructs that efficiently transmit water, gas, and nutrients highly required for cultured cells (Maisani et al., 2017). Hydrogels can also be solidified through a chemical or non-chemical phase transformation; however, they are usually printed in the liquid state (Vega et al., 2017; Francis et al., 2018). The recent substantial progress in synthetic scaffolds has led to producing ECM-like materials that can properly mimic and regulate the tissue microenvironment, manage adherence of biomolecules and adjust the material’s degradability (Oksdath et al., 2018). These controllable properties provide essential advantages for craniofacial tissue engineering (TE). Another reason for the growing application of synthetic materials in dental and oral regeneration is their outstanding reproducibility, immunogenicity, and degradation rates (Marti et al., 2013). TE mainly aims to restore, preserve, or improve the structure and/or function of the damaged tissues due to disease or trauma using scaffolds with or without cells and biochemical factors (Sergey, 2018; Jenkins and Little, 2019). In one meaning, TE occurs at the cross-section of life science and engineering and, importantly, involves the cell-ECM interactions. Involving proteoglycans and fibrous proteins, ECM mainly supports the cells’ structure and morphology, migration or attachment, differentiation, and immune response (Heboyan and Avetisyan, 2019a; Jenkins and Little, 2019; Tanweer et al., 2022; Vardhan et al., 2022; Yadalam et al., 2022). Cells’ homing and proliferation properties highly depend on their microenvironment, and in turn, the biocompatibility of the counterpart artificial ECM. Bioengineered and cell-free scaffolds are two types of artificial ECM. The former is usually supplied with cells, growth factors, cytokines, and/or genetic material before implant (Yaremenko et al., 2018; Soufdoost et al., 2019; Mosaddad et al., 2020; Soufdoost et al., 2020). The three-dimensional (3D) porous biodegradable scaffold is a modern TE technique in clinical dentistry that overcomes the drawbacks of previously introduced cancellous or particulate graft materials. Such scaffolds provide the proper physical space for regenerating the craniofacial tissues, prevent the regeneration site from undesired cells’ invasion, help the endogenous osteogenic cells to reside, grow, and differentiate in the site (Yaremenko et al., 2018; Heboyan and Avetisyan, 2019b; Heboyan, 2019; Heboyan et al., 2019; Abhay et al., 2021; Heboyan et al., 2021a; Avetisyan et al., 2021; Durairaj et al., 2021; Heboyan et al., 2022a; Ahmed et al., 2022; Baskaran et al., 2022; Dam et al., 2022; Gopalakrishnan et al., 2022). The most recent cell-scaffold technique is the personalized scaffold that is fabricated from synthetic polymers such as polycaprolactone (PCL) by 3D bioprinting systems (3DPs) in the precise shape and size. They present valuable features such as thermoplasticity, biocompatibility, biodegradability, and the potential of layer-by-layer processing which have provided a wide application for them in the scaffold fabrication area (Yaremenko et al., 2018). Accordingly, the promising outcomes of TE and clinical regenerative medicine, especially for bone regeneration purposes, have prompted researchers and clinicians to develop such intervention strategies for repairing complicated craniofacial defects despite their associated limitations with synthetic materials. Different synthetic materials and scaffolds have been developed to overcome these limitations. In this review, the recent progressions are discussed in dental and oral regenerative medicine using synthetics materials and scaffolds.
Craniofacial tissue engineering
The craniomaxillofacial region contains various tissues such as cranial and facial bones, skin, muscles, blood vessels, tendons, ligaments, and nerves (Gaihre et al., 2017; Marya et al., 2022a; Marya et al., 2022b; Ghandhi et al., 2022). As is general in various TE techniques, stem cell (SC), scaffold, and growth factor (Dissanayaka et al., 2015) are the three essential components required for craniofacial TE (Gao et al., 2014). 3D porous bioscaffolds are introduced as appropriate matrixes for this purpose that ideally support attachment, propagation, and differentiation of cells, developing new blood vessels and forming new tissue. In the development of medical technology tailored to each patient’s needs, 3D/4D printing can be combined with artificial intelligence and machine learning (Tatullo et al., 2019; Rokaya et al., 2022). Mesenchymal stem cells (MSCs) are a promising and easily accessible cellular component for craniofacial TE, which, despite their limited self-renewal, they are capable of multi-lineage differentiation. Therefore, different tissues, including bone, cartilage, muscle, fat, nerve, and vessels, can be developed during craniofacial regeneration using MSCs; however, they require a considerable time to expand and undergo aging (Cooper et al., 2019; Soudi et al., 2021; Hussain et al., 2022). In the following, several tissues are present in the craniofacial part of the body, and the studies aimed at regenerating them are implied.
Muscle
Muscle TE provides attractive models for disease studies and drug screening, regenerative therapies, and meat production (van der Schaft et al., 2013). Various materials have been used as a scaffold for skeletal muscle TE from synthetic polymers or biomimetic materials. The polymers mainly used for such purposes include polypyrrole (PPy), polyglycolide (PGA), polycaprolactone (PCL), and decellularized ECM materials (Grasman et al., 2015; MacQueen et al., 2019; Saberi et al., 2019). At the same time, the biomimetic scaffolds used for the same aim include ECM derivatives such as collagen (COL), fibrin (the most abundant structural protein in skeletal muscle), polysaccharides such as HA, CS, keratin, alginate, fibrous gelatin, and decellularized matrices (Fuoco et al., 2016; Laternser et al., 2018; Nakayama et al., 2019). The specific skeletal muscle from the craniofacial region has been mostly regenerated using PCL-COL nanofibers as a promising scaffold that correctly simulates the skeletal muscle structure and promotes MSCs and myoblasts to align parallelly (Witt et al., 2017). Acellular skeletal muscle ECM has also been reported as a promising scaffold for musculoskeletal TE (Cheng et al., 2014).
Regarding the cells for craniofacial skeletal muscle regeneration, autologous laryngeal muscle repair and reconstruction have been done by primary multi-lineage progenitor cells (Brookes et al., 2018). Also, the gingiva-derived mesenchymal stem cells (GMSCs) can remodel scar/fibrosis and regulate the function of endogenous myoblast progenitor cells to facilitate tongue regeneration (Xu et al., 2017a). Other SCs, including adipose-derived stem cells (ADSCs), induced pluripotent stem cells (iPSCs), and embryonic stem cells (ESCs), have also been used for craniofacial skeletal muscle regeneration (del Carmen Ortuño‐Costela et al., 2019; Kesireddy, 2016; Christ et al., 2015).
Bone
The previous limitations and problems with the incorporation of cells, osteoconductivity, osteogenicity, and osteoinductivity in traditional grafts have triggered several pieces of multidisciplinary research on developing new tissue grafts and scaffolds over the last decade. Researchers from various specialties, such as clinicians, engineers, material scientists, chemists, and molecular biologists, have attempted to produce allogenic bone grafts using either mineralized or demineralized bone matrixed GFs, and SCs (Figure 1) (Gali et al., 2018). In addition, structural, mechanical, and spatial stimulation of the signal required for proper bone regeneration is exerted via developing natural-based nanocomposites (Gali et al., 2018; Fragogeorgi et al., 2019). Despite the significant limitations of bone TE, such as lack of reproducibility, long-term viability, osseointegration, degree of internal revascularization, and anastomotic potential, ongoing research continues to construct an ideal engineered bone tissue (Mercado-Pagán et al., 2015). In this regard, several groups have utilized the calvarial bone defect in rodent species to assess various types of scaffolds in combination with or absence of cells or GFs (McGovern et al., 2018). The strategies for bone repair are categorized into eight groups based on the trauma extent they cause, including singular synthetic substitution, fibrous/nonfibrous biomimetic substitutes, scaffold-active molecule combination, nano-medicine, SC-based products, 3D cell-printing biomaterials, bioactive composites (can be either porous polymers or inorganic), and finally, nano-scaffolds combined with magnetic field and SCs (Ansari, 2019). Moreover, some ECM cell-binding proteins (e.g., COL I and vitronectin) have been shown to have osteogenic potential (Lopes et al., 2018). The bone TE paradigm comprises an osteoinductive scaffold, an osteogenic cell source, and a bioreactor that provides biophysical stimuli and improves transport. Graft survival and bone regeneration in vivo could be enhanced by promoting the formation of functional blood vessels perfused with blood and by stimulating the natural sequence of inflammatory and anti-inflammatory signals that positively interact with the inflammatory response (Ng et al., 2017).
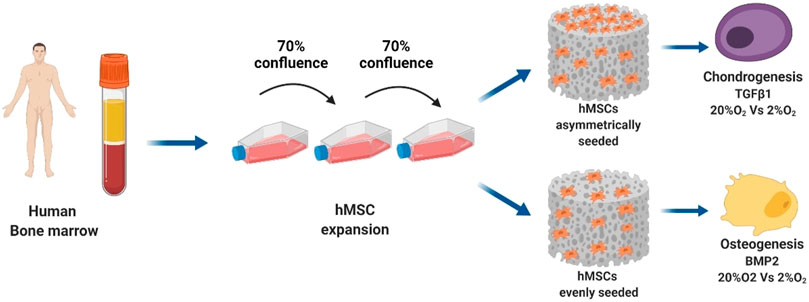
FIGURE 1. The bone TE paradigm. Scaffold, stem cells, and bioreactor culture systems enable the growth of grafts in vitro for bone and cartilage repair applications in vivo (Monaco et al., 2021).
Currently, autografts and allografts are used for craniofacial bone TE, but reconstruction with these standard methods may lead to donor site morbidity and flaws to host tooth aesthetic characteristics (Gaihre et al., 2017). In addition, conventional treatments using bone TE scaffolds face challenges such as high expenses, complicated fabrication, unmanageable degradation, inflammation risk, and immunity rejection that may require debriding by surgery (Fu et al., 2019). Alg/HAp/SF are examples of effective scaffolds for bone TE applications (Figure 2) (Jo et al., 2017).
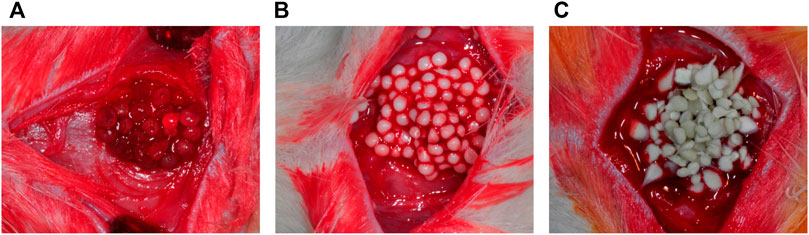
FIGURE 2. (A) Alginate; (B) HAp/Alg; and (C) Silk Fibroin/Hydroxyapatite particles/Alginate beads were grafted into rat calvarial defects (Jo et al., 2017).
Biomaterials used in TE should provide certain properties in biological, mechanical, and osteoinductive qualities. Documented interactions between various biomaterials and targeted cell types get involved in the selection scaffold for different clinical cases (Roi et al., 2019). The signaling mechanisms contributing to the coupled angiogenesis and osteogenesis during bone healing involve several cell types, including MSCs, osteoblasts, osteoclasts, endothelial cells, and osteoprogenitor cells (Marrella et al., 2018). In addition, several growth factors (frequently BMPs) are used in TE. Some other examples are FGF-2, VEGF, TGF-β1, -β2, and -β3, PDGF, IGF-1, and SDF-1 (Samorezov and Alsberg, 2015). PTH1-34 is another GF shown to increase the MSCs’ proliferation and differentiation in the primary phases of bone healing (Takahata et al., 2012). BMP-2, BMP-4, BMP-7, and rhPGDFBB are the main GFs used in clinical procedures (Ansari, 2019). Finally, there is a considerable number of methods available for fabricating bone TE scaffolds. Some examples are electrospun nanofibrous scaffolds and rapid prototyping technologies such as SLA, DLP, SLS, and 3DP (Yuan et al., 2017; Udomluck et al., 2020). Bone matrix production shows differences in endochondral and intramembranous grafts suggesting a site-specific bone formation pattern based on the form of cranial bone ossification (Thompson et al., 2017). Osteoconduction and osteoinduction properties of demineralized bone matrix (DBM), especially from cortical bone have made it a proper osteoinductive matrix (Ansari, 2019). HAp crystals and dentin matrix proteins (DMPs) are prerequisites for forming vertebrate bio-minerals (Ravindran and George, 2015). Other supporting factors for bone formation are MSCs combined with α-TCP that help bone TE strategies to be favorably comparable with autogenous bone transfer (Raposo-Amaral et al., 2014). Also, recent studies introduce bisphosphonates as beneficial reagents for bone TE, especially when associated with composite 3D scaffolds (Cattalini et al., 2012).
Cartilage
Hyaline cartilage is an avascular tissue characterized by copious ECM, primarily composed of COLII and PG, and the chondrocyte, which can retainthe integrated form of ECM (Phull et al., 2016; Vaca-González et al., 2019). Several defects may occur for articular cartilage in terms of depth, size, and the extension of injury that necessitate providing effective medications to repair and restore the defects. The platforms used as a framework for articular TE should have essential qualities such as porosity and inter-connection to conduct cell differentiation and support tissue mechanical properties. Moreover, cell attachment is guaranteed by the surface properties of the materials and scaffolds. Biocompatibility, biodegradability, and non-toxicity of byproducts during the matrix degradation are other necessities for proper contact with the in situ native compartments such as COL fibers (Doulabi et al., 2014; Wang et al., 2018a). Chondrocytes are also used in scaffold-based approaches, including porous matrixes that form neo-cartilage within the pores after degrading the scaffold. Generally, two types of scaffolds are used in cartilage TE: microporous scaffolds (hydrogels) and macroporous scaffolds. Hydrogels can contain water as much as articular cartilage making them a standard option for cartilage regeneration in vitro and in situ (Xiao et al., 2013; Bernhard and Vunjak-Novakovic, 2016; Huang et al., 2016). However, using hydrogels obtained from solubilized dECM is commonly associated with poor mechanical properties and dense ECM that challenges the cartilage decellularization and later cell seeding (Kim et al., 2019a). One of the few methods that have successfully improved the limitations of hydrogels is making pepsin-soluble hydrogels obtained from decellularized cartilage and ligament ECM. Although, the chondrogenesis may decrease in this method compared to the gelatin methacrylate (GelMA) method (Cheng et al., 2014). To compensate for this limitation, several chondrogenic factors such as transforming growth factor-beta (TGF-β), fibroblast growth factors (FGFs), insulin-like growth factor-1 (IGF-1), wingless-type (Wnt) family members, and cartilage-derived matrix proteins (CDMPs) have been used (Grasman et al., 2015; Brookes et al., 2018). Nanofibers, developed with electro-spinning advances, have been broadly connected in cartilage TE (Eftekhari et al., 2020). Bone-like scaffolds and self-organized biphasic cartilage in conjugation with a HAp layer are other materials used for improving articular cartilage injuries and osteochondral defects (Kumai et al., 2019). Additionally, photo-cross-linkable chondroitin sulfate-methacrylate (CSMA) and methacrylated hyaluronic acid (MAHA) have shown the ability to strengthen the mechanical properties of cell-based hybrid-structured scaffolds for cartilage TE in vitro and in vivo (Kim et al., 2017a).
Temporomandibular joint disc
Mandibular jaw joints or TMJs, including the mandibular condyle’s cartilage, are mainly composed of fibrous tissue rather than hyaline cartilage at the articular surfaces. They have special features such as embryonic origin deriving from cranial neural crest (CNC) cells, lower COLI content, and COLII-free superficial layers (Suzuki and Iwata, 2016). TMJ may be conflicted with a heterogeneous cluster of diseases such as severe osteoarthritis and rheumatoid arthritis and disability of masticatory functions due to weakening. TMJ disc has a unique structure involving condyle and glenoid fossa that is appropriately fit at their articular surfaces (Wang et al., 2012). Temporomandibular disorders (TMD) affect joints and their associated structures, as in two-third of cases, the TMJ disc is involved (Matuska et al., 2016; Rokaya et al., 2018a). Simple treatments can mainly treat TMDs, but no successful substitution is available for TMJ disks yet (Ângelo et al., 2017). However, regenerative medicine attempts to provide a clinical alternative to TMJ disc resection using complex biochemical and biomechanical in vitro approaches (Willard et al., 2012). The significant challenges in this regard are the lack of adequate collagen production and the inability to imitate native mechanical properties. On the contrary, biomaterial approaches have been likely more successful in imitating the native features of TMJ disc, but their viability in vivo remains to be determined (Lowe and Almarza, 2017). For example, a unique scaffold has been designed by Van Bellinghen et al., which is suggested to be a perfect approach for TMJ restoration. This complex scaffold is functionalized with dynamic osteochondral molecular gradients such as IGF-1, TGF-1, and bFGF and contains a single SC population that can differentiate toward osteogenesis and chondrogenesis. These characteristics help orchestrate a natural-like 3D arrangement of TMJ tissues (Van Bellinghen et al., 2018). Other methods used for TMJ disk regeneration include matrix-associated chondrocyte transplantation, biologic ECM scaffold obtained from the xenogeneic urinary bladder, acellular scaffold implantation, avascular graft scaffold, and TMJ-derived synovial stem cells (TMJ-SDSCs) (Brown et al., 2012; Xiao, 2014; Bhumiratana et al., 2016; Akar et al., 2018; Undt et al., 2018). Poly glycerol sebacate (PGS) is another potential material to be used as a scaffold for TMJ disc TE (Hagandora et al., 2013). Moreover, Wu et al. could repair the TMJ disc perforation by seeding TMJ synovium-derived MSCs in a fibrin/chitosan scaffold (Wu et al., 2014). Recent studies have also demonstrated the potential of a cell-seeded non-woven mesh made of PGA for application as a scaffold for TMJ disc TE (Mehrotra, 2013). In another report, primary chondrocytes driving from distinctive zones in articular cartilage were seeded in layers in an agarose hydrogel. The coming about neocartilage imitated zonal biochemical and cellular organization (DuRaine et al., 2015). Scaffolds have been used with hyaline cartilage and condylar cartilage to compare their regenerated tissues’ quality and collagen level. While the fibrocartilaginous tissue regenerated by hyaline cartilage-seeded scaffold contained both COLI and COLII, the fibrous tissue regenerated by condylar cartilage-seeded scaffolds contained COLI (Murphy et al., 2013). As a result, TMJ’s condylar nature is supposed to be better regenerated using the condylar cartilage-seeded scaffolds, in contrast to the ankle with a hyaline cartilage nature.
Salivary glands
Progress in regenerative pharmaceuticals has encouraged studies on improving bioengineered SG TE for transplantation, which might give a selective venue for treating xerostomia (Gao et al., 2014). Non-epithelial stem cells can be differentiated into structurally and functionally effective acinar cells, potentially substituting damaged or lost SG tissues (Xu et al., 2017b).
Skin
Collagen is the most abundant ECM protein in the human body. The main collagen content of the skin is collagen type I (Guazzo et al., 2018) and III (COLIII), whereas cartilage is mainly comprised of collagen type II (COLII). Therefore, COL-based scaffolds have been widely used for skin tissue regeneration (Law et al., 2017). The epithelial nature of components and fibroblasts are two critical factors in epithelial autografts cultured for skin reconstruction (Mohamed and Xing, 2012). Dermal tissue regeneration is required for severe cases of skin loss such as extent burn wounds. Self-assembling peptide-based hydrogel scaffolds have been used, showing promising potential for accelerating the proliferation of skin cells and wound healing (Chaudhari et al., 2016). Also, the combination of nano-fibrous membranes with fibrin nano-coating and ascorbic acid appears to be particularly helpful for dermal TE (Bacakova et al., 2016). Polyhydroxybutyrate (PHB) combined with organic soluble chitosan (CH), tropoelastin in connection with COLI, COL cross-linked CS, and heparin cross-linked COL with or without growth factors (e.g., FGF-2 or VEGF) are other attempted scaffolds that have shown to be advantageously involved in skin repair (Bi and Jin, 2013). These studies have successfully led to an actual product: MatriDerm® is a natural dermal graft made up of bovine collagen and an elastin hydrolysate. This skin autograft has provided a single-stage procedure for dermal repair in burn wounds (Chua et al., 2016). Other attempted therapies for skin TE are stem cell-based treatments, especially using adipose-derived stem cells (ASCs) (Klar et al., 2017).
Nerve
The treatment of facial paralysis has dramatically advanced in recent years using tissue-engineered constructs (Langhals et al., 2014). For instance, the high biocompatibility, natural biodegradation kinetics, and adjustable chemical properties of collagen make it a suitable scaffold for neural TE (Boni et al., 2018). Some other materials used as a scaffold for neural regeneration include innovative scaffolds (e.g., piezoelectric scaffolds) (Zaszczynska et al., 2020) and graphene nanocomposites (Karimi et al., 2016). Additionally, different SC types, including ESCs, NSCs, human iPSCs, MSCs, and ASCs, have shown promising results for application in neural TE (Karimi et al., 2016). Cell-based therapy using neural crest precursors of rat bone marrow is another likely successful repair method for peripheral nerve defects (Shi et al., 2019). The ability of dental pulp stem cells (DPSCs) to express neuronal markers, support neural growth, and differentiate into Schwann cells has made them promising candidates for cell-based therapies for peripheral nerve defects (Martens et al., 2014; Luo et al., 2018). It seems that the neural crest origin of DPSCs, similar to the origin of cells forming the peripheral nervous system, enables them to differentiate better into the neural and glial cells (Bhangra et al., 2016).
Blood vessel
Vascular regeneration is a crucial challenge required to be overcome in large tissue engineering applications because deprived perfusion drives cells into necrosis or ischemia. The problem is more severe in the central parts of the graft due to inadequate support of oxygen and supplements (Avolio et al., 2017). Studies report a greater volume of successful results on vascular TE on small scales. Tubular structures of vessels are generally regenerated through three strategies: sheet rolling, tubular molding, or direct scaffolding (Song et al., 2018). Scaffolds such as silk fibrous, COL, gelatin fibrous, and elastin have been used for small-diameter blood vessel fabrication (Awad et al., 2018). Moreover, a wide range of polymers from PGA-derived materials such as PGA/poly L-lactic acid (PLLA), COL/elastin, CS, PGS, poly glycolide knitted fiber, and L-lactide/ε-caprolactone copolymer have been used as a scaffold for vascular TE. Amniotic fluid has been mentioned to decrease the fabricating time of scaffold-based blood vessels using autologous cells (Nemeno-Guanzon et al., 2012). Decellularized blood vessels are other suggested matrixes for vascular engineering (Simsa et al., 2018). Stem cells used for vascular TE can be originated from either progenitor cells or adult stem cells from diverse sources, including bone marrow, adipose tissue, hair follicle, and umbilical cord (Wang et al., 2017). In addition, relevant studies have indicated the critical role of key growth factors (VEGF, Ang 1, BMP-4) in vascularization by different subtypes of endothelial cells in a concentration-dependent manner (Traore and George, 2017).
Synthetic scaffolds
Synthetic scaffolds may be comprised of polymers, bioceramics, or hybrids of natural/synthetic materials. The main advantages of polymeric scaffolds are biodegradability and manageable degradation time. Nevertheless, when the pH is lower than 5.6, the produced acidic abused materials stimulate a local inflammatory reaction and dissolve the surrounding apatite, which is the major drawback of these scaffolds. Among the polymeric scaffolds, PLGA, PLCL, and other polyester and their copolymer are widely used (Pangesty et al., 2017; Mirchandani et al., 2021; Shetty et al., 2021; Srimaneepong et al., 2021; Srimaneepong et al., 2022a; Mirza et al., 2022; Mubaraki et al., 2022; Patel et al., 2022; Ramzan et al., 2022; Syed et al., 2022). They can also be produced in different sizes, including nano-scales; however, insufficient ECM-mimicry and possible toxic byproducts after degradation are noticeable limitations of synthetic materials (Marti et al., 2013). Due to the machine’s ability to form sharp corners and clear boundaries, there is a limit on the amount of detail that can be created. In addition to this product’s limited mechanical, residual solvents, and Porogen material properties, there are several disadvantages. There is a more extended time lapse between the process’s beginning and end. It is important to note that they have many disadvantages, including the fact that they are highly susceptible to contamination by bacteria (like endotoxins), lack tunability, react immunologically, degrade at an uncontrollable rate, and do not have a mechanical expectations. In terms of biological aspects, these have several disadvantages in terms of their lack of cellular adhesion sites, so they require chemical modification to enhance the cells’ adhesion to them to improve their performance (Heboyan et al., 2018a; Heboyan et al., 2018b; Heboyan et al., 2020a; Heboyan et al., 2020b; Heboyan et al., 2021b; Reddy et al., 2021; Heboyan et al., 2022b; Heboyan et al., 2022c; Marya et al., 2022c; Heboyan et al., 2022d; Heboyan et al., 2022e; Heboyan et al., 2022f; Kakkad et al., 2022; Karobari et al., 2022). The following describes the most synthetic materials used in craniofacial TE. Moreover, the recent studies of the applied synthetic materials in craniofacial tissue engineering are summarized in Supplementary Table S1.
Graphene
The field of research seeking novel materials has gained much attention to graphene-based materials. Drug delivery, antimicrobial agents, biocompatibility, and other applications can be achieved with graphene-based nanomaterials. The graphene family is a group of carbon-based nanomaterials with a hexagonal molecular structure containing several derivatives such as Few-Layered Graphene (FLG), ultrathin graphite, graphene oxide (GO), reduced GO, and graphene nano-sheets. In biomedical fields, graphene has many potential uses, which is why it must have good surface adhesion to the surface (Guazzo et al., 2018; Rokaya et al., 2019; Rokaya et al., 2021). The graphene family’s unique mechanical, electrochemical, and physical properties, such as excellent biocompatibility, bioactivity, dispersity, and hydrophilicity, have extended their application in TE (Zhang et al., 2017a). In addition, its capacity to adsorb GFs, drugs, and other biomolecules, because of its superficial functional groups, is much beneficial to TE therapy (Nishida et al., 2016), especially for bone and periodontal tissue regeneration (Kawamoto et al., 2018). Therefore, GO has been combined with other materials, such as poly(vinyl) alcohol (PVA)/CS and methacrylated chondroitin sulfate, to improve the cell growth rate and articular cartilage differentiation (Liao et al., 2015; Zhou et al., 2018). Covalent or non-covalent functionalization is possible for graphene. Nitrogen, carbene, and aryl intermediates can form covalent bonds with graphene. Noncovalent interactions modify graphene, on the other hand. Tissue engineering for bone regeneration and substitution is becoming increasingly popular. As well as bone regeneration, functionalized graphene and its derivatives are also used in tissue engineering and tissue regeneration scaffolds. Functionalized graphene has also been shown to have a high mechanical and therapeutic potential. Supporting specific cells and serving as templates to guide the growth and construction of new tissues are essential features of scaffolds in tissue engineering (Srimaneepong et al., 2022b).
Calcium phosphate cement
CPC is an outstanding biocompatible, biologically active, mechanically strong, and physicochemically stable synthetic material widely used as a scaffold today (Qin et al., 2016). It has excellent osteoconductive properties and can be ideally resorbed in vivo and replaced by regenerated bone (Liu et al., 2013; Osorio et al., 2016). CPC scaffolds are also widely used as composites accompanied by SC types such as MSCs, BMSCs, and human umbilical cord mesenchymal stem cells (hUCMSCs) for regenerating different bone tissues, including cortical osseous autografts (Sun and Yang, 2015; Qiu et al., 2016). BMPs achieve better osteogenic differentiation, non-rigid and strong construct, and optimized mineral synthesis (Thein-Han et al., 2013; Sun and Yang, 2015).
Polyglycolic acid, polyester poly hydroxyl alkanoate, and poly hydroxybutyrate
PGA is a popular material for scaffolding applications alone or in combination with other materials because of its fast degradation (4–12 months), tensile strength, and proper elasticity (Neto and Ferreira, 2018). PGA has been used in combination with CH for bone TE in extraction sockets (Chang et al., 2014), with collagen for better cellular adhesion (Kinoshita and Maeda, 2013), and PLLA/PCL co-polyesters for cellular-based TE repairing cranial bone defect (Kwon et al., 2015). PHA is an amorphous polymer with biocompatibility, bio‐degradability, and mechanical properties that convey it as a proper candidate for TE applications (Fu et al., 2019). PHB copolymerized with hydroxy valerate (PHBV) has been used for cartilage TE studies and has shown promising results (Xue et al., 2019a).
Polylactic acid
PLA is an aliphatic thermoplastic polyester with linear polymeric chains, which is susceptible to enzymatic degradation. Its mechanical and thermal properties, biocompatibility, and source sustainability make PLA an affordable and available material for biomedical applications (Sheikh et al., 2015). In an in vivo study, the combination of PLA with collagen has been used as a scaffold for seeding with bone marrow-derived MSCs (BMMSCs) and implanted into an animal model. The adequate subchondral bone appearance, cartilage development, and functional repair of deep osteochondral interfacial tissue structure suggested PLA/COL as a potential graft (Kazemnejad et al., 2017). PLLA -A chiral isoform of PLA- has been developed as a dental scaffold model and has become a popular model for dental mechanistic studies and pulp regeneration (Huang, 2009; Demarco et al., 2011; Soares et al., 2018). Also, the injectable nanofibrous form of PLLA microspheres carrying BMP-2 has been shown to improve the regeneration of dentin tissues (Wang et al., 2016a). Wang et al. successfully repaired the mandibular bone defects using nano-HAp/COL/PLA scaffold seeded with alveolar bone marrow SCs (aBMSCs) (Wang et al., 2016b). The potential use of tetracycline-containing fibers for dental implants is beyond their antibacterial properties. However, PLA’s poor toughness and lower elongation at break limit its use for applications requiring plastic deformation at high-stress levels. Researchers have researched to toughen PLLA to overcome these constraints (Rokaya et al., 2018b). PLA has also been seeded with multiple cell types (e.g., Schwann cells and DPSCs) for successfully repairing the nerve defects in an animal model (De Carvalho et al., 2019). PLA is also one of the biomaterials introduced as a potential for TMJ disc substitution, providing a suitable cell adhesion and rearrangement (Ahtiainen et al., 2013).
Zein
Zein is the main constituent endosperm protein with unique properties such as thermos- resistance, solubility, biocompatibility, porosity, film-forming, and antimicrobial and anti-oxidative effects for scaffold fabrication. Zein scaffold has been used for PDLCs growth for repairing periodontal tissue defects, either alone or with GFs such as Shuanghuangbu (a Chinese medicine). Shuanghuangbu can enhance the proliferation activity of PDLCs (Yan-Zhi et al., 2010).
Poly (caprolactone)
Slow biodegradability, biocompatibility, and safety approval from Food and Drug Administration (FDA) make PCL an appropriate scaffold for clinical application in medicine and dentistry as well as tissue regeneration (Figure 3) (Jamal et al., 2018; Asa’ad et al., 2016; Wongsupa et al., 2017). Other materials are combined with PCL to improve mechanical, electrical, and biological properties (Flores-Cedillo et al., 2016). Among these additional materials are carbon nanotube (CNT) (Flores-Cedillo et al., 2016), Ibuprofen (Batool et al., 2018), and albumin nanoparticles (Kamath et al., 2014). Albumin-combined PCL has provided promising osteoconductivity and osteoinductivity with a prolonged-release profile ideal for promoting osteogenesis (Kamath et al., 2014). The dose- and spatially-controlled release of antimicrobials and GFs has been investigated by the Ibuprofen-PCL membrane scaffold (Batool et al., 2018). The electrospun membrane forms of PCL have been used to locate the sheet on the dental surface that enhanced adherence between fibrous connective tissue, the regenerated PDL, and bone (Park et al., 2016).
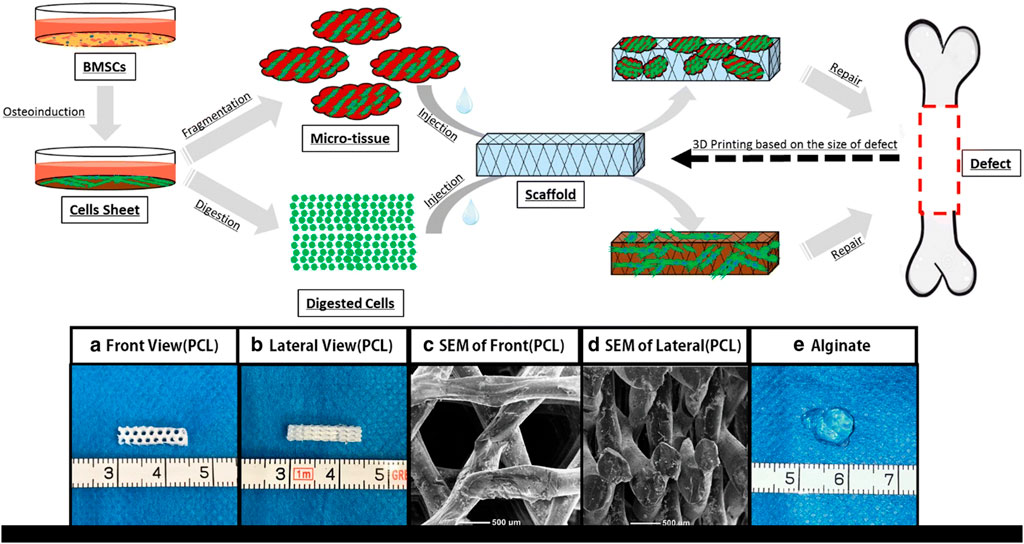
FIGURE 3. Schematic view of the micro-tissue construction procedures comparison to the digested cells. (A) Front view of Polycaprolactone (PCL) scaffold; (B) Side view of PCL scaffold; (C) A front view of PCL scaffold by electron microscope; (D) A side view of PCL scaffold by electron microscope; (E) Calcium alginate gel (General view) (Wu et al., 2018).
Poly methylmethacrylate
PMMA has been combined with gelatin and GMPs to carry antibiotics for trauma treatment applications, successfully acting as both porogen and drug carrier. The gelatin and drug solution determine the gelatin swelling percentage and porosity. This construct and its drug release kinetics can be modified and optimized for clinical applications (Shi et al., 2011).
Polylactide-Co-glycolide
The beneficial mechanical properties, adjustable degradation rates, and high biocompatibility of PLGA (a synthetic aliphatic polyester) make it a suitable scaffold for periodontal therapy (Sood et al., 2012; Campos et al., 2014; Sun et al., 2017). For example, adding HAp can improve the mechanical properties and osteoconductivity of PLGA to meet better bone TE requirements (Figure 4) (Gentile et al., 2014). In another modification try, Cai et al. observed that the ultrasonic treatment of wet electrospun PLGA/PCL scaffolds makes them desirable scaffolds for dental TE (Cai et al., 2017). Also, the combination of Ag nanoparticles with PLGA/CH construct has been employed for periodontal TE. The optimized ratio of PLGA/CS and Ag nanoparticles can achieve high cell mineralization without cytotoxicity (Xue et al., 2019b). PLGA microparticles have also been used for consecutive drug-release at different delivery rates (Santo et al., 2013).
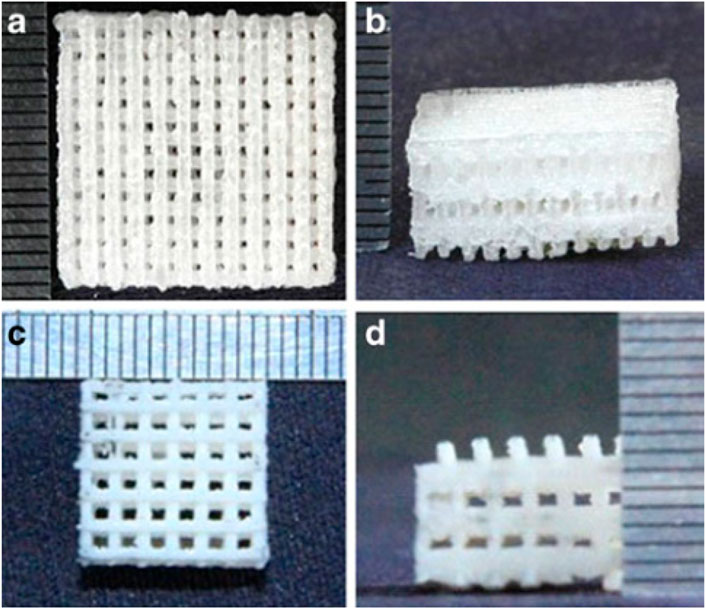
FIGURE 4. The top and front views of porous poly(lactic-co-glycolic acid) (PLGA) scaffold alone (A,B) and in combination with nano- Hydroxyapatite (HA) (C,D) (Gentile et al., 2014).
Poly D, L-lactide, and glycolide
PLG scaffold has been used in an in vivo study to regenerate the dental pulp tissue using a heterogeneous population of DPSCs and stem cells from the apical papilla (SCAP). The construct transplanted subcutaneously in the animal model could generate a vascularized pulp-like tissue and dentin-like layer on the canal dentin wall. Additionally, a mineral trioxide aggregate (MTA) cement was observed after a couple of months (Huang, 2011).
Hydroxyapatite
HAp is one of the vital components of the acellular section of the bone, and then, its synthetic form has been vastly used in bone engineering research (Kumar et al., 2016; Calis et al., 2017). HAp is synthesized in either form of fibrous (by electrospinning) or porous (blended with other biomaterials) (Stratton et al., 2016). The combination of HAp with various polymers, including PLA, PLGA, gelatin, CH, and COL, has been successfully employed for bone formation and arrangement both in vitro and in vivo (Amini et al., 2012). Since nHAp substantially affects cell proliferation, differentiation, and ECM production considered a bioactive building block of bioengineered dental scaffolds (Lee et al., 2012; van Manen et al., 2014). Also, nHAp and nHAp-coated silk scaffolds have been used for periodontal regeneration (Yang et al., 2013). An avidin-biotin-biotin binding system enhanced the periodontal ligament (PDL) fibroblasts’ adherence to three-dimensionally constructed nHAp scaffolds (Jang et al., 2011). HA surfaces coated with PMP50 were less adhesive and resistant to protein adsorption than surfaces coated with other polymers. PMP films are highly stable and antiadsorptive, making them ideal for dentistry and medicine. Due to its complicated synthesis, MPC is limited in its application because of its high production costs (Rokaya et al., 2018b).
Poly methoxyethyl acrylate, octacalcium phosphate, and carbon nanotube-based biomaterials
PMEA has been used to reproduce PDL cells showing low adherence to platelets, making it a promising scaffold for TE in blood-rich environments and culturing tissue-derived cells (Kitakami et al., 2014). OCP/COL implantation enhanced bone healing at tooth extraction socket, alveolar cleft model, and mandibular bone defect in a canine model (Kawai et al., 2014). One of the main productive sub-areas of nanomedicine and nanobiotechnology is the biomedical usage of CNT nanomaterials. Outstanding biocompatibility and biophysicochemical properties of CNT-based or -reinforced scaffolds are appropriate for osteoblast distinction and lead bone renewal during bone TE procedures. In addition, the CNTs’ application has been extended as nanoparticles for drug conveyance and cellular transport in tissue engineering, owing to their expansive surface area and great incitement (Pei et al., 2019).
Gelatin methacrylate
GelMA is the result of combining methacrylate bunches with amine-containing side bunches of gelatins (Rider et al., 2018a). GelMA is frequently used as a scaffold for tissue engineering. Up to now, GelMA’s application has been reported in cartilage TE (Bao et al., 2020), chondrogenic differentiation of BMSCs (Lin et al., 2014), and DSCs and HUVEC differentiation into mineralized dental tissues (Smith et al., 2018a; Smith and Yelick, 2019). Moreover, Smith et al. could produce a mineralized and vascularized tooth bud model by culturing post-natal (embryonic) dental progenitor cell-derived on GelMA hydrogels (Khayat et al., 2017). Similarly, Athirasala et al. could produce pre-vascularized dental pulp constructs on GelMA scaffold using odontoblast-like cells and endothelial colony shaping cells (Athirasala et al., 2017). GelMA-encapsulated DPSC/HUVEC constructs are a promising therapy for pulpal revascularization instead of using devitalized tooth endodontic therapy (Khayat et al., 2017). Cell-encapsulated 3D GelMA hydrogels have also been used with cell sheets (dental epithelial and dental mesenchymal cells). Using this construct could successfully form dental ECM, upregulate the expression of dental cell differentiation markers, and produce biomimetic enamel and pulp organ layers (Monteiro et al., 2016). The GelMA/nHAp microgels have also contributed to periodontal TE in vitro and in vivo. This construct has promoted new bone formation and facilitated osteogenic differentiation, reproduction, and human PDLSCs viability (Chen et al., 2016a).
Ceramic scaffolds
Calcium phosphate and hydroxyapatite (HA) are the most widely known and used examples of bioceramic materials recognized as safe substitutes for bone grafts (Nadeem et al., 2013; Murakami et al., 2017). The more flexible bioceramics with progressed mechanical properties are accomplished by adding polymers to bioceramics (Abdulghani and Mitchell, 2019). The osteoconductivity and osteoinductivity of CPCs qualify them for bone tissue repair. HAp, TCP, and their combination as biphasic calcium phosphates (BCPs) and amorphous calcium phosphates (ACPs) are common types of CPCs used in bone TE (Ghassemi et al., 2018).
Bioactive glass-ceramics
he excellent bioactivity and biosafety of BGC have caused its wide application as scaffolds for bone TE, coatings on metallic implants, and dental filling materials (Mourino et al., 2012; Wang et al., 2013; Zhang et al., 2018a; Kumar et al., 2018; Chocholata et al., 2019). This material is composed of CaO, Na2O, SiO2, and P2O5 and can be categorized into resorbable or non-resorbable groups, depending on the relative proportion of its compounds (Siaili et al., 2018). BGC has priority over other ceramic scaffolds, such as HAp and related crystalline calcium phosphates, due to silicon’s ability in osteogenesis management and adjusting mechanical strength and degradation rate (Chen et al., 2012a). For example, bioglass 45S5 has been used for bone formation. The native tissues and bones strongly bond to the carbonate-substituted hydroxyapatite layer on Bioglass’s surface. Over time, bioglass degrades, and new bone is formed (Sriranganathan et al., 2016). Kumar et al. reported that combining bioglass scaffolds and ibuprofen-loaded CTS/nHAp is an improved alternative for TE applications compared to other natural polymer-based scaffolds (Kumar et al., 2019). Moreover, the hybrid of bioactive glass nanoparticles and PCL showed promising results for osteoblast attachment in contrast to the PCL scaffold alone (Lei et al., 2019). Among the coated-bioglass materials with bone regeneration ability, gelatin-coated bioglass scaffolds exhibited the highest cell formation (Zafar et al., 2019). El-Gendy et al. also reported the efficiency of the 45S5 bioglass scaffold for vascularized bone TE (El-Gendy et al., 2015). Additionally, a modified form of 45S5 bioglass-based scaffolds (PHBV-coated) has ideal bone TE characteristics showing increased porosity, proper permeability, and higher pore interconnections (Kashte et al., 2017). As a result of their compositional similarity to bone and tooth structure, bioactive properties, and apparent antimicrobial properties, BAGs have been studied in dentistry as bone substitutes for dentoalveolar and maxillofacial reconstruction, periodontal regeneration, and implants. At present, bone graft surgery uses autologous, allogeneic, xenograft, and synthetic bone grafts. Bone regeneration with autologous bone grafts is considered the gold standard due to its osteogenic properties and absence of immune reactions. There is a limit to how much graft can be harvested because of second bone defects and donor site morbidities. Xenografts, autologous grafts, or allografts cannot achieve the qualities that Janicki and Schmidmaier outlined as ideal graft materials. With the growing demand for BG, the market has begun shifting towards synthetic BGs. Synthetic bone grafts have an advantage over natural bone grafts since they are tailored to meet the needs of bone grafting (Skallevold et al., 2019).
β-tricalcium phosphate
A key biomaterial for orthopedic and dental applications is β-TCP because of its outstanding biocompatibility and biodegradability (Chanchareonsook et al., 2014; Ke et al., 2015). β-TCP scaffolds are widely studied for personalized craniofacial defect treatment using computer-aided design/computer-aided manufacturing (CAD/CAM) techniques (Wang et al., 2019a). In addition, Henrich et al. have shown a promising application for β-TCP and demineralized bone matrix in treating the bone defect mediated by bone marrow concentrate (BMC) (Henrich et al., 2015). In another study by Su et al., the essential periodontal ligament stem cells (PDLSC) were seeded on β-TCP scaffolds and were able to conduct osteogenesis and adipogenesis (Su et al., 2015). β-TCP has also been used in combination with HAp and COL for producing a viable bone substitute shown to be efficient for preserving the tooth socket after extraction (Ho et al., 2016).
Pullulan/dextran polysaccharides and calcium silicate cement
A promising substitute with comparable osteogenic properties to HA/TCP ceramics is pullulan/dextran-based hydrogel. These polymers provide fast resorption capacity, a favorable trait for complementary biomaterials used in bone repair applications (Frasca et al., 2017). CaSi-based materials have extensive application in bone TE owing to their osteoconductivity and ability to decrease inflammation markers, especially in primary human DPCs (Chen et al., 2016b; Nagy et al., 2018). These types of cement are widely used for direct pulp capping, root canal sealing, root-end filling, and other endodontic purposes (Tatullo et al., 2019).
Hydrogel scaffolds
Hydrogels have a wide range of applications, from regenerative endodontics to new tissue formation (Galler et al., 2012). For example, ECM-derived hydrogels are used for bone regeneration and have shown promising results (Pacifici et al., 2018). Single-component self-assembling peptide hydrogels are hydrogels suitable for regenerative periodontal therapy (Koch et al., 2018). Dissanayaka et al. worked on pulp regeneration in an animal-modeled study by utilizing prevascularized PuramatrixTM (a commercially available peptide hydrogel scaffold) and DPSCs (Dissanayaka et al., 2015). Generally, outstanding features of hydrogel-based biomaterials, such as their easy handling, make them desirable for bone regeneration applications, especially in cases of extended defects (Pacifici et al., 2018). In addition, the hydrogel’s capability to transmit biological/chemical factors and entrap progenitor cells such as MSCs or endothelial progenitor cells are highly advantageous for bone TE. Then, some studies aim to enhance their properties by modifying hydrogel structure and stiffness. Hydrogels’ convenient formation and shaping capacity has made them proper scaffolds for short-bone regeneration, such as maxillo-facial bones too (Frasca et al., 2017). Modified hydrogels can change the cells’ affinity for the scaffold or modify their differentiation response (Tan and Hung, 2017). Chitin is a hydrogel scaffold that is extensively employed for upgrading the performance of tissue-engineered constructs (Jayakumar et al., 2011). Another example of modified hydrogels is amelogenin-chitosan, designed and used for the prevention, restoration, and treatment of defective dental enamel (Ruan et al., 2016).
Nanoparticles
TThe nanoparticle-modified scaffolds have been shown to improve cell growth and tissue engineering, including oral TE, because of their unique physicochemical and biomimetic features (Figures 5, 6) (Funda et al., 2020). The TCP nanoparticle/collagen scaffold is suggested as practical for periodontal TE (Ogawa et al., 2016). CaP nanomaterial scaffolds can mimic the structure of natural bone with increased protein adsorption, higher surface-to-volume ratio, improved wettability, and strengthened mechanical properties compared to older counterparts (Wang et al., 2014). Nano-HAp and granular PRF, in combination with MSC sheets, have been used for the treatment of extensive bone losses. Nanofibrous spongy microspheres seeded with human DPSCs have shown a new perspective for treating dental pulp diseases (Kuang et al., 2016). Carbon nanostructures are other composites shown to be appropriately osteogenic, osteoconductive, and osteoinductive (Perkins and Naderi, 2016). There is an increasing need for MNPs in bone tissue engineering. MNPs, made from metals such as iron and cobalt, can be magnetically manipulated by an external magnetic field. They can enhance their performance using magnetic cores, biocompatible caps, and optional coatings. Various shapes, sizes, and coatings are possible with MNPs, making them highly versatile. Due to their physicochemical properties, they are versatile enough to be applied in various biomedical applications. Since MNPs are intrinsically biocompatible, they have been used in various diagnostic and therapeutic applications. Various methods have been demonstrated in the literature for improving cells, bioactive agents, and scaffolds. In recent years, a way to manipulate cell behavior more accurately and controllably remotely has been explored using magnetic nanoactuation. Incorporating MNPs in scaffold matrices can be accomplished through electrospinning, covalent linking, and freeze-drying. The scaffold-containing MNPs focus on their role in promoting osteogenesis and then on their role in promoting angiogenesis (Dasari et al., 2022).
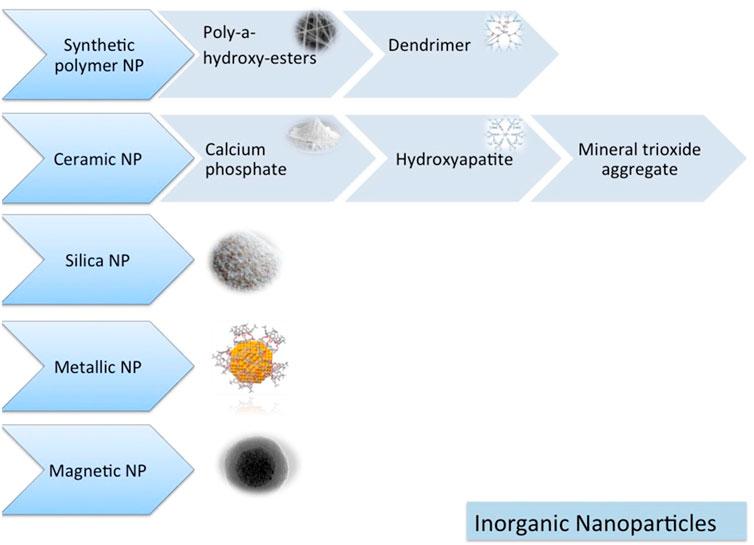
FIGURE 5. Inorganic nanomaterials (Funda et al., 2020).
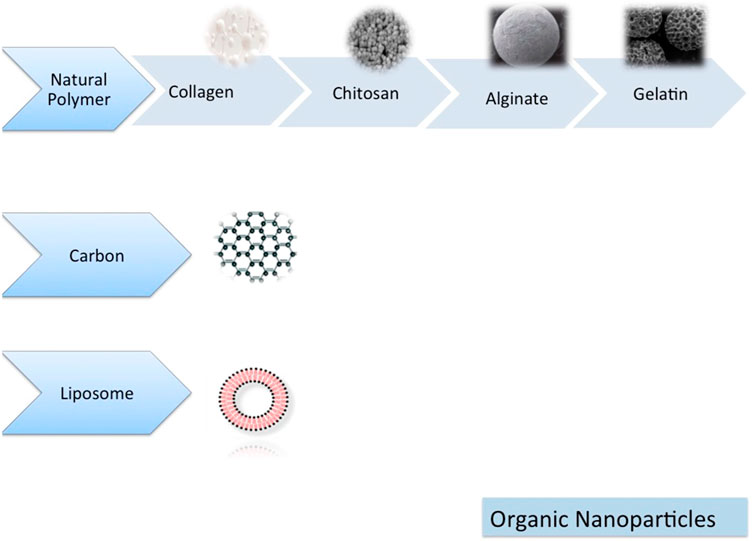
FIGURE 6. Organic nanomaterials (Funda et al., 2020).
Conclusion and future direction
Autografts and allografts are used for craniofacial bone tissue engineering, but reconstruction with these standard approaches may lead to donor site morbidity and flaws to host tooth aesthetic characteristics. The treatments with synthetic materials and scaffolds face challenges, such as high expenses, complicated fabrication, unmanageable degradation, inflammation risk, and immunity rejection. The synthetic materials used in regenerative medicine should provide specific properties regarding biological, mechanical, and osteoinductive qualities. Today, the necessity of developing functional materials and scaffolds for skeletal tissue regeneration is evident regarding the severe deficiency of bone grafts. In this regard, notable scientific progress has been achieved in the past decades, and an assortment of scaffolding materials has been developed for craniofacial TE applications. These materials can provide porosity, biodegradability, cell adhesion, and tissue regeneration. However, they still need to meet some requirements to be clinically approved, which is challenged by the considerable gap between the laboratory and clinic. Moreover, such engineered products’ complexity must be converted to simplicity and feasibility in function and handling. On the other hand, for the best mimicking of the targeted tissue properties, endogenous regenerative capabilities of the host tissues need to be more accounted for. However, considering the complexities of endogenous tissues- especially craniofacial tissues, this is a challenging aim to be achieved. In conclusion, more in vitro and in vivo studies are suggested to help extend our understanding of the engineered tissue performance in humans and cells’ responding to different parameters.
Author contributions
MY, AF, KA, MA, RR, MR, ET, AHA, and HT were involved in the study design, data collection, and the original draft. MY, HT, ET, and RR were involved in helping in writing the review article and critically reviewing the data. All authors have reviewed and accepted the final draft and are in charge of the content and similarity index of the manuscript.
Acknowledgments
The authors would like to acknowledge colleagues who gave valuable comments.
Conflict of interest
The authors declare that the research was conducted in the absence of any commercial or financial relationships that could be construed as a potential conflict of interest.
Publisher’s note
All claims expressed in this article are solely those of the authors and do not necessarily represent those of their affiliated organizations, or those of the publisher, the editors and the reviewers. Any product that may be evaluated in this article, or claim that may be made by its manufacturer, is not guaranteed or endorsed by the publisher.
Supplementary material
The Supplementary Material for this article can be found online at: https://www.frontiersin.org/articles/10.3389/fbioe.2022.987195/full#supplementary-material
References
Abdulghani, S., and Mitchell, G. R. (2019). Biomaterials for in situ tissue regeneration: A review. Biomolecules 9 (11), 750. doi:10.3390/biom9110750
Abhay, S. S., Ganapathy, D., Veeraiyan, D. N., Ariga, P., Heboyan, A., Amornvit, P., et al. (2021). Wear resistance, color stability and displacement resistance of milled PEEK crowns compared to zirconia crowns under stimulated chewing and high-performance aging. Polym. (Basel) 13 (21), 3761. doi:10.3390/polym13213761
Ahmed, N., Khan, M., Saleem, W., Karobari, M. I., Mohamed, R. N., Heboyan, A., et al. (2022). Evaluation of Bi-lateral Co-infections and antibiotic resistance rates among COVID-19 patients. Antibiotics 11 (2), 276. doi:10.3390/antibiotics11020276
Ahtiainen, K., Mauno, J., Ellä, V., Hagström, J., Lindqvist, C., Miettinen, S., et al. (2013). Autologous adipose stem cells and polylactide discs in the replacement of the rabbit temporomandibular joint disc. J. R. Soc. Interface 10 (85), 20130287. doi:10.1098/rsif.2013.0287
Akar, B., Tatara, A. M., Sutradhar, A., Hsiao, H-Y., Miller, M., Cheng, M-H., et al. (2018). Large animal models of an in vivo bioreactor for engineering vascularized bone. Tissue Eng. Part B Rev. 24 (4), 317–325. doi:10.1089/ten.teb.2018.0005
Akino, N., Tachikawa, N., Miyahara, T., Ikumi, R., and Kasugai, S. (2019). Vertical ridge augmentation using a porous composite of uncalcined hydroxyapatite and poly-DL-lactide enriched with types 1 and 3 collagen. Int. J. Implant Dent. 5 (1), 16–17. doi:10.1186/s40729-019-0167-5
Aldemir Dikici, B., Dikici, S., Reilly, G. C., MacNeil, S., and Claeyssens, F. (2019). A novel bilayer polycaprolactone membrane for guided bone regeneration: Combining electrospinning and emulsion templating. Materials 12 (16), 2643. doi:10.3390/ma12162643
Alizadeh, A., Razmjou, A., Ghaedi, M., Jannesar, R., Tabatabaei, F., Pezeshkpour, V., et al. (2019). <p>Culture of dental pulp stem cells on nanoporous alumina substrates modified by carbon nanotubes</p>. Int. J. Nanomedicine 14, 1907–1918. doi:10.2147/ijn.s189730
Amini, A. R., Laurencin, C. T., and Nukavarapu, S. P. (2012). Bone tissue engineering: Recent advances and challenges. Crit. Rev. Biomed. Eng. 40 (5), 363–408. doi:10.1615/critrevbiomedeng.v40.i5.10
Ammar, M. M., Waly, G. H., Saniour, S. H., and Moussa, T. A. (2018). Growth factor release and enhanced encapsulated periodontal stem cells viability by freeze-dried platelet concentrate loaded thermo-sensitive hydrogel for periodontal regeneration. Saudi Dent. J. 30 (4), 355–364. doi:10.1016/j.sdentj.2018.06.002
Anbu, R. T., Suresh, V., Gounder, R., and Kannan, A. (2019). Comparison of the efficacy of three different bone regeneration materials: An animal study. Eur. J. Dent. 13 (01), 022–028. doi:10.1055/s-0039-1688735
Ângelo, D. F., Monje, F. G., González-García, R., Little, C. B., Mónico, L., Pinho, M., et al. (2017). Bioengineered temporomandibular joint disk implants: Study protocol for a two-phase exploratory randomized preclinical pilot trial in 18 black merino sheep (TEMPOJIMS). JMIR Res. Protoc. 6 (3), e37. doi:10.2196/resprot.6779
Ansari, M. (2019). Bone tissue regeneration: Biology, strategies and interface studies. Prog. Biomater. 8, 223–237. doi:10.1007/s40204-019-00125-z
Aquino-Martínez, R., Angelo, A. P., and Pujol, F. V. (2017). Calcium-containing scaffolds induce bone regeneration by regulating mesenchymal stem cell differentiation and migration. Stem Cell Res. Ther. 8 (1), 265. doi:10.1186/s13287-017-0713-0
Asa’ad, F., Pagni, G., Pilipchuk, S. P., Giannì, A. B., Giannobile, W. V., and Rasperini, G. (2016). 3D-printed scaffolds and biomaterials: Review of alveolar bone augmentation and periodontal regeneration applications. Int. J. Dent. 2016, 1–15. doi:10.1155/2016/1239842
Athirasala, A., Lins, F., Tahayeri, A., Hinds, M., Smith, A. J., Sedgley, C., et al. (2017). A novel strategy to engineer pre-vascularized full-length dental pulp-like tissue constructs. Sci. Rep. 7 (1), 3323–3411. doi:10.1038/s41598-017-02532-3
Athirasala, A., Tahayeri, A., Thrivikraman, G., França, C. M., Monteiro, N., Tran, V., et al. (2018). A dentin-derived hydrogel bioink for 3D bioprinting of cell laden scaffolds for regenerative dentistry. Biofabrication 10 (2), 024101. doi:10.1088/1758-5090/aa9b4e
Aval, F. S., Arab, M. R., Sargolzaei, N., Noushadi, F., Eteghadi, A., Keykhaei, A., et al. (2018). Efficacy of octacalcium phosphate and octacalcium phosphate/gelatin composite on the repair of critical-sized calvarial defects in rats. J. Dent. 15 (2), 86–96.
Avetisyan, A., Markaryan, M., Rokaya, D., Tovani-Palone, M. R., Zafar, M. S., Khurshid, Z., et al. (2021). Characteristics of periodontal tissues in prosthetic treatment with fixed dental prostheses. Mol. (Basel, Switz. 26 (5), 1331. doi:10.3390/molecules26051331
Avolio, E., Alvino, V. V., Ghorbel, M. T., and Campagnolo, P. (2017). Perivascular cells and tissue engineering: Current applications and untapped potential. Pharmacol. Ther. 171, 83–92. doi:10.1016/j.pharmthera.2016.11.002
Awad, N. K., Niu, H., Ali, U., Morsi, Y. S., and Lin, T. (2018). Electrospun fibrous scaffolds for small-diameter blood vessels: A review. Membranes 8 (1), 15. doi:10.3390/membranes8010015
Bacakova, M., Musilkova, J., Riedel, T., Stranska, D., Brynda, E., Zaloudkova, M., et al. (2016). The potential applications of fibrin-coated electrospun polylactide nanofibers in skin tissue engineering. Int. J. Nanomedicine 11, 771–789. doi:10.2147/ijn.s99317
Bang, S., Jung, U-W., and Noh, I. (2018). Synthesis and biocompatibility characterizations of in situ chondroitin sulfate–gelatin hydrogel for tissue engineering. Tissue Eng. Regen. Med. 15 (1), 25–35. doi:10.1007/s13770-017-0089-3
Bao, W., Li, M., Yang, Y., Wan, Y., Wang, X., Bi, N., et al. (2020). Advancements and Frontiers in the high performance of natural hydrogels for cartilage tissue engineering. Front. Chem. 8, 53. doi:10.3389/fchem.2020.00053
Baskaran, P., Prakash, P. S. G., Appukuttan, D., Mugri, M. H., Sayed, M., Subramanian, S., et al. (2022). Clinical and radiological outcomes for guided implant placement in sites preserved with bioactive glass bone graft after tooth extraction: A controlled clinical trial. Biomimetics 7 (2), 43. doi:10.3390/biomimetics7020043
Basnett, P., Lukasiewicz, B., Marcello, E., Gura, H. K., Knowles, J. C., and Roy, I. (2017). Production of a novel medium chain length poly (3‐hydroxyalkanoate) using unprocessed biodiesel waste and its evaluation as a tissue engineering scaffold. Microb. Biotechnol. 10 (6), 1384–1399. doi:10.1111/1751-7915.12782
Batool, F., Morand, D-N., Thomas, L., Bugueno, I. M., Aragon, J., Irusta, S., et al. (2018). Synthesis of a novel electrospun polycaprolactone scaffold functionalized with ibuprofen for periodontal regeneration: An in vitro andin vivo study. Materials 11 (4), 580. doi:10.3390/ma11040580
Beltrán-Partida, E., Valdez-Salas, B., Escamilla, A., Curiel, M., Valdez-Salas, E., Nedev, N., et al. (2016). Disinfection of titanium dioxide nanotubes using super-oxidized water decrease bacterial viability without disrupting osteoblast behavior. Mater. Sci. Eng. C 60, 239–245. doi:10.1016/j.msec.2015.11.042
Bernhard, J. C., and Vunjak-Novakovic, G. (2016). Should we use cells, biomaterials, or tissue engineering for cartilage regeneration? Stem Cell Res. Ther. 7 (1), 56. doi:10.1186/s13287-016-0314-3
Bhangra, K. S., Busuttil, F., Phillips, J. B., and Rahim, A. A. (2016). Using stem cells to grow artificial tissue for peripheral nerve repair. Stem cells Int. 2016, 1–18. doi:10.1155/2016/7502178
Bhumiratana, S., Bernhard, J. C., Alfi, D. M., Yeager, K., Eton, R. E., Bova, J., et al. (2016). Tissue-engineered autologous grafts for facial bone reconstruction. Sci. Transl. Med. 8 (343), 343ra83–ra83. doi:10.1126/scitranslmed.aad5904
Bi, H., and Jin, Y. (2013). Current progress of skin tissue engineering: Seed cells, bioscaffolds, and construction strategies. Burns Trauma 1 (2), 63–72. doi:10.4103/2321-3868.118928
Boni, R., Ali, A., Shavandi, A., and Clarkson, A. N. (2018). Current and novel polymeric biomaterials for neural tissue engineering. J. Biomed. Sci. 25 (1), 90. doi:10.1186/s12929-018-0491-8
Bouwman, W., Bravenboer, N., Frenken, J., Ten Bruggenkate, C., and Schulten, E. (2017). The use of a biphasic calcium phosphate in a maxillary sinus floor elevation procedure: A clinical, radiological, histological, and histomorphometric evaluation with 9-and 12-month healing times. Int. J. Implant Dent. 3 (1), 34. doi:10.1186/s40729-017-0099-x
Brookes, S., Voytik‐Harbin, S., Zhang, H., and Halum, S. (2018). Three‐dimensional tissue‐engineered skeletal muscle for laryngeal reconstruction. Laryngoscope 128 (3), 603–609. doi:10.1002/lary.26771
Brown, B. N., Chung, W. L., Almarza, A. J., Pavlick, M. D., Reppas, S. N., Ochs, M. W., et al. (2012). Inductive, scaffold-based, regenerative medicine approach to reconstruction of the temporomandibular joint disk. J. Oral Maxillofac. Surg. 70 (11), 2656–2668. doi:10.1016/j.joms.2011.12.030
Cai, X., ten Hoopen, S., Zhang, W., Yi, C., Yang, W., Yang, F., et al. (2017). Influence of highly porous electrospun PLGA/PCL/nHA fibrous scaffolds on the differentiation of tooth bud cells in vitro. J. Biomed. Mat. Res. A 105 (9), 2597–2607. doi:10.1002/jbm.a.36120
Calis, M., Demirtas, T. T., Vatansever, A., Irmak, G., Sakarya, A. H., Atilla, P., et al. (2017). A biomimetic alternative to synthetic hydroxyapatite:“boron-containing bone-like hydroxyapatite” precipitated from simulated body fluid. Ann. plastic Surg. 79 (3), 304–311. doi:10.1097/sap.0000000000001072
Campos, D. M., Gritsch, K., Salles, V., Attik, G. N., and Grosgogeat, B. (2014). Surface entrapment of fibronectin on electrospun PLGA scaffolds for periodontal tissue engineering. BioResearch open access 3 (3), 117–126. doi:10.1089/biores.2014.0015
Cattalini, J. P., Boccaccini, A. R., Lucangioli, S., and Mouriño, V. (2012). Bisphosphonate-based strategies for bone tissue engineering and orthopedic implants. Tissue Eng. Part B Rev. 18 (5), 323–340. doi:10.1089/ten.teb.2011.0737
Chanchareonsook, N., Junker, R., Jongpaiboonkit, L., and Jansen, J. A. (2014). Tissue-engineered mandibular bone reconstruction for continuity defects: A systematic approach to the literature. Tissue Eng. Part B Rev. 20 (2), 147–162. doi:10.1089/ten.teb.2013.0131
Chandrahasa, S., Murray, P. E., and Namerow, K. N. (2011). Proliferation of mature ex vivo human dental pulp using tissue engineering scaffolds. J. Endod. 37 (9), 1236–1239. doi:10.1016/j.joen.2011.05.030
Chang, H-H., Wang, Y-L., Chiang, Y-C., Chen, Y-L., Chuang, Y-H., Tsai, S-J., et al. (2014). A novel chitosan-γPGA polyelectrolyte complex hydrogel promotes early new bone formation in the alveolar socket following tooth extraction. PloS one 9 (3), e92362. doi:10.1371/journal.pone.0092362
Chaudhari, A. A., Vig, K., Baganizi, D. R., Sahu, R., Dixit, S., Dennis, V., et al. (2016). Future prospects for scaffolding methods and biomaterials in skin tissue engineering: A review. Int. J. Mol. Sci. 17 (12), 1974. doi:10.3390/ijms17121974
Chen, Q., Zhu, C., and Thouas, G. A. (2012). Progress and challenges in biomaterials used for bone tissue engineering: Bioactive glasses and elastomeric composites. Prog. Biomater. 1 (1), 2. doi:10.1186/2194-0517-1-2
Chen, W., Liu, X., Chen, Q., Bao, C., Zhao, L., Zhu, Z., et al. (2018). Angiogenic and osteogenic regeneration in rats via calcium phosphate scaffold and endothelial cell co-culture with human bone marrow mesenchymal stem cells (MSCs), human umbilical cord MSCs, human induced pluripotent stem cell-derived MSCs and human embry. J. Tissue Eng. Regen. Med. 12 (1), 191–203. doi:10.1002/term.2395
Chen, W., Zhou, H., Weir, M. D., Bao, C., and Xu, H. H. (2012). Umbilical cord stem cells released from alginate–fibrin microbeads inside macroporous and biofunctionalized calcium phosphate cement for bone regeneration. Acta biomater. 8 (6), 2297–2306. doi:10.1016/j.actbio.2012.02.021
Chen, X., Bai, S., Li, B., Liu, H., Wu, G., Liu, S., et al. (2016). Fabrication of gelatin methacrylate/nanohydroxyapatite microgel arrays for periodontal tissue regeneration. Int. J. Nanomedicine 11, 4707–4718. doi:10.2147/ijn.s111701
Chen, Y-W., Hsu, T-T., Wang, K., and Shie, M-Y. (2016). Preparation of the fast setting and degrading Ca–Si–Mg cement with both odontogenesis and angiogenesis differentiation of human periodontal ligament cells. Mater. Sci. Eng. C 60, 374–383. doi:10.1016/j.msec.2015.11.064
Chen, Y-Y., He, S-T., Yan, F-H., Zhou, P-F., Luo, K., Zhang, Y-D., et al. (2016). Dental pulp stem cells express tendon markers under mechanical loading and are a potential cell source for tissue engineering of tendon-like tissue. Int. J. Oral Sci. 8 (4), 213–222. doi:10.1038/ijos.2016.33
Cheng, C. W., Solorio, L. D., and Alsberg, E. (2014). Decellularized tissue and cell-derived extracellular matrices as scaffolds for orthopaedic tissue engineering. Biotechnol. Adv. 32 (2), 462–484. doi:10.1016/j.biotechadv.2013.12.012
Cheng, H., Chabok, R., Guan, X., Chawla, A., Li, Y., Khademhosseini, A., et al. (2018). Synergistic interplay between the two major bone minerals, hydroxyapatite and whitlockite nanoparticles, for osteogenic differentiation of mesenchymal stem cells. Acta biomater. 69, 342–351. doi:10.1016/j.actbio.2018.01.016
Cheng, H., Xiong, W., Fang, Z., Guan, H., Wu, W., Li, Y., et al. (2016). Strontium (Sr) and silver (Ag) loaded nanotubular structures with combined osteoinductive and antimicrobial activities. Acta biomater. 31, 388–400. doi:10.1016/j.actbio.2015.11.046
Chien, K-H., Chang, Y-L., Wang, M-L., Chuang, J-H., Yang, Y-C., Tai, M-C., et al. (2018). Promoting induced pluripotent stem cell-driven biomineralization and periodontal regeneration in rats with maxillary-molar defects using injectable BMP-6 hydrogel. Sci. Rep. 8 (1), 114–213. doi:10.1038/s41598-017-18415-6
Chiu, Y-C., Shie, M-Y., Lin, Y-H., Lee, A. K-X., and Chen, Y-W. (2019). Effect of strontium substitution on the physicochemical properties and bone regeneration potential of 3D printed calcium silicate scaffolds. Int. J. Mol. Sci. 20 (11), 2729. doi:10.3390/ijms20112729
Chocholata, P., Kulda, V., and Babuska, V. (2019). Fabrication of scaffolds for bone-tissue regeneration. Materials 12 (4), 568. doi:10.3390/ma12040568
Christ, G. J., Siriwardane, M. L., and de Coppi, P. (2015). Engineering muscle tissue for the fetus: Getting ready for a strong life. Front. Pharmacol. 6, 53. doi:10.3389/fphar.2015.00053
Chua, A. W. C., Khoo, Y. C., Tan, B. K., Tan, K. C., Foo, C. L., and Chong, S. J. (2016). Skin tissue engineering advances in severe burns: Review and therapeutic applications. Burns Trauma 4 (1), 3. doi:10.1186/s41038-016-0027-y
Cooper, L. F., Ravindran, S., Huang, C-C., and Kang, M. (2019). A role for exosomes in craniofacial tissue engineering and regeneration. Front. Physiol. 10, 1569. doi:10.3389/fphys.2019.01569
Coringa, R., de Sousa, E. M., Botelho, J. N., Diniz, R. S., de Sá, J. C., da Cruz, M. C. F. N., et al. (2018). Bone substitute made from a Brazilian oyster shell functions as a fast stimulator for bone-forming cells in an animal model. PloS one 13 (6), e0198697. doi:10.1371/journal.pone.0198697
D'Amora, U., Russo, T., Gloria, A., Rivieccio, V., D'Antò, V., Negri, G., et al. (2017). 3D additive-manufactured nanocomposite magnetic scaffolds: Effect of the application mode of a time-dependent magnetic field on hMSCs behavior. Bioact. Mater. 2 (3), 138–145. doi:10.1016/j.bioactmat.2017.04.003
da Costa Pereira, L., de Almeida Barros Mourão, C. F., Neves Novellino Alves, A. T., Figueiredo de Brito Resende, R., de Uzeda, P. G., José, M., et al. (2019). In vitro physico-chemical characterization and standardized in vivo evaluation of biocompatibility of a new synthetic membrane for guided bone regeneration. Materials 12 (7), 1186. doi:10.3390/ma12071186
Dam, V. V., Trinh, H. A., Rokaya, D., and Trinh, D. H. (2022). Bone augmentation for implant placement: Recent advances. Int. J. Dent. 2022, 1–7. doi:10.1155/2022/8900940
Dang, M., Koh, A. J., Jin, X., McCauley, L. K., and Ma, P. X. (2017). Local pulsatile PTH delivery regenerates bone defects via enhanced bone remodeling in a cell-free scaffold. Biomaterials 114, 1–9. doi:10.1016/j.biomaterials.2016.10.049
Dasari, A., Xue, J., and Deb, S. (2022). Magnetic nanoparticles in bone tissue engineering. Nanomater. (Basel, Switz. 12 (5), 757. doi:10.3390/nano12050757
De Carvalho, C. R., Oliveira, J. M., and Reis, R. L. (2019). Modern trends for peripheral nerve repair and regeneration: Beyond the hollow nerve guidance conduit. Front. Bioeng. Biotechnol. 7, 337. doi:10.3389/fbioe.2019.00337
del Carmen Ortuño‐Costela, M., García‐López, M., Cerrada, V., and Gallardo, M. E. (2019). iPSC s: A powerful tool for skeletal muscle tissue engineering. J. Cell. Mol. Med. 23 (6), 3784–3794. doi:10.1111/jcmm.14292
del Castillo-Santaella, T., Ortega-Oller, I., Padial-Molina, M., O’Valle, F., Galindo-Moreno, P., Jódar-Reyes, A. B., et al. (2019). Formulation, colloidal characterization, and in vitro biological effect of BMP-2 loaded PLGA nanoparticles for bone regeneration. Pharmaceutics 11 (8), 388. doi:10.3390/pharmaceutics11080388
Demarco, F. F., Conde, M. C. M., Cavalcanti, B. N., Casagrande, L., Sakai, V. T., and Nör, J. E. (2011). Dental pulp tissue engineering. Braz. Dent. J. 22 (1), 3–13. doi:10.1590/s0103-64402011000100001
Denry, I., Goudouri, O-M., Fredericks, D. C., Akkouch, A., Acevedo, M. R., and Holloway, J. A. (2018). Strontium-releasing fluorapatite glass-ceramic scaffolds: Structural characterization and in vivo performance. Acta biomater. 75, 463–471. doi:10.1016/j.actbio.2018.05.047
Deschamps, I. S., Magrin, G. L., Magini, R. S., Fredel, M. C., Benfatti, C. A., and Souza, J. C. (2017). On the synthesis and characterization of β-tricalcium phosphate scaffolds coated with collagen or poly (D, L-lactic acid) for alveolar bone augmentation. Eur. J. Dent. 11 (04), 496–502. doi:10.4103/ejd.ejd_4_17
Diniz, I. M., Chen, C., Xu, X., Ansari, S., Zadeh, H. H., Marques, M. M., et al. (2015). Pluronic F-127 hydrogel as a promising scaffold for encapsulation of dental-derived mesenchymal stem cells. J. Mat. Sci. Mat. Med. 26 (3), 153. doi:10.1007/s10856-015-5493-4
Diomede, F., Gugliandolo, A., Cardelli, P., Merciaro, I., Ettorre, V., Traini, T., et al. (2018). Three-dimensional printed PLA scaffold and human gingival stem cell-derived extracellular vesicles: A new tool for bone defect repair. Stem Cell Res. Ther. 9 (1), 104. doi:10.1186/s13287-018-0850-0
Diomede, F., Gugliandolo, A., Scionti, D., Merciaro, I., Cavalcanti, M. F., Mazzon, E., et al. (2018). Biotherapeutic effect of gingival stem cells conditioned medium in bone tissue restoration. Int. J. Mol. Sci. 19 (2), 329. doi:10.3390/ijms19020329
Dissanayaka, W. L., Hargreaves, K. M., Jin, L., Samaranayake, L. P., and Zhang, C. (2015). The interplay of dental pulp stem cells and endothelial cells in an injectable peptide hydrogel on angiogenesis and pulp regeneration in vivo. Tissue Eng. Part A 21 (3-4), 550–563. doi:10.1089/ten.tea.2014.0154
Doulabi, A. H., Mequanint, K., and Mohammadi, H. (2014). Blends and nanocomposite biomaterials for articular cartilage tissue engineering. Materials 7 (7), 5327–5355. doi:10.3390/ma7075327
Du, F., Wu, H., Li, H., Cai, L., Wang, Q., Liu, X., et al. (2017). Bone marrow mononuclear cells combined with beta-tricalcium phosphate granules for alveolar cleft repair: A 12-month clinical study. Sci. Rep. 7 (1), 13773–13778. doi:10.1038/s41598-017-12602-1
DuRaine, G. D., Brown, W. E., Hu, J. C., and Athanasiou, K. A. (2015). Emergence of scaffold-free approaches for tissue engineering musculoskeletal cartilages. Ann. Biomed. Eng. 43 (3), 543–554. doi:10.1007/s10439-014-1161-y
Durairaj, R., SivasaravananSharma, D., Sharma, D.K., Ramachandran, S., and Heboyan, A. (2021). Investigations on mechanical properties of titanium reinforced glass ionomer cement (GiC)—ceramic composites suitable for dental implant applications. Dig. J. Nanomater Biostruct 16, 161–167.
Eftekhari, A., Maleki Dizaj, S., Sharifi, S., Salatin, S., Rahbar Saadat, Y., Zununi Vahed, S., et al. (2020). The use of nanomaterials in tissue engineering for cartilage regeneration; current approaches and future perspectives. Int. J. Mol. Sci. 21 (2), 536. doi:10.3390/ijms21020536
El-Gendy, R., Kirkham, J., Newby, P. J., Mohanram, Y., Boccaccini, A. R., and Yang, X. B. (2015). Investigating the vascularization of tissue-engineered bone constructs using dental pulp cells and 45S5 Bioglass® scaffolds. Tissue Eng. Part A 21 (13-14), 2034–2043. doi:10.1089/ten.tea.2014.0485
Eltom, A., Zhong, G., and Muhammad, A. (2019). Scaffold techniques and designs in tissue engineering functions and purposes: A review. Adv. Mater. Sci. Eng. 2019, 1–13. doi:10.1155/2019/3429527
Engelke, W., Lazzarini, M., and Beltrán, V. (2018). Hard tissue preservation in minimally invasive mandibular third molar surgery using in situ hardening TCP bone filler. BioMed Res. Int. 2018, 1–9. doi:10.1155/2018/5274754
Ezati, M., Safavipour, H., Houshmand, B., and Faghihi, S. (2018). Development of a PCL/gelatin/chitosan/β-TCP electrospun composite for guided bone regeneration. Prog. Biomater. 7 (3), 225–237. doi:10.1007/s40204-018-0098-x
Fahimipour, F., Dashtimoghadam, E., Rasoulianboroujeni, M., Yazdimamaghani, M., Khoshroo, K., Tahriri, M., et al. (2018). Collagenous matrix supported by a 3D-printed scaffold for osteogenic differentiation of dental pulp cells. Dent. Mater. 34 (2), 209–220. doi:10.1016/j.dental.2017.10.001
Fairbairn, P., Leventis, M., Mangham, C., and Horowitz, R. (2018). Alveolar ridge preservation using a novel synthetic grafting material: A case with two-year follow-up. Case Rep. Dent. 2018, 1–8. doi:10.1155/2018/6412806
Fakheran, O., Birang, R., Schmidlin, P. R., Razavi, S. M., and Behfarnia, P. (2019). Retro MTA and tricalcium phosphate/retro MTA for guided tissue regeneration of periodontal dehiscence defects in a dog model: A pilot study. Biomater. Res. 23 (1), 14–17. doi:10.1186/s40824-019-0163-0
Fang, C-H., Lin, Y-W., Lin, F-H., Sun, J-S., Chao, Y-H., Lin, H-Y., et al. (2019). Biomimetic synthesis of nanocrystalline hydroxyapatite composites: Therapeutic potential and effects on bone regeneration. Int. J. Mol. Sci. 20 (23), 6002. doi:10.3390/ijms20236002
Farré-Guasch, E., Bravenboer, N., Helder, M. N., Schulten, E. A., Ten Bruggenkate, C. M., and Klein-Nulend, J. (2018). Blood vessel formation and bone regeneration potential of the stromal vascular fraction seeded on a calcium phosphate scaffold in the human maxillary sinus floor elevation model. Materials 11 (1), 161. doi:10.3390/ma11010161
Ferreira, M. M., Brito, A. F., Brazete, D., Pereira, I. C., Carrilho, E., Abrantes, A. M., et al. (2019). Doping β-TCP as a strategy for enhancing the regenerative potential of composite β-TCP—alkali-free bioactive glass bone grafts. Experimental study in rats. Materials 12 (1), 4. doi:10.3390/ma12010004
Flores-Cedillo, M., Alvarado-Estrada, K., Pozos-Guillén, A., Murguia-Ibarra, J., Vidal, M., Cervantes-Uc, J., et al. (2016). Multiwall carbon nanotubes/polycaprolactone scaffolds seeded with human dental pulp stem cells for bone tissue regeneration. J. Mat. Sci. Mat. Med. 27 (2), 35. doi:10.1007/s10856-015-5640-y
Fouad, H., AlFotawi, R., Alothman, O. Y., Alshammari, B. A., Alfayez, M., Hashem, M., et al. (2018). Porous polyethylene coated with functionalized hydroxyapatite particles as a bone reconstruction material. Materials 11 (4), 521. doi:10.3390/ma11040521
Fragogeorgi, E. A., Rouchota, M., Georgiou, M., Velez, M., Bouziotis, P., and Loudos, G. (2019). In vivo imaging techniques for bone tissue engineering. J. Tissue Eng. 10, 204173141985458. doi:10.1177/2041731419854586
Francis, S. L., Di Bella, C., Wallace, G. G., and Choong, P. F. (2018). Cartilage tissue engineering using stem cells and bioprinting technology—Barriers to clinical translation. Front. Surg. 5, 70. doi:10.3389/fsurg.2018.00070
Frasca, S., Norol, F., Le Visage, C., Collombet, J-M., Letourneur, D., Holy, X., et al. (2017). Calcium-phosphate ceramics and polysaccharide-based hydrogel scaffolds combined with mesenchymal stem cell differently support bone repair in rats. J. Mat. Sci. Mat. Med. 28 (2), 35. doi:10.1007/s10856-016-5839-6
Fu, L., Wang, Z., Dong, S., Cai, Y., Ni, Y., Zhang, T., et al. (2017). Bilayer poly (lactic-co-glycolic acid)/nano-hydroxyapatite membrane with barrier function and osteogenesis promotion for guided bone regeneration. Materials 10 (3), 257. doi:10.3390/ma10030257
Fu, N., Meng, Z., Jiao, T., Luo, X., Tang, Z., Zhu, B., et al. (2019). P34HB electrospun fibres promote bone regeneration in vivo. Cell Prolif. 52 (3), e12601. doi:10.1111/cpr.12601
Funda, G., Taschieri, S., Bruno, G. A., Grecchi, E., Paolo, S., Girolamo, D., et al. (2020). Nanotechnology scaffolds for alveolar bone regeneration. Materials 13 (1), 201. doi:10.3390/ma13010201
Fuoco, C., Petrilli, L. L., Cannata, S., and Gargioli, C. (2016). Matrix scaffolding for stem cell guidance toward skeletal muscle tissue engineering. J. Orthop. Surg. Res. 11 (1), 86. doi:10.1186/s13018-016-0421-y
Gaharwar, A. K., Mukundan, S., Karaca, E., Dolatshahi-Pirouz, A., Patel, A., Rangarajan, K., et al. (2014). Nanoclay-enriched poly (ɛ-caprolactone) electrospun scaffolds for osteogenic differentiation of human mesenchymal stem cells. Tissue Eng. Part A 20 (15-16), 2088–2101. doi:10.1089/ten.tea.2013.0281
Gaihre, B., Uswatta, S., and Jayasuriya, A. C. (2017). Reconstruction of craniomaxillofacial bone defects using tissue-engineering strategies with injectable and non-injectable scaffolds. J. Funct. Biomater. 8 (4), 49. doi:10.3390/jfb8040049
Gali, R. S., Chinnaswamy, R., Devireddy, S. K., Shaik, M. V., Kumar, R. V. K., Kanubaddy, S. R., et al. (2018). Concentrated bone marrow aspirate-coated hydroxyapatite for reconstruction of small-to-moderate-sized mandibular defects caused by the removal of benign pathologies. Contemp. Clin. Dent. 9 (4), 535. doi:10.4103/ccd.ccd_745_18
Galler, K. M., Brandl, F. P., Kirchhof, S., Widbiller, M., Eidt, A., Buchalla, W., et al. (2018). Suitability of different natural and synthetic biomaterials for dental pulp tissue engineering. Tissue Eng. Part A 24 (3-4), 234–244. doi:10.1089/ten.tea.2016.0555
Galler, K. M., Hartgerink, J. D., Cavender, A. C., Schmalz, G., and D'Souza, R. N. (2012). A customized self-assembling peptide hydrogel for dental pulp tissue engineering. Tissue Eng. Part A 18 (1-2), 176–184. doi:10.1089/ten.tea.2011.0222
Gao, Z., Wu, T., Xu, J., Liu, G., Xie, Y., Zhang, C., et al. (2014). Generation of bioartificial salivary gland using whole-organ decellularized bioscaffold. Cells Tissues Organs 200 (3-4), 171–180. doi:10.1159/000371873
Gentile, P., Chiono, V., Carmagnola, I., and Hatton, P. V. (2014). An overview of poly (lactic-co-glycolic) acid (PLGA)-based biomaterials for bone tissue engineering. Int. J. Mol. Sci. 15 (3), 3640–3659. doi:10.3390/ijms15033640
Ghandhi, D., Bodani, N., Lal, A., Maqsood, A., Ahmed, N., Basha, S., et al. (2022). Evaluation of social media usage by dental practitioners of Pakistan for professional purposes - a cross-sectional study. Clin. Cosmet. Investig. Dent. 14, 245–252. doi:10.2147/ccide.s374111
Ghassemi, T., Shahroodi, A., Ebrahimzadeh, M. H., Mousavian, A., Movaffagh, J., and Moradi, A. (2018). Current concepts in scaffolding for bone tissue engineering. Arch. Bone Jt. Surg. 6 (2), 90–99.
Ghensi, P., Bressan, E., Gardin, C., Ferroni, L., Soldini, M. C., Mandelli, F., et al. (2017). The biological properties of OGI surfaces positively act on osteogenic and angiogenic commitment of mesenchymal stem cells. Materials 10 (11), 1321. doi:10.3390/ma10111321
Godoy-Gallardo, M., Guillem-Marti, J., Sevilla, P., Manero, J. M., Gil, F. J., and Rodriguez, D. (2016). Anhydride-functional silane immobilized onto titanium surfaces induces osteoblast cell differentiation and reduces bacterial adhesion and biofilm formation. Mater. Sci. Eng. C 59, 524–532. doi:10.1016/j.msec.2015.10.051
Gopalakrishnan, U., Murthy, R. T., Felicita, A. S., Alshehri, A., Awadh, W., Almalki, A., et al. (2022). Sulfate-reducing bacteria in patients undergoing fixed orthodontic treatment. Int. Dent. J. S0020-6539(22)00181-2. doi:10.1016/j.identj.2022.07.007
Grasman, J. M., Zayas, M. J., Page, R. L., and Pins, G. D. (2015). Biomimetic scaffolds for regeneration of volumetric muscle loss in skeletal muscle injuries. Acta biomater. 25, 2–15. doi:10.1016/j.actbio.2015.07.038
Guazzo, R., Gardin, C., Bellin, G., Sbricoli, L., Ferroni, L., Ludovichetti, F. S., et al. (2018). Graphene-based nanomaterials for tissue engineering in the dental field. Nanomaterials 8 (5), 349. doi:10.3390/nano8050349
Haeri, M., Sagomonyants, K., Mina, M., Kuhn, L. T., and Goldberg, A. J. (2017). Enhanced differentiation of dental pulp cells cultured on microtubular polymer scaffolds in vitro. Regen. Eng. Transl. Med. 3 (2), 94–105. doi:10.1007/s40883-017-0033-z
Hagandora, C. K., Gao, J., Wang, Y., and Almarza, A. J. (2013). Poly (glycerol sebacate): A novel scaffold material for temporomandibular joint disc engineering. Tissue Eng. Part A 19 (5-6), 729–737. doi:10.1089/ten.tea.2012.0304
Hamlet, S. M., Vaquette, C., Shah, A., Hutmacher, D. W., and Ivanovski, S. (2017). 3‐Dimensional functionalized polycaprolactone‐hyaluronic acid hydrogel constructs for bone tissue engineering. J. Clin. Periodontol. 44 (4), 428–437. doi:10.1111/jcpe.12686
Hara, E., Honda, Y., Suzuki, O., Tanaka, T., and Matsumoto, N. (2018). Epigallocatechin gallate-modified gelatins with different compositions alter the quality of regenerated bones. Int. J. Mol. Sci. 19 (10), 3232. doi:10.3390/ijms19103232
Hashemi-Beni, B., Khoroushi, M., Foroughi, M. R., Karbasi, S., and Khademi, A. A. (2018). Cytotoxicity assessment of polyhydroxybutyrate/chitosan/nano-bioglass nanofiber scaffolds by stem cells from human exfoliated deciduous teeth stem cells from dental pulp of exfoliated deciduous tooth. Dent. Res. J. 15 (2), 136. doi:10.4103/1735-3327.226524
Heboyan, A., and Avetisyan, A. (2019). Clinical case of root resorption due to improper orthodontic treatment. J. Res. Med. Dent. Sci. 7, 91–93.
Heboyan, A., Avetisyan, A., Karobari, M. I., Marya, A., Khurshid, Z., Rokaya, D., et al. (2022). Tooth root resorption: A review. Sci. Prog. 105 (3), 003685042211092. doi:10.1177/00368504221109217
Heboyan, A., Avetisyan, A., Skallevold, H. E., Rokaya, D., Marla, V., and Vardanyan, A. (2021). Occurrence of recurrent aphthous stomatitis (RAS) as a rare oral manifestation in a patient with gilbert’s syndrome. Case Rep. Dent. 2021, 1–5. doi:10.1155/2021/6648729
Heboyan, A., Avetisyan, A., and Vardanyan, A. (2019). Halitosis as an issue of social and psychological significance. J. Res. Med. Dent. Sci. 7 (4), 33–40.
Heboyan, A., Karobari, M. I., Alwadaani, A. H., Marya, A., and Zafar, M. S. (2022). Bruxism as a consequence of stress and movement disorders: Brief review. Eur. J. General Dent. doi:10.1055/s-0042-1754374
Heboyan, A., Lo Giudice, R., Kalman, L., Zafar, M. S., and Tribst, J. P. M. (2022). Stress distribution pattern in zygomatic implants supporting different superstructure materials. Mater. (Basel, Switz. 15 (14), 4953. doi:10.3390/ma15144953
Heboyan, A., Manrikyan, M., Markaryan, M., and Vardanyan, I. (2020). Changes in the parameters of gingival crevicular fluid in masticatory function restoration by various prosthodontic constructions. Int. J. Pharm. Res. 12, 2088–2093.
Heboyan, A., Manrikyan, M., Zafar, M. S., Rokaya, D., Nushikyan, R., Vardanyan, I., et al. (2021). Bacteriological evaluation of gingival crevicular fluid in teeth restored using fixed dental prostheses: An in vivo study. Int. J. Mol. Sci. 22 (11), 5463. doi:10.3390/ijms22115463
Heboyan, A. G. (2019). Marginal and internal fit of fixed prosthodontic constructions: A literature review Int. j. dent. res. rev. 2 (19).
Heboyan, A., Marya, A., Syed, A. U. Y., Khurshid, Z., Zafar, M. S., Rokaya, D., et al. (2022). In vitro microscopic evaluation of metal- and zirconium-oxide-based crowns’ marginal fit. Pesqui. Bras. Odontopediatria Clin. Integr. 22. doi:10.1590/pboci.2022.010
Heboyan, A., Syed, A. U. Y., Rokaya, D., Cooper, P. R., Manrikyan, M., and Markaryan, M. (2020). Cytomorphometric analysis of inflammation dynamics in the periodontium following the use of fixed dental prostheses. Mol. (Basel, Switz. 25 (20), 4650. doi:10.3390/molecules25204650
Heboyan, A., Zafar, M. S., Karobari, M. I., and Tribst, J. P. M. (2022). Insights into polymeric materials for prosthodontics and dental implantology. Materials 15 (15), 5383. doi:10.3390/ma15155383
Heboyan, A., Zafar, M. S., Rokaya, D., and Khurshid, Z. (2022). Insights and advancements in biomaterials for prosthodontics and implant dentistry. Mol. (Basel, Switz. 27 (16), 5116. doi:10.3390/molecules27165116
Heboyan, A. G., and Avetisyan, A. A. (2019). Comparative assessment of periodontal complex by experimental method and elaboration of new protocols for corresponding treatment of external and perforating internal resorption resulted many years after trauma. Biomed. Res. (Aligarh). 30 (5), 693–696. doi:10.35841/biomedicalresearch.30-19-285
Heboyan, A. G., Avetisyan, A. A., and Mm, M. (2018). Clinical case of a rarely diagnosed tooth root internal resorption. New Armen. Med. J. 12, 87–92.
Heboyan, A. G., Avetisyan, A. A., and Mm, M. (2018). Rare clinical case of tooth root external resorption as a delayed post-traumatic complication. New Armen. Med. J. 12, 93–98.
Hegedűs, O., Juriga, D., Sipos, E., Voniatis, C., Juhász, Á., Idrissi, A., et al. (2019). Free thiol groups on poly (aspartamide) based hydrogels facilitate tooth-derived progenitor cell proliferation and differentiation. PloS one 14 (12), e0226363. doi:10.1371/journal.pone.0226363
Helder, M. N., van Esterik, F. A., Kwehandjaja, M. D., ten Bruggenkate, C. M., Klein‐Nulend, J., and Schulten, E. A. (2018). Evaluation of a new biphasic calcium phosphate for maxillary sinus floor elevation: Micro‐CT and histomorphometrical analyses. Clin. Oral Implants Res. 29 (5), 488–498. doi:10.1111/clr.13146
Henrich, D., Verboket, R., Schaible, A., Kontradowitz, K., Oppermann, E., Brune, J. C., et al. (2015). Characterization of bone marrow mononuclear cells on biomaterials for bone tissue engineering in vitro. BioMed Res. Int. 2015, 1–12. doi:10.1155/2015/762407
Hernández-Monjaraz, B., Santiago-Osorio, E., Ledesma-Martínez, E., Alcauter-Zavala, A., and Mendoza-Núñez, V. M. (2018). Retrieval of a periodontally compromised tooth by allogeneic grafting of mesenchymal stem cells from dental pulp: A case report. J. Int. Med. Res. 46 (7), 2983–2993. doi:10.1177/0300060518773244
Hettich, G., Schierjott, R. A., Epple, M., Gbureck, U., Heinemann, S., Mozaffari-Jovein, H., et al. (2019). Calcium phosphate bone graft substitutes with high mechanical load capacity and high degree of interconnecting porosity. Materials 12 (21), 3471. doi:10.3390/ma12213471
Hidalgo Pitaluga, L., Trevelin Souza, M., Dutra Zanotto, E., Santocildes Romero, M. E., and Hatton, P. V. (2018). Electrospun F18 bioactive glass/PCL—poly (ε-caprolactone)—membrane for guided tissue regeneration. Materials 11 (3), 400. doi:10.3390/ma11030400
Ho, K-N., Salamanca, E., Chang, K-C., Shih, T-C., Chang, Y-C., Huang, H-M., et al. (2016). A novel HA/β-TCP-collagen composite enhanced new bone formation for dental extraction socket preservation in beagle dogs. Materials 9 (3), 191. doi:10.3390/ma9030191
Holmes, R., Yang, X-B., Dunne, A., Florea, L., Wood, D., and Tronci, G. (2017). Thiol-ene photo-click collagen-PEG hydrogels: Impact of water-soluble photoinitiators on cell viability, gelation kinetics and rheological properties. Polymers 9 (6), 226. doi:10.3390/polym9060226
Huang, B. J., Hu, J. C., and Athanasiou, K. A. (2016). Cell-based tissue engineering strategies used in the clinical repair of articular cartilage. Biomaterials 98, 1–22. doi:10.1016/j.biomaterials.2016.04.018
Huang, G. T. (2009). Pulp and dentin tissue engineering and regeneration: Current progress. Regen. Med. 4 (5), 697–707. doi:10.2217/rme.09.45
Huang, G. T-J. (2011). Dental pulp and dentin tissue engineering and regeneration–advancement and challenge. Front. Biosci. 3, 286–800. doi:10.2741/e286
Hussain, A., Tebyaniyan, H., and Khayatan, D. (2022). The role of epigenetic in dental and oral regenerative medicine by different types of dental stem cells: A comprehensive overview. Stem Cells Int. 2022, 1–15. doi:10.1155/2022/5304860
Hwang, K-S., Choi, J-W., Kim, J-H., Chung, H. Y., Jin, S., Shim, J-H., et al. (2017). Comparative efficacies of collagen-based 3D printed PCL/PLGA/β-TCP composite block bone grafts and biphasic calcium phosphate bone substitute for bone regeneration. Materials 10 (4), 421. doi:10.3390/ma10040421
Jain, D., Mohan, R., and Singh, V. D. (2019). Comparison of microsurgical and macrosurgical technique using bioactive synthetic bone graft and collagen membrane for an implant site development: A randomized controlled clinical trial. J. Indian Soc. Periodontol. 23 (5), 448–460. doi:10.4103/jisp.jisp_738_18
Jamal, M., Greish, Y., Chogle, S., Goodis, H., and Karam, S. M. (2018). Growth and differentiation of dental stem cells of apical papilla on polycaprolactone scaffolds. Adv. Exp. Med. Biol. 1077, 31–40. doi:10.1007/978-981-13-0947-2_3
Jang, Y-J., Jung, I-H., Park, J-C., Jung, U-W., Kim, C-S., Lee, Y-K., et al. (2011). Effect of seeding using an avidin-biotin binding system on the attachment of periodontal ligament fibroblasts to nanohydroxyapatite scaffolds: Three-dimensional culture. J. Periodontal Implant Sci. 41 (2), 73–78. doi:10.5051/jpis.2011.41.2.73
Jang, Y-S., Manivong, P., Kim, Y-K., Kim, K-S., Lee, S-J., Bae, T-S., et al. (2018). In vitro and in vivo characterization of N-Acetyl-L-Cysteine loaded beta-tricalcium phosphate scaffolds. Int. J. Biomat. 2018, 1–11. doi:10.1155/2018/9457910
Jauregui, C., Yoganarasimha, S., and Madurantakam, P. (2018). Mesenchymal stem cells derived from healthy and diseased human gingiva support osteogenesis on electrospun Polycaprolactone scaffolds. Bioengineering 5 (1), 8. doi:10.3390/bioengineering5010008
Jayakumar, R., Chennazhi, K. P., Srinivasan, S., Nair, S. V., Furuike, T., and Tamura, H. (2011). Chitin scaffolds in tissue engineering. Int. J. Mol. Sci. 12 (3), 1876–1887. doi:10.3390/ijms12031876
Jenkins, T. L., and Little, D. (2019). Synthetic scaffolds for musculoskeletal tissue engineering: Cellular responses to fiber parameters. npj Regen. Med. 4 (1), 15. doi:10.1038/s41536-019-0076-5
Ji, S., Dube, K., Chesterman, J. P., Fung, S. L., Liaw, C-Y., Kohn, J., et al. (2019). Polyester-based ink platform with tunable bioactivity for 3D printing of tissue engineering scaffolds. Biomater. Sci. 7 (2), 560–570. doi:10.1039/c8bm01269e
Jian, B., Wu, W., Song, Y., Tan, N., and Ma, C. (2019). <p>Microporous elastomeric membranes fabricated with polyglycerol sebacate improved guided bone regeneration in a rabbit model</p>. Int. J. Nanomedicine 14, 2683–2692. doi:10.2147/ijn.s192167
Jo, S. B., Erdenebileg, U., Dashnyam, K., Jin, G-Z., Cha, J-R., El-Fiqi, A., et al. (2020). Nano-graphene oxide/polyurethane nanofibers: Mechanically flexible and myogenic stimulating matrix for skeletal tissue engineering. J. Tissue Eng. 11, 204173141990042. doi:10.1177/2041731419900424
Jo, Y-Y., Kim, S-G., Kwon, K-J., Kweon, H., Chae, W-S., Yang, W-G., et al. (2017). Silk fibroin-alginate-hydroxyapatite composite particles in bone tissue engineering applications in vivo. Int. J. Mol. Sci. 18 (4), 858. doi:10.3390/ijms18040858
Joo, M-J., Cha, J-K., Lim, H-C., Choi, S-H., and Jung, U-W. (2017). Sinus augmentation using rhBMP-2-loaded synthetic bone substitute with simultaneous implant placement in rabbits. J. Periodontal Implant Sci. 47 (2), 86–95. doi:10.5051/jpis.2017.47.2.86
Jose, G., Shalumon, K., Liao, H-T., Kuo, C-Y., and Chen, J-P. (2020). Preparation and characterization of surface heat sintered nanohydroxyapatite and nanowhitlockite embedded poly (Lactic-co-glycolic acid) microsphere bone graft scaffolds: In vitro and in vivo studies. Int. J. Mol. Sci. 21 (2), 528. doi:10.3390/ijms21020528
Kajii, F., Iwai, A., Tanaka, H., Matsui, K., Kawai, T., and Kamakura, S. (2018). Single‐dose local administration of teriparatide with a octacalcium phosphate collagen composite enhances bone regeneration in a rodent critical‐sized calvarial defect. J. Biomed. Mat. Res. 106 (5), 1851–1857. doi:10.1002/jbm.b.33993
Kakkad, N., Yadav, N. S., Hazari, P., Narwani, S., Somkuwar, K., Basha, S., et al. (2022). Comparative evaluation of tensile bond strength of poly ether ether ketone (PEEK) and zirconia copings using resin cement with or without adhesive: An in vitro study. Mater. (Basel, Switz. 15 (12), 4167. doi:10.3390/ma15124167
Kamath, M. S., Ahmed, S. S., Dhanasekaran, M., and Santosh, S. W. (2014). Polycaprolactone scaffold engineered for sustained release of resveratrol: Therapeutic enhancement in bone tissue engineering. Int. J. Nanomedicine 9, 183–195. doi:10.2147/IJN.S49460
Kämmerer, P. W., Scholz, M., Baudisch, M., Liese, J., Wegner, K., Frerich, B., et al. (2017). Guided bone regeneration using collagen scaffolds, growth factors, and periodontal ligament stem cells for treatment of peri-implant bone defects in vivo. Stem cells Int. 2017, 1–9. doi:10.1155/2017/3548435
Kang, Y., Scully, A., Young, D. A., Kim, S., Tsao, H., Sen, M., et al. (2011). Enhanced mechanical performance and biological evaluation of a PLGA coated β-TCP composite scaffold for load-bearing applications. Eur. Polym. J. 47 (8), 1569–1577. doi:10.1016/j.eurpolymj.2011.05.004
Karimi, M., Bahrami, S., Mirshekari, H., Basri, S. M. M., Nik, A. B., Aref, A. R., et al. (2016). Microfluidic systems for stem cell-based neural tissue engineering. Lab. Chip 16 (14), 2551–2571. doi:10.1039/c6lc00489j
Karobari, M. I., Selvaraj, S., Adil, A., Mansoor Khan, M., Venugopal, A., Rokaya, D., et al. (2022). Modifiable and non-modifiable risk factors affecting oral and periodontal health and quality of life in south asia. Open Dent. J. 22 (16), HT23–2263. doi:10.2174/18742106-v16-e2209270
Kashte, S., Jaiswal, A. K., and Kadam, S. (2017). Artificial bone via bone tissue engineering: Current scenario and challenges. Tissue Eng. Regen. Med. 14 (1), 1–14. doi:10.1007/s13770-016-0001-6
Kawai, T., Echigo, S., Matsui, K., Tanuma, Y., Takahashi, T., Suzuki, O., et al. (2014). First clinical application of octacalcium phosphate collagen composite in human bone defect. Tissue Eng. Part A 20 (7-8), 1336–1341. doi:10.1089/ten.tea.2013.0508
Kawamoto, K., Miyaji, H., Nishida, E., Miyata, S., Kato, A., Tateyama, A., et al. (2018). Characterization and evaluation of graphene oxide scaffold for periodontal wound healing of class II furcation defects in dog. Int. J. Nanomedicine 13, 2365–2376. doi:10.2147/ijn.s163206
Kazem-Arki, M., Kabiri, M., Rad, I., Roodbari, N. H., Hosseinpoor, H., Mirzaei, S., et al. (2018). Enhancement of osteogenic differentiation of adipose-derived stem cells by PRP modified nanofibrous scaffold. Cytotechnology 70 (6), 1487–1498. doi:10.1007/s10616-018-0226-4
Kazemnejad, S., Khanmohammadi, M., Baheiraei, N., and Arasteh, S. (2017). Current state of cartilage tissue engineering using nanofibrous scaffolds and stem cells. Avicenna J. Med. Biotechnol. 9 (2), 50–65.
Ke, D., Dernell, W., Bandyopadhyay, A., and Bose, S. (2015). Doped tricalcium phosphate scaffolds by thermal decomposition of naphthalene: Mechanical properties and in vivo osteogenesis in a rabbit femur model. J. Biomed. Mat. Res. 103 (8), 1549–1559. doi:10.1002/jbm.b.33321
Kesireddy, V. (2016). Evaluation of adipose-derived stem cells for tissue-engineered muscle repair construct-mediated repair of a murine model of volumetric muscle loss injury. Int. J. Nanomedicine 11, 1461–1473. doi:10.2147/ijn.s101955
Khayat, A., Monteiro, N., Smith, E., Pagni, S., Zhang, W., Khademhosseini, A., et al. (2017). GelMA-encapsulated hDPSCs and HUVECs for dental pulp regeneration. J. Dent. Res. 96 (2), 192–199. doi:10.1177/0022034516682005
Kim, F., Chen, T., Burgess, T., Rasie, P., Selinger, T. L., Greschner, A., et al. (2019). Functionalized DNA nanostructures as scaffolds for guided mineralization. Chem. Sci. 10 (45), 10537–10542. doi:10.1039/c9sc02811k
Kim, H. D., Lee, Y., Kim, Y., Hwang, Y., and Hwang, N. S. (2017). Biomimetically reinforced polyvinyl alcohol-based hybrid scaffolds for cartilage tissue engineering. Polymers 9 (12), 655. doi:10.3390/polym9120655
Kim, I-J., and Shin, S-Y. (2018). Comparative study of new bone formation capability of zirconia bone graft material in rabbit calvarial. J. Adv. Prosthodont. 10 (3), 167–176. doi:10.4047/jap.2018.10.3.167
Kim, I. A., and Rhee, S. H. (2017). Preparation of a non‐woven poly (ε‐caprolactone) fabric with partially embedded apatite surface for bone tissue engineering applications by partial surface melting of poly (ε‐caprolactone) fibers. J. Biomed. Mat. Res. A 105 (7), 1973–1983. doi:10.1002/jbm.a.36069
Kim, J., Kim, H. D., Park, J., Lee, E-S., Kim, E., Lee, S. S., et al. (2018). Enhanced osteogenic commitment of murine mesenchymal stem cells on graphene oxide substrate. Biomater. Res. 22 (1), 1. doi:10.1186/s40824-017-0112-8
Kim, J-W., Shin, Y. C., Lee, J-J., Bae, E-B., Jeon, Y-C., Jeong, C-M., et al. (2017). The effect of reduced graphene oxide-coated biphasic calcium phosphate bone graft material on osteogenesis. Int. J. Mol. Sci. 18 (8), 1725. doi:10.3390/ijms18081725
Kim, S-M., Yi, S-A., Choi, S-H., Kim, K-M., and Lee, Y-K. (2012). Gelatin-layered and multi-sized porous β-tricalcium phosphate for tissue engineering scaffold. Nanoscale Res. Lett. 7 (1), 78. doi:10.1186/1556-276x-7-78
Kim, S-Y., Bae, E-B., Huh, J-W., Ahn, J-J., Bae, H-Y., Cho, W-T., et al. (2019). Bone regeneration using a three-dimensional hexahedron channeled BCP block combined with bone morphogenic protein-2 in rat calvarial defects. Materials 12 (15), 2435. doi:10.3390/ma12152435
Kim, Y. S., Majid, M., Melchiorri, A. J., and Mikos, A. G. (2019). Applications of decellularized extracellular matrix in bone and cartilage tissue engineering. Bioeng. Transl. Med. 4 (1), 83–95. doi:10.1002/btm2.10110
Kinoshita, Y., and Maeda, H. (2013). Recent developments of functional scaffolds for craniomaxillofacial bone tissue engineering applications. Sci. World J. 2013, 1–21. doi:10.1155/2013/863157
Kitakami, E., Aoki, M., Sato, C., Ishihata, H., and Tanaka, M. (2014). Adhesion and proliferation of human periodontal ligament cells on poly (2-methoxyethyl acrylate). BioMed Res. Int. 2014, 1–14. doi:10.1155/2014/102648
Klar, A. S., Zimoch, J., and Biedermann, T. (2017). Skin tissue engineering: Application of adipose-derived stem cells. BioMed Res. Int. 2017, 1–12. doi:10.1155/2017/9747010
Koch, F., Wolff, A., Mathes, S., Pieles, U., Saxer, S. S., Kreikemeyer, B., et al. (2018). Amino acid composition of nanofibrillar self-assembling peptide hydrogels affects responses of periodontal tissue cells in vitro. Int. J. Nanomedicine 13, 6717–6733. doi:10.2147/ijn.s173702
Kouketsu, A., Matsui, K., Kawai, T., Ezoe, Y., Yanagisawa, T., Yasuda, A., et al. (2020). Octacalcium phosphate collagen composite stimulates the expression and activity of osteogenic factors to promote bone regeneration. J. Tissue Eng. Regen. Med. 14 (1), 99–107. doi:10.1002/term.2969
Krishnamurithy, G., Mohan, S., Yahya, N. A., Mansor, A., Murali, M. R., Raghavendran, H. R. B., et al. (2019). The physicochemical and biomechanical profile of forsterite and its osteogenic potential of mesenchymal stromal cells. PloS one 14 (3), e0214212. doi:10.1371/journal.pone.0214212
Kuang, R., Zhang, Z., Jin, X., Hu, J., Shi, S., Ni, L., et al. (2016). Nanofibrous spongy microspheres for the delivery of hypoxia-primed human dental pulp stem cells to regenerate vascularized dental pulp. Acta biomater. 33, 225–234. doi:10.1016/j.actbio.2016.01.032
Kumai, T., Yui, N., Yatabe, K., Sasaki, C., Fujii, R., Takenaga, M., et al. (2019). <p>A novel, self-assembled artificial cartilage&ndash;hydroxyapatite conjugate for combined articular cartilage and subchondral bone repair: Histopathological analysis of cartilage tissue engineering in rat knee joints</p>. Int. J. Nanomedicine 14, 1283–1298. doi:10.2147/ijn.s193963
Kumar, A., Akkineni, A. R., Basu, B., and Gelinsky, M. (2016). Three-dimensional plotted hydroxyapatite scaffolds with predefined architecture: Comparison of stabilization by alginate cross-linking versus sintering. J. Biomater. Appl. 30 (8), 1168–1181. doi:10.1177/0885328215617058
Kumar, P., Dehiya, B. S., and Sindhu, A. (2019). Ibuprofen-loaded CTS/nHA/nBG scaffolds for the applications of hard tissue engineering. Iran. Biomed. J. 23 (3), 190–199. doi:10.29252/ibj.23.3.190
Kumar, S. D., Abudhahir, K. M., Selvamurugan, N., Vimalraj, S., Murugesan, R., Srinivasan, N., et al. (2018). Formulation and biological actions of nano-bioglass ceramic particles doped with Calcarea phosphorica for bone tissue engineering. Mater. Sci. Eng. C 83, 202–209. doi:10.1016/j.msec.2017.08.077
Kwon, D. Y., Kwon, J. S., Park, S. H., Park, J. H., Jang, S. H., Yin, X. Y., et al. (2015). A computer-designed scaffold for bone regeneration within cranial defect using human dental pulp stem cells. Sci. Rep. 5, 12721. doi:10.1038/srep12721
Lai, W-Y., Feng, S-W., Chan, Y-H., Chang, W-J., Wang, H-T., and Huang, H-M. (2018). In vivo investigation into effectiveness of Fe3O4/PLLA nanofibers for bone tissue engineering applications. Polymers 10 (7), 804. doi:10.3390/polym10070804
Lambrichts, I., Driesen, R. B., Dillen, Y., Gervois, P., Ratajczak, J., Vangansewinkel, T., et al. (2017). Dental pulp stem cells: Their potential in reinnervation and angiogenesis by using scaffolds. J. Endod. 43 (9), S12–S16. doi:10.1016/j.joen.2017.06.001
Langhals, N. B., Urbanchek, M. G., Ray, A., and Brenner, M. J. (2014). Update in facial nerve paralysis: Tissue engineering and new technologies. Curr. Opin. otolaryngology head neck Surg. 22 (4), 291–299. doi:10.1097/moo.0000000000000062
Laternser, S., Keller, H., Leupin, O., Rausch, M., Graf-Hausner, U., and Rimann, M. (2018). A novel microplate 3D bioprinting platform for the engineering of muscle and tendon tissues. SLAS Technol. 23 (6), 599–613. doi:10.1177/2472630318776594
Law, J. X., Liau, L. L., Saim, A., Yang, Y., and Idrus, R. (2017). Electrospun collagen nanofibers and their applications in skin tissue engineering. Tissue Eng. Regen. Med. 14 (6), 699–718. doi:10.1007/s13770-017-0075-9
Lee, J-S., Park, W-Y., Cha, J-K., Jung, U-W., Kim, C-S., Lee, Y-K., et al. (2012). Periodontal tissue reaction to customized nano-hydroxyapatite block scaffold in one-wall intrabony defect: A histologic study in dogs. J. Periodontal Implant Sci. 42 (2), 50–58. doi:10.5051/jpis.2012.42.2.50
Lee, J. B., Kim, J. E., Bae, M. S., Park, S. A., Balikov, D. A., Sung, H-J., et al. (2016). Development of poly (ɛ-caprolactone) scaffold loaded with simvastatin and beta-cyclodextrin modified hydroxyapatite inclusion complex for bone tissue engineering. Polymers 8 (2), 49. doi:10.3390/polym8020049
Lee, J. W., Chu, S. G., Kim, H. T., Choi, K. Y., Oh, E. J., Shim, J-H., et al. (2017). Osteogenesis of adipose-derived and bone marrow stem cells with polycaprolactone/tricalcium phosphate and three-dimensional printing technology in a dog model of maxillary bone defects. Polymers 9 (9), 450. doi:10.3390/polym9090450
Lee, K-S., Jeon, Y-S., Shin, S-W., and Lee, J-Y. (2017). Effects of rhBMP-2 loaded titanium reinforced collagen membranes on horizontal bone augmentation in dogs. BioMed Res. Int. 2017, 1–8. doi:10.1155/2017/7141296
Lee, M-H., You, C., and Kim, K-H. (2015). Combined effect of a microporous layer and type I collagen coating on a biphasic calcium phosphate scaffold for bone tissue engineering. Materials 8 (3), 1150–1161. doi:10.3390/ma8031150
Lee, S-Y., Wu, S-C., Chen, H., Tsai, L-L., Tzeng, J-J., Lin, C-H., et al. (2018). Synthesis and characterization of polycaprolactone-based polyurethanes for the fabrication of elastic guided bone regeneration membrane. BioMed Res. Int. 2018, 1–13. doi:10.1155/2018/3240571
Lei, B., Guo, B., Rambhia, K. J., and Ma, P. X. (2019). Hybrid polymer biomaterials for bone tissue regeneration. Front. Med. 13 (2), 189–201. doi:10.1007/s11684-018-0664-6
Leventis, M., Agrogiannis, G., Fairbairn, P., Vasiliadis, O., Papavasileiou, D., Theodoropoulou, E., et al. (2018). Evaluation of an in situ hardening β-tricalcium phosphate graft material for alveolar ridge preservation. A histomorphometric animal study in pigs. Dent. J. 6 (3), 27. doi:10.3390/dj6030027
Li, J-H., Liu, D-Y., Zhang, F-M., Fan, W., Zhang, W-K., and Zhang, Z-T. (2011). Human dental pulp stem cell is a promising autologous seed cell for bone tissue engineering. Chin. Med. J. 124 (23), 4022–4028.
Li, K., Wang, C., Yan, J., Zhang, Q., Dang, B., Wang, Z., et al. (2018). Evaluation of the osteogenesis and osseointegration of titanium alloys coated with graphene: An in vivo study. Sci. Rep. 8 (1), 1843–1910. doi:10.1038/s41598-018-19742-y
Liao, H. T., Tsai, M-J., Brahmayya, M., and Chen, J-P. (2018). Bone regeneration using adipose-derived stem cells in injectable thermo-gelling hydrogel scaffold containing platelet-rich plasma and biphasic calcium phosphate. Int. J. Mol. Sci. 19 (9), 2537. doi:10.3390/ijms19092537
Liao, J., Qu, Y., Chu, B., Zhang, X., and Qian, Z. (2015). Biodegradable CSMA/PECA/graphene porous hybrid scaffold for cartilage tissue engineering. Sci. Rep. 5, 9879. doi:10.1038/srep09879
Lim, H-P., Mercado-Pagan, A. E., Yun, K-D., Kang, S-S., Choi, T-H., Bishop, J., et al. (2013). The effect of rhBMP-2 and PRP delivery by biodegradable β-tricalcium phosphate scaffolds on new bone formation in a non-through rabbit cranial defect model. J. Mat. Sci. Mat. Med. 24 (8), 1895–1903. doi:10.1007/s10856-013-4939-9
Lin, H., Cheng, A. W-M., Alexander, P. G., Beck, A. M., and Tuan, R. S. (2014). Cartilage tissue engineering application of injectable gelatin hydrogel with in situ visible-light-activated gelation capability in both air and aqueous solution. Tissue Eng. Part A 20 (17-18), 2402–2411. doi:10.1089/ten.tea.2013.0642
Liu, J., Chen, W., Zhao, Z., and Xu, H. H. (2014). Effect of NELL1 gene overexpression in iPSC-MSCs seeded on calcium phosphate cement. Acta biomater. 10 (12), 5128–5138. doi:10.1016/j.actbio.2014.08.016
Liu, J., Chen, W., Zhao, Z., and Xu, H. H. (2013). Reprogramming of mesenchymal stem cells derived from iPSCs seeded on biofunctionalized calcium phosphate scaffold for bone engineering. Biomaterials 34 (32), 7862–7872. doi:10.1016/j.biomaterials.2013.07.029
Liu, X., Chen, W., Zhang, C., Thein-Han, W., Hu, K., Reynolds, M. A., et al. (2017). Co-seeding human endothelial cells with human-induced pluripotent stem cell-derived mesenchymal stem cells on calcium phosphate scaffold enhances osteogenesis and vascularization in rats. Tissue Eng. Part A 23 (11-12), 546–555. doi:10.1089/ten.tea.2016.0485
Liu, X., Cheng, C., Peng, X., Xiao, H., Guo, C., Wang, X., et al. (2019). A promising material for bone repair: PMMA bone cement modified by dopamine-coated strontium-doped calcium polyphosphate particles. R. Soc. open Sci. 6 (10), 191028. doi:10.1098/rsos.191028
Loison-Robert, L. S., Tassin, M., Bonte, E., Berbar, T., Isaac, J., Berdal, A., et al. (2018). In vitro effects of two silicate-based materials, Biodentine and BioRoot RCS, on dental pulp stem cells in models of reactionary and reparative dentinogenesis. PloS one 13 (1), e0190014. doi:10.1371/journal.pone.0190014
Lopes, D., Martins-Cruz, C., Oliveira, M. B., and Mano, J. F. (2018). Bone physiology as inspiration for tissue regenerative therapies. Biomaterials 185, 240–275. doi:10.1016/j.biomaterials.2018.09.028
Lowe, J., and Almarza, A. J. (2017). A review of in-vitro fibrocartilage tissue engineered therapies with a focus on the temporomandibular joint. Archives oral Biol. 83, 193–201. doi:10.1016/j.archoralbio.2017.07.013
Luo, L., He, Y., Wang, X., Key, B., Lee, B. H., Li, H., et al. (2018). Potential roles of dental pulp stem cells in neural regeneration and repair. Stem cells Int. 2018, 1–15. doi:10.1155/2018/1731289
MacQueen, L. A., Alver, C. G., Chantre, C. O., Ahn, S., Cera, L., Gonzalez, G. M., et al. (2019). Muscle tissue engineering in fibrous gelatin: Implications for meat analogs. npj Sci. Food 3, 20. doi:10.1038/s41538-019-0054-8
Maisani, M., Pezzoli, D., Chassande, O., and Mantovani, D. (2017). Cellularizing hydrogel-based scaffolds to repair bone tissue: How to create a physiologically relevant micro-environment? J. Tissue Eng. 8, 204173141771207. doi:10.1177/2041731417712073
Mangano, F. G., Zecca, P. A., van Noort, R., Apresyan, S., Iezzi, G., Piattelli, A., et al. (2015). Custom-made computer-aided-design/computer-aided-manufacturing biphasic calcium-phosphate scaffold for augmentation of an atrophic mandibular anterior ridge. Case Rep. Dent. 2015, 1–11. doi:10.1155/2015/941265
Mangione, F., Ezeldeen, M., Bardet, C., Lesieur, J., Bonneau, M., Decup, F., et al. (2017). Implanted dental pulp cells fail to induce regeneration in partial pulpotomies. J. Dent. Res. 96 (12), 1406–1413. doi:10.1177/0022034517725523
Marrella, A., Lee, T. Y., Lee, D. H., Karuthedom, S., Syla, D., Chawla, A., et al. (2018). Engineering vascularized and innervated bone biomaterials for improved skeletal tissue regeneration. Mater. Today 21 (4), 362–376. doi:10.1016/j.mattod.2017.10.005
Martens, W., Sanen, K., Georgiou, M., Struys, T., Bronckaers, A., Ameloot, M., et al. (2014). Human dental pulp stem cells can differentiate into Schwann cells and promote and guide neurite outgrowth in an aligned tissue-engineered collagen construct in vitro. FASEB J. 28 (4), 1634–1643. doi:10.1096/fj.13-243980
Marti, M. E., Sharma, A. D., Sakaguchi, D. S., and Mallapragada, S. K. (2013). “10 - nanomaterials for neural tissue engineering,” in Nanomaterials in tissue engineering. Editors A. K. Gaharwar, S. Sant, M. J. Hancock, and S. A. Hacking (United States: Woodhead Publishing), 275–301.
Marya, A., Karobari, M. I., and Heboyan, A. (2022). Rare non-syndromic bilateral maxillary and mandibular permanent canine agenesis. Clin. Case Rep. 10 (7), e6059. doi:10.1002/ccr3.6059
Marya, A., Venugopal, A., Karobari, M. I., Chaudhari, P. K., Heboyan, A., and Rokaya, D. (2022). The contemporary management of cleft lip and palate and the role of artificial intelligence: A review. Open Dent. J. 16 (1). doi:10.2174/18742106-v16-e2202240
Marya, A., Venugopal, A., Karobari, M. I., and Heboyan, A. (2022). Computer vision syndrome: Will the pandemic lead to eye problems for dentists? Pesqui. Bras. Odontopediatria Clin. Integr. 22. doi:10.1590/pboci.2022.009
Matuska, A. M., Muller, S., Dolwick, M. F., and McFetridge, P. S. (2016). Biomechanical and biochemical outcomes of porcine temporomandibular joint disc deformation. Archives oral Biol. 64, 72–79. doi:10.1016/j.archoralbio.2016.01.003
McGovern, J. A., Griffin, M., and Hutmacher, D. W. (2018). Animal models for bone tissue engineering and modelling disease. Dis. Model. Mech. 11 (4), dmm033084. doi:10.1242/dmm.033084
Mehrotra, D. (2013). TMJ bioengineering: A review. J. oral Biol. Craniof. Res. 3 (3), 140–145. doi:10.1016/j.jobcr.2013.07.007
Mercado-Pagán, Á. E., Stahl, A. M., Shanjani, Y., and Yang, Y. (2015). Vascularization in bone tissue engineering constructs. Ann. Biomed. Eng. 43 (3), 718–729. doi:10.1007/s10439-015-1253-3
Mirchandani, B., Zhou, T., Heboyan, A., Yodmongkol, S., and Buranawat, B. (2021). Biomechanical aspects of various attachments for implant overdentures: A review. Polym. (Basel) 13 (19), 3248. doi:10.3390/polym13193248
Mirza, M. B., Gufran, K., Alhabib, O., Alafraa, O., Alzahrani, F., Abuelqomsan, M. S., et al. (2022). CBCT based study to analyze and classify root canal morphology of maxillary molars - a retrospective study. Eur. Rev. Med. Pharmacol. Sci. 26 (18), 6550–6560. doi:10.26355/eurrev_202209_29753
Mohamed, A., and Xing, M. M. (2012). Nanomaterials and nanotechnology for skin tissue engineering. Int. J. Burns Trauma 2 (1), 29–41.
Mohamed, D. A., Abdelfattah, M. I., and Aboulezz, E. H. (2017). The effect of three different biomaterials on proliferation and viability of human dental pulp stem cells (in-vitro study). Open Access Maced. J. Med. Sci. 5 (5), 657–663. doi:10.3889/oamjms.2017.089
Monaco, G., Ladner, Y. D., El Haj, A. J., Forsyth, N. R., Alini, M., and Stoddart, M. J. (2021). Mesenchymal stromal cell differentiation for generating cartilage and bone-like tissues in vitro. Cells 10 (8), 2165. doi:10.3390/cells10082165
Monteiro, N., Smith, E. E., Angstadt, S., Zhang, W., Khademhosseini, A., and Yelick, P. C. (2016). Dental cell sheet biomimetic tooth bud model. Biomaterials 106, 167–179. doi:10.1016/j.biomaterials.2016.08.024
Monteiro, N., Thrivikraman, G., Athirasala, A., Tahayeri, A., França, C. M., Ferracane, J. L., et al. (2018). Photopolymerization of cell-laden gelatin methacryloyl hydrogels using a dental curing light for regenerative dentistry. Dent. Mater. 34 (3), 389–399. doi:10.1016/j.dental.2017.11.020
Mosaddad, S. A., Yazdanian, M., Tebyanian, H., Tahmasebi, E., Yazdanian, A., Seifalian, A., et al. (2020). Fabrication and properties of developed collagen/strontium-doped Bioglass scaffolds for bone tissue engineering. J. Mat. Res. Technol. 9 (6), 14799–14817. doi:10.1016/j.jmrt.2020.10.065
Mourino, V., Cattalini, J. P., and Boccaccini, A. R. (2012). Metallic ions as therapeutic agents in tissue engineering scaffolds: An overview of their biological applications and strategies for new developments. J. R. Soc. Interface 9 (68), 401–419. doi:10.1098/rsif.2011.0611
Mubaraki, M. Q., Moaleem, M. M. A., Alzahrani, A. H., Shariff, M., Alqahtani, S. M., Porwal, A., et al. (2022). Assessment of conventionally and digitally fabricated complete dentures: A comprehensive review. Materials 15 (11), 3868. doi:10.3390/ma15113868
Munir, A., Døskeland, A., Avery, S. J., Fuoco, T., Mohamed-Ahmed, S., Lygre, H., et al. (2019). Efficacy of copolymer scaffolds delivering human demineralised dentine matrix for bone regeneration. J. Tissue Eng. 10, 204173141985270. doi:10.1177/2041731419852703
Murakami, S., Miyaji, H., Nishida, E., Kawamoto, K., Miyata, S., Takita, H., et al. (2017). Dose effects of beta-tricalcium phosphate nanoparticles on biocompatibility and bone conductive ability of three-dimensional collagen scaffolds. Dent. Mat. J. 36, 573–583. doi:10.4012/dmj.2016-295
Murphy, M. K., MacBarb, R. F., Wong, M. E., and Athanasiou, K. A. (2013). Temporomandibular disorders: A review of etiology, clinical management, and tissue engineering strategies. Int. J. Oral Maxillofac. Implants 28 (6), e393–e414. doi:10.11607/jomi.te20
Nadeem, D., Kiamehr, M., Yang, X., and Su, B. (2013). Fabrication and in vitro evaluation of a sponge-like bioactive-glass/gelatin composite scaffold for bone tissue engineering. Mater. Sci. Eng. C 33 (5), 2669–2678. doi:10.1016/j.msec.2013.02.021
Nagasawa, M., Cooper, L., Ogino, Y., Mendonca, D., Liang, R., Yang, S., et al. (2016). Topography influences adherent cell regulation of osteoclastogenesis. J. Dent. Res. 95 (3), 319–326. doi:10.1177/0022034515616760
Nagy, K., Lang, O., Láng, J., Perczel-Kovach, K., Gyulai-Gaal, S., Kádár, K., et al. (2018). A novel hydrogel scaffold for periodontal ligament stem cells. Interv. Med. Appl. Sci. 10 (3), 162–170. doi:10.1556/1646.10.2018.21
Nakamura, S., Ito, T., Okamoto, K., Mima, T., Uchida, K., Siddiqui, Y. D., et al. (2019). Acceleration of bone regeneration of horizontal bone defect in rats using collagen‐binding basic fibroblast growth factor combined with collagen scaffolds. J. Periodontol. 90 (9), 1043–1052. doi:10.1002/jper.18-0674
Nakayama, K. H., Shayan, M., and Huang, N. F. (2019). Engineering biomimetic materials for skeletal muscle repair and regeneration. Adv. Healthc. Mat. 8 (5), 1801168. doi:10.1002/adhm.201801168
Neel, E. A. A., Chrzanowski, W., and Knowles, J. C. (2014). Biological performance of titania containing phosphate-based glasses for bone tissue engineering applications. Mater. Sci. Eng. C 35, 307–313. doi:10.1016/j.msec.2013.10.029
Nemeno-Guanzon, J. G., Lee, S., Berg, J. R., Jo, Y. H., Yeo, J. E., Nam, B. M., et al. (2012). Trends in tissue engineering for blood vessels. J. Biomed. Biotechnol. 2012, 1–14. doi:10.1155/2012/956345
Nery, J. C., Pereira, L. A. V. D., Guimarães, G. F., Scardueli, C. R., França, F. M. G., Spin-Neto, R., et al. (2017). β-TCP/HA with or without enamel matrix proteins for maxillary sinus floor augmentation: A histomorphometric analysis of human biopsies. Int. J. Implant Dent. 3 (1), 18. doi:10.1186/s40729-017-0080-8
Neto, A. S., and Ferreira, J. M. (2018). Synthetic and marine-derived porous scaffolds for bone tissue engineering. Materials 11 (9), 1702. doi:10.3390/ma11091702
Ng, J., Spiller, K., Bernhard, J., and Vunjak-Novakovic, G. (2017). Biomimetic approaches for bone tissue engineering. Tissue Eng. Part B Rev. 23 (5), 480–493. doi:10.1089/ten.teb.2016.0289
Nishida, E., Miyaji, H., Kato, A., Takita, H., Iwanaga, T., Momose, T., et al. (2016). Graphene oxide scaffold accelerates cellular proliferative response and alveolar bone healing of tooth extraction socket. Int. J. Nanomedicine 11, 2265–2277. doi:10.2147/ijn.s104778
Norowski, P. A., Fujiwara, T., Clem, W. C., Adatrow, P. C., Eckstein, E. C., Haggard, W. O., et al. (2015). Novel naturally crosslinked electrospun nanofibrous chitosan mats for guided bone regeneration membranes: Material characterization and cytocompatibility. J. Tissue Eng. Regen. Med. 9 (5), 577–583. doi:10.1002/term.1648
Ogawa, K., Miyaji, H., Kato, A., Kosen, Y., Momose, T., Yoshida, T., et al. (2016). Periodontal tissue engineering by nano beta-tricalcium phosphate scaffold and fibroblast growth factor-2 in one-wall infrabony defects of dogs. J. Periodontal Res. 51 (6), 758–767. doi:10.1111/jre.12352
Oksdath, M., Perrin, S. L., Bardy, C., Hilder, E. F., DeForest, C. A., Arrua, R. D., et al. (2018). Review: Synthetic scaffolds to control the biochemical, mechanical, and geometrical environment of stem cell-derived brain organoids. Apl. Bioeng. 2 (4), 041501. doi:10.1063/1.5045124
Oryan, A., Alidadi, S., Bigham-Sadegh, A., and Moshiri, A. (2018). Healing potentials of polymethylmethacrylate bone cement combined with platelet gel in the critical-sized radial bone defect of rats. PloS one 13 (4), e0194751. doi:10.1371/journal.pone.0194751
Osorio, R., Alfonso-Rodríguez, C. A., Medina-Castillo, A. L., Alaminos, M., and Toledano, M. (2016). Bioactive polymeric nanoparticles for periodontal therapy. PloS one 11 (11), e0166217. doi:10.1371/journal.pone.0166217
Pacifici, A., Laino, L., Gargari, M., Guzzo, F., Luz, A. V., Polimeni, A., et al. (2018). Decellularized hydrogels in bone tissue engineering: A topical review. Int. J. Med. Sci. 15 (5), 492–497. doi:10.7150/ijms.22789
Pajoumshariati, S., Shirali, H., Yavari, S. K., Sheikholeslami, S. N., Lotfi, G., Abbas, F. M., et al. (2018). GBR membrane of novel poly (butylene succinate-co-glycolate) co-polyester co-polymer for periodontal application. Sci. Rep. 8 (1), 7513–7516. doi:10.1038/s41598-018-25952-1
Palasuk, J., Kamocki, K., Hippenmeyer, L., Platt, J. A., Spolnik, K. J., Gregory, R. L., et al. (2014). Bimix antimicrobial scaffolds for regenerative endodontics. J. Endod. 40 (11), 1879–1884. doi:10.1016/j.joen.2014.07.017
Pangesty, A. I., Arahira, T., and Todo, M. (2017). Development and characterization of hybrid tubular structure of PLCL porous scaffold with hMSCs/ECs cell sheet. J. Mat. Sci. Mat. Med. 28 (10), 165. doi:10.1007/s10856-017-5985-5
Park, C. H., Kim, K-H., Lee, Y-M., and Seol, Y-J. (2016). Advanced engineering strategies for periodontal complex regeneration. Materials 9 (1), 57. doi:10.3390/ma9010057
Park, S. A., Lee, H-J., Kim, K-S., Lee, S. J., Lee, J-T., Kim, S-Y., et al. (2018). In vivo evaluation of 3D-printed polycaprolactone scaffold implantation combined with β-TCP powder for alveolar bone augmentation in a beagle defect model. Materials 11 (2), 238. doi:10.3390/ma11020238
Patel, V., Sadiq, M. S., Najeeb, S., Khurshid, Z., Zafar, M. S., and Heboyan, A. (2022). Effects of metformin on the bioactivity and osseointegration of dental implants: A systematic review. J. Taibah Univ. Med. Sci. doi:10.1016/j.jtumed.2022.07.003
Pei, B., Wang, W., Dunne, N., and Li, X. (2019). Applications of carbon nanotubes in bone tissue regeneration and engineering: Superiority, concerns, current advancements, and prospects. Nanomaterials 9 (10), 1501. doi:10.3390/nano9101501
Peng, C., Zheng, J., Chen, D., Zhang, X., Deng, L., Chen, Z., et al. (2018). Response of hPDLSCs on 3D printed PCL/PLGA composite scaffolds in vitro. Mol. Med. Rep. 18 (2), 1335–1344. doi:10.3892/mmr.2018.9076
Perkins, B. L., and Naderi, N. (2016). Suppl-3, M7: Carbon nanostructures in bone tissue engineering. Open Orthop. J. 10, 877–899. doi:10.2174/1874325001610010877
Phull, A-R., Eo, S-H., Abbas, Q., Ahmed, M., and Kim, S. J. (2016). Applications of chondrocyte-based cartilage engineering: An overview. BioMed Res. Int. 2016, 1–17. doi:10.1155/2016/1879837
Posritong, S., Chavez, R. F., Chu, T-M. G., and Bruzzaniti, A. (2019). A Pyk2 inhibitor incorporated into a PEGDA-gelatin hydrogel promotes osteoblast activity and mineral deposition. Biomed. Mat. 14 (2), 025015. doi:10.1088/1748-605x/aafffa
Probst, F. A., Fliefel, R., Burian, E., Probst, M., Eddicks, M., Cornelsen, M., et al. (2020). Bone regeneration of minipig mandibular defect by adipose derived mesenchymal stem cells seeded tri-calcium phosphate-poly (D, L-lactide-co-glycolide) scaffolds. Sci. Rep. 10 (1), 2062–2116. doi:10.1038/s41598-020-59038-8
Puttini, I. D. O., Poli, P. P., Maiorana, C., Vasconcelos, I. R. D., Schmidt, L. E., Colombo, L. T., et al. (2019). Evaluation of osteoconduction of biphasic calcium phosphate ceramic in the calvaria of rats: Microscopic and histometric analysis. J. Funct. Biomater. 10 (1), 7. doi:10.3390/jfb10010007
Puwanun, S., Delaine‐Smith, R. M., Colley, H. E., Yates, J. M., MacNeil, S., and Reilly, G. C. (2018). A simple rocker‐induced mechanical stimulus upregulates mineralization by human osteoprogenitor cells in fibrous scaffolds. J. Tissue Eng. Regen. Med. 12 (2), 370–381. doi:10.1002/term.2462
Qin, H., Yang, Z., Li, L., Yang, X., Liu, J., Chen, X., et al. (2016). A promising scaffold with excellent cytocompatibility and pro-angiogenesis action for dental tissue engineering: Strontium-doped calcium polyphosphate. Dent. Mat. J. 35 (2), 241–249. doi:10.4012/dmj.2015-272
Qin, W., Chen, J-Y., Guo, J., Ma, T., Weir, M. D., Guo, D., et al. (2018). Novel calcium phosphate cement with metformin-loaded chitosan for odontogenic differentiation of human dental pulp cells. Stem cells Int. 2018, 1–10. doi:10.1155/2018/7173481
Qiu, G., Wang, P., Li, G., Shi, Z., Weir, M. D., Sun, J., et al. (2016). Minipig-BMSCs combined with a self-setting calcium phosphate paste for bone tissue engineering. Mol. Biotechnol. 58 (11), 748–756. doi:10.1007/s12033-016-9974-6
Qu, T., Jing, J., Jiang, Y., Taylor, R. J., Feng, J. Q., Geiger, B., et al. (2014). Magnesium-containing nanostructured hybrid scaffolds for enhanced dentin regeneration. Tissue Eng. Part A 20 (17-18), 2422–2433. doi:10.1089/ten.tea.2013.0741
Quintiliano, K., Crestani, T., Silveira, D., Helfer, V. E., Rosa, A., Balbueno, E., et al. (2016). Neural differentiation of mesenchymal stem cells on scaffolds for nerve tissue engineering applications. Cell. Reprogr. 18 (6), 369–381. doi:10.1089/cell.2016.0024
Rachman, A., Rantam, F. A., Bachtiar, I., Fatchiyah, F., Hakim, L., Putri, I. L., et al. (2018). Biocompatibility of yttria-tetragonal zirconia polycrystal seeded with human adipose derived mesenchymal stem cell. Acta Inf. Med. 26 (4), 249. doi:10.5455/aim.2018.26.249-253
Ramzan, M., Karobari, M. I., Heboyan, A., Mohamed, R. N., Mustafa, M., Basheer, S. N., et al. (2022). Synthesis of silver nanoparticles from extracts of wild ginger (zingiber zerumbet) with antibacterial activity against selective multidrug resistant oral bacteria. Mol. (Basel, Switz. 27 (6), 2007. doi:10.3390/molecules27062007
Rapino, M., Di Valerio, V., Zara, S., Gallorini, M., Marconi, G. D., Sancilio, S., et al. (2019). Chitlac-coated thermosets enhance osteogenesis and angiogenesis in a co-culture of dental pulp stem cells and endothelial cells. Nanomaterials 9 (7), 928. doi:10.3390/nano9070928
Raposo-Amaral, C. E., Bueno, D. F., Almeida, A. B., Jorgetti, V., Costa, C. C., Gouveia, C. H., et al. (2014). Is bone transplantation the gold standard for repair of alveolar bone defects? J. Tissue Eng. 5, 204173141351935. doi:10.1177/2041731413519352
Raspini, G., Wolff, J., Helminen, M., Raspini, G., Raspini, M., and Sándor, G. K. (2018). Dental stem cells harvested from third molars combined with bioactive glass can induce signs of bone formation in vitro. J. Oral Maxillofac. Res. 9 (1), e2. doi:10.5037/jomr.2018.9102
Ravindran, S., and George, A. (2015). Dentin matrix proteins in bone tissue engineering. Adv. Exp. Med. Biol. 881, 129–142. doi:10.1007/978-3-319-22345-2_8
Razavi, S. M., Rismanchian, M., Jafari-Pozve, N., and Nosouhian, S. (2017). Comparing the efficacy of three different nano-scale bone substitutes: In vivo study. Adv. Biomed. Res. 6, 64. doi:10.4103/2277-9175.192627
Reardon, P., Konwarh, R., Knowles, J. C., and Mandal, B. B. (2017). Mimicking hierarchical complexity of the osteochondral interface using electrospun silk-bioactive glass composites. ACS Appl. Mat. Interfaces 9 (9), 8000–8013. doi:10.1021/acsami.6b16590
Reddy, M. S. B., Ponnamma, D., Choudhary, R., and Sadasivuni, K. K. (2021). A comparative review of natural and synthetic biopolymer composite scaffolds. Polym. (Basel) 13 (7), 1105. doi:10.3390/polym13071105
Requicha, J. F., Viegas, C. A., Muñoz, F., Azevedo, J. M., Leonor, I. B., Reis, R. L., et al. (2014). A tissue engineering approach for periodontal regeneration based on a biodegradable double-layer scaffold and adipose-derived stem cells. Tissue Eng. Part A 20 (17-18), 2483–2492. doi:10.1089/ten.tea.2013.0360
Rezaeeyazdi, M., Colombani, T., Memic, A., and Bencherif, S. A. (2018). Injectable hyaluronic acid-co-gelatin cryogels for tissue-engineering applications. Materials 11 (8), 1374. doi:10.3390/ma11081374
Rider, P., Kačarević, Ž. P., Alkildani, S., Retnasingh, S., Schnettler, R., and Barbeck, M. (2018). Additive manufacturing for guided bone regeneration: A perspective for alveolar ridge augmentation. Int. J. Mol. Sci. 19 (11), 3308. doi:10.3390/ijms19113308
Rider, P. M., Brook, I., Smith, P. J., and Miller, C. A. (2018). Reactive inkjet printing of regenerated silk fibroin films for use as dental barrier membranes. Micromachines 9 (2), 46. doi:10.3390/mi9020046
Roi, A., Ardelean, L. C., Roi, C. I., Boia, E-R., Boia, S., and Rusu, L-C. (2019). Oral bone tissue engineering: Advanced biomaterials for cell adhesion, proliferation and differentiation. Materials 12 (14), 2296. doi:10.3390/ma12142296
Rokaya, D., Kongkiatkamon, S., Heboyan, A., Dam, V. V., Amornvit, P., Khurshid, Z., et al. (2022). “3D-Printed biomaterials in biomedical application,” in Functional biomaterials: Drug delivery and biomedical applications. Editors S. Jana, and S. Jana (Singapore: Springer), 319–339.
Rokaya, D., Srimaneepong, V., Qin, J., Thunyakitpisal, P., and Siraleartmukul, K. (2019). Surface adhesion properties and cytotoxicity of graphene oxide coatings and graphene oxide/silver nanocomposite coatings on biomedical NiTi alloy. Sci. Adv. Mat. 11 (10), 1474–1487. doi:10.1166/sam.2019.3536
Rokaya, D., Srimaneepong, V., Sapkota, J., Qin, J., Siraleartmukul, K., and Siriwongrungson, V. (2018). Polymeric materials and films in dentistry: An overview. J. Adv. Res. 14, 25–34. doi:10.1016/j.jare.2018.05.001
Rokaya, D., Srimaneepong, V., Thunyakitpisal, P., Qin, J., Rosa, V., and Sapkota, J. (2021). “Potential applications of graphene-based nanomaterials in biomedical, dental, and implant applications,” in Advances in dental implantology using nanomaterials and allied technology applications. Editors R. S. Chaughule, and R. Dashaputra (Cham: Springer International Publishing), 77–105.
Rokaya, D., Suttagul, K., Joshi, S., Bhattarai, B. P., Shah, P. K., and Dixit, S. (2018). An epidemiological study on the prevalence of temporomandibular disorder and associated history and problems in Nepalese subjects. J. Dent. Anesth. Pain Med. 18 (1), 27–33. doi:10.17245/jdapm.2018.18.1.27
Ruan, Q., Liberman, D., Bapat, R., Chandrababu, K. B., Phark, J-H., and Moradian-Oldak, J. (2016). Efficacy of amelogenin-chitosan hydrogel in biomimetic repair of human enamel in pH-cycling systems. J. Biomed. Eng. Inf. 2 (1), 119. doi:10.5430/jbei.v2n1p119
Saberi, A., Jabbari, F., Zarrintaj, P., Saeb, M. R., and Mozafari, M. (2019). Electrically conductive materials: Opportunities and challenges in tissue engineering. Biomolecules 9 (9), 448. doi:10.3390/biom9090448
Salamanca, E., Hsu, C-C., Huang, H-M., Teng, N-C., Lin, C-T., Pan, Y-H., et al. (2018). Bone regeneration using a porcine bone substitute collagen composite in vitro and in vivo. Sci. Rep. 8 (1), 984–988. doi:10.1038/s41598-018-19629-y
Salamanca, E., Pan, Y-H., Tsai, A. I., Lin, P-Y., Lin, C-K., Huang, H-M., et al. (2017). Enhancement of osteoblastic-like cell activity by glow discharge plasma surface modified hydroxyapatite/β-tricalcium phosphate bone substitute. Materials 10 (12), 1347. doi:10.3390/ma10121347
Samorezov, J. E., and Alsberg, E. (2015). Spatial regulation of controlled bioactive factor delivery for bone tissue engineering. Adv. drug Deliv. Rev. 84, 45–67. doi:10.1016/j.addr.2014.11.018
Santo, V. E., Gomes, M. E., Mano, J. F., and Reis, R. L. (2013). Controlled release strategies for bone, cartilage, and osteochondral engineering—Part II: Challenges on the evolution from single to multiple bioactive factor delivery. Tissue Eng. Part B Rev. 19 (4), 327–352. doi:10.1089/ten.teb.2012.0727
Sargolzaei-Aval, F., Saberi, E. A., Arab, M. R., Sargolzaei, N., Zare, E., Shahraki, H., et al. (2020). Reconstruction of mandibular defects using synthetic octacalcium phosphate combined with bone matrix gelatin in rat model. Dent. Res. J. 17 (1), 10. doi:10.4103/1735-3327.276228
Sasaki, T., Niizuma, K., Kanoke, A., Matsui, K., Ogita, S., Rashad, S., et al. (2020). Octacalcium phosphate collagen composite (OCP/Col) enhance bone regeneration in a rat model of skull defect with dural defect. Heliyon 6 (2), e03347. doi:10.1016/j.heliyon.2020.e03347
Sergey, V. D. (2018). Calcium orthophosphate (CaPO4) scaffolds for bone tissue engineering applications. J. Biotechnol. Biomed. Sci. 1 (3), 25–93. doi:10.14302/issn.2576-6694.jbbs-18-2143
Shafieian, R., Matin, M. M., Rahpeyma, A., Fazel, A., Sedigh, H. S., Sadr-Nabavi, A., et al. (2017). The effect of platelet-rich plasma on human mesenchymal stem cell-induced bone regeneration of canine alveolar defects with calcium phosphate-based scaffolds. Iran. J. Basic Med. Sci. 20 (10), 1131–1140. doi:10.22038/IJBMS.2017.9447
Shahsavari-Pour, S., Aliabadi, E., Latifi, M., Zareifard, N., Namavar, M. R., and Talaei-Khozani, T. (2018). Evaluation of the possible synergic regenerative effects of platelet-rich plasma and hydroxyapatite/zirconia in the rabbit mandible defect model. Iran. J. Med. Sci. 43 (6), 633–644.
Sharma, S., Sapkota, D., Xue, Y., Rajthala, S., Yassin, M. A., Finne-Wistrand, A., et al. (2018). Delivery of VEGFA in bone marrow stromal cells seeded in copolymer scaffold enhances angiogenesis, but is inadequate for osteogenesis as compared with the dual delivery of VEGFA and BMP2 in a subcutaneous mouse model. Stem Cell Res. Ther. 9 (1), 23. doi:10.1186/s13287-018-0778-4
Sharma, S., Sapkota, D., Xue, Y., Sun, Y., Finne-Wistrand, A., Bruland, O., et al. (2016). Adenoviral mediated expression of BMP2 by bone marrow stromal cells cultured in 3D copolymer scaffolds enhances bone formation. PloS one 11 (1), e0147507. doi:10.1371/journal.pone.0147507
Sheikh, Z., Najeeb, S., Khurshid, Z., Verma, V., Rashid, H., and Glogauer, M. (2015). Biodegradable materials for bone repair and tissue engineering applications. Materials 8 (9), 5744–5794. doi:10.3390/ma8095273
Shen, Y-F., Huang, T-H., Ng, H-Y., Fang, H-Y., and Hsu, T-T. (2018). Mineral trioxide aggregate mixed with 5-aminolevulinic acid for the photodynamic antimicrobial strategy in hard tissue regeneration. Materials 11 (9), 1734. doi:10.3390/ma11091734
Shetty, H., Shetty, S., Kakade, A., Shetty, A., Karobari, M. I., Pawar, A. M., et al. (2021). Three-dimensional semi-automated volumetric assessment of the pulp space of teeth following regenerative dental procedures. Sci. Rep. 11 (1), 21914–22011. doi:10.1038/s41598-021-01489-8
Shi, H., Li, X., Yang, J., Zhao, Y., Xue, C., Wang, Y., et al. (2019). Bone marrow-derived neural crest precursors improve nerve defect repair partially through secreted trophic factors. Stem Cell Res. Ther. 10 (1), 397–415. doi:10.1186/s13287-019-1517-1
Shi, M., Kretlow, J. D., Spicer, P. P., Tabata, Y., Demian, N., Wong, M. E., et al. (2011). Antibiotic-releasing porous polymethylmethacrylate/gelatin/antibiotic constructs for craniofacial tissue engineering. J. Control. release 152 (1), 196–205. doi:10.1016/j.jconrel.2011.01.029
Shim, J-H., Won, J-Y., Park, J-H., Bae, J-H., Ahn, G., Kim, C-H., et al. (2017). Effects of 3D-printed polycaprolactone/β-tricalcium phosphate membranes on guided bone regeneration. Int. J. Mol. Sci. 18 (5), 899. doi:10.3390/ijms18050899
Siaili, M., Chatzopoulou, D., and Gillam, D. (2018). An overview of periodontal regenerative procedures for the general dental practitioner. Saudi Dent. J. 30 (1), 26–37. doi:10.1016/j.sdentj.2017.11.001
Simsa, R., Padma, A. M., Heher, P., Hellström, M., Teuschl, A., Jenndahl, L., et al. (2018). Systematic in vitro comparison of decellularization protocols for blood vessels. PloS one 13 (12), e0209269. doi:10.1371/journal.pone.0209269
Skallevold, H. E., Rokaya, D., Khurshid, Z., and Zafar, M. S. (2019). Bioactive glass applications in dentistry. Int. J. Mol. Sci. 20 (23), 5960. doi:10.3390/ijms20235960
Smith, B. T., Lu, A., Watson, E., Santoro, M., Melchiorri, A. J., Grosfeld, E. C., et al. (2018). Incorporation of fast dissolving glucose porogens and poly (lactic-co-glycolic acid) microparticles within calcium phosphate cements for bone tissue regeneration. Acta biomater. 78, 341–350. doi:10.1016/j.actbio.2018.07.054
Smith, E., Angstadt, S., Monteiro, N., Zhang, W., Khademhosseini, A., and Yelick, P. (2018). Bioengineered tooth buds exhibit features of natural tooth buds. J. Dent. Res. 97 (10), 1144–1151. doi:10.1177/0022034518779075
Smith, E. E., and Yelick, P. C. (2019). Bioengineering tooth bud constructs using GelMA hydrogel. Methods Mol. Biol. 1922, 139–150. doi:10.1007/978-1-4939-9012-2_14
Soares, D. G., Zhang, Z., Mohamed, F., Eyster, T. W., de Souza Costa, C. A., and Ma, P. X. (2018). Simvastatin and nanofibrous poly (l-lactic acid) scaffolds to promote the odontogenic potential of dental pulp cells in an inflammatory environment. Acta biomater. 68, 190–203. doi:10.1016/j.actbio.2017.12.037
Song, H-H. G., Rumma, R. T., Ozaki, C. K., Edelman, E. R., and Chen, C. S. (2018). Vascular tissue engineering: Progress, challenges, and clinical promise. Cell stem Cell 22 (3), 340–354. doi:10.1016/j.stem.2018.02.009
Song, Y-G., and Cho, I-H. (2014). Characteristics and osteogenic effect of zirconia porous scaffold coated with β-TCP/HA. J. Adv. Prosthodont. 6 (4), 285–294. doi:10.4047/jap.2014.6.4.285
Sood, S., Gupta, S., and Mahendra, A. (2012). Gene therapy with growth factors for periodontal tissue engineering–A review. Med. Oral Patol. Oral Cir. Bucal 17 (2), e301–e310. doi:10.4317/medoral.17472
Soudi, A., Yazdanian, M., Ranjbar, R., Tebyanian, H., Yazdanian, A., Tahmasebi, E., et al. (2021). Role and application of stem cells in dental regeneration: A comprehensive overview. EXCLI J. 20, 454–489. doi:10.17179/excli2021-3335
Soufdoost, R. S., Mosaddad, S. A., Salari, Y., Yazdanian, M., Tebyanian, H., Tahmasebi, E., et al. (2020). Surgical suture assembled with tadalafil/polycaprolactone drug-delivery for vascular stimulation around wound: Validated in a preclinical model. Biointerface Res. Appl. Chem. 10 (5), 6317–6327.
Soufdoost, R. S., Yazdanian, M., Tahmasebi, E., Yazdanian, A., Tebyanian, H., Karami, A., et al. (2019). In vitro and in vivo evaluation of novel tadalafil/β-TCP/collagen scaffold for bone regeneration: A rabbit critical-size calvarial defect study. Biocybern. Biomed. Eng. 39 (3), 789–796. doi:10.1016/j.bbe.2019.07.003
Srimaneepong, V., Heboyan, A., Syed, A. U. Y., Trinh, H. A., Amornvit, P., and Rokaya, D. (2021). Recent advances in myoelectric control for finger prostheses for multiple finger loss. Appl. Sci. 11 (10), 4464. doi:10.3390/app11104464
Srimaneepong, V., Heboyan, A., Zafar, M. S., Khurshid, Z., Marya, A., Fernandes, G. V., et al. (2022). Fixed prosthetic restorations and periodontal health: A narrative review. J. Funct. Biomater. 13 (1), 15. doi:10.3390/jfb13010015
Srimaneepong, V., Skallevold, H. E., Khurshid, Z., Zafar, M. S., Rokaya, D., and Sapkota, J. (2022). Graphene for antimicrobial and coating application. Int. J. Mol. Sci. 23 (1), 499. doi:10.3390/ijms23010499
Sriranganathan, D., Kanwal, N., Hing, K. A., and Hill, R. G. (2016). Strontium substituted bioactive glasses for tissue engineered scaffolds: The importance of octacalcium phosphate. J. Mat. Sci. Mat. Med. 27 (2), 39. doi:10.1007/s10856-015-5653-6
Stratton, S., Shelke, N. B., Hoshino, K., Rudraiah, S., and Kumbar, S. G. (2016). Bioactive polymeric scaffolds for tissue engineering. Bioact. Mater. 1 (2), 93–108. doi:10.1016/j.bioactmat.2016.11.001
Su, C-J., Tu, M-G., Wei, L-J., Hsu, T-T., Kao, C-T., Chen, T-H., et al. (2017). Calcium silicate/chitosan-coated electrospun poly (lactic acid) fibers for bone tissue engineering. Materials 10 (5), 501. doi:10.3390/ma10050501
Su, F., Liu, S-S., Ma, J-L., Wang, D-S., Ling-Ling, E., and Liu, H-C. (2015). Enhancement of periodontal tissue regeneration by transplantation of osteoprotegerin-engineered periodontal ligament stem cells. Stem Cell Res. Ther. 6 (1), 22. doi:10.1186/s13287-015-0023-3
Sun, H., and Yang, H-L. (2015). Calcium phosphate scaffolds combined with bone morphogenetic proteins or mesenchymal stem cells in bone tissue engineering. Chin. Med. J. 128 (8), 1121–1127. doi:10.4103/0366-6999.155121
Sun, X., Xu, C., Wu, G., Ye, Q., and Wang, C. (2017). Poly (lactic-co-glycolic acid): Applications and future prospects for periodontal tissue regeneration. Polymers 9 (6), 189. doi:10.3390/polym9060189
Sunandhakumari, V. J., Vidhyadharan, A. K., Alim, A., Kumar, D., Ravindran, J., Krishna, A., et al. (2018). Fabrication and in vitro characterization of bioactive glass/nano hydroxyapatite reinforced electrospun poly (ε-caprolactone) composite membranes for guided tissue regeneration. Bioengineering 5 (3), 54. doi:10.3390/bioengineering5030054
Suzuki, A., and Iwata, J. (2016). Mouse genetic models for temporomandibular joint development and disorders. Oral Dis. 22 (1), 33–38. doi:10.1111/odi.12353
Syed, S., Yassin, S. M., Almalki, A. Y., Ali, S. A. A., Alqarni, A. M. M., Moadi, Y. M., et al. (2022). Structural changes in primary teeth of diabetic children: Composition and ultrastructure analysis. Children 9 (3), 317. doi:10.3390/children9030317
Takahata, M., Awad, H. A., O’Keefe, R. J., Bukata, S. V., and Schwarz, E. M. (2012). Endogenous tissue engineering: PTH therapy for skeletal repair. Cell Tissue Res. 347 (3), 545–552. doi:10.1007/s00441-011-1188-4
Tallarico, M., Ceruso, F. M., Muzzi, L., Meloni, S. M., Kim, Y-J., Gargari, M., et al. (2019). Effect of simultaneous immediate implant placement and guided bone reconstruction with ultra-fine titanium mesh membranes on radiographic and clinical parameters after 18 Months of loading. Materials 12 (10), 1710. doi:10.3390/ma12101710
Talley, A. D., Boller, L. A., Kalpakci, K. N., Shimko, D. A., Cochran, D. L., and Guelcher, S. A. (2018). Injectable, compression‐resistant polymer/ceramic composite bone grafts promote lateral ridge augmentation without protective mesh in a canine model. Clin. Oral Implants Res. 29 (6), 592–602. doi:10.1111/clr.13257
Tan, A. R., and Hung, C. T. (2017). Concise review: Mesenchymal stem cells for functional cartilage tissue engineering: Taking cues from chondrocyte‐based constructs. Stem cells Transl. Med. 6 (4), 1295–1303. doi:10.1002/sctm.16-0271
Tang, M., Chen, W., Liu, J., Weir, M. D., Cheng, L., and Xu, H. H. (2014). Human induced pluripotent stem cell-derived mesenchymal stem cell seeding on calcium phosphate scaffold for bone regeneration. Tissue Eng. Part A 20 (7-8), 1295–1305. doi:10.1089/ten.tea.2013.0211
Tanweer, N., Qazi, F. U., Das, G., Bilgrami, A., Basha, S., Ahmed, N., et al. (2022). Effect of erosive agents on surface characteristics of nano-fluorapatite ceramic: An iIn-vVitro study. Mol. (Basel, Switz. 27 (15), 4691. doi:10.3390/molecules27154691
Tatullo, M., Spagnuolo, G., Codispoti, B., Zamparini, F., Zhang, A., Esposti, M. D., et al. (2019). Pla-based mineral-doped scaffolds seeded with human periapical cyst-derived mscs: A promising tool for regenerative healing in dentistry. Materials 12 (4), 597. doi:10.3390/ma12040597
Thein-Han, W., Weir, M. D., Simon, C. G., and Xu, H. H. (2013). Non-rigid calcium phosphate cement containing hydrogel microbeads and absorbable fibres seeded with umbilical cord stem cells for bone engineering. J. Tissue Eng. Regen. Med. 7 (10), 777–787. doi:10.1002/term.1466
Thein-Han, W., and Xu, H. H. (2013). Prevascularization of a gas-foaming macroporous calcium phosphate cement scaffold via coculture of human umbilical vein endothelial cells and osteoblasts. Tissue Eng. Part A 19 (15-16), 1675–1685. doi:10.1089/ten.tea.2012.0631
Thickett, S. C., Hamilton, E., Yogeswaran, G., Zetterlund, P. B., Farrugia, B. L., and Lord, M. S. (2019). Enhanced osteogenic differentiation of human fetal cartilage rudiment cells on graphene oxide-PLGA hybrid microparticles. J. Funct. Biomater. 10 (3), 33. doi:10.3390/jfb10030033
Thompson, K. D., Weiss-Bilka, H. E., McGough, E. B., and Ravosa, M. J. (2017). Bone up: Craniomandibular development and hard-tissue biomineralization in neonate mice. Zoology 124, 51–60. doi:10.1016/j.zool.2017.01.002
Thorpe, A. A., Freeman, C., Farthing, P., Callaghan, J., Hatton, P. V., Brook, I. M., et al. (2018). In vivo safety and efficacy testing of a thermally triggered injectable hydrogel scaffold for bone regeneration and augmentation in a rat model. Oncotarget 9 (26), 18277–18295. doi:10.18632/oncotarget.24813
Torres-Lagares, D., Castellanos-Cosano, L., Serrera-Figallo, M. Á., García-García, F. J., López-Santos, C., Barranco, A., et al. (2017). In vitro and in vivo study of poly (lactic–co–glycolic)(plga) membranes treated with oxygen plasma and coated with nanostructured hydroxyapatite ultrathin films for guided bone regeneration processes. Polymers 9 (9), 410. doi:10.3390/polym9090410
Traore, M. A., and George, S. C. (2017). Tissue engineering the vascular tree. Tissue Eng. Part B Rev. 23 (6), 505–514. doi:10.1089/ten.teb.2017.0010
Tsai, C-H., Hung, C-H., Kuo, C-N., Chen, C-Y., Peng, Y-N., and Shie, M-Y. (2019). Improved bioactivity of 3D printed porous titanium alloy scaffold with chitosan/magnesium-calcium silicate composite for orthopaedic applications. Materials 12 (2), 203. doi:10.3390/ma12020203
Tsukioka, T., Hiratsuka, T., Nakamura, M., Watanabe, T., Kitamura, Y., Isobe, K., et al. (2019). An on‐site preparable, novel bone‐grafting complex consisting of human platelet‐rich fibrin and porous particles made of a recombinant collagen‐like protein. J. Biomed. Mat. Res. 107 (5), 1420–1430. doi:10.1002/jbm.b.34234
Udomluck, N., Koh, W-G., Lim, D-J., and Park, H. (2020). Recent developments in nanofiber fabrication and modification for bone tissue engineering. Int. J. Mol. Sci. 21 (1), 99. doi:10.3390/ijms21010099
Undt, G., Jahl, M., Pohl, S., Marlovits, S., Moser, D., Yoon, H-H., et al. (2018). Matrix-associated chondrocyte transplantation for reconstruction of articulating surfaces in the temporomandibular joint: A pilot study covering medium-and long-term outcomes of 6 patients. Oral Surg. oral Med. oral pathology oral radiology 126 (2), 117–128. doi:10.1016/j.oooo.2018.02.017
Urruela-Barrios, R., Ramírez-Cedillo, E., Díaz de León, A., Alvarez, A. J., and Ortega-Lara, W. (2019). Alginate/gelatin hydrogels reinforced with TiO2 and β-TCP fabricated by microextrusion-based printing for tissue regeneration. Polymers 11 (3), 457. doi:10.3390/polym11030457
Vaca-González, J. J., Guevara, J. M., Moncayo, M. A., Castro-Abril, H., Hata, Y., and Garzón-Alvarado, D. A. (2019). Biophysical stimuli: A review of electrical and mechanical stimulation in hyaline cartilage. Cartilage 10 (2), 157–172. doi:10.1177/1947603517730637
Van Bellinghen, X., Idoux-Gillet, Y., Pugliano, M., Strub, M., Bornert, F., Clauss, F., et al. (2018). Temporomandibular joint regenerative medicine. Int. J. Mol. Sci. 19 (2), 446. doi:10.3390/ijms19020446
van der Schaft, D. W., van Spreeuwel, A. C., Boonen, K. J., Langelaan, M. L., Bouten, C. V., and Baaijens, F. P. (2013). Engineering skeletal muscle tissues from murine myoblast progenitor cells and application of electrical stimulation. J. Vis. Exp. 73, e4267. doi:10.3791/4267
van Manen, E. H., Zhang, W., Walboomers, X. F., Vazquez, B., Yang, F., Ji, W., et al. (2014). The influence of electrospun fibre scaffold orientation and nano-hydroxyapatite content on the development of tooth bud stem cells in vitro. Odontology 102 (1), 14–21. doi:10.1007/s10266-012-0087-9
Vardhan, P. K., Paramashivaiah, R., Prabhuji, M. L. V., Bhavikatti, S. K., Basha, S., Arora, S., et al. (2022). The effect of photodynamic therapy on the early outcome of implants placed on patients with periodontitis. Photonics 9 (7), 480. doi:10.3390/photonics9070480
Vega, S. L., Kwon, M. Y., and Burdick, J. A. (2017). Recent advances in hydrogels for cartilage tissue engineering. Eur. Cell. Mat. 33, 59–75. doi:10.22203/ecm.v033a05
Vidovic Zdrilic, I., de Azevedo Queiroz, I., Matthews, B., Gomes‐Filho, J., Mina, M., and Kalajzic, I. (2017). Mineral trioxide aggregate improves healing response of periodontal tissue to injury in mice. J. Periodontal Res. 52 (6), 1058–1067. doi:10.1111/jre.12478
Vining, K. H., Scherba, J. C., Bever, A. M., Alexander, M. R., Celiz, A. D., and Mooney, D. J. (2018). Synthetic light‐curable polymeric materials provide a supportive niche for dental pulp stem cells. Adv. Mat. 30 (4), 1704486. doi:10.1002/adma.201704486
Wang, C-Z., Wang, Y-H., Lin, C-W., Lee, T-C., Fu, Y-C., Ho, M-L., et al. (2018). Combination of a bioceramic scaffold and simvastatin nanoparticles as a synthetic alternative to autologous bone grafting. Int. J. Mol. Sci. 19 (12), 4099. doi:10.3390/ijms19124099
Wang, L., Wang, P., Weir, M. D., Reynolds, M. A., Zhao, L., and Xu, H. H. (2016). Hydrogel fibers encapsulating human stem cells in an injectable calcium phosphate scaffold for bone tissue engineering. Biomed. Mat. 11 (6), 065008. doi:10.1088/1748-6041/11/6/065008
Wang, M. M., Flores, R. L., Witek, L., Torroni, A., Ibrahim, A., Wang, Z., et al. (2019). Dipyridamole-loaded 3D-printed bioceramic scaffolds stimulate pediatric bone regeneration in vivo without disruption of craniofacial growth through facial maturity. Sci. Rep. 9 (1), 18439–18515. doi:10.1038/s41598-019-54726-6
Wang, P., Liu, X., Zhao, L., Weir, M. D., Sun, J., Chen, W., et al. (2015). Bone tissue engineering via human induced pluripotent, umbilical cord and bone marrow mesenchymal stem cells in rat cranium. Acta biomater. 18, 236–248. doi:10.1016/j.actbio.2015.02.011
Wang, P., Song, Y., Weir, M. D., Sun, J., Zhao, L., Simon, C. G., et al. (2016). A self-setting iPSMSC-alginate-calcium phosphate paste for bone tissue engineering. Dent. Mater. 32 (2), 252–263. doi:10.1016/j.dental.2015.11.019
Wang, P., Wang, W., Geng, T., Liu, Y., Zhu, S., Liu, Z., et al. (2019). EphrinB2 regulates osteogenic differentiation of periodontal ligament stem cells and alveolar bone defect regeneration in beagles. J. Tissue Eng. 10, 204173141989436. doi:10.1177/2041731419894361
Wang, P., Zhao, L., Liu, J., Weir, M. D., Zhou, X., and Xu, H. H. (2014). Bone tissue engineering via nanostructured calcium phosphate biomaterials and stem cells. Bone Res. 2, 14017. doi:10.1038/boneres.2014.17
Wang, Q. G., Wimpenny, I., Dey, R. E., Zhong, X., Youle, P. J., Downes, S., et al. (2018). The unique calcium chelation property of poly (vinyl phosphonic acid‐co‐acrylic acid) and effects on osteogenesis in vitro. J. Biomed. Mat. Res. A 106 (1), 168–179. doi:10.1002/jbm.a.36223
Wang, S., Bao, Y., Guan, Y., Zhang, C., Liu, H., Yang, X., et al. (2018). Strain distribution of repaired articular cartilage defects by tissue engineering under compression loading. J. Orthop. Surg. Res. 13 (1), 19. doi:10.1186/s13018-018-0726-0
Wang, W., Dang, M., Zhang, Z., Hu, J., Eyster, T. W., Ni, L., et al. (2016). Dentin regeneration by stem cells of apical papilla on injectable nanofibrous microspheres and stimulated by controlled BMP-2 release. Acta biomater. 36, 63–72. doi:10.1016/j.actbio.2016.03.015
Wang, X., Kou, X., Mao, J., Gan, Y., and Zhou, Y. (2012). Sustained inflammation induces degeneration of the temporomandibular joint. J. Dent. Res. 91 (5), 499–505. doi:10.1177/0022034512441946
Wang, X., Schröder, H. C., Feng, Q., Draenert, F., and Müller, W. E. (2013). The deep-sea natural products, biogenic polyphosphate (Bio-PolyP) and biogenic silica (Bio-Silica), as biomimetic scaffolds for bone tissue engineering: Fabrication of a morphogenetically-active polymer. Mar. drugs 11 (3), 718–746. doi:10.3390/md11030718
Wang, X., Xing, H., Zhang, G., Wu, X., Zou, X., Feng, L., et al. (2016). Restoration of a critical mandibular bone defect using human alveolar bone-derived stem cells and porous nano-HA/collagen/PLA scaffold. Stem Cells Int. 2016, 1–13. doi:10.1155/2016/8741641
Wang, Y., Yin, P., Bian, G-L., Huang, H-Y., Shen, H., Yang, J-J., et al. (2017). The combination of stem cells and tissue engineering: An advanced strategy for blood vessels regeneration and vascular disease treatment. Stem Cell Res. Ther. 8 (1), 194. doi:10.1186/s13287-017-0642-y
Willard, V. P., Arzi, B., and Athanasiou, K. A. (2012). The attachments of the temporomandibular joint disc: A biochemical and histological investigation. Archives oral Biol. 57 (6), 599–606. doi:10.1016/j.archoralbio.2011.10.004
Witt, R., Weigand, A., Boos, A., Cai, A., Dippold, D., Boccaccini, A., et al. (2017). Mesenchymal stem cells and myoblast differentiation under HGF and IGF-1 stimulation for 3D skeletal muscle tissue engineering. BMC Cell Biol. 18 (1), 15. doi:10.1186/s12860-017-0131-2
Wongsupa, N., Nuntanaranont, T., Kamolmattayakul, S., and Thuaksuban, N. (2017). Biological characteristic effects of human dental pulp stem cells on poly-ε-caprolactone-biphasic calcium phosphate fabricated scaffolds using modified melt stretching and multilayer deposition. J. Mat. Sci. Mat. Med. 28 (2), 25. doi:10.1007/s10856-016-5833-z
Wu, D., Wang, Z., Wang, J., Geng, Y., Zhang, Z., Li, Y., et al. (2018). Development of a micro-tissue-mediated injectable bone tissue engineering strategy for large segmental bone defect treatment. Stem Cell Res. Ther. 9 (1), 331. doi:10.1186/s13287-018-1064-1
Wu, Y., Gong, Z., Li, J., Meng, Q., Fang, W., and Long, X. (2014). The pilot study of fibrin with temporomandibular joint derived synovial stem cells in repairing TMJ disc perforation. BioMed Res. Int. 2014, 1–10. doi:10.1155/2014/454021
Xia, Y., Chen, H., Zhang, F., Bao, C., Weir, M. D., Reynolds, M. A., et al. (2018). Gold nanoparticles in injectable calcium phosphate cement enhance osteogenic differentiation of human dental pulp stem cells. Nanomedicine Nanotechnol. Biol. Med. 14 (1), 35–45. doi:10.1016/j.nano.2017.08.014
Xiao, Y. (2014). Bone tissue engineering for dentistry and orthopaedics. BioMed Res. Int. 2014, 1–2. doi:10.1155/2014/241067
Xiao, Y., Friis, E. A., Gehrke, S. H., and Detamore, M. S. (2013). Mechanical testing of hydrogels in cartilage tissue engineering: Beyond the compressive modulus. Tissue Eng. Part B Rev. 19 (5), 403–412. doi:10.1089/ten.teb.2012.0461
Xie, H., Cao, T., Franco-Obregón, A., and Rosa, V. (2019). Graphene-induced osteogenic differentiation is mediated by the integrin/FAK axis. Int. J. Mol. Sci. 20 (3), 574. doi:10.3390/ijms20030574
Xing, Z., Xue, Y., Finne‐Wistrand, A., Yang, Z. Q., and Mustafa, K. (2013). Copolymer cell/scaffold constructs for bone tissue engineering: Co‐culture of low ratios of human endothelial and osteoblast‐like cells in a dynamic culture system. J. Biomed. Mat. Res. A 101 (4), 1113–1120. doi:10.1002/jbm.a.34414
Xu, Q., Furuhashi, A., Zhang, Q., Jiang, C., Chang, T-H., and Le, A. (2017). Induction of salivary gland–like cells from dental follicle epithelial cells. J. Dent. Res. 96 (9), 1035–1043. doi:10.1177/0022034517711146
Xu, Q., Shanti, R. M., Zhang, Q., Cannady, S. B., O'Malley, B. W., and Le, A. D. (2017). A gingiva-derived mesenchymal stem cell-laden porcine small intestinal submucosa extracellular matrix construct promotes Myomucosal regeneration of the tongue. Tissue Eng. Part A 23 (7-8), 301–312. doi:10.1089/ten.tea.2016.0342
Xue, K., Zhang, X., Gao, Z., Xia, W., Qi, L., and Liu, K. (2019). Cartilage progenitor cells combined with PHBV in cartilage tissue engineering. J. Transl. Med. 17 (1), 104. doi:10.1186/s12967-019-1855-x
Xue, Y., Hong, X., Gao, J., Shen, R., and Ye, Z. (2019). Preparation and biological characterization of the mixture of poly (lactic-co-glycolic acid)/chitosan/ag nanoparticles for periodontal tissue engineering. Int. J. Nanomedicine 14, 483–498. doi:10.2147/ijn.s184396
Yadalam, P. K., Sivasankari, T., Rengaraj, S., Mugri, M. H., Sayed, M., Khan, S. S., et al. (2022). Gene interaction network analysis reveals IFI44L as a drug target in rheumatoid arthritis and periodontitis. Mol. (Basel, Switz. 27 (9), 2749. doi:10.3390/molecules27092749
Yan-Zhi, X., Jing-Jing, W., Chen, Y. P., Liu, J., Li, N., and Yang, F. Y. (2010). The use of zein and Shuanghuangbu for periodontal tissue engineering. Int. J. Oral Sci. 2 (3), 142–148. doi:10.4248/ijos10056
Yang, C., Lee, J-S., Jung, U-W., Seo, Y-K., Park, J-K., and Choi, S-H. (2013). Periodontal regeneration with nano-hyroxyapatite-coated silk scaffolds in dogs. J. Periodontal Implant Sci. 43 (6), 315–322. doi:10.5051/jpis.2013.43.6.315
Yaremenko, A., Lysenko, A., Ivanova, E., Vilesov, A., Galibin, O., Petrov, N., et al. (2018). Prospectives for using artificial scaffolds in oral and craniofacial surgery: Literature review. Cell. Ther. Transpl. 7, 21–27. doi:10.18620/ctt-1866-8836-2018-7-1-21-27
Yin, B., Xue, B., Wu, Z., Ma, J., and Wang, K. (2018). A novel hybrid 3D-printed titanium scaffold for osteogenesis in a rabbit calvarial defect model. Am. J. Transl. Res. 10 (2), 474–482.
Yoo, H-S., Bae, J-H., Kim, S-E., Bae, E-B., Kim, S-Y., Choi, K-H., et al. (2017). The effect of bisphasic calcium phosphate block bone graft materials with polysaccharides on bone regeneration. Materials 10 (1), 17. doi:10.3390/ma10010017
Yoon, S-J., Yoo, Y., Nam, S. E., Hyun, H., Lee, D-W., Um, S., et al. (2018). The cocktail effect of BMP-2 and TGF-β1 loaded in visible light-cured glycol chitosan hydrogels for the enhancement of bone formation in a rat tibial defect model. Mar. drugs 16 (10), 351. doi:10.3390/md16100351
Yuan, B., Zhou, S-Y., and Chen, X-S. (2017). Rapid prototyping technology and its application in bone tissue engineering. J. Zhejiang Univ. Sci. B 18 (4), 303–315. doi:10.1631/jzus.b1600118
Zafar, M. J., Zhu, D., and Zhang, Z. (2019). 3D printing of bioceramics for bone tissue engineering. Materials 12 (20), 3361. doi:10.3390/ma12203361
Zanwar, K., Ganji, K. K., and Bhongade, M. L. (2017). Efficacy of human umbilical stem cells cultured on polylactic/polyglycolic acid membrane in the treatment of multiple gingival recession defects: A randomized controlled clinical study. J. Dent. 18 (2), 95–103.
Zaszczynska, A., Sajkiewicz, P., and Gradys, A. (2020). Piezoelectric scaffolds as smart materials for neural tissue engineering. Polymers 12 (1), 161. doi:10.3390/polym12010161
Zhang, B., Zhang, P-B., Wang, Z-L., Lyu, Z-W., and Wu, H. (2017). Tissue-engineered composite scaffold of poly (lactide-co-glycolide) and hydroxyapatite nanoparticles seeded with autologous mesenchymal stem cells for bone regeneration. J. Zhejiang Univ. Sci. B 18 (11), 963–976. doi:10.1631/jzus.b1600412
Zhang, J., Chen, Y., Xu, J., Wang, J., Li, C., and Wang, L. (2018). Tissue engineering using 3D printed nano-bioactive glass loaded with NELL1 gene for repairing alveolar bone defects. Regen. Biomater. 5 (4), 213–220. doi:10.1093/rb/rby015
Zhang, K., Wang, S., Zhou, C., Cheng, L., Gao, X., Xie, X., et al. (2018). Advanced smart biomaterials and constructs for hard tissue engineering and regeneration. Bone Res. 6 (1), 31–15. doi:10.1038/s41413-018-0032-9
Zhang, W., Yang, G., Wang, X., Jiang, L., Jiang, F., Li, G., et al. (2017). Magnetically controlled growth‐factor‐immobilized multilayer cell sheets for complex tissue regeneration. Adv. Mat. 29 (43), 1703795. doi:10.1002/adma.201703795
Zhang, X., Lin, X., Liu, T., Deng, L., Huang, Y., and Liu, Y. (2019). Osteogenic enhancement between icariin and bone morphogenetic protein 2: A potential osteogenic compound for bone tissue engineering. Front. Pharmacol. 10, 201. doi:10.3389/fphar.2019.00201
Zhou, H., Weir, M. D., and Xu, H. H. (2011). Effect of cell seeding density on proliferation and osteodifferentiation of umbilical cord stem cells on calcium phosphate cement-fiber scaffold. Tissue Eng. Part A 17 (21-22), 2603–2613. doi:10.1089/ten.tea.2011.0048
Zhou, Y., Chyu, J., and Zumwalt, M. (2018). Recent progress of fabrication of cell scaffold by electrospinning technique for articular cartilage tissue engineering. Int. J. Biomat. 2018, 1–10. doi:10.1155/2018/1953636
Keywords: synthetic materials, biocompatible materials, craniofacial regeneration, dental, tissue engineering
Citation: Yazdanian M, Alam M, Abbasi K, Rahbar M, Farjood A, Tahmasebi E, Tebyaniyan H, Ranjbar R and Hesam Arefi A (2022) Synthetic materials in craniofacial regenerative medicine: A comprehensive overview. Front. Bioeng. Biotechnol. 10:987195. doi: 10.3389/fbioe.2022.987195
Received: 05 July 2022; Accepted: 26 October 2022;
Published: 09 November 2022.
Edited by:
Mona Kamal Marei, Alexandria University, EgyptReviewed by:
Jingang Xiao, Southwest Medical University, ChinaArtak Heboyan, Yerevan State Medical University, Armenia
Dinesh Rokaya, Walailak University International College of Dentistry, Thailand
Copyright © 2022 Yazdanian, Alam, Abbasi, Rahbar, Farjood, Tahmasebi, Tebyaniyan, Ranjbar and Hesam Arefi. This is an open-access article distributed under the terms of the Creative Commons Attribution License (CC BY). The use, distribution or reproduction in other forums is permitted, provided the original author(s) and the copyright owner(s) are credited and that the original publication in this journal is cited, in accordance with accepted academic practice. No use, distribution or reproduction is permitted which does not comply with these terms.
*Correspondence: Elahe Tahmasebi, ZWxhaGUudGFobWFzZWJpLmRlbGZhbkBnbWFpbC5jb20=; Hamid Tebyaniyan, dGVieWFuLmhhbWlkQHlhaG9vLmNvbQ==