- 1Beijing Key Laboratory of Rehabilitation Technical Aids for Old-Age Disability and Key Laboratory of Human Motion Analysis and Rehabilitation Technology of the Ministry of Civil Affairs, National Research Center for Rehabilitation Technical Aids, Beijing, China
- 2School of Mechanical Engineering, Jiangsu University of Technology, Changzhou, China
- 3Department of Biomedical Engineering, Shantou University, Shantou, China
- 4School of Biological Science and Medical Engineering, Beihang University, Beijing, China
Orthopedic implants are widely used for the treatment of bone defects caused by injury, infection, tumor and congenital diseases. However, poor osseointegration and implant failures still occur frequently due to the lack of direct contact between the implant and the bone. In order to improve the biointegration of implants with the host bone, surface modification is of particular interest and requirement in the development of implant materials. Implant surfaces that mimic the inherent surface roughness and hydrophilicity of native bone have been shown to provide osteogenic cells with topographic cues to promote tissue regeneration and new bone formation. A growing number of studies have shown that cell attachment, proliferation and differentiation are sensitive to these implant surface microtopography. This review is to provide a summary of the latest science of surface modified bone implants, focusing on how surface microtopography modulates osteoblast differentiation in vitro and osseointegration in vivo, signaling pathways in the process and types of surface modifications. The aim is to systematically provide comprehensive reference information for better fabrication of orthopedic implants.
1 Introduction
The replacement and healing of bone tissue has become a major challenge worldwide, due to the high incidence of accidents and the prevalence of age-related diseases. Application of bone implant is one of the effective means for the treatment of traumatic and congenital bone defects. However, there is usually no direct contact between the implant and the host bone, which leads to poor osseointegration and implant failure (Xia et al., 2018). Poor interfacial bonding between host bone and implant was mainly associated with minimal osseointegration leading to implant instability, micro-movements and fibrous tissue formation (Srivas et al., 2019). Osteointegration relies not only on mechanical interdigitation to ensure initial fixation, but also on cellular interactions at the surface level to promote osteoconduction, osteoinduction, and healing during the early stage of implantation.
Osseointegration is a dynamic process involving a sequence of cascade responses in which the surface properties of implant play a crucial role (Figure 1, Liu et al., 2020a). Once the implant is placed into the body, an inflammatory response will occur and lead to the release of various proteins such as growth factors and cytokines that form a blood clot (Lotz et al., 2018). The proteins will soon be absorbed by the implant surface from the blood clot, which may act as a signal for cell migration and proliferation (Rivera-Chacon et al., 2013). The specific types of proteins and firmness of adhesion depend largely on the characteristics of the implant surface, such as roughness, and hydrophilicity (Boyan et al., 2017). Cytokines and growth factors stimulate the recruitment of mesenchymal stem cells (MSCs), which proliferate, then differentiate into osteoblasts that are responsible for producing a mineralized matrix and immature woven bone surrounding the implant. Over time, the woven bone gradually matures into lamellar bone, further reinforcing the bone-implant interface (Popat et al., 2007). However, MSCs can also differentiate into fibroblasts that may stimulate the formation of a fibrous membrane on the implant surface and impede the process of bone ingrowth (Razzouk and Schoor, 2012). It is influenced by the properties of implant surface. Therefore, surface properties play a crucial role in the long-term stability and functionality of implants.
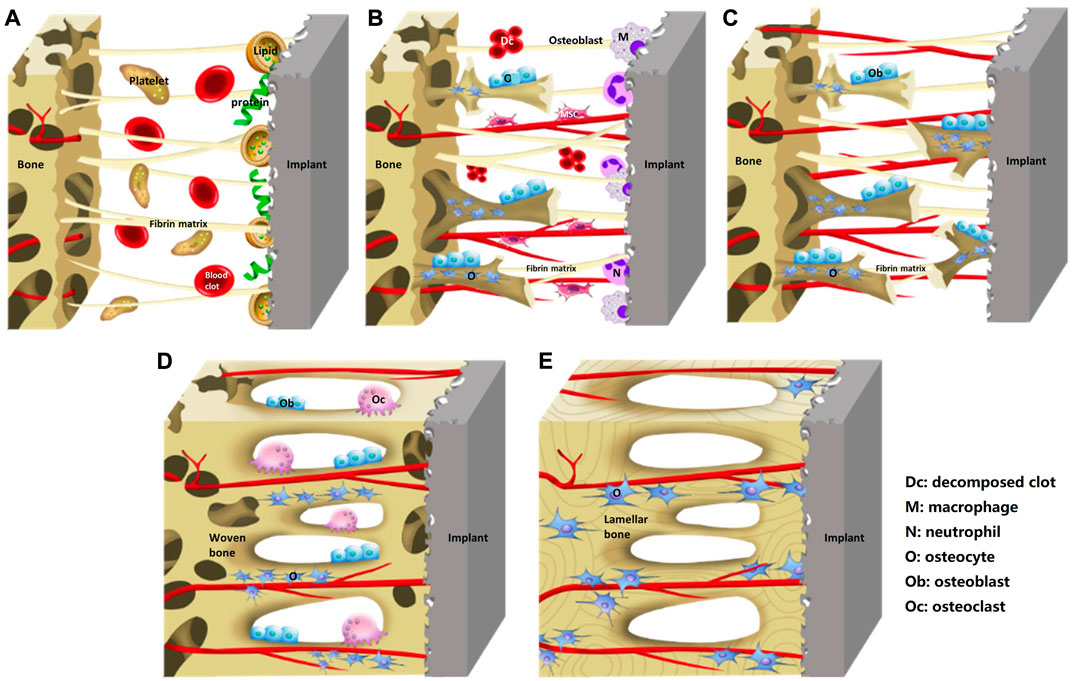
FIGURE 1. Schematic diagram of the process of implants osseointegration. (A) Blood clot and fibrin matrix formation. (B) Woven bone formation. (C) Distance osteogenesis and contact osteogenesis. (D) Newborn woven bones fill up the gap. (E) Woven bone matures into lamellar bone. Reprinted with permission (Liu et al., 2020a). Copyright 2019, the Authors. Published by Wiley.
An ideal implant should have the ability to stimulate osteogenic cells responses such as adhesion and proliferation on the surface. The interface between implants and the surrounding tissue is the critical area and plays a pivotal role in directing cell responses to biomedical devices (Lumetti et al., 2016). Implants surface have effects on cellular responses including contact guidance and influence on cellular functions. It is widely believed that microstructural surface is beneficial to increase bone-implant contact and anchorage for improving the interface bonding strength (Kubo et al., 2009; Lee et al., 2015; Zhu et al., 2018; Piglionico et al., 2020; Di Tinco et al., 2021). Therefore, surface modification is necessary and of particular interest to improve implants bioactivity and other biofunctionalities, and hence enhances the cellular and tissue responses (Lu et al., 2020; Wang et al., 2020; Xue et al., 2020). In an attempt to improve bone formation and implant fixation, implant surface with various microtopographical cues have been investigated to induce the osteogenic differentiation of cells, which may assist more rapid and stable osseointegration, and improve bone bonding at implant surfaces (Karazisis et al., 2016). More attention was paid to the mechanism of interface microenvironment affecting osteogenic behavior in recent years. Although microtopography can enhance osteogenic differentiation of cells, there is no consensus on the optimal structure and feature, and the mechanism is still unclear.
The aim of this review is to provide an overview of the recent experimental evidence to the relationship between microtopography (including microporous structure, microgrooves, micropillars, micropits, nanotubes, and other features at macro-, micro-, and nanoscales) and cell adhesive/osteoinductive properties. Implant with such potential would accelerate implant osseointegration and healing. To this end, this review particularly focuses on surface microtopography modification and its application in implant biomaterials. Briefly, the manuscript has the following aspects: the physical properties of the microtopography, the relationship between the surface microtopography and the cellular in vitro and osteogenesis in vivo, the potential mechanism regarding how microtopography affects osteogenic differentiation of cells, the presently available strategies of surface microtopography modification and the pros and cons for every technique. It will provide reference for the future design of implant surface morphology.
2 Physical properties of microtopographies
Roughness and hydrophilicity are important physical properties of the implant’s microtopography, which have important effects on the biocompatibility. Surface with microstructure creates higher roughness and specific surface area compared to smooth ones, which enhances the contact area between bone tissue and implant; make it more conducive to the anchoring of bone tissue on material, thus improving the stability of bone tissue. Cell adhesion state (Yang et al., 2016), migration (Martínez-Calderon et al., 2016), proliferation (Rabel et al., 2020) and differentiation (Manivasagam and Popat, 2021) can be governed by surface properties.
2.1 Surface roughness
Surface roughness of the implant is essential for integration in tissue regeneration (Matos, 2021). Increasing the average roughness of the implant surface was capable of achieving higher rates of osseointegration. This may be due to the positive influence of surface roughness on protein adsorption and osteoblastic function, as well as higher micromechanical retention of bone on rougher substrates. Surface roughness has high surface energy, which enhances initial protein adsorption and facilitates cell interaction at the implant interface (Srivas et al., 2019). Surface roughness can strongly influence cell adhesion (Majhy et al., 2021), migration and the geometric shape of cells (Petrini et al., 2021). Study has shown that higher levels of cell adhesion were obtained on the rougher surfaces functionalized with the peptide. It has been reported that surface roughness affects cell behavior directly by enhancing the formation of focal contacts or indirectly via selective adsorption of serum proteins required for cell adhesion (Zhang et al., 2016). By sensing the roughness gradient, the cytoskeleton of cells exhibited higher tension on the rougher surface, which was further transferred to the nucleus and ultimately affected the expression of osteogenic related proteins (Chen et al., 2022).
2.2 Surface hydrophilicity
In the presence of the microstructure, the hydrophilicity increased appreciably. The surface wettability can be tuned from hydrophobicity to hydrophilicity by controlling surface topography (Han et al., 2021). The effect of microtopography on surface hydrophilicity has been reported. Compared with untreated surfaces, the contact angle of the microgroove surface is lower (Wang C. et al., 2019). Surface hydrophilicity can modulate the bonding strength, total amount, and conformation of adsorbed proteins that can influence early cell adhesion, proliferation and differentiation. It was found that cells on hydrophilic surface expressed higher level of integrin genes than those on hydrophobic surfaces (Zan et al., 2020). As we know, integrins are a large family of cell surface receptors, which mediate the interactions between cells and matrix (Siebers et al., 2005). They can regulate some members of the cyclin family in a cooperative manner to progress in the cell cycle. The establishment of specific integrin-matrix stimulation can also lead to enhancement gene expression related to differentiation. Studies have demonstrated that integrin mediated focal adhesion maturation promotes osteoblast differentiation (Hendesi et al., 2015). Integrins play an important role in regulating cell behaviors during bone development and repair (Allori et al., 2008). Surface hydrophilicity has been shown to influence osteogenic differentiation of cells via integrin (Kwon and Park, 2018). The higher the hydrophilicity of the material, the better the adhesion of the cells and the better the binding ability to the bone (Sun et al., 2021).
3 Microtopography on bone formation and osseointegration
An ideal bone implant should promote early cells adhesion, osteogenic differentiation and adequate bone integration at the bone-implant interface. After the material is implanted, osteoprogenitor cells migrate to the implant site and differentiate into osteoblasts. Therefore, the properties of implant surface play critical roles in the interactions between the biological environment and the implant, interacting with cells to harmonize their adhesion, migration, proliferation, differentiation, and the consequential bone formation (Srivas et al., 2019). Various functional microstructure surfaces have been proposed to improve the osteogenic differentiation behavior of osteoprogenitor cells and improve osseointegration, achieving immediate or early functional loading in patients with reduced bone density (Chen et al., 2022).
3.1 Osteogenic differentiation behavior of cells
A number of in vitro studies have been performed to evaluate whether surface microtopography can promote cellular reactions that promote bone formation. The most important finding is that textured substrate surfaces can be exploited in inducing osteogenic differentiation of MSCs without any differentiation supplements (Hasturk et al., 2019). At the implant-bone interface, cell adhesion is considered important in defining cells to osteoblast differentiation (Kurashina et al., 2019). What’s important for bone formation is not the number of adhesions that a cell can form, but the size of adhesions (Mas-Moruno et al., 2019). It was reported that stem cells with large spreading and strong contractility prefer osteogenic differentiation, while cells with small spreading and low contractility tend to adipogenic differentiation (Chen and Kawazoe, 2020). Microtopographies with appropriate size and spatial arrangement may provide the basic physical clues required by cell receptors to regulate cell morphology, reorganize cytoskeleton and transmit mechanical signals to the nucleus, which may eventually promote cell differentiation. Numerous studies proved that surface microtopography was able to trigger osteogenic commitment of stem cells, as confirmed by the activation of bone-related markers RUNX2, ALP or ongoing deposition of extracellular matrix supported by the expression of COLI, OPN and OCN (Di Tinco et al., 2020; Xia et al., 2020). For instance, hMSCs on modified topography displayed a noteworthy higher expression of osteogenic transcription factors and the formation of mineralized extracellular matrix when compared to the unmodified materials (Carvalho et al., 2018). Further research also found that the feature size, such as width, height, depth, length, diameter or gap size, can also affect the expression of diverse genes including cellular adhesion, migration and osteogenic differentiation (Stanciuc et al., 2018; Hasturk et al., 2019; Zhu et al., 2019). Therefore, the specific microtopographies feature could induce the osteogenic differentiation of cells, as well as regulate the cell adhesion and morphology (Figure 2, Sun et al., 2021).
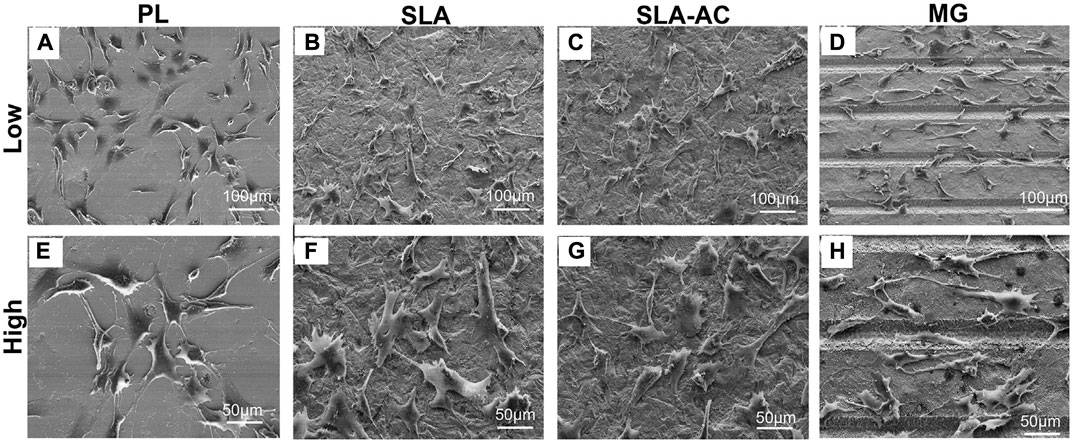
FIGURE 2. Cell adhesion on materials with different microtopography. (A–D) Low magnification and (E–H) high magnification. PL: polished group, SLA: sandblasted group, SLA-AC: sandblasted acid-etched group, MG: microgrooves (Sun et al., 2021). Copyright 2021, the Authors. Published by Elsevier.
3.2 Osseointegration in vivo
One of the requirements for biocompatibility of implants is that the material should integrate with the bone, known as osseointegration. For a long-term and reliable fixation of implants, osseointegration has been proven to be a powerful solution. Numerous reports have demonstrated that the surface microtopography of implants affects the rate of osseointegration and biomechanical fixation. Rough surfaces seem to accelerate and enhance osseointegration by increasing contact osteogenesis (Pellegrini et al., 2018). Wang and his collaborators (Wang G. et al., 2019) fabricated microgroove with graphene oxide coating on Ti6Al4V alloys implant surface by laser processing and chemical assembly techniques. Results indicated that the binding capacity between bone and implants was obviously enhanced in vivo. Wang et al. (Wang G. et al., 2016) constructed hierarchical structure on titanium surface by 6-h treatment of thermal oxidation. Results confirmed that the in vivo bone-implant contact showed enhanced osseointegration than the control group. Another study showed that the hierarchical micro-nano (HMN) surface structure remarkably improved the hydrophilicity of Ti6Al4V and the bone-to-implant contact area and new bone volume were significantly improved on the HMN surface structure compared with the mciro-roughened surface on Ti6Al4V (Moon et al., 2017). A recent experiment also showed that micro/nano structure on composite implant could enhance osteogenesis and osseointegration, evidenced with greater bone-implant contacts and push-out force (Wu et al., 2020). Topographical differences can result in differences in bone-to-implant contact and pullout strength in vivo (Schwartz et al., 2008). Nanostructuring on a surface with micro-sized roughness has been proposed to induce fast regeneration of the surrounding tissues by regulating the protein interactions (Mendonca et al., 2008). The structural similarity of an HMN structure with native bone that composed of macro-, micro-, and nano-scale components might endow implant surface with favorable osseointegrative activity.
4 Signaling pathways of interface morphology on osteogenesis
Extracellular mechanical signals induced by microtopography are transformed into intracellularly biochemical signals, which are achieved by mechanotransduction process (Burridge et al., 2019; Stewart et al., 2020). This process involves mechanosensing, which in turn activates multiple signaling pathways. Studies have proven that mechanotransduction is involved in micromorphology induced osteogenesis (Fu et al., 2020).Microtopography-induced signals can be transported via cytoskeleton and the changes in cell shape to adapt to the underlying surface. Actin cytoskeleton has been shown to remodel in response to microtopography. This remodeling instigates subsequent mechanotransductive pathways, ultimately leading to the osteogenic differentiation (Chen et al., 2022). The molecular mechanisms are mainly known to include Wnt/β-catenin and yes-associated protein and transcriptional coactivator with the PDZ-binding motif (YAP/TAZ) pathway (Figure 3).
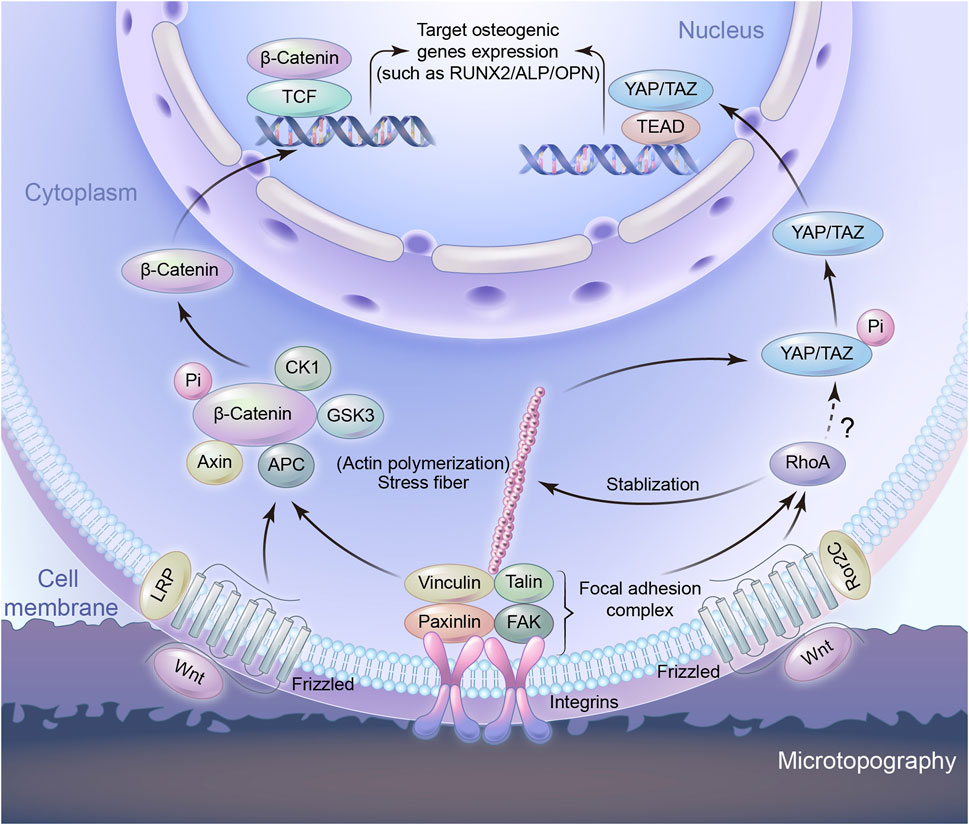
FIGURE 3. Schematic representation of signaling pathways to regulate osteogenic gene expression induced by microtopography.
4.1 Wnt/β-catenin pathway
Wnt signaling is one of the pivotal pathways controlling cell proliferation and differentiation in tissue homeostasis and is known to play a crucial role in bone regeneration, bone formation and osseointegration (Steinhart and Angers, 2018; Staehlke et al., 2020). Hydrophilic rough surface enhanced the expression level of osteogenesis related genes and significantly increased the expression of several Wnt ligands such as Wnt3a, Wnt5a, and the Wnt receptors of the Frizzled family in Wnt signaling pathway (Galli et al., 2010; Donos et al., 2011; Li et al., 2017). Wnt proteins interact with frizzled-LRP surface receptors such that the down stream effector β-catenin is increasingly translocated to the nucleus (Li et al., 2017), while at the same time inhibiting the complex that degrades β-catenin, namely AXIN1 (Hutchings et al., 2020; Rougerie et al., 2021). Under these conditions, the increased signaling through Wnt/β-catenin signaling pathway is associated with the expression of calcification and osteogenic markers. In addition, RhoA plays a role in controlling Wnt upregulation on microstructured titanium surfaces (Lumetti et al., 2014). Noncanonical Wnt factors bind to receptor Frizzled-Ror2C, which activate RhoA. RhoA, in turns, activates downstream target to phosphorylate Myosin II. Acto-Myosin filaments create cell tension, which facilitates the activation of canonical Wnt/β-catenin signaling. A feedback loop from tension-activated structures controls RhoA activation levels. Actin microfilaments can also control RhoA, too (Lumetti et al., 2014). It is likely that the modification of integrin-based adhesions by the topography is involved at the starting point of the Wnt-mediated response to topography. The silencing of the integrin β3 not only blocked the osteogenic behaviour of MSCs cultured on nanoroughness, but also decreased the expression of β-catenin and Frizzled (Lopes et al., 2019). Osteogenic rough surfaces also increase the expression of Wnt5a together with the expression of the integrins in MSC (Olivares-Navarrete et al., 2011). Importantly, the exogenous addition of Wnt5a and Wnt5a knock-down experiments demonstrated that the integrin upregulation was Wnt-dependent (Olivares-Navarrete et al., 2011), suggesting a mutually reinforcing crosstalk between integrin adhesions and Wnt signalling (Barlian and Vanya, 2022). Integrin regulates differentiation through the Wnt pathway, and Wnt increases the expression of integrin, resulting in further regulation of osteogenic differentiation (Sun et al., 2018; Rougerie et al., 2021).
4.2 YAP/TAZ pathway
YAP and TAZ are homologous transcriptional coactivators in the Hippo signaling pathway, identified as an important regulatory pathway that restricts cell proliferation and differentiation. Phosphorylated forms of YAP/TAZ are sequestered in the cytoplasm. Both of them act as the nuclear relays of mechanical signals exerted by ECM properties and cell shape and their function depends on Rho and tension of the actin cytoskeleton, as well as cell spreading (Dupont et al., 2011). Studies have shown cell adhesion to matrix proteins can trigger YAP nuclear localization through and integrin/FAK/Src axis, which was involved in nanotube induced differentiation (Tong et al., 2020). Cell mechanotransduction comprises signaling pathways, such as the Rho/ROCK pathway, that result in cell contractility, cytoskeletal and ECM remodeling, or activation of YAP/TAZ that triggers the initiation of a cascade of transcription factors. Contractile actomyosin complexes act as central mediators between microtopography cues and YAP/TAZ signaling in various mechanotransduction environments (Jo et al., 2020). Controlling cells adhesive area on micropatterned substrates can regulate the nucleo-cytoplasmic localization of YAP/TAZ, whose target genes partly explained the proliferation and differentiation of MSCs. Cells with large spreading area exhibited enhanced formation of actin stress fibers and YAP nuclear location. Inhibiting either the actin polymerization or actomyosin contractility reduced the nuclear localization of YAP through disrupting the formation of actin stress fibers. It demonstrates that the mechanical stimuli induced nuclear translocation of YAP/TAZ is determined by cytoskeletal interaction (Wada et al., 2011). A stiffer or tailored surface will increase integrin clustering and FA formation, thereby increasing F-actin polymerization and stress fiber formation. Larger cell spreading area on the microstructure surface will support the translocation of YAP to the nucleus, as well as the expression of preosteogenic genes (Zhang et al., 2016). It is this balance between the YAP/TAZ ratio in cytoplasm and nucleus that plays a role in the regulation of cell differentiation (Heng et al., 2020; Seong et al., 2020; Zhang et al., 2021). The role of TAZ protein in osteogenesis is coactivating transcription by RUNX2 for osteogenesis, while at the same time inhibiting transcription by PPARγ for adipogenesis (Heng et al., 2020).
The Wnt/β-catenin and YAP/TAZ pathways have in common with the remodeling of integrin-mediated adhesions in contact with microtopography. This indicates that the transduction of the topographical cues starts at the cell-implant interface and may be attributed to the features of adhesion sites. Compared to a flat surface, the microtopography provides fewer adhesion sites for cells. Therefore, for cells in order to adhere to the morphology surface steadily, the focal adhesion complex begins to assemble and mature, the F-actin becomes strong and stable. F-actin is one of the major components of the cytoskeleton and can be regulated by RhoA (Tong et al., 2020). Integrins couple the substrate to the other focal adhesion proteins so as to facilitate cell attachment. The adhesion mediated topography sensing involves the signaling pathways classically associated with osteogenic differentiation. Topography, however, can be sensed by the cell as a whole due to the morphological deformation it imposes. Deformation induced microtopography sensing is still unclear, because it involves the whole cell as a unique and complex mechanical unit. Therefore, that is really an attractive future challenge in this field.
5 Strategies of surface topology modification
Improvement of the implant’s bioactivation to achieve enhanced interfacial bonding and osseointegration has been classically addressed by various surface engineering methods. These methods mainly focused on increasing the roughness and hydrophilicity by constructing micro, nano or nano/micro hierarchical structure of the implant surface (Wang X. et al., 2016; Wang G. et al., 2019; Sun et al., 2021). The surface roughness is associated with increased wettability. Surface-roughening procedures may also affect the surface chemical composition of the implants, which impacts the hydrophilicity of the implant surface (Junker et al., 2009). A study has shown that micro roughness induces hydrophobicity but subsequently enhances hydrophilicity compared with Ti surfaces without microstructuring (Rupp et al., 2004). Surface modification with high roughness and hydrophilicity were capable of promoting the proliferation and differentiation of cells and achieving higher rates of osseointegration (Wennerberg et al., 2014). The following techniques can be utilized either individually or in combination. Each method has its own specific advantages and limitations (Table 1).
5.1 Uncontrolled microtopography
5.1.1 Plasma spraying
Plasma spray is one of the methods to produce roughness that projected out from the implant surface. This is a thermal spraying technology using plasma arc as heat source and has been widely used in the biomedical field. The technology is simple and easy to operate, and provides a cost-effective, straightforward, and reliable approach for constructing materials with micron scale characteristics on implant surface. Many parameters involved in this method, which can potentially affect the microstructure of coatings. Plasma spray was highly irregular in form, but it can stimulate osteoblast differentiation in vitro and support osteogenesis in vivo (Ratha et al., 2022; Udduttula et al., 2022). Liu et al. proposed a novel vapor-induced poreforming atmospheric plasma spraying to prepare bioactive porous HA coating with the potential to promote osteoblast attachment and differentiation (Liu et al., 2020b). However, plasma spray implants were gradually replaced by implants with more stable and improved surface morphology in the marketplace because of the leakage of ions, which were taken up by the surrounding cells (Rautray et al., 2011). In addition, the temperature of plasma spray is extremely high, and the coating surface subjects to large thermal stresses. More attention should be paid to the improvement in the preparation of coatings on small and special-shaped workpieces.
5.1.2 Grit blasting
Blasting the implants with forcing abrasive particles against the surface is another approach for roughening the surface., thereby facilitating cell adhesion. According to the size of grit particles and the length of sand blasting process, pits of various sizes can be created on the implant surface (Guehennec et al., 2007). Roughness was in the scale of hundreds of microns to tens of microns. Different ceramic particles have been utilized, such as aluminum oxide, titanium oxide, calcium phosphate and magnesium sulfate particles. Experimental studies have indicated a higher bone-to-implant contact for blasted surfaces in comparison with control surfaces (Hoornaert et al., 2020). Other experiments have demonstrated higher marginal bone levels and survival rates for TiO2 grit-blasted implants than for machined turned implants (Steenberghe et al., 2000; Sirello et al., 2018). Grit blasting may also have the disadvantage of low processing efficiency. The blasting material is commonly embedded into the implant surface and residue remains even after ultrasonic cleaning, acidic passivation and sterilization (Guehennec et al., 2007).
5.1.3 Chemical etching
Chemical etching including acid, alkali, hydrothermal treatments and other similar treatment have been reported to develop superhydrophobic surfaces (Kim et al., 2019; Lian et al., 2020). This method consists of a selective and controlled corrosion process and is one of the most promising methods for its commercial application development, due to its low manufacturing costs (Sun et al., 2020). It has been reported that leaf-like, needle-like or pyramid-like nanostructures can be developed on implant surface by autoclaving in presence of distilled water or NaOH solution at 120–240°C (Obata and Kasuga, 2007). The nest-like structure on the surface of metal implants can be prepared by utilizing hydrothermal method in KOH (Wandiyanto et al., 2020) or NaOH solution (Lin et al., 2014), most of which are hydrothermally treated at 110–150°C for 2–24 h, and some need to be calcined at 450–500°C for 2–4 h. However, high KOH/NaOH concentration and longer fabrication duration (often exceeds 24 h) limit the usage of this method. Hydrothermal utilizes aqueous solution as the reaction system in a specific sealed reactor, such as autoclave. Hydrothermal treatment is considered as the inexpensive technique with high engineering potential for irregularly shaped implants (Srivas et al., 2019). Generally, this method often utilizes expensive apparatus and corrosive fluoride based chemicals, has a long reaction cycle, restricts equipment requirements and has technical difficulties such as strict temperature and pressure control.
5.1.4 Anodic oxidation
Anodic oxidation is an accelerated electrochemical process, in which oxide film is applied to the implants surface while immersed in an electrolyte bath. It is now ordinarily utilized to increase the thickness of TiO2 layer and fabricate controllable nanostructures on titanium implant surfaces (Liu et al., 2020a). Several possible morphologies can be produced by anodic oxidation technique, which depend not only on cell voltage, but also on the composition of the electrolytic solution, in which the electrodes are immersed. Microarc oxidation (MAO) is developed based on conventional anodizing technology and its process predominantly relies on the matching adjustment of the electrolyte and the electrical parameters. The process is performed on the surfaces of Ti and other valve metals and their alloys at instantaneous high temperatures and pressures generated by arc discharge (Xue et al., 2020). By the principle of plasma electrolytic oxidation, MAO can generate a macro porous and tightly adherent TiO2 film on the Ti substrate, which got a lot of attention (Zhang L. et al., 2020; Shen et al., 2020). The enhanced surface hydrophilicity of the porous coating prepared by MAO can stimulate the interaction between the implant and the surrounding biological environment. Although anodic oxidation is convenient and economical, the bonding strength with implant matrix needs to be further improved. The multilevel structure designs, multiscale coating or coating with novel surface morphology should also be developed.
5.2 Controlled microtopography
Micromachining may be the best way to prepare an implant surface in a controlled manner. Micro/nano fabrication provides a wide opportunity for the modification of surface with ordered shape, size, and spatial arrangement for controlling or inducing stem cell differentiation. Compared with traditional mechanical and chemical processing methods, it has the advantages of high precision, less pollution, flexible use and high controllability.
5.2.1 Laser treatment
Laser treatment is a contactless process, which does not pollute the implant surface, and has the advantages of wide applicability, high resolution, fast speed, good repeatability (Vanithakumari et al., 2021; Chen et al., 2022). Femtosecond laser micromachining has been efficiently applied to supply microtopography in implant surface (Carvalho et al., 2018; Stanciuc et al., 2018; Wang C. et al., 2019; Trueba et al., 2021). The principle of femtosecond laser is that the laser pulses travelled through air to the focusing device, which can focus the beam and position the sample. The sample is placed on a motorised platform with three-axis motion, so that the pulse can impinge perpendicularly onto its surfaces (Luo et al., 2021). Femtosecond laser technology has the advantages of high precision and low heat production on the material surface (Vanaei et al., 2021). Femtosecond laser treatment can significantly increase the surface roughness, hydrophilicity and surface area of the implant, and reduce residual elements, which provides greater potential for the integration of material and bone (Sun et al., 2021). Many previous studies on the use of laser treatment of ceramic surfaces reported that the hydrophilicity of the surfaces was improved (Hao et al., 2005; Hao and Lawrence, 2006). However, the optimization of all parameters, including power density, scanning speed, frequency and pulse duration, is still a big challenge.
5.2.2 Photolithography
Photolithography is widely used in the microelectronics industry to create micropatterns on flat surfaces. Desired pattern in titanium and silicon have also been generated by photolithography (Kim et al., 2018; Ponomarev et al., 2019). Mainly, the patterning of a layer of photosensitive polymer (photoresist) was produced by utilizing UV or X-ray light at first. The light is shone through a patterned mask giving the designed pattern in the form of UV-opaque features on a UV-transparent background. Then, the desired pattern was transferred to the substrate by dry etching or wet etching of the uncoated areas (Kang et al., 2017). Photolithography is an ideal method for fabricating microstructure, but the disadvantage is that it usually requires a flat surface to start with and needs chemical post-treatment.
5.2.3 Hot embossing
Hot embossing is another technique used for micropatterning. Sun et al. produced microgrooved polystyrene substrates by hot embossing and demonstrated the effect of microgrooved PS surfaces on the morphology, metabolic activity, proliferation and osteogenic differentiation of MG-63 osteoblast-like cells (Sun et al., 2016). Briefly, hot embossing imprint lithography is to press a micromachined master directly into the polymer at an elevated temperature to form a relief pattern. The major advantages of this technology are cost-effectiveness, accuracy and the ability to generate 3D features. However, this technology is limited to thermoplastics and it is difficult to fabricate complex 3D structures (Chen et al., 2022).
5.2.4 Micro-milling
As one of the micromachining methods, micro-milling technology provides high flexibility by manufacturing complex 3D microscale products and has promising applications in preparation of controllable, microscale surface modification, which provides firm support for the surface modification of titanium (Yue et al., 2019; Zhang X. et al., 2020; Kiswanto et al., 2020). By selecting appropriate cutting parameters, superior surface quality can be obtained using micro-milling. The cutting force, temperature, vibration and deflection have a great impact on the quality of the machining process and results. Several studies have been done to identify the appropriate cutting parameters in micro-milling process of Ti6Al4V (Bandapalli et al., 2018; Dadgari et al., 2018). Cai et al. manufactured microstructures on stainless steel using micro-milling, and investigated the effect of microstructure on surface contact angle (Cai et al., 2017).
It should be noted that the aforementioned strategies could be combined to achieve synergies and special structures to improve biological responses (Liang et al., 2022). Generally, microstructures at micron level can be prepared by sandblasting, acid etching, micromachining, electrochemical machining, etc, while nanoscale surface microstructure can be achieved by hydrothermal, alkali heat treatment, anodic oxidation and other technologies. Although all scales of topography are capable of inducing cell alterations, different scales can influence the osteogenic behavior of cells in different ways due to the cellular interaction with the surrounding environment. Nanoscale topography regulates cell behavior primarily through spatial restrictions on the location and size of adhesion complexes, which can modulate cell adhesion to adsorbed proteins through integrin and consequently induce intracellular expression of target genes (Davison et al., 2016). Cell-matrix interaction with microscale topography generates effects by modifying the entire cell morphology, that is, inducing cell deformation due to cytoskeletal remodeling to adapt to substrate shape (Rabel et al., 2020). Natural bone is a hierarchical structure containing macroscale, microscale, and nanoscale organizations (Wang X. et al., 2016). From a biomimetic perspective, micro/nano textured surface can mimic the structure of bone tissue and enhance cell responses. The micro/nano hierarchical architecture has been accounted to accelerate cell functions by synergistic equilibrating between cellular proliferation and differentiation (Srivas et al., 2019; Sun et al., 2022). Studies have shown that it is possible to create hierarchical textures through the combination of micro and nano features on the same surface. For instance, Maher et al. (Maher et al., 2021) created bioinspired multistructured surfaces on SLM-printed Ti6Al4V implants by combining electrochemical anodization and hydrothermal methods. The implants display unique surfaces with a distinctive dual micro to nano topography composed of micron-sized spherical features and vertically aligned nanoscale pillar structures, which can increase the deposition of hydroxyapatite minerals in simulated body fluids and the adhesion of human osteoblast-like cells. It should be pointed out that most of the modification strategies resulted in a surface that had a complex multi-scale roughness. The hydrophilic properties added to the roughened surfaces have shown higher biocompatibility and have induced faster osseointegration, compared to the existing modified surfaces (Yeo, 2014). After roughening the surface by combination of sandblasting and acid etching (SLA), increased hydrophilicity can promote bone formation and exhibit higher osteogenic potential compared to samples without modifications to hydrophilic properties (Wall et al., 2009). A hydrophilization technique evaluated clinically used phosphonic acid coupled to blasted and acid-etched surfaces to generate a “biomimetic” surface with increased hydrophilicity (Esposito et al., 2013). Taken together, it is essential to choose an appropriate method in terms of implant materials, applying situations and fabricating procedures. Construction of hierarchical microtopography mimicking native bone on the implant surface is an effective modification strategy to improve cell responses and osseointegration.
6 Summary
For a long-term and stable fixation of implants, osseointegration has been considered as a pivotal process. Osseointegration is affected by various factors including materials, surface microtopography, the environment of bone-implant interface, the design of the implant itself, and so on. Materials determine the principal characteristics of implants. Qualified materials should have enough mechanical properties, high corrosion and good biocompatibility (Liu et al., 2020b). Surface modification is used to modify the chemical, physical, and biological properties of implant surface for better osseointegration and has raised increasing attention in modern orthopedic medicine recently. Moreover, bone is a 3D inhomogeneous structure with complex topography. Porous implants that have a similar hierarchical structure on multiple scales with native bone may facilitate osseointegration. The pores enhance the permeability of implants and create the space for nutrient exchanges, which grant better biocompatibility and osseointegration potential for implants (Cheng et al., 2018).
Microtopography of the implant surface is potent modulator of the osseointegration. Different cell types prefer various microenvironments. Fibroblasts adhere more strongly to smooth surfaces while rough surfaces facilitate the adhesion and proliferation of osteoblasts. Modifying the biomaterial surface properties to enhance host cell adhesion and function has been a major focus. Biomaterial surfaces with physical properties of roughness or hydrophilicity and have complex HMN surface structure, are more effective in supporting osseointegration. Roughness, hydrophilicity and chemical composition are the bridges connecting the physicochemical properties and biological properties of implant surfaces. Although it is possible to induce stem cells differentiation towards the osteoblast lineage by controlling the microtopography, no optimal micropattern structure and size have been confirmed yet. To further analyze the connection between cell behaviors and the microtopography, potential mechanotransduction mechanisms need to be investigated. At present, Wnt/β-catenin and YAP/TAZ signal pathways were recognized as mediating the regulation of cell adhesion and cytoskeleton organization on the microtopography, which ultimately affected the expression of osteogenic genes.
A large number of surface treatment approaches emerged for modifying implant surfaces with the purpose of increasing surface roughness. These modification techniques can be combined to create hierarchical textures mimicking host bone structure. However, each method has its own specific advantages and limitations. It is still urgent to develop a simple, efficient, easy-to-operate, safe, and controllable method or to combine various surface modification methods to play a synergistic effect, and combine their advantages to conquer deficiencies. Nonetheless, it is increasingly accepted that infection is also a major cause of implant failure. Implant surfaces that support osseointegration may also favor colonization of bacterial cells. Therefore, to improve the success of implants, biomaterial surfaces should ideally discourage the attachment of bacteria without affecting osteogenic cell functions (Spriano et al., 2018). Future strategies should explore a combined goal.
In conclusion, surface microtopography of the implant plays a very important role in osseointegration. Further research is still required on the preparation of tailored and standardized microtopography. In addition, how cells sense the microenvironment and transform mechanical cues into intracellular signals, the underlying mechanisms of interaction between microtopography and cells need further investigation, which could help to create tailored implant surfaces to promote bone formation around the implant.
Author contributions
YZ and HG conceived the study and wrote the manuscript. ZF and YX searched the database. SJ contributed intellectually throughout the study and ZM revised the manuscript and finalized the manuscript.
Funding
This review was supported by the National Natural Science Foundation of China (grant numbers 11902090, 11902126 and 11672078).
Conflict of interest
The authors declare that the research was conducted in the absence of any commercial or financial relationships that could be construed as a potential conflict of interest.
Publisher’s note
All claims expressed in this article are solely those of the authors and do not necessarily represent those of their affiliated organizations, or those of the publisher, the editors and the reviewers. Any product that may be evaluated in this article, or claim that may be made by its manufacturer, is not guaranteed or endorsed by the publisher.
References
Allori, A. C., Sailon, A. M., and Warren, S. M. (2008). Biological basis of bone formation, remodeling, and repair-part II: Extracellular matrix. Tissue Eng. Part B Rev. 14, 275–283. doi:10.1089/ten.teb.2008.0083
Bandapalli, C., Sutaria, B. M., Prasad Bhatt, D. V., and Singh, K. K. (2018). Tool wear analysis of micro end mills- uncoated and PVD coated TiAlN & AlTiN in high speed micro milling of titanium Alloy-Ti-0.3Mo-0.8Ni. Procedia CIRP 77, 626–629. doi:10.1016/j.procir.2018.08.191
Barlian, A., and Vanya, K. (2022). Nanotopography in directing osteogenic differentiation of mesenchymal stem cells: Potency and future perspective. Future Sci. OA 8 (1), FSO765. doi:10.2144/fsoa-2021-0097
Boyan, B. D., Lotz, E. M., and Schwartz, Z. (2017). <sup/>Roughness and hydrophilicity as osteogenic biomimetic surface properties. Tissue Eng. Part A 23 (23-24), 1479–1489. doi:10.1089/ten.TEA.2017.0048
Burridge, K., Monaghan-Benson, E., and Graham, D. M. (2019). Mechanotransduction: From the cell surface to the nucleus via RhoA. Phil. Trans. R. Soc. B 374 (1779), 20180229. doi:10.1098/rstb.2018.0229
Cai, Y., Chang, W., Luo, X., and Qin, Y. (2017). “Hydrophobicity of pyramid structures fabricated by micro milling,” in 2017 World Congress on Micro and Nano Manufacturing, Garden Villa, March 27–30, 2017.
Carvalho, A., Cangueiro, L., Oliveira, V., Vilar, R., Fernandes, M. H., and Monteiro, F. J. (2018). Femtosecond laser microstructured alumina toughened zirconia: A new strategy to improve osteogenic differentiation of hMSCs. Appl. Surf. Sci. 435, 1237–1245. doi:10.1016/j.apsusc.2017.11.206
Chen, C., Zhu, Y., Wang, R., Han, Y., and Zhou, H. (2022). Effect of controlled microtopography on osteogenic differentiation of mesenchymal stem cells. J. Healthc. Eng. 2022, 1–10. doi:10.1155/2022/7179723
Chen, G., and Kawazoe, N. (2020). “Regulation of stem cell functions by micro-patterned structures,” in Biomimicked biomaterials: Advances in tissue engineering and regenerative medicine. Editors H. J. Chun, R. L. Reis, A. Motta, and G. Khang (Singapore: Springer Singapore), 141–155.
Cheng, B. C., Koduri, S., Wing, C. A., Woolery, N., Cook, D. J., and Spiro, R. C. (2018). Porous titanium-coated polyetheretherketone implants exhibit an improved bone–implant interface: An in vitro and in vivo biochemical, biomechanical, and histological study. Med. Devices (Auckl) 11, 391–402. doi:10.2147/MDER.S180482
Dadgari, A., Huo, D., and Swailes, D. (2018). Investigation on tool wear and tool life prediction in micro-milling of Ti-6Al-4V. Nanotechnol. Precis. Eng. 1 (4), 218–225. doi:10.1016/j.npe.2018.12.005
Davison, M. J., McMurray, R. J., Smith, C. A., Dalby, M. J., and Meek, R. D. (2016). Nanopit-induced osteoprogenitor cell differentiation: The effect of nanopit depth. J. Tissue Eng. 7, 204173141665277. doi:10.1177/2041731416652778
Di Tinco, R., Bertani, G., Pisciotta, A., Bertoni, L., Bertacchini, J., Colombari, B., et al. (2021). Evaluation of antimicrobial effect of air-polishing treatments and their influence on human dental pulp stem cells seeded on titanium disks. Int. J. Mol. Sci. 22 (2), 865. doi:10.3390/ijms22020865
Di Tinco, R., Sergi, R., Bertani, G., Pisciotta, A., Bellucci, D., Carnevale, G., et al. (2020). Effects of a novel bioactive glass composition on biological properties of human dental pulp stem cells. Materials 13 (18), 4049. doi:10.3390/ma13184049
Donos, N., Hamlet, S., Lang, N., Salvi, G., Huynhba, G., Bosshardt, D., et al. (2011). Gene expression profile of osseointegration of a hydrophilic compared with a hydrophobic microrough implant surface. Clin. Oral Implants Res. 22 (4), 365–372. doi:10.1111/j.1600-0501.2010.02113.x
Dupont, S., Morsut, L., Aragona, M., Enzo, E., Giulitti, S., Cordenonsi, M., et al. (2011). Role of YAP/TAZ in mechanotransduction. Nature 474 (7350), 179–183. doi:10.1038/nature10137
Esposito, M., Dojcinovic, I., Germon, L., Levy, N., Curno, R., Buchini, S., et al. (2013). Safety and efficacy of a biomimetic monolayer of permanently bound multiphosphonic acid molecules on dental implants: 1 year post-loading results from a pilot quadruple-blinded randomised controlled trial. Eur. J. Oral Implantol. 6 (3), 227–236. doi:10.5137/1019-5149.JTN.6565-12.0
Fu, J., Liu, X., Tan, L., Cui, Z., Liang, Y., Li, Z., et al. (2020). Modulation of the mechanosensing of mesenchymal stem cells by laser-induced patterning for the acceleration of tissue reconstruction through the Wnt/β-catenin signaling pathway activation. Acta Biomater. 101, 152–167. doi:10.1016/j.actbio.2019.10.041
Galli, C., Passeri, G., Ravanetti, F., Elezi, E., Pedrazzoni, M., and Macaluso, G. M. (2010). Rough surface topography enhances the activation of Wnt/β-catenin signaling in mesenchymal cells. J. Biomed. Mat. Res. A 95a (3), 682–690. doi:10.1002/jbm.a.32887
Guehennec, L. L., Soueidan, A., Layrolle, P., and Amouriq, Y. (2007). Surface treatments of titanium dental implants for rapid osseointegration. Dent. Mat. 23 (7), 844–854. doi:10.1016/j.dental.2006.06.025
Han, J., Zhang, F., Van Meerbeek, B., Vleugels, J., Braem, A., and Castagne, S. (2021). Laser surface texturing of zirconia-based ceramics for dental applications: A review. Mater. Sci. Eng. C 123, 112034. doi:10.1016/j.msec.2021.112034
Hao, L., and Lawrence, J. (2006). Effects of Nd:YAG laser treatment on the wettability characteristics of a zirconia-based bioceramic. Opt. Lasers Eng. 44 (8), 803–814. doi:10.1016/j.optlaseng.2005.08.001
Hao, L., Ma, D. R., Lawrence, J., and Zhu, X. (2005). Enhancing osteoblast functions on a magnesia partially stabilised zirconia bioceramic by means of laser irradiation. Mat. Sci. Eng. C. 25 (4), 496–502. doi:10.1016/j.msec.2005.03.003
Hasturk, O., Ermis, M., Demirci, U., Hasirci, N., and Hasirci, V. (2019). Square prism micropillars on poly(methyl methacrylate) surfaces modulate the morphology and differentiation of human dental pulp mesenchymal stem cells. Colloids Surfaces B Biointerfaces 178, 44–55. doi:10.1016/j.colsurfb.2019.02.037
Hendesi, H., Barbe, M. F., Safadi, F. F., Monroy, M. A., and Popoff, S. N. (2015). Integrin mediated adhesion of osteoblasts to connective tissue growth factor (CTGF/CCN2) induces cytoskeleton reorganization and cell differentiation. PLoS One 10 (2), e0115325. doi:10.1371/journal.pone.0115325
Heng, B. C., Zhang, X., Aubel, D., Bai, Y., Li, X., Wei, Y., et al. (2020). Role of YAP/TAZ in cell lineage fate determination and related signaling pathways. Front. Cell Dev. Biol. 8, 735. doi:10.3389/fcell.2020.00735
Hoornaert, A., Vidal, L., Besnier, R., Morlock, J. F., Louarn, G., and Layrolle, P. (2020). Biocompatibility and osseointegration of nanostructured titanium dental implants in minipigs. Clin. Oral Implants Res. 31 (6), 526–535. doi:10.1111/clr.13589
Hutchings, G., Moncrieff, L., Dompe, C., Janowicz, K., Sibiak, R., Bryja, A., et al. (2020). Bone regeneration, reconstruction and use of osteogenic cells; from basic knowledge, animal models to clinical trials. J. Clin. Med. 9 (1), 139. doi:10.3390/jcm9010139
Jo, J., Abdi Nansa, S., and Kim, D. H. (2020). Molecular regulators of cellular mechanoadaptation at cell–material interfaces. Front. Bioeng. Biotechnol. 8, 608569. doi:10.3389/fbioe.2020.608569
Junker, R., Dimakis, A., Thoneick, M., and Jansen, J. A. (2009). Effects of implant surface coatings and composition on bone integration: A systematic review. Clin. Oral Implants Res. 20, 185–206. doi:10.1111/j.1600-0501.2009.01777.x
Kang, M., Byun, J. H., Na, S., and Jeon, N. L. (2017). Fabrication of functional 3D multi-level microstructures on transparent substrates by one step back-side UV photolithography. RSC Adv. 7 (22), 13353–13361. doi:10.1039/C6RA28812J
Karazisis, D., Ballo, A. M., Petronis, S., Agheli, H., Emanuelsson, L., Thomsen, P., et al. (2016). The role of well-defined nanotopography of titanium implants on osseointegration: Cellular and molecular events in vivo. Int. J. Nanomedicine 11, 1367–1382. doi:10.2147/IJN.S101294
Kim, E. C., Lee, D. Y., Lee, M. H., Lee, H. J., Kim, K. H., Leesungbok, R., et al. (2018). The effect of fibronectin-immobilized microgrooved titanium substrata on cell proliferation and expression of genes and proteins in human gingival fibroblasts. Tissue Eng. Regen. Med. 15, 615–627. doi:10.1007/s13770-018-0153-7
Kim, H. M., Choi, J. W., Kwon, J. S., Lee, C. H., and Kim, B. (2019). Super-hydrophobic properties of aluminum surfaces synthesized by a two-step chemical etching process. J. Nanosci. Nanotechnol. 19 (10), 6452–6457. doi:10.1166/jnn.2019.17060
Kiswanto, G., Mandala, A., Azmi, M., and Ko, T. J. (2020). The effects of cutting parameters to the surface roughness in high speed cutting of micro-milling titanium alloy Ti-6Al-4V. Key Eng. Mat. 846, 133–138. doi:10.4028/www.scientific.net/KEM.846.133
Kubo, K., Tsukimura, N., Iwasa, F., Ueno, T., Lei, S., Aita, H., et al. (2009). Cellular behavior on TiO2 nanonodular structures in a micro-to-nanoscale hierarchy model. Biomaterials 30 (29), 5319–5329. doi:10.1016/j.biomaterials.2009.06.021
Kurashina, Y., Ezura, A., Murakami, R., Mizutani, M., and Komotori, J. (2019). Effect of hydroxy groups and microtopography generated by a nanosecond-pulsed laser on pure Ti surfaces. J. Mat. Sci. Mat. Med. 30, 57. doi:10.1007/s10856-019-6259-1
Kwon, Y. S., and Park, J. W. (2018). Osteogenic differentiation of mesenchymal stem cells modulated by a chemically modified super-hydrophilic titanium implant surface. J. Biomater. Appl. 33 (2), 205–215. doi:10.1177/0885328218786873
Lee, H. J., Lee, J., Lee, J. T., Hong, J. S., Lim, B. S., Park, H. J., et al. (2015). Microgrooves on titanium surface affect peri-implant cell adhesion and soft tissue sealing; an in vitro and in vivo study. J. Periodontal Implant Sci. 45 (3), 120–126. doi:10.5051/jpis.2015.45.3.120
Li, G., Song, Y., Shi, M., Du, Y., Wang, W., and Zhang, Y. (2017). Mechanisms of Cdc42-mediated rat MSC differentiation on micro/nanotextured topography. Acta Biomater. 49, 235–246. doi:10.1016/j.actbio.2016.11.057
Lian, Z., Xu, J., Wang, Z., and Yu, H. (2020). Biomimetic superlyophobic metallic surfaces: Focusing on their fabrication and applications. J. Bionic Eng. 17 (1), 1–33. doi:10.1007/s42235-020-0002-y
Liang, L., Huang, Q., Wu, H., He, H., Lei, G., Zhao, D., et al. (2022). Engineering nano-structures with controllable dimensional features on micro-topographical titanium surfaces to modulate the activation degree of M1 macrophages and their osteogenic potential. J. Mat. Sci. Technol. 96, 167–178. doi:10.1016/j.jmst.2021.03.078
Lin, L., Wang, H., Ni, M., Rui, Y., Cheng, T., Cheng, C., et al. (2014). Enhanced osteointegration of medical titanium implant with surface modifications in micro/nanoscale structures. J. Orthop. Transl. 2 (1), 35–42. doi:10.1016/j.jot.2013.08.001
Liu, Y., Lin, G., Lee, Y., Huang, T., Chang, T., Chen, Y., et al. (2020a). Microstructures and cell reaction of porous hydroxyapatite coatings on titanium discs using a novel vapour-induced pore-forming atmospheric plasma spraying. Surf. Coat. Technol. 393, 125837. doi:10.1016/j.surfcoat.2020.125837
Liu, Y., Rath, B., Tingart, M., and Eschweiler, J. (2020b). Role of implants surface modification in osseointegration: A systematic review. J. Biomed. Mat. Res. A 108 (3), 470–484. doi:10.1002/jbm.a.36829
Lopes, H. B., Freitas, G. P., Elias, C. N., Tye, C., Stein, J. L., Stein, G. S., et al. (2019). Participation of integrin β3 in osteoblast differentiation induced by titanium with nano or microtopography. J. Biomed. Mat. Res. A 107, 1303–1313. doi:10.1002/jbm.a.36643
Lotz, E. M., Berger, M. B., Schwartz, Z., and Boyan, B. D. (2018). Regulation of osteoclasts by osteoblast lineage cells depends on titanium implant surface properties. Acta Biomater. 68, 296–307. doi:10.1016/j.actbio.2017.12.039
Lu, M., Chen, H., Yuan, B., Zhou, Y., Min, L., Xiao, Z., et al. (2020). The morphological effect of nanostructured hydroxyapatite coatings on the osteoinduction and osteogenic capacity of porous titanium. Nanoscale 12 (47), 24085–24099. doi:10.1039/D0NR06306A
Lumetti, S., Manfredi, E., Ferraris, S., Spriano, S., Passeri, G., Ghiacci, G., et al. (2016). The response of osteoblastic MC3T3-E1 cells to micro- and nano-textured, hydrophilic and bioactive titanium surfaces. J. Mat. Sci. Mat. Med. 27 (4), 68. doi:10.1007/s10856-016-5678-5
Lumetti, S., Mazzotta, S., Ferrillo, S., Piergianni, M., Piemontese, M., Passeri, G., et al. (2014). RhoA controls Wnt upregulation on microstructured titanium surfaces. Biomed. Res. Int. 2014, 1–9. doi:10.1155/2014/401859
Luo, F., Wang, L., Xiao, Z., Zhu, X., Fan, Y., Wang, K., et al. (2021). Application of femtosecond laser microfabrication in the preparation of advanced bioactive titanium surfaces. J. Mat. Chem. B 9 (18), 3912–3924. doi:10.1039/D1TB00231G
Maher, S., Wijenayaka, A. R., Lima-Marques, L., Yang, D., Atkins, G. J., and Losic, D. (2021). Advancing of additive-manufactured titanium implants with bioinspired micro- to nanotopographies. ACS Biomater. Sci. Eng. 7 (2), 441–450. doi:10.1021/acsbiomaterials.0c01210
Majhy, B., Priyadarshini, P., and Sen, A. K. (2021). Effect of surface energy and roughness on cell adhesion and growth – facile surface modification for enhanced cell culture. RSC Adv. 11 (25), 15467–15476. doi:10.1039/D1RA02402G
Manivasagam, V. K., and Popat, K. C. (2021). Hydrothermally treated titanium surfaces for enhanced osteogenic differentiation of adipose derived stem cells. Mat. Sci. Eng. C. 128, 112315. doi:10.1016/j.msec.2021.112315
Martínez-Calderon, M., Manso-Silván, M., Rodríguez, A., Gómez-Aranzadi, M., García-Ruiz, J. P., Olaizola, S. M., et al. (2016). Surface micro- and nano- texturing of stainless steel by femtosecond laser for the control of cell migration. Sci. Rep. 6, 36296. doi:10.1038/srep36296
Mas-Moruno, C., Su, B., and Dalby, M. J. (2019). Multifunctional coatings and nanotopographies: Toward cell instructive and antibacterial implants. Adv. Healthc. Mat. 8 (1), 1801103. doi:10.1002/adhm.201801103
Matos, G. R. M. (2021). Surface roughness of dental implant and osseointegration. J. Maxillofac. Oral Surg. 20 (1), 1–4. doi:10.1007/s12663-020-01437-5
Mendonca, G., Mendonca, D. B., Aragao, F. J., and Cooper, L. F. (2008). Advancing dental implant surface technology-- from micron- to nanotopography. Biomaterials 29, 3822–3835. doi:10.1016/j.biomaterials.2008.05.012
Moon, B. S., Kim, S., Kim, H. E., and Jang, T. S. (2017). Hierarchical micro-nano structured Ti6Al4V surface topography via two-step etching process for enhanced hydrophilicity and osteoblastic responses. Mater. Sci. Eng. C 73, 90–98. doi:10.1016/j.msec.2016.12.064
Obata, A., and Kasuga, T. (2007). Surface modification of titanium with hydrothermal treatment. Mater. Sci. Forum 561-565, 1521–1524. doi:10.4028/www.scientific.net/MSF.561-565.1521
Olivares-Navarrete, R., Hyzy, S. L., Berg, M. E., Schneider, J. M., Hotchkiss, K., Schwartz, Z., et al. (2014). Osteoblast lineage cells can discriminate microscale topographic features on titanium-aluminum- vanadium surfaces. Ann. Biomed. Eng. 42, 2551–2561. doi:10.1007/s10439-014-1108-3
Olivares-Navarrete, R., Hyzy, S. L., Park, J. H., Dunn, G. R., Haithcock, D. A., Wasilewski, C. E., et al. (2011). Mediation of osteogenic differentiation of human mesenchymal stem cells on titanium surfaces by a Wnt-integrin feedback loop. Biomaterials 32, 6399–6411. doi:10.1016/j.biomaterials.2011.05.036
Pellegrini, G., Francetti, L., Barbaro, B., and del Fabbro, M. (2018). Novel surfaces and osseointegration in implant dentistry. J. Invest. Clin. Dent. 9 (4), e12349. doi:10.1111/jicd.12349
Petrini, M., Pierfelice, T. V., D’Amico, E., Di Pietro, N., Pandolfi, A., D’Arcangelo, C., et al. (2021). Influence of nano, micro, and macro topography of dental implant surfaces on human gingival fibroblasts. Int. J. Mol. Sci. 22 (18), 9871. doi:10.3390/ijms22189871
Piglionico, S., Bousquet, J., Fatima, N., Renaud, M., Collart-Dutilleul, P. Y., and Bousquet, P. (2020). Porous tantalum vs. titanium implants: Enhanced mineralized matrix formation after stem cells proliferation and differentiation. J. Clin. Med. 9 (11), 3657. doi:10.3390/jcm9113657
Ponomarev, V. A., Shvindina, N. V., Permyakova, E. S., Slukin, P. V., Ignatov, S. G., Sirota, B., et al. (2019). Structure and antibacterial properties of Ag-doped micropattern surfaces produced by photolithography method. Colloids Surfaces B Biointerfaces 173, 719–724. doi:10.1016/j.colsurfb.2018.10.040
Popat, K. C., Leoni, L., Grimes, C. A., and Desai, T. A. (2007). Influence of engineered titania nanotubular surfaces on bone cells. Biomaterials 28 (21), 3188–3197. doi:10.1016/j.biomaterials.2007.03.020
Rabel, K., Kohal, R. J., Steinberg, T., Tomakidi, P., Rolauffs, B., Adolfsson, E., et al. (2020). Controlling osteoblast morphology and proliferation via surface micro-topographies of implant biomaterials. Sci. Rep. 10, 12810. doi:10.1038/s41598-020-69685-6
Ratha, I., Datta, P., Chand Reger, N., Das, H., Balla, V. K., Devi, K. B., et al. (2022). In vivo osteogenesis of plasma sprayed ternary-ion doped hydroxyapatite coatings on Ti6Al4V for orthopaedic applications. Ceram. Int. 48 (8), 11475–11488. doi:10.1016/j.ceramint.2022.01.004
Rautray, T. R., Narayanan, R., and Kim, K. H. (2011). Ion implantation of titanium based biomaterials. Prog. Mat. Sci. 56 (8), 1137–1177. doi:10.1016/j.pmatsci.2011.03.002
Razzouk, S., and Schoor, R. (2012). Mesenchymal stem cells and their challenges for bone regeneration and osseointegration. J. Periodontol. 83 (5), 547–550. doi:10.1902/jop.2011.110384
Rivera-Chacon, D. M., Alvarado-Velez, M., Acevedo-Morantes, C. Y., Singh, S. P., Gultepe, E., Nagesha, D., et al. (2013). Fibronectin and vitronectin promote human fetal osteoblast cell attachment and proliferation on nanoporous titanium surfaces. J. Biomed. Nanotechnol. 9 (6), 1092–1097. doi:10.1166/jbn.2013.1601
Rougerie, P., Santos, R., Farina, M., and Anselme, K. (2021). Molecular mechanisms of topography sensing by osteoblasts: An update. Appl. Sci. (Basel). 11 (4), 1791. doi:10.3390/app11041791
Rupp, F., Scheideler, L., Rehbein, D., Axmann, D., and Geis-Gerstorfer, J. (2004). Roughness induced dynamic changes of wettability of acid etched titanium implant modifications. Biomaterials 25, 1429–1438. doi:10.1016/j.biomaterials.2003.08.015
Schwartz, Z., Raz, P., Zhao, G., Barak, Y., Tauber, M., Yao, H., et al. (2008). Effect of micrometer-scale roughness of the surface of Ti6Al4V pedicle screws in vitro and in vivo. J. Bone Jt. Surgery-American Volume 90, 2485–2498. doi:10.2106/JBJS.G.00499
Seong, H., Higgins, S. G., Penders, J., Armstrong, J. P. K., Crowder, S. W., Moore, A. C., et al. (2020). Size-tunable nanoneedle arrays for influencing stem cell morphology, gene Expression, and nuclear membrane curvature. ACS Nano 14 (5), 5371–5381. doi:10.1021/acsnano.9b08689
Shen, X., Ping, L., Wang, L., Liu, C., Liu, J., and Deng, Z. (2020). Improving the stability and bioactivity of micro-arc oxidized calcium phosphate/titania porous coatings by high energy shot peening pretreatment. Ceram. Int. 46 (2), 2041–2048. doi:10.1016/j.ceramint.2019.09.183
Siebers, M. C., ter Brugge, P. J., Walboomers, X. F., and Jansen, J. A. (2005). Integrins as linker proteins between osteoblasts and bone replacing materials. A critical review. Biomaterials 26, 137–146. doi:10.1016/j.biomaterials.2004.02.021
Sirello, R., Frattini, D., Banci, L., Baracchino, A., Canciani, E., and Dellavia, C. (2018). Short-term clinical results of a calcium magnesium carbonate-blasted dental implant. Int. J. Oral Health Med. Res. 4 (6), 1–6.
Spriano, S., Yamaguchi, S., Baino, F., and Ferraris, S. (2018). A critical review of multifunctional titanium surfaces: New frontiers for improving osseointegration and host response, avoiding bacteria contamination. Acta Biomater. 79, 1–22. doi:10.1016/j.actbio.2018.08.013
Srivas, P. K., Kapat, K., Das, B., Pal, P., Ray, P. G., and Dhara, S. (2019). Hierarchical surface morphology on Ti6Al4V via patterning and hydrothermal treatment towards improving cellular response. Appl. Surf. Sci. 478, 806–817. doi:10.1016/j.apsusc.2019.02.039
Staehlke, S., Haack, F., Waldner, A. C., Koczan, D., Moerke, C., Mueller, P., et al. (2020). ROS dependent wnt/β-catenin pathway and its regulation on defined micro-pillars—a combined in vitro and in silico study. Cells 9 (8), 1784. doi:10.3390/cells9081784
Stanciuc, A. M., Flamant, Q., Sprecher, C. M., Alini, M., Anglada, M., and Peroglio, M. (2018). Femtosecond laser multi-patterning of zirconia for screening of cell-surface interactions. J. Eur. Ceram. Soc. 38 (3), 939–948. doi:10.1016/j.jeurceramsoc.2017.08.019
Steenberghe, D. V., Mars, G. D., Quirynen, M., Jacobs, R., and Naert, I. (2000). A prospective split-mouth comparative study of two screw: Haped self-tapping pure titanium implant systems. Clin. Oral Implants Res. 11 (3), 202–209. doi:10.1034/j.1600-0501.2000.011003202.x
Steinhart, Z., and Angers, S. (2018). Wnt signaling in development and tissue homeostasis. Development 145 (11), dev146589. doi:10.1242/dev.146589
Stewart, S., Darwood, A., Masouros, S., Higgins, C., and Ramasamy, A. (2020). Mechanotransduction in osteogenesis. Bone Jt. Res. 9 (1), 1–14. doi:10.1302/2046-3758.91.BJR-2019-0043.R2
Sun, L., Chen, X., Mu, H., Xu, Y., Chen, R., Xia, R., et al. (2022). Titanium nanobowl-based nest-like nanofiber structure prepared at room temperature and pressure promotes osseointegration of beagle implants. Front. Bioeng. Biotechnol. 10, 841591. doi:10.3389/fbioe.2022.841591
Sun, L., Pereira, D., Wang, Q., Barata, D. B., Truckenmüller, R., Li, Z., et al. (2016). Controlling growth and osteogenic differentiation of osteoblasts on microgrooved polystyrene surfaces. PloS one 11 (8), e0161466. doi:10.1371/journal.pone.0161466
Sun, M., Chi, G., Xu, J., Tan, Y., Xu, J., Lv, S., et al. (2018). Extracellular matrix stiffness controls osteogenic differentiation of mesenchymal stem cells mediated by integrin α5. Stem Cell Res. Ther. 9, 52. doi:10.1186/s13287-018-0798-0
Sun, R., Zhao, J., Li, Z., Qin, N., Mo, J., Pan, Y., et al. (2020). Robust superhydrophobic aluminum alloy surfaces with anti-icing ability, thermostability, and mechanical durability. Prog. Org. Coatings 147, 105745. doi:10.1016/j.porgcoat.2020.105745
Sun, Y., Sun, J., Wu, X., Li, Y., Li, X., Li, R., et al. (2021). Mechanism of zirconia microgroove surface structure for osseointegration. Mat. Today Adv. 12, 100159. doi:10.1016/j.mtadv.2021.1001599
Tong, Z., Liu, Y., Xia, R., Chang, Y., Hu, Y., Liu, P., et al. (2020). F-actin regulates osteoblastic differentiation of mesenchymal stem cells on TiO2 nanotubes through MKL1 and YAP/TAZ. Nanoscale Res. Lett. 15, 183. doi:10.1186/s11671-020-03415-9
Trueba, P., Giner, M., Rodríguez, Á., Beltrán, A. M., Amado, J. M., Montoya-García, M. J., et al. (2021). Tribo-mechanical and cellular behavior of superficially modified porous titanium samples using femtosecond laser. Surf. Coat. Technol. 422, 127555. doi:10.1016/j.surfcoat.2021.127555
Udduttula, A., Li, J., Ma, Z., Teng, B., Zhang, J. V., Ferreira, A. M., et al. (2022). A novel apatite-inspired Sr5(PO4)2SiO4 plasma-sprayed coating on Ti alloy promoting biomineralization, osteogenesis and angiogenesis. Ceram. Int. 48 (8), 10979–10989. doi:10.1016/j.ceramint.2021.12.317
Vanaei, S., Parizi, M. S., Vanaei, S., Salemizadehparizi, F., and Vanaei, H. R. (2021). An overview on materials and techniques in 3D bioprinting toward biomedical application. Eng. Regen. 2, 1–18. doi:10.1016/j.engreg.2020.12.001
Vanithakumari, S. C., Choubey, A. K., Thinaharan, C., Gupta, R. K., George, R. P., Kaul, R., et al. (2021). Laser patterned titanium surfaces with superior antibiofouling, superhydrophobicity, self-cleaning and durability: Role of line spacing. Surf. Coat. Technol. 418, 127257. doi:10.1016/j.surfcoat.2021.127257
Wada, K.-I., Itoga, K., Okano, T., Yonemura, S., and Sasaki, H. (2011). Hippo pathway regulation by cell morphology and stress fibers. Development 138, 3907–3914. doi:10.1242/dev.070987
Wall, I., Donos, N., Carlqvist, K., Jones, F., and Brett, P. (2009). Modified titanium surfaces promote accelerated osteogenic differentiation of mesenchymal stromal cells in vitro. Bone 45 (1), 17–26. doi:10.1016/j.bone.2009.03.662
Wandiyanto, J. V., Tamanna, T., Linklater, D. P., Truong, V. K., Al Kobaisi, M., Baulin, V. A., et al. (2020). Tunable morphological changes of asymmetric titanium nanosheets with bactericidal properties. J. Colloid Interface Sci. 560, 572–580. doi:10.1016/j.jcis.2019.10.067
Wang, C., Hu, H., Li, Z., Shen, Y., Xu, Y., Zhang, G., et al. (2019a). Enhanced osseointegration of titanium alloy implants with laser microgrooved surfaces and graphene oxide coating. ACS Appl. Mat. Interfaces 11 (43), 39470–39483. doi:10.1021/acsami.9b12733
Wang, G., Li, J., Lv, K., Zhang, W., Ding, X., Yang, G., et al. (2016a). Surface thermal oxidation on titanium implants to enhance osteogenic activity and in vivo osseointegration. Sci. Rep. 6 (1), 31769. doi:10.1038/srep31769
Wang, G., Wan, Y., and Liu, Z. (2019b). Construction of complex structures containing micro-pits and nano-pits on the surface of titanium for cytocompatibility improvement. Materials 12 (17), 2820. doi:10.3390/ma12172820
Wang, Q., Zhou, P., Liu, S., Attarilar, S., Ma, R. L. W., Zhong, Y., et al. (2020). Multi-scale surface treatments of titanium implants for rapid osseointegration: A review. Nanomaterials 10 (6), 1244. doi:10.3390/nano10061244
Wang, X., Xu, S., Zhou, S., Xu, W., Leary, M., Choong, P., et al. (2016b). Topological design and additive manufacturing of porous metals for bone scaffolds and orthopaedic implants: A review. Biomaterials 83, 127–141. doi:10.1016/j.biomaterials.2016.01.012
Wennerberg, A., Jimbo, R., Stübinger, S., Obrecht, M., Dard, M., and Berner, S. (2014). Nanostructures and hydrophilicity influence osseointegration: A biomechanical study in the rabbit tibia. Clin. Oral Implants Res. 25 (9), 1041–1050. doi:10.1111/clr.12213
Wu, H., Liu, T., Xu, Z., Qian, J., Shen, X., Li, Y., et al. (2020). Enhanced bacteriostatic activity, osteogenesis and osseointegration of silicon nitride/polyetherketoneketone composites with femtosecond laser induced micro/nano structural surface. Appl. Mat. Today 18, 100523. doi:10.1016/j.apmt.2019.100523
Xia, J., Yuan, Y., Wu, H., Huang, Y., and Weitz, D. A. (2020). Decoupling the effects of nanopore size and surface roughness on the attachment, spreading and differentiation of bone marrow-derived stem cells. Biomaterials 248, 120014. doi:10.1016/j.biomaterials.2020.120014
Xia, L., Xie, Y., Fang, B., Wang, X., and Lin, K. (2018). In situ modulation of crystallinity and nano-structures to enhance the stability and osseointegration of hydroxyapatite coatings on Ti-6Al-4V implants. Chem. Eng. J. 347, 711–720. doi:10.1016/j.cej.2018.04.045
Xue, T., Attarilar, S., Liu, S., Liu, J., Song, X., Li, L., et al. (2020). Surface modification techniques of titanium and its alloys to functionally optimize their biomedical properties: Thematic review. Front. Bioeng. Biotechnol. 8, 603072. doi:10.3389/fbioe.2020.603072
Yang, W., Han, W., He, W., Li, J., Wang, J., Feng, H., et al. (2016). Surface topography of hydroxyapatite promotes osteogenic differentiation of human bone marrow mesenchymal stem cells. Mat. Sci. Eng. C. 60, 45–53. doi:10.1016/j.msec.2015.11.012
Yeo, I. S. (2014). Reality of dental implant surface modification: A short literature review. Open Biomed. Eng. J. 8, 114–119. doi:10.2174/1874120701408010114
Yue, C., Gao, H., Liu, X., Liang, S. Y., and Wang, L. (2019). A review of chatter vibration research in milling. Chin. J. Aeronautics 32 (2), 215–242. doi:10.1016/j.cja.2018.11.007
Zan, F., Wei, Q., Fang, L., Xian, M., Ke, Y., and Wu, G. (2020). Role of stiffness versus wettability in regulating cell behaviors on polymeric surfaces. ACS Biomater. Sci. Eng. 6 (2), 912–922. doi:10.1021/acsbiomaterials.9b01430
Zhang, L., Chen, L., and Wang, L. (2020a). Surface modification of titanium and titanium alloys: Technologies, developments, and future interests. Adv. Eng. Mat. 22 (5), 1901258. doi:10.1002/adem.201901258
Zhang, W., Yang, Y., and Cui, B. (2021). New perspectives on the roles of nanoscale surface topography in modulating intracellular signaling. Curr. Opin. Solid State Mat. Sci. 25 (1), 100873. doi:10.1016/j.cossms.2020.100873
Zhang, X., Wan, Y., Ren, B., Wang, H., Yu, M., Liu, A., et al. (2020b). Preparation of superhydrophobic surface on titanium alloy via micro-milling, anodic oxidation and fluorination. Micromachines 11 (3), 316. doi:10.3390/mi11030316
Zhang, Y., Gong, H., Sun, Y., Huang, Y., and Fan, Y. (2016). Enhanced osteogenic differentiation of MC3T3-E1 cells on grid-topographic surface and evidence for involvement of YAP mediator. J. Biomed. Mat. Res. A 104 (5), 1143–1152. doi:10.1002/jbm.a.35648
Zhu, C., Lv, Y., Qian, C., Ding, Z., Jiao, T., Gu, X., et al. (2018). Microstructures, mechanical, and biological properties of a novel Ti-6V-4V/zinc surface nanocomposite prepared by friction stir processing. Int. J. Nanomedicine 13, 1881–1898. doi:10.2147/IJN.S154260
Zhu, M., Ye, H., Fang, J., Zhong, C., Yao, J., Park, J., et al. (2019). Engineering high-resolution micropatterns directly onto titanium with optimized contact guidance to promote osteogenic differentiation and bone regeneration. ACS Appl. Mat. Interfaces 11 (47), 43888–43901. doi:10.1021/acsami.9b16050
Keywords: implants, microtopography, surface modification, osseointegration, mechanism
Citation: Zhang Y, Fan Z, Xing Y, Jia S, Mo Z and Gong H (2022) Effect of microtopography on osseointegration of implantable biomaterials and its modification strategies. Front. Bioeng. Biotechnol. 10:981062. doi: 10.3389/fbioe.2022.981062
Received: 26 July 2022; Accepted: 12 September 2022;
Published: 26 September 2022.
Edited by:
Masoud Mozafari, University of Toronto, CanadaReviewed by:
Jiaxiang Bai, Soochow University, ChinaRosanna Di Tinco, University of Modena and Reggio Emilia, Italy
Copyright © 2022 Zhang, Fan, Xing, Jia, Mo and Gong. This is an open-access article distributed under the terms of the Creative Commons Attribution License (CC BY). The use, distribution or reproduction in other forums is permitted, provided the original author(s) and the copyright owner(s) are credited and that the original publication in this journal is cited, in accordance with accepted academic practice. No use, distribution or reproduction is permitted which does not comply with these terms.
*Correspondence: Zhongjun Mo, bW96aG9uZ2p1bi5idWFhQGZveG1haWwuY29t; He Gong, Ym1lZ29uZ2hlQGJ1YWEuZWR1LmNu