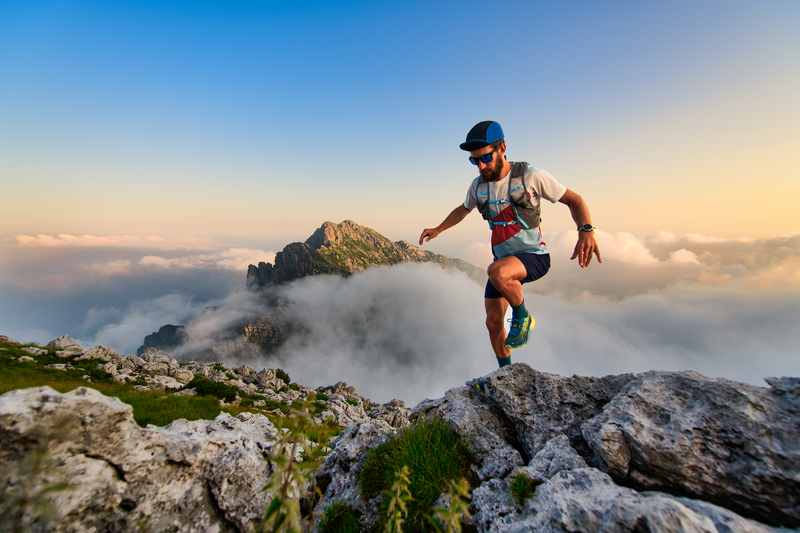
95% of researchers rate our articles as excellent or good
Learn more about the work of our research integrity team to safeguard the quality of each article we publish.
Find out more
REVIEW article
Front. Bioeng. Biotechnol. , 08 September 2022
Sec. Biomaterials
Volume 10 - 2022 | https://doi.org/10.3389/fbioe.2022.977282
This article is part of the Research Topic Metallic Alloys in Medical Applications View all 11 articles
A correction has been applied to this article in:
Corrigendum: Bio-high entropy alloys: Progress, challenges, and opportunities
With the continuous progress and development in biomedicine, metallic biomedical materials have attracted significant attention from researchers. Due to the low compatibility of traditional metal implant materials with the human body, it is urgent to develop new biomaterials with excellent mechanical properties and appropriate biocompatibility to solve the adverse reactions caused by long-term implantation. High entropy alloys (HEAs) are nearly equimolar alloys of five or more elements, with huge compositional design space and excellent mechanical properties. In contrast, biological high-entropy alloys (Bio-HEAs) are expected to be a new bio-alloy for biomedicine due to their excellent biocompatibility and tunable mechanical properties. This review summarizes the composition system of Bio-HEAs in recent years, introduces their biocompatibility and mechanical properties of human bone adaptation, and finally puts forward the following suggestions for the development direction of Bio-HEAs: to improve the theory and simulation studies of Bio-HEAs composition design, to quantify the influence of composition, process, post-treatment on the performance of Bio-HEAs, to focus on the loss of Bio-HEAs under actual service conditions, and it is hoped that the clinical application of the new medical alloy Bio-HEAs can be realized as soon as possible.
As one of the material foundations of human production and life, metal materials have always played an essential role in the development history of human civilization. In recent decades, with the continuous development of science and technology, people have put forward higher and higher requirements for the comprehensive properties of metal materials. People have been changing the properties of materials by adding relatively small amounts of secondary elements to the primary elements. For example, C and Cr elements are added to steel to improve strength and corrosion resistance, and Al-Mn and Al-Mg alloys formed by adding Mn and Mg to aluminum have good corrosion resistance and plasticity (Zhang et al., 2008; Serda, 2013; Liu et al., 2021; Zhou et al., 2022). However, such a primary-element approach dramatically limits the total number of possible element combinations and, therefore, the total number of alloys, most of which have been identified and exploited. New approaches are needed if the compositional space for exploration is significantly enlarged. To obtain alloy materials with better properties, in the 1990s, researchers got alloys with high mixing entropy by adding alloy components (Peker and Johnson, 1993; Choi-Yim and Johnson, 1997). In 2004, Ye et al. (Yeh et al., 2004) prepared multi-principal composition alloys with equal or nearly equal molar ratios, and named such multi-principal alloys as high entropy alloys (HEAs) for the first time. And unlike conventional alloys, the properties of HEAs are jointly influenced by multiple constituent elements. High entropy alloys have advantages not found in conventional alloys, such as high strength, high-temperature resistance, corrosion resistance, etc. (Yeh, 2006, 2013; Senkov et al., 2010, 2011; Miracle et al., 2014; Zhang et al., 2014, 2018; George et al., 2019; Xin et al., 2020).
At present, high-entropy alloys refer to a class of alloys composed of five or more elements, and the atoms of each component are smelted and alloyed according to an equal atomic ratio or close to an equal atomic ratio and have high mixing entropy and solid solution formation tendency (Schopphoven et al., 2016; Liang et al., 2022). Scholars have conducted a lot of research on HEA and have concluded four core effects: high entropy effect, sluggish diffusion, lattice distortion, and cocktail effect, as shown in Figure 1 (Xin et al., 2020). Among them, high lattice distortion and high mixing entropy will lead to a large degree of atomic disorder in the alloy. This allows HEA to have low Gibbs free energy, which significantly improves the stability of the single solid solution phase and inhibits the formation of intermetallic compounds (Wang B. et al., 2018; Huo et al., 2018; Nong et al., 2018). Structural “lattice distortion effect,” that is, the difference in atomic size among various elements, can cause severe lattice distortion, which is considered to be the primary reason for the high strength of high-entropy alloys and has an essential impact on the morphology and movement of dislocation lines (Ma Y. et al., 2020; Xie et al., 2020). The cocktail effect refers to the fact that HEA is an alloy formed by mixing multiple elements and will exhibit properties that a single pure metal element does not have (Lin C. L. et al., 2021). These properties give HEA a more comprehensive range of applications (Wang et al., 2019; Wei et al., 2021; Jiang et al., 2022a, 2022b; Cheng et al., 2022).
FIGURE 1. Schematic illustration of the properties and characteristics of HEAs (Xin et al., 2020).
Currently, potential applications for HEAs include corrosion-resistant materials, nuclear materials, molds, and biomedicine (George et al., 2020; Soto et al., 2020). Among these, HEAs have great potential in the biomedical field, and biomaterials science continues to be at the forefront of research and innovation in clinical and biomedical applications as medical technology advances and the needs of the population increase. An illustrative example of the significant impact is that degenerative diseases of the bones and joints, such as osteoporosis, affect many people worldwide, mainly middle-aged and postmenopausal women (Cohen et al., 2022; Iki et al., 2022; Mattia et al., 2022). Growing clinical demand for reconstructive joint replacements is prompting researchers to develop implants with better function, biocompatibility, and improved clinical outcomes. Alloys have been used as bone implants for many years. Among them, stainless steel, cobalt-based alloys (CoCrMo), and titanium and their alloys are widely used for their good biocompatibility, sufficient mechanical strength, and excellent corrosion resistance (Gross et al., 2020; Shipilova et al., 2020). However, implants made from these materials are usually much stiffer than natural bone, leading to stress shielding - a significant source of bone resorption and eventual failure of such implants. The human skeleton can be divided into dense bone (cortical bone) and trabecular bone (cancellous bone). Dense bone is almost solid, while the porosity of trabecular bone varies between 50 and 90%, and the mechanical properties of the bone vary significantly with age, anatomical location, and bone mass (Lei et al., 2013; Al-Hafidh et al., 2020; Gaffuri et al., 2021). Such complex mechanical property modulation is difficult to achieve with a single principal element alloy, which requires substantial elemental modulation by HEA to meet specific bone-implant needs. Such complex mechanical property modulation is difficult to achieve with a single primary element alloy, which requires significant elemental modulation of HEA to meet specific bone-implant needs.
We call this HEA with biomedical application potential Biological High Entropy Alloy (Bio-HEAs) [41], and many scholars have already researched the related direction. Metal biomaterials must be composed of raw materials with good biocompatibility, such as non-toxic and non-allergenic materials (Meloni et al., 2019; Kazemi et al., 2020; Spataru et al., 2021). The selection of constituent elements is particularly critical for Bio-HEA. Bio-HEA is mainly composed of Ti, Zr, Hf, Nb, Ta, V, Mo, and W. These elements do not cause side effects to the tissue cells at the implantation site or are within the safe range of side effects to the body throughout the service phase. High-entropy alloys have become one of the most promising medical metal materials in recent years due to their biological safety, high strength, high corrosion resistance, high wear resistance, and ease of forming simple objects. Since high entropy alloys are tunable in terms of properties, the desired properties can be obtained by changing the type or content of the elements in the HEAs, which gives the HEAs a broader scope of application. In the field of biomedicine, high-entropy alloys have a similar hardness to the bone, high specific strength, good corrosion, and wear resistance, and these characteristics are in line with the typical attributes of biomedical metal materials, which means that there is a good potential for its application in the medical health field.
Figure 2 shows the structure and main content of the paper. The second section reviews the composition design theory of Bio-HEAs. It presents the progress of research on developing Bio-HEAs compositions based on the first principles of density functional theory (DFT) simulations calculation. The third section discusses the biocompatibility of the designed and developed Bio-HEAs, including cytocompatibility and corrosion resistance. The fourth section compares the mechanical properties of Bio-HEAs with human bone and commonly used bio-alloys, including strength-ductility, elastic properties, and wear resistance. The fifth section discusses the future development prospects of Bio-HEAs and explores the possibility of high-entropy alloys in biomedicine.
FIGURE 2. A mind map to explore the design theory, biocompatibility, and mechanical properties of Bio-HEAs in this paper.
The proposal of the concept of the high-entropy alloy not only improves the freedom of alloy material composition but also dramatically increases the difficulty of its composition exploration and performance optimization. Selecting alloying elements and the appropriate proportions are particularly important to obtain high-entropy alloys with specific phase structures or properties (Pradeep et al., 2015; Takeuchi et al., 2015; Tian et al., 2015; Ye et al., 2015). The core problems involved in the current high entropy alloys research can often be summarized as the problem of alloy composition design and property optimization (LaRosa et al., 2019; George et al., 2020; Soto et al., 2020).
As one of the members of high-entropy alloys, Bio-HEA has the same component design concept as high-entropy alloys. Due to the large number of optional components of HEAs and the high content of each component, the complex physical properties and chemical synergism between various alloying elements will ultimately significantly affect the mechanical properties and microstructure of high-entropy alloys (Cann et al., 2021; Ostovari Moghaddam et al., 2021). This chapter summarizes the research on Bio-HEAs in recent years as a composition system. The combined effects of all the components are considered from composition design theory. The phase formation laws and mechanisms are discussed through solid-solution phase formation rules. The Bio-HEAs alloy properties are predicted by simulation, such as first principles and molecular dynamics. Machine learning is introduced to provide a reference database for the composition design guidelines of new high-entropy alloys.
Bio-HEA alloys were developed based on the research of refractory high-entropy alloys (RHEAs). In 2010, Senkov et al. (Senkov et al., 2010) synthesized near-equivalent atoms WTaMoNb and WTaMoNbV alloys with single-phase body-centered cubic (BCC) lattice using the vacuum arc melting technique. Since then, many other HEAs based on refractory elements (Ti, Zr, Hf, V, Nb, Ta, Cr, Mo, and W) have been the focus of experimental studies (Gu P. et al., 2022; Chen S. H. et al., 2022; Huang et al., 2022; Peng et al., 2022; Zhou et al., 2022). The original RHEA was designed based on five refractory elements (Ta, Nb, Mo, W, and V). The broader elemental system of RHEAs includes Group IV (Ti, Zr, and Hf), Group V (V, Nb, and Ta), and Group VI (Cr, Mo, and W). There are also non-refractory metals such as Al, Si, Co, Cu, or Ni; the number of studies on RHEA is steadily increasing, as shown in Figure 3. Overall, the number of publications shows a roughly exponential relationship with year trends:
FIGURE 3. (A). The number of RHEA publications from 2011 to 2021 and the frequency of RHEA occurrences involved. The number of RHEA articles can be described by exponential growth
FIGURE 4. In the statistical publications, the frequencies of RHEAs of 3,4,5,6,7 group elements, respectively (A), while the frequencies of RHEAs based on the most common 4 group element systems of Ta-Nb-Mo-W, Ti-Nb-V-Zr, Ti-Nb-Mo-V, and Ti-Ta-Nb-V are in (B).
Bio-HEAs are based on the study of RHEAs, and the selected metal elements require no cytotoxicity or low cytotoxicity. The following eight alloy compositions are commonly used: Ti, Ta, Nb, Mo, V, W, Hf, and Zr. The refractory high-entropy alloys with four or more group elements are usually designed based on Ta-Nb-Mo-W, Ti-Nb-Zr-Hf, Ti-Nb-V-Zr, Ti-Nb-Mo-V, Ti-Ta-Nb-Zr, and Ti-Ta-Nb-V, as shown in Figure 4. In addition, alloys can contain Al or Si to reduce the alloy density to improve Bio-HEAs performance, and there are also studies to improve the wear performance of Bio-HEAs by adding Cr elements (Tong et al., 2020).
Bio-HEAs have excellent mechanical properties, and their strong solid solution strengthening mechanism plays an important role. The phase formation mode of HEAs was investigated to explore the composition design of different HEAs and many empirical parameters, such as atomic radius difference, mixing entropy, valence electron concentration, mixing enthalpy, etc. These parameters are usually constructed based on different perspectives such as thermodynamics, lattice distortion, and electronic behavior to explain the stability of the solid solution phase in HEAs. These empirical parameters can distinguish solid solution from intermetallic compound phases, discriminate the formation of phases such as FCC/BCC/HCP, and predict the single-phase and multi-phase structures of HEAs [8]. The researchers have summarized a large amount of data and proposed some semi-empirical criteria for the formation of simple solid solutions:
(1) The mixing entropy (ΔSmix) is 12–17.5 J/(molK), and the calculation formula of ΔSmix is (Zhou et al., 2022):
where R is the gas constant, and ci is the mole fraction of the ith element.
(2) The mixing enthalpy (ΔHmix) is −15–5 kJ/mol, and the calculation formula of ΔHmix is (Zhou et al., 2022):
where cj denotes the mole fraction of the jth element; HAB is the enthalpy of mixing between the A and B elements.
(3) The atomic size difference δ ≤ 6.5%, and the calculation formula of δ is (Zhou et al., 2022):
where ri denotes the atomic radius of the ith component; is the molar average atomic radius.
In addition, the formulae for calculating the three relevant features of HEAs are added (Lilensten et al., 2018), including valence electron concentration (VEC), electronegativity difference (Δχ), and melting temperature (Tm), and the calculation formula is as follows:
Where ci denotes the atomic concentrations for the ith element, VECi is the valence electron concentration of the ith element. Tmi is the melting point and Pauling electronegativity of the ith element. The structure and properties of Bio-HEA elements are listed in Table 1.
Based on the six eigenvalues of the designed HEAs, Zhu et al. (Zhu et al., 2022) used an Artificial neural network (ANN) to count a dataset containing 529 HEAs. The Pearson correlation coefficients between the six eigenvalues are listed in Figure 5. The values in the matrix describe the correlation of the two eigenvalues, and the correlations quantitatively vary from 1 to −1, indicating a highly positive or negative relationship (After removing the autocorrelation value of 1, the matrix elements range from −0.65 to 0.65). For example, the atomic size difference is negatively correlated with the mixing enthalpy, which means that as the mixing enthalpy decreases, the atomic size difference appears larger. The electronegativity difference is positively correlated with the melting temperature. Overall, the absence of a strong positive or negative correlation matrix between any two features implies that each of these six feature values has a unique influence on the final properties of the alloy, which needs to be taken into account when designing new group element alloys.
FIGURE 5. Heatmap of the correlation matrix between six relevant features, including VEC, electronegativity difference, mixing enthalpy, atomic size difference, mixing entropy, and melting temperature (Zhu et al., 2022).
The solid solution-phase structure of Bio-HEAs is a hot topic of research. For conventional binary solid solution materials, the classical Hume-Rothery criterion predicts the phase composition of the alloy from the atomic size, electronic structure, and other elemental properties (Ye et al., 2016). The alloys should have a high mixing entropy, low atomic size difference, and mixing enthalpies with small absolute values to form solid solutions (Zhang et al., 2008). In addition to the Hume-Rothery criterion, materials workers have proposed many simple but practical parameters to determine the phase composition tendency of high entropy alloys according to the properties of the alloy’s atoms. From Figure 6, it can be seen that Ω and ϕ affect the phase of HEAs, respectively, and a single eigenvalue cannot predict the trend of solid solution phase formation of HEAs. Still, it should be combined with the interaction of multiple eigenvalues. For example, several criteria have been proposed by experts and scholars to predict the formation conditions of solid solutions effectively. Zhang et al. (Zhang et al., 2008) indicated that the favorable conditions for the formation of the solid solution phase are −20 ≤ ΔHmix ≤5 kJ/mol, δ ≤ 6.4, and 12 ≤ ΔSmix ≤17.5 J/(mol K). Guo et al. (Guo et al., 2013) demonstrated that the parameter ranges for forming the solid solution phase are −11.6 ≤ ΔHmix ≤3.2 kJ/mol and δ ≤ 6.6. Yang and Zhang (Yang and Zhang, 2012) noted that the favorable criteria for predicting the formation conditions of the solid solution phase are Ω ≥ 1.1 and δ ≤ 6.6. The possibility of forming a solid solution phase can be notably enhanced during the design process of HEAs, reflecting on the criteria as mentioned above.
FIGURE 6. The plot of (A) the Ω values versus phases and (B) the ϕ values versus phases for the HEAs. (C) The plot of the VEC versus ϕ for different HEAs. The FCC solid solution mainly forms around a VEC of 8.5, BCC around a VEC of 5, and HCP around a VEC of 2.8, each within a narrow band (Ye et al., 2016)
For the whole alloy system of HEAs, the valence electron concentration (VEC) is the key parameter affecting the crystallization of the solid solution phase in the absence of strong atomic size effects, and a generalization work on the impact of VEC on Bio-HEAs in the literature is presented. To reduce the difficulty of computational work caused by multicomponent alloys, we use the cluster formula approach A cluster formula approach to simplify the calculation based on chemical short-range orders (CSROs). We can consider the solute atoms with similar properties in a multicomponent alloy as a cluster and the remaining solvent atoms with similar properties as a class of gum atoms, and most of the HEAs can be expressed as [cluster](gum atom)x (Yang W. et al., 2022). In Bio-HEAs, the BCC solid solution structure is predominant, guided by Friedel oscillation theory, the gum atomic number is ideally calculated as x = 1–5, with a stable electronic structure. In the Bio-HEAs system, Ti, Zr, and Hf could be regarded as an averaged virtual element, M, since they are in the same group in the periodic table of elements, the value of VEC is four, and the ΔHTi-Zr, ΔHTi-Hf, and ΔHHf-Zr are zero. Ta, Nb, and V can also be considered an average virtual element, A, while Mo and V can be regarded as another average virtual element, B. We use MxAyBz to generalize all [Ti-Zr-Hf]x∼ (Ta-Nb-V)y∼(Mo-W)z, and based on existing studies, we make a classification including [Ti-Zr-Hf]x∼ (Ta-Nb-V)y [Ti-Zr-Hf]x∼ (Mo-W)z and [Ta-Nb-V]y ∼(Mo-W)z, and [Ti-Zr-Hf]x∼ (Ta-Nb-V)y∼(Mo-W)z, where x,y,z≠0. As can be seen from Figure 7, the VECs of the discussed Bio-HEAs range from 4.0 to 5.8, among which [Ti-Zr-Hf]x∼ (Ta-Nb-V)y fluctuate between 4.2 and 4.8 due to the generally low VECs of their constituent elements, and are primarily single BCC solid solution phases. The VEC of Bio-HEAs [Ta-Nb-V]y ∼(Mo-W)z in the high VEC element group is concentrated in the range of 5.2–5.6, mainly in the BCC phase and multiphase, where the multiphase components include Laves phase, BCC2 phase, B2 phase, FCC phase and other precipitated phases (Wang et al., 2020; Mao et al., 2022). The interesting point is that alloys of the system [Ti-Zr-Hf]x∼ (Mo-W)z hardly appear in the surveyed literature, implying that for Bio-HEAs, the V-subgroup elements Ta, Nb, and V with BCC lattice and high mutual solubility are indispensable.
FIGURE 7. Statistical correspondence between VEC and phase composition in Bio-HEAs, Bio-HEAs are classified by [Ti-Zr-Hf]x∼ (Ta-Nb-V)y [Ti-Zr-Hf]x∼ (Mo-W)z and [Ti-Zr-Hf]x∼ (Ta-Nb-V)y∼ (Mo-W)z, where x,y,z ≠ 0.
The relationship between VEC and the solid solution phase has been studied thoroughly throughout the high entropy alloy system. The VEC of the alloy plays a crucial role in determining the crystallinity of the solid solution phase, especially for single-phase solid solutions. As mentioned earlier, the predominance of the BCC phase in the reported Bio-HEAs is quite reasonable, as the alloy is based on the V-subgroup elements and the IV-subgroup elements (Ti, Zr, and Hf), which are also BCC-phase structures at high temperatures. However, they undergo isotropic transformations and are HCP structures at RT, like Ti and Zr, as shown in Table 1. The phases included in the high VEC Bio-HEAs vary depending on the elemental composition and ratio and the effects of the process. Since the elements used are BCC, the generated solid solution phases are still based on the BCC phase, and the synergistic precipitation phases contain other solid solution phases such as Laves phase, BCC2 phase, B2, and FCC phase (Wei et al., 2022b).
The molybdenum equivalent (Moeq) has been widely used to measure the β-phase stability in the multicomponent Ti-based alloy quantitatively. In addition, it has been shown that the Moeq parameter is also reliable for predicting the phase stability of solid solution in HEA, especially for the BCC/HCP phase (Wang et al., 2015); Ishida (Ishida, 2017) suggested a new Moeq based on the thermodynamic database of the Ti alloys, as shown in Eq. 7
Where [M] is the weight percent concentration of element M. The inclusion of Ti, Zr, Hf, Nb, Ta, V, Mo, and W, the basic elements of Bio-HEAs, implies that the Moeq index is a guide to determining the desired stability of the solid solution phase in Bio-HEAs. Yang et al. (Yang W. et al., 2022) used Moeq to predict the phase stability of BCC/HCP solid solution in the Ti-Zr-Hf-Nb-Ta HEAs system. According to Yang’s calculations, the values of Moeq for Ti-Zr-Hf-Nb-Ta HEAs were in the range of 13.5–21.1 wt%, representing a high trend of BCC solid solution phase formation, which is consistent with the actual results. Iijima et al. (Iijima et al., 2021) used the Moeq parameter to predict various characteristic quantities of solid solution formation, including ΔHmix, Ω, and δ parameters, as shown in Figure 8. The calculations included 1.5×107 Ti-Zr-Hf-Nb-Ta-Mo Bio-HEAs, and it was found that there was no clear relationship between the Moeq parameter and other eigenvalues. The Moeq parameter can be considered independent of other empirical alloy parameters, which can directly be predicted for the Bio-HEAs solid solution phase.
FIGURE 8. Relationship between Moeq and various empirical alloy parameters used to predict solid solution formation, including ΔHmix, δ (ΔHmix), Ω, and δ parameters in Ti-Zr-Hf-Nb-Ta-Mo alloys with ΔSmix ≥1.5 R (red dots). The green dot indicates the alloys whose Ti concentration was at and above 35 at% and considered the Ti-rich alloys. Hollow black circles (○) indicate the Ti–Zr–Hf–Nb–Ta–Mo alloy investigated in the present study. (A) Moeq vs ΔHmix, (B) Moeq vs δ(ΔHmix), (C) Moeq vs Ω, and (D) Moeq vs δ parameters (Iijima et al., 2021).
Bio-HEA has a “cocktail” effect and is characterized by a multi-component synergistic effect. The design and development of new Bio-HEAs biomaterials require continuous changes in elemental composition and content (Li J. et al., 2021; Ostovari Moghaddam et al., 2021). However, Bio-HEAs have too many permutations of elemental composition and content ratios, and the elements used contain and are not only Ti, Zr, Hf, Nb, Ta, Mo, V, and W, which are expensive. Therefore, direct experimental verification of Bio-HEA alloys’ design is laborious and expensive. DFT has become one of the main methods for exploring material properties in theoretical solid-state physics. Over time, DFT calculations have entered the Bio-HEA field, and the number of corresponding studies has gradually increased (Yang F. et al., 2022; Biermair et al., 2022). Simulations based on the first principles of DFT are an essential method for designing Bio-HEA compositions. They are widely used to predict material properties such as energy band density, electronic structure, and charge density from atomic and electrical scales (Yu et al., 2019; Zhao, 2020; Wu et al., 2022).
Troparevsky et al. (Troparevsky et al., 2015) evaluated the energy of formation of binary compounds by DFT calculations and therefore did not require experimentally or empirical inputs as shown in Figure 9. The model correctly ranks the combinations of elements of known single-phase HEAs based on statistics from numerous studies and eliminates combinations that are not single-phase. The constructed elemental matrix can predict all currently known single-phase HEAs. In addition, this method can predict feasible five-, six-, and seven-component alloys, thus guiding exploring new HEAs.
FIGURE 9. The DFT calculates the formation energy of the lowest energy structures in various binary relative to their phase separation into pure elements. If the numbers are in bold blue, the energies are evaluated relative to the respective solid solution (Troparevsky et al., 2015)
As the most important influencing factor of Bio-HEA biocompatibility, the selection of elements plays a significant role in the simulation design. Bai et al. (Bai et al., 2021) predicted the impact of Ti elements on the mechanical properties of iso-atomic NbMoTaW HEA from the first-principles calculation based on DFT. The phase structure, density, lattice constant, elastic, and electrical properties of NbMoTaW HEA by Ti elements were calculated. The energy band, total and partial densities of states, and charge density were calculated to investigate the strength and ductility enhancement mechanism of NbMoTaW alloy using Ti alloying. Tong et al. (Tong et al., 2020) used DFT calculations to simulate the effects of Ti, Zr, Cr, V, Hf, and Re additions on the properties of NbMoTaW HEAs and further analyzed the role played by various strengthening mechanisms. Chen et al. (Chen S. M. et al., 2022) used the first-principles approach combined with a thermodynamic model to study phase decomposition in alloys by considering HEA as various pseudo-binary systems, as shown in Figure 10, which predicts that phase decomposition in Hf-Nb-Ti-Zr alloys with a BCC structure occurs at temperatures below the critical temperature of 1298 K. The HEA decomposes most favorably into NbTa-rich and HfZr-rich BCC phases, while the BCC-rich HfZr phase is transferred to the hexagonal compact stacking structure (HCP) phase at low temperatures. (Chen X. et al., 2022; Chen Z. W. et al., 2022). In addition, the effects of solid solution and precipitation strengthening mechanisms on the strength of HEA were calculated based on the predicted phase decomposition results, combined with experimental data.
FIGURE 10. The final members of the lattice constants of the 1–4 (A) and 2–3 (B) pseudo-binary AxB1- x alloys as a function of the molar fraction. In subfigure (A), A represents one of the Hf-Nb-Ta-Ti-Zr elements, and B represents the other four elements. In subfigure (B), A represents two elements of Hf-Nb-Ta-Ti-Zr, while B represents the other three. The lattice constants that conform to Vegard’s law are shown as dashed lines (Chen S. M. et al., 2022)
In the simulation studies of HEAs, apart from DFT simulations, molecular dynamics also occupy a relatively large proportion, and molecular dynamics-based simulations are more reliable in analyzing the microscopic deformation mechanism of HEAs. Progress has been made in using molecular dynamics simulations to explain the deformation mechanisms and mechanical properties of 3D transition group HEAs (Liu et al., 2020). This approach has significant implications for predicting the performance of HEAs, especially for assessing complex service conditions of Bio-HEAs. Simulation methods still have great research potential in the composition design of Bio-HEAs, and more research is needed to discover the intrinsic connections.
With the increasing number of high-entropy components, the composition design becomes more and more complex, and the traditional empirical trial-and-error method, first-principles calculations, and molecular dynamics simulations introduced earlier gradually fail to meet the needs of the growing performance-oriented high-entropy alloy composition. Build machine learning models to predict the performance of various target alloys for fast and cost-effectively solved material performance evaluation (Kumar et al., 2021). Using a multi-objective genetic algorithm to find the Pareto Frontier of multi-objective performance in the prediction results can solve the problem of searching for elements of material composition (Bao et al., 2022). Combined with adaptive iterative methods for model uncertainty-based material screening, optimized material compositions or processes can be identified, guiding materials research (Chen H. et al., 2022; Thebelt et al., 2022). In the context of multi-objective performance requirements, the application of machine learning to the field of high-entropy alloys is particularly critical, such as the co-optimization of strength and corrosion resistance of high-strength and corrosion-resistant high-entropy alloys, the co-optimization of density and strong toughness properties of lightweight high-entropy alloys, the co-optimization of high-temperature strength and oxidation resistance properties needed for refractory high-entropy alloys, and the co-optimization of biocompatibility, mechanical properties matching those of living organisms, and excellent corrosion resistance required for biological high-entropy alloys.
Machine learning is applied to the strength and hardness properties of HEAs with data and set samples, either derived from experimental studies or obtained with the help of computational simulations (Bakr et al., 2022; Chen Z. W. et al., 2022; Hou et al., 2022). The research mainly focuses on machine learning to accurately predict the strength and hardness properties of new alloys and to guide the design of alloy compositions based on the property prediction results. Klimenko (Klimenko et al., 2021) and Bhandari (Bhandari et al., 2021) et al. developed support vector machine and random forest machine learning models for accurate prediction of yielding of HEAs with an accuracy of more than 95% based on a sample of high-entropy alloy data with characteristic parameters such as composition, modulus, density, mixing entropy, and atomic radius difference of HEAs as inputs. Several well-known machine learning models, including a radial basis function kernel (svr. r), a regression tree model (cart), a back propagation neural network model (bpnn), and a k-nearest neighbor model (knn) to produce a non-convex input/output fitness function to estimate the hardness (Wen et al., 2019). As shown in Figure 11, 42 newly synthesized HEAs were designed based on machine learning and experimental feedback results, with 35 of them having alloy hardness values higher than the best values in the training dataset.
FIGURE 11. Test error evaluation of three different models, (A) a holdout method and (B) bootstrapping methods, indicating sur. r outperforms the bpnn and knn model. (C) The predicted values as a function of the measured values of the svr. r model for training data (randomly chosen 70%) and testing data (the rest 30%) (Wen et al., 2019)
In addition to the prediction of properties such as strength and hardness of HEAs, machine learning has also done a lot of work on elastic properties, which are the most critical concern for medical high-entropy alloys, to achieve the design of Bio-HEAs compositions corresponding to modulus and strength requirements based on the model’s prediction of elastic properties. Chanda et al. (Chanda et al., 2021) trained a neural network model using 140 samples of high-entropy alloy data with seven characteristic quantities such as electronegativity difference, mixing enthalpy, and mixing entropy as inputs and predicted the alloy elastic modulus with an accuracy of 94%. Roy et al. (Roy et al., 2020) trained gradient boosted tree regression models based on 89 high-entropy alloy data samples with ten empirical parameters as input characteristic parameters to predict the elastic modulus for 26 equimolar high-entropy alloys with low, medium, and high entropy compositions of the MoTaTiWZr system, and the agreement with the experimental results was high. The correlation coefficients revealed that the enthalpy of mixing and alloy melting point is the most critical for the prediction of elastic modulus, which provides a theoretical database for the subsequent design of Bio-HEAs with specific Young’s modulus to solve the stress shielding problem.
As a new type of medical alloy, Bio-HEAs have the characteristics of biosafety, high strength, high corrosion resistance, high wear resistance, etc. It has recently become the most promising medical metal material for research (Liu et al., 2022b; 2022a). Biocompatibility is the focus of attention for Bio-HEAs in clinical applications, but the long development cycle of biomaterials (about 20 years) limits the speed of development of new materials. Rapid interaction between Biological Omics and Material attributes through machine learning will significantly shorten this process (Basu et al., 2022). It revealed the relationship between biological data and material properties through the concept of biomaterialomics, as shown in Figure 12. Therefore, material design measurement methods that integrate machine learning, search algorithms, and adaptive iteration are essential to guide the efficient design of Bio-HEAs with small data samples, large cost space, and multi-objective performance requirements.
FIGURE 12. Introducing the concept of biomaterialomics, an interdisciplinary study unraveling the relationship between biological data and material properties (Basu et al., 2022).
Due to the frequent occurrence of diseases, and other human injuries, the demand for implant materials has increased, and more attention has been paid to medical metal materials. In contrast, high-entropy alloys have become one of the most promising new medical materials in recent years due to their excellent performance and have been explored and researched by many scholars at home and abroad (Nagase et al., 2020). Bio-HEAs use Ti, Ta, Zr, Nb, and Hf elements with high biosafety and good biocompatibility. As a new bio-alloy material, Bio-HEAs require a series of biosafety assessments before they can be implanted in organisms, and the common factors affecting the biology of biomedical implants are shown in Figure 13. For Bio-HEA to be truly used in the clinical field as a biomedical implant, its biocompatibility needs to be experimentally proven by the mechanical, physical, and degradation properties, by sterilization (freeing the implant surface from all types of microorganisms), by toxicological aspects (examination and treatment of any toxins or toxic substances), by surgery, implant site and load-bearing capacity (Davis et al., 2022). Based on the existing research work, this chapter summarizes the biocompatibility performance of Bio-HEAs in terms of their cytocompatibility and corrosion resistance in physiological solutions.
FIGURE 13. The primary factors are affecting the functional biocompatibility of a biomedical implant.
Most of the research on Bio-HEA biocompatibility currently focuses on evaluating the cytocompatibility of HEA alloys by the direct cell contact method (Wang J. C. et al., 2022; Wei et al., 2022a; Guo et al., 2022). The cytocompatibility of alloy materials includes cell adhesion ability and cytotoxicity (Ma N. et al., 2020); cell adhesion is essential to maintain the stability of tissue structure and is a regulator of cell motility and function, with significant effects on cell proliferation and differentiation (Yang et al., 2011). Cytotoxicity is an indicator to assess the biological activity of cells on the surface of the alloy, which is one of the critical indicators for the in vitro evaluation of Bio-HEAs. The commonly used cell lines are human aortic smooth muscle cell, osteoblast, human osteosarcoma cell, human epithelial fibroblast, L929 mouse fibroblast cells, and Mouse embryo osteoblast precursor cells。
Currently, the cytotoxicity tests on Bio-HEAs are conducted by culturing cells in vitro and measuring their survival rate on the alloy surface to assess their biological properties. Todai et al. (Todai et al., 2017) cultured human osteoblasts on the surface of TiNbTaZrMo Bio-HEA, which exhibited superior biological activity to pure Ti. Yang et al. (Yang W. et al., 2022) used MC3T3-E1 cells on Ti20Zr20Hf20Nb20Ta20 (Alloy-I), Ti25Zr25Hf25Nb12.5Ta12.5 (Alloy-II), Ti27.78Zr27.78Hf27.78Nb8.33Ta8.33 (Alloy- III) and Ti6Al4V substrates were incubated to evaluate the biocompatibility of Ti-Zr-Hf-Nb-Ta HEA, as shown in Figure 14. The biological activities of HEA and Ti-6Al-4V were observed by fluorescence staining after 72 h of culture, as shown in Figure 14. It was found that a large number of MC3T3-E1 cells adhered to the surface of HEA and Ti-6Al-4V with a high survival rate of adherent cells, indicating that MC3T3-E1 cells had a high survival rate and good initial adhesion on this HEA. In addition, the survival numbers of cells in HEA and Ti-6Al-4V were counted on the first, second, and third days, respectively, and no statistically significant differences were found, indicating that TiZrHfNbTa HEA has the same level of biocompatibility as Ti-6Al-4V. Iijima et al. (Iijima et al., 2021) used mouse primary osteoblasts cultured on TiZrHfNbTaMo surface for 24 h. After 24 h in a humidified atmosphere of 5% CO2, the cells were fixed in methanol, stained with 5% Giemsa aqueous solution for staining, and observed under a light microscope, as shown in Figure 14. In addition, Bio-HEAs such as TiZrNbHfTa (Braic et al., 2012; Motallebzadeh et al., 2018) have good mechanical properties and excellent friction and wear resistance, showing close to or even better biocompatibility than conventional medical alloys such as Ti-6Al-4V.
FIGURE 14. Live/dead staining of MC3T3-E1 cells on (A) Alloy-I, (B) Alloy-II, (C) Alloy-III, and (D) Ti64 after 72 h of incubation. (E) The proliferation of MC3T3-E1 cells on Alloy-I, Alloy-II, Alloy-III, and Ti64 after 1, 3, and 5 days of cell culture, respectively (Yang W. et al., 2022). Biocompatibility of the arc-melted isoatomic TiNbTaZrMo (TZHNTM-Eq) and non-isoatomic Ti28.32Zr28.32Hf28.32Nb6.74Ta6.74Mo1.55 (TZHNTM-3) and SUS316L stainless steel, ASTM F1537-08 of Co-Cr-Mo alloy and commercially pure titanium (CP-Ti) were used as references. (F) Quantitative analysis of the density of osteoblasts cultured on the fabricated specimens by staining images with Giemsa and (G) fluorescent images of osteoblast adhesion on the fabricated specimens (Iijima et al., 2021).
Bio-HEAs have been shown to have excellent cytocompatibility. Still, the application of the material should also consider the working service environment, where all metals and alloys are subject to corrosion when in contact with body fluids because the body environment is very aggressive due to the presence of hydrogen ions and chloride ions, and proteins (Khodaei et al., 2020). Bio-HEAs, as metal implants, undergo various chemical reactions with body fluids, where the metal components of the alloy are oxidized to ionic form and dissolved oxygen is reduced to hydroxide ions, causing various forms of corrosion damage (Cui et al., 2022). For the study of the corrosion resistance of Bio-HEAs, the solutions commonly used to simulate the physiological environment include saline (0.9% NaCl solution), Hank’s solution (with a composition of 0.137 M of NaCl, 5.4 mM of KCl, 0.25 mM of Na2HPO4, 0.44 mM of KH2PO4, 1.3 mM of CaCl2, 1.0 mM of MgSO4, and 4.2 mM of NaHCO3, pH = 7.4), phosphate buffer saline (PBS) and fetal bovine serum (FBS).
Potentiodynamic polarization curve measurement is one of the most widely used measurements in electrochemical corrosion (Gu M. et al., 2022; Li et al., 2022), which can provide information on the corrosion rate and corrosion mechanism of bio-alloy materials under a simulated physiological environment and evaluate the bio-suitability of Bio-HEAs by chemical corrosion conditions. In the corrosion reaction, corrosion potential (Ecorr), corrosion current density (Icorr), and AC impedance are essential parameters for analyzing the corrosion resistance of the material, and the relevant parameters of some Bio-HEAs are listed as shown in Table 2. For Bio-HEAs with Ti and Ta elements, their lower Ecorr indicates that they will both readily form protective passivation layers (usually oxides) (Zheng et al., 2018). Most Bio-HEAs have no pitting reaction until 2V, which means promising applications in human environments around 0–0.2 V. No pitting and no localized corrosion due to the breakdown of the protective passivation films, dramatically increasing the lifetime of alloy implants (Hwang et al., 2019; Li T. et al., 2021). The corrosion current density indicates the corrosion rate of the material. The lower the Icorr value, the lower the corrosion rate of the material, and the low Icorr value of Bio-HEAs indicates that it is preferable in medical alloy implant applications (Zhu et al., 2021; Feng H. et al., 2022).
The Ti-Zr-Ta-Hf-Nb system of Bio-HEAs has promising properties as a novel superior metallic biomaterial with an ideal combination of wear resistance, wettability, pitting, and resistance to general corrosion outperforming the conventional metallic biomaterials 316L, CoCrMo, and Ti-6Al-4V in these aspects (Cui et al., 2022). Wang et al. (Wang and Xu, 2017) investigated the electrochemical behavior of HEA in PBS by kinetic potential polarization test. They initially evaluated its corrosion resistance in a physiological environment and compared it with Ti6Al4V, 316L SS, and CoCrMo alloys, as shown in Figure 15. TiZrNbTaMo HEA exhibits an extensive passive plateau in the curve up to 1.2 VSCE without pitting or turning passivation. Such a response is similar to that of Ti-6Al-4V, except for a more positive Ecorr and a slightly higher pass for Ti-6Al-4V. TiZrNbTaMo HEA exhibited excellent corrosion resistance comparable to Ti6Al4V alloy and significantly better-pitting resistance than 316 L SS and CoCrMo alloys in a physiological environment simulated by PBS media. TiZrNbTaMo HEA exhibited excellent corrosion resistance comparable to Ti6Al4V alloy and significantly better-pitting resistance than 316 L SS and CoCrMo alloys in a physiological environment simulated by PBS media. Gurel et al. (Gurel et al., 2021) studied the biocompatibility of three TiTaHf-based high-entropy alloys, namely TiTaHfNb, TiTaHfNbZr, and TiTaHfMoZr alloys, using FBS as a test environment to compare their corrosion resistance as bone implants by the level of ions released from HEA etched by FBS solution, TiTaHfNb HEA exhibited the highest corrosion resistance to FBS, as shown in Figure 15. In addition, after 28 days of immersion in FBS solution, the three HEA were found to produce hydroxyapatite on their surfaces when in contact with FBS, further demonstrating their great potential for use in orthopedic implants.
FIGURE 15. (A) Potentiodynamic polarization curves of arc-melted TiZrNbTaMo HEA, as well as Ti-6Al-4V, 316L, and CoCrMo alloys in PBS for comparison of biocompatibility (Wang and Xu, 2017). Ti2p peaks after etching times of 360 and 1,080 s immersed in FBS for 28 days: (B) and (C) for TiTaHfNb, (D) and (E) for TiTaHfNbZr, (F) and (G) for TiTaHfMoZr (Gurel et al., 2021).
In addition to applications in human implants, Bio-HEAs have great potential for medical devices. Conventional medical devices, despite their strict sterilization procedures, are still subject to bacterial infections, which greatly threaten the health of patients (Grischke et al., 2016; Zhang et al., 2021). Antibacterial metals and alloys are more suitable metal materials for medical devices, prepared by elemental alloying and processing processes, exhibiting strong inhibition of bacterial adhesion, growth, and proliferation. Currently, common antibacterial medical devices are antibacterial stainless steel, antibacterial magnesium alloy and antibacterial titanium alloy, and the elements that play an antibacterial role are mainly Cu, Ag and Zn (Chopra, 2007; Tie et al., 2013; Nan and Yang, 2016). Biological HEAs with antibacterial properties usually contain these antibacterial elements, and Chen et al. (Chen C. et al., 2022) designed CrFeNiCuSi HEA with antibacterial properties, which achieved 97.45% inhibition of E. coli. Similarly, the synergistic effect of copper ions and copper-rich phase greatly improved the antibacterial performance of Bio-HEAs, and CoFeCrCu HEA showed superior antibacterial performance with 99.97% inhibition of E. coli and 99.96% inhibition of Staphylococcus aureus after 24 h, much higher than conventional antibacterial alloy materials (Ren et al., 2022).
The antibacterial mechanism exhibited by Bio-HEAs containing Cu elements has been explored and verified (Sarell et al., 2010; Squitti et al., 2015; Zhang et al., 2019). On the one hand, the electrostatic force of Cu2+ can directly disrupt the adhesion of bacteria and rupture their cell walls, leading to cell rupture and death. On the other hand, in HEA, Cu and other elements (such as Fe) form a potential difference to form a miniature galvanic cell, releasing a large amount of Cu2+, which further exerts the bactericidal effect of Cu ions. In addition, according to Ren et al. (Ren et al., 2022), direct contact of bacteria with the copper-rich phase in Bio-HEAs produces an effective concentration of ROS (H2O2) during incubation, which induces oxidative stress, resulting in an antibacterial effect.
HEAs with antibacterial ability not only have strong and long-lasting bactericidal ability but also have good corrosion resistance and mechanical properties, indicating that Bio-HEAs also have great potential in the field of medical devices. Bio-HEAs containing Cu ions or Ag ions show stronger antibacterial ability, but the release of Cu2+ and Ag + may cause some damage to human body, which requires finding the right combination of elements to make Bio-HEAs have antibacterial ability and at the same time have basically no side effects on human body.
In addition to the biological safety of the material, meeting specific medical functions is a fundamental requirement of Bio-HEA. The implant generally serves as a replacement for damaged bone or as extra support. For different disease sites, an alloy with mechanical properties appropriate to the tissue is required. The mechanical match between the material and the biological organism during the implantation and functionalization of the material also influences the service effect of the implant in the damaged area. Too low modulus of elasticity can reduce the medical therapeutic impact of the alloy, while the too high modulus of elasticity can lead to stress shielding problems (Uppal et al., 2022; Xing et al., 2022). Therefore, Bio-HEA should not be pursued for high-strength mechanical properties but should be consistent with human tissues, close to or complete the therapeutic effect before failure. The natural selection of Bio-HEAs for implants is due to the multiple biomaterials advantages, including high strength, low density (high specific strength), high corrosion resistance, complete inertness to the body environment, and enhanced biocompatibility, low modulus, and increased capacity to join with bone and other tissues. These properties fit with the specific properties of biomedical metal materials, which means there is a good potential for its application in the medical field.
From an engineering perspective, the multi-component nature of HEAs predestines the strengthening mechanism of HEAs to be equally multidimensional. Current research on HEAs is characterized by numerous discoveries, intense discussions, and illuminating scientific research questions (Chen X. et al., 2022; Jin et al., 2022). Strength and ductility are widely studied as core mechanical properties of metallic materials. Complex alloying and thermo-mechanical processing affect the microstructure of HEAs, which has a dramatic effect on strength and ductility (Wu and Fan, 2020; He et al., 2021).
Several factors may influence the strength and ductility of Bio-HEA. Undoubtedly, the elemental composition is the most critical factor. Moreover, the strength and ductility may be changed by various factors such as microstructure, processing method, and post-heat treatment (Liu Z. et al., 2022; Jin et al., 2022). At the same time, these influencing factors interact with each other. For example, the microstructure manifests the combined effect of elemental composition and processing routes of Bio-HEAs. This composition-processing route-microstructure-property study idea, widely applied in conventional alloys, is also applicable in HEAs alloy systems. Still, the challenges are enormous, and the verification is more complex.
The elements composition of Bio-HEAs has the most critical effect on its strength and ductility. First, alloying elements determine the elastic behavior and atomic interactions of Bio-HEA, affecting the strength and ductility of the alloy at the atomic level. In addition, the ratio of elements can determine the phase composition and fraction of Bio-HEAs. In multi-component Bio-HEAs, the type and content of modulating elements can have a significant impact on the mechanical properties of the HEA, and common element additions include metallic and non-metallic elements (Tancret, 2021).
As the most common element in Bio-HEAs, Nb can provide an effective strengthening effect. The strengthening effect of Nb is due to its large atomic radius, which produces severe lattice distortion when added. It is widely used as a precipitation hardening element in the Bio-HEAs system (Li W. et al., 2021). Like Nb, Ti element with a larger atomic radius also strengthens solid solution in Bio-HEAs (Zhang et al., 2017). Still, the difference is that the strengthening effect of Ti on Bio-HEAs occurs mainly at low Ti content. Low Ti element concentration promotes solid solution formation, strengthened BCC phase, and hard Laves phase. In contrast, high Ti concentration leads to the precipitation of intermetallic compounds in HEAs to embrittle the alloy (Zhou et al., 2007). The strengthening mechanism of element Ta (Mohd Pauzi et al., 2016) for Al0.5FeCrNiMnCo (the primary phase is BCC + FCC) is mainly characterized by the precipitation strengthening effect and the presence of more Laves intermetallic phase precipitation. In addition, the strengthening effects of W (Waseem and Ryu, 2017), Zr (Yurchenko et al., 2017), and Cr (Stepanov et al., 2015) in Bio-HEAs were analyzed separately, and the compressive and tensile strengths increased to varying degrees with the addition of small amounts of the elements. Still, their strength benefits were compromised as the elements were alloyed. On the other hand, ductility tends to be the opposite of strength, and depending on the type of alloy, strength and ductility may vary roughly linear or nonlinearly with element concentration.
Small amounts of non-metallic elements, such as O, B, C, N, and Si, are usually added to Bio-HEAs to improve the overall performance of the alloy. According to the traditional experience, adding C elements to steel materials can greatly improve the mechanical properties of steel, and this method is also applicable to Bio-HEAs. The trace addition of C elements will form MC-type alloy carbides on the BCC matrix of Hf0.5Mo0.5NbTiZr, and the work-hardening ability and plastic strain of the alloy is enhanced (Gao et al., 2021). Chen et al. (Chen et al., 2018) investigated the effect of O element content on the microstructure and compressive properties of ZrTiHfNb0.5Ta0.5 Bio-HEAs. O atoms were present in the lattice of ZrTiHfNb0.5Ta0.5 Bio-HEAs and did not precipitate the MO alloy oxide phase. With the increase of the O element, the interstitial solid solution strengthening effect of the O atom increases, and the yield strength of the alloy gradually increases but the plasticity decreases. Further, Lei et al. (Lei et al., 2018) used ordered oxygen complexes to replace O atoms in the gap enhancement, changed the dislocation shear mode from planar slip to wave slip through ordered gap complexes, and promoted double cross-slip through the formation of Frank-Read sources (a mechanism to explain the generation of multiple dislocations), thus promoting dislocation proliferation, deformation, and stretching to achieve a massive increase in strength and plasticity of TiZrHfNb Bio-HEAs, as shown in Figure 16, the tensile strength is enhanced (by 48.5 ± 1.8 percent), and ductility is substantially improved (by 95.2 ± 8.1 percent). As with metallic elements, small amounts of specific non-metallic elements have a strengthening effect on Bio-HEAs, while transitional doping deteriorates the strengthening effect.
FIGURE 16. (A) Room-temperature tensile stress-strain curves for TiZrHfNb (TiZrHfNb)98O2, and (TiZrHfNb)98N2 HEA. The inset shows the corresponding strain-hardening response (dσ/dε) (B) Variation in strength and ductility of the HEA presented here, relative to several established high-performance alloys (Lei et al., 2018).
Processing is another crucial factor affecting the strength and ductility of the alloy under the premise that the compositions of the Bio-HEAs group elements are clearly defined. Common preparation methods for Bio-HEAs include vacuum arc melting (Chen et al., 2016), electromagnetic induction melting (George et al., 2020), powder metallurgy techniques (Hu et al., 2022), magnetron sputtering (Dvurečenskij et al., 2022), laser electron beam melting (Arif et al., 2022), and 3D printing additive manufacturing (Wang S. et al., 2022). Smelting is the most common preparation method for Bio-HEA, followed by powder metallurgy and additive manufacturing (Arif et al., 2021). The preparation of homogeneous bulk bioethanol by conventional arc melting is challenging, it requires several post-processing (such as remelting), which is a complex process and only yields samples of simple shapes (Cieslak et al., 2019; Güler et al., 2022). As a fabrication process that overcomes the limitations of traditional machining methods, additive manufacturing (AM) is considered as an advanced machining method for generating low-defect HEA specimens, which has the advantage of producing parts with complex geometries with high precision and achieving large-scale customization to maximize material, energy and time savings (Cui et al., 2021; Du et al., 2021). Recently, AM techniques commonly used for Bio-HEA preparation include directed energy deposition (DED) and powder bed fusion (PBF) (Lin Y. C. et al., 2021; Hassan et al., 2021). Directed energy deposition (DED) is an advanced preparation method based on powder feeding technology that allows higher precision shape customization. The advantages of DED are material design, preparation of different types of coatings and fast repair (Yadav et al., 2021; Yang F. et al., 2022). In contrast, selective laser melting (SLM) and selective electron beam melting (SEBM) offer higher processing accuracy and minimal surface roughness. In addition, in PBF technology, due to the extremely high cooling rate (105–107 K/s), smaller microstructures can be obtained compared to the conventional casting process (100 K/s), which greatly improves the mechanical properties of the part (Huang et al., 2021; Muftah et al., 2021; Ocak and Goller, 2021). For HEAs, the high cooling rate can greatly inhibit the segregation of elements and make it easier to achieve a solid solution structure with a strengthening effect (Zhu et al., 2021).
A change from one preparation technique to another can dramatically affect strength and ductility (Feng et al., 2021, Feng et al., 2022 J.; Wei et al., 2022b). Even for the same technology, the influence of the processing route on the product forming has to be taken into account. Adjusting the processing time and the processing temperature can cause changes in the microstructure and internal defects of the alloy. In addition, the as-prepared alloy samples may have undergone extensive post-treatment processes, such as homogenization, forging, cold and hot rolling, annealing, tempering, aging, etc. This complex process situation, coupled with the elemental diversity of Bio-HEAs, makes it nearly impossible to quantify the effect of processing on the strength and ductility of the alloy, and more precise characterization and analytical tools are needed to address this issue.
The microstructure that most directly affects the mechanical properties of Bio-HEAs also has various forms, including phase composition, grain size, dislocation density, twinning, layer dislocations, size and distribution of precipitated phases, etc. The main phase structures in Bio-HEAs mentioned earlier include BCC, FCC, HCP, Laves, B2, and IC, and MB, MC, MN, MO, and MSi formed by doping with non-metallic elements, as shown in Figure 7. Most single-phase Bio-HEAs are BCC structures, where the elements randomly occupy lattice positions and start BCC phases under the effect of high entropy. The atomic packing density of the BCC phase is 68%, smaller than that of the FCC phase (74%) and the HCP phase (74%). The spatial structure of the BCC phase structure has a higher gap, which makes it easier for small radius solute atoms to enter between the BCC lattice to form interstitial reinforcements, such as the non-metallic elements mentioned previously, without changing the phase structure. Dual solid solution phases Bio-HEAs involve BCC1+BCC2, BCC + HCP, BCC + FCC, BCC + Laves, BCC + B2, and BCC + TC, usually with the B2 phase or BCC phase as the matrix, and the second phase is induced in the alloy by composition adjustment or heat treatment. The multi-phase structure of Bio-HEAs still mainly uses a single-phase or a double phase as the dominant phase and the rest of the phases as the precipitating phases, which play a role in regulating the strength and ductility of Bio-HEAs. To date, there have been many studies on reconciling the strength and ductility of HEAs, including inducing transformation-induced plasticity (TRIP) by stressing (Radi et al., 2022), and creating specially tailored eutectic structures (Ostovari Moghaddam and Trofimov, 2021), introducing interstitially ordered oxygen complexes, and producing nanoscale precipitates or lamellar structures.
Even though Bio-HEAs have a large scope for compositional tuning, it is still difficult to design materials with both high strength and good ductility. At the same time, a large number of researchers have also worked on the strength-ductility conflict, for example, in the case of sacrificing a small amount of strength, the ductility can be greatly improved, or a certain ductility can be guaranteed so that the strength of the alloy is greatly enhanced. Various selectable alloying elements, sophisticated processing methods, and microstructure design allow Bio-HEAs to have more strength-ductility options to design specific alloys according to actual needs (Sheikh et al., 2016).
Elastic properties characterize the reversible stress-strain of material when sufficient load is applied (Lv et al., 2022). These parameters are essential for analyzing complex mechanical properties (e.g., ductile-brittle behavior), and studying the elastic properties of Bio-HEAs is necessary because they open up an almost unlimited compositional space in material design. And as a new medical alloy material, elastic properties are also an essential factor in determining the application prospects of Bio-HEAs.
Metals and alloys have a long history of use as bone implants. Still, implants made of traditional medical metal material types are usually much harder than natural bone, leading to stress shielding effects, which is the primary factor in bone resorption and eventual failure of such implants (Wang et al., 2016). The stress shielding effect refers to the fact that when metal implants are aligned parallel to human bone, the modulus of the metal is often more than ten times that of the bone. The huge difference in modulus makes the stresses mainly borne by the alloy. At the same time, the human bone is left unstressed, or under less stress for a long period, bone regeneration is inhibited, and bone atrophy occurs, which leads to implant failure. The modulus of elasticity of human cortical bone (dense bone) is 3–30 Gpa, while the modulus of elasticity of bone trabeculae (cancellous bone) is even lower at 0.02–2 GPa (Wu et al., 2018). Most current metallic materials for implants have a much higher modulus than bone. For example, Ti-6Al-4V has a modulus of about 110 Gpa, while CoCrMo alloys have a modulus of 210 GPa (Che Ghani et al., 2020) and Table 3 shows the modulus of elasticity of various biomedical alloys compared to bone. Therefore, new materials with low Young’s modulus and other bone-adapted mechanical properties need to be developed to avoid stress shielding at the bone-implant interface.
A high-priority goal in designing new metallic materials for load-bearing implant applications is to reduce Young’s modulus in the major loading direction approximating that of cortical bone. In recent years, some biological HEAs and MEAs have been developed (Wang et al., 2017; Wang L. et al., 2018), and the elastic moduli of TiZrHf (HCP), TiZrNbHfTa (BCC), TiZrNbHf (BCC), and TiZrTaHf (BCC) alloys are 111, 103, 83, and 86GPa, respectively (Hu et al., 2021). The elastic modulus of these alloys is relatively low, about half that of CoCr alloys and stainless steel, which is closer to human bone and can effectively reduce the effect of stress shielding.
To further verify the advantages of elastic properties, in addition to the research on Young’s modulus, the current research on Bio-HEAs involves single-crystal elastic constant, polycrystalline elastic modulus, and Debye temperature (Huang and Vitos, 2022). The single-crystal elastic constant Cij describes the response of a material to an external load and can be determined from the stress-strain and or energy-strain relationships (Chanda et al., 2021). The single-crystal elastic constants and polycrystalline elastic moduli of Bio-HEAs can be studied experimentally (in situ neutron diffraction, tensile tests, and ultrasonic resonance frequency techniques) and computationally (first-principles calculations) (Diao et al., 2017). For Bio-HEAs cubic crystals, there are three fundamental, independent modes of elastic deformation, namely expansion due to hydrostatic stress, shearing along the cubic crystal axis on the cubic plane of the crystal, and the shear resulting from the plane rotated 45° about the cube axis from the cube face is sheared in a direction perpendicular to that axis. The single-crystal elastic constants describe the relationship between the measured h, k, and l-specific elastic lattice strains, using neutron diffraction and the macroscopic stresses applied to the polycrystalline HEA material. The polycrystalline elastic modulus is calculated by the Voigt–Reuss–Hill method based on the single-crystal elastic coefficients to obtain Young’s modulus E, bulk modulus B, shear modulus G, microhardness H, Poisson’s ratio υ, and Cauchy pressure of Bio-HEAs, and thus further evaluate the mechanical properties of Bio-HEAs such as strength, hardness, and elastic anisotropy.
The elastic anisotropy is related to the formation of microcracks in the material and impacts the mechanical durability of Bio-HEAs. The study of the elastic anisotropy of Bio-HEAs was examined theoretically and experimentally (Duesbery and Vitek, 1998; Fazakas et al., 2014; Tian et al., 2014; Dirras et al., 2016; Lilensten et al., 2018; Laplanche et al., 2019; Akdim et al., 2021; Raturi et al., 2022). Virtual crystal approximation (VCA), coherent potential approximation (CPA), and special quasi-random structure (SQS) simulations by calculating the BCC HEA with elastic stiffness constants (Fazakas et al., 2014; Tian et al., 2014). For example, Tian et al. (Tian et al., 2014) showed that molybdenum-containing alloys are almost isotropic compared to molybdenum-free alloys of the TiZrNbMoVx system. By studying the single-crystal elastic properties of 21 Bio-HEAs, as shown in Figure 17, Schonecher et al. (Schönecker et al., 2022) verified that the valence electron number has a dominant effect on the elastic anisotropy and crystal orientation of low Young’s modulus and high torsional modulus in HEAs, and discussed the potential of using single crystals or woven aggregates to reduce Young’s modulus in Bio-HEAs.
FIGURE 17. Higher single crystal elastic anisotropy for the present HEAs typically implies a lower minimum Young’s modulus. For comparison, the vertical yellow bars indicate the targeted Young’s modulus of cortical bone (Schönecker et al., 2022).
When conventional biomedical alloys are implanted, friction and wear are usually accelerated due to the corrosive physiological environment, resulting in considerable corrosion and wear of the material, severely reducing the service period (Chiba et al., 2007). Therefore, biomedical metal implant alloys need excellent biocompatibility, high corrosion, and good wear resistance. However, due to the low wear resistance of Ti-based alloys, particle diseases resulting from the wear debris may occur when they are performing in physiological environments (Long and Rack, 1998; Geetha et al., 2009). Ti-Zr-Nb-based refractory HEAs typically exhibit a body-centered cubic (BCC) solid solution structure and thus have higher hardness, yield strength, and wear resistance than Ti-6Al-4V, making them highly resistant to plastic deformation and fracture under high loads.
The wear resistance of Bio-HEAs is the key to their service life (Moazzen et al., 2022; Zhang et al., 2022). Poulia et al. (Poulia et al., 2016) analyzed the influence mechanism of the solid solution phase on relative wear resistance. They found that MoTaWNbV high-entropy alloy with a single-phase BCC structure could effectively absorb the energy during wear due to the abundant slip system in the BCC structure of the alloy, the BCC structure of this alloy possesses more slip systems. However, MoTaNbZrTi high-entropy alloy with a dual-phase BCC + HCP structure will form an oxide on the surface during wear to lubricate the surface and improve wear resistance. Both alloys have higher wear resistance than conventional high-temperature alloys. Figure 18 shows the wear resistance of MoTaWNbV and MoTaNbZrTi alloys compared to conventional high temperature alloys. To further investigate the wear resistance of Bio-HEAs under physiological environments, some researchers used PBS solutions to test the wear resistance of TiZrHfNbFex HEAs under real service conditions (Wang W. et al., 2022). Figure 18 shows the fatigue wear of Bio-HEAs under applied load, where the deformed layer of the alloy surface is stripped off, and the exposed fresh metal surface reacts with the PBS solution and exhibits better resistance to wet wear than Ti-6Al-4V. In addition, the content of Fe elements was found to affect the wear mechanism of these Bio-HEAs, from abrasive and corrosive wear of Fe0 to Fe1 alloy to fatigue and corrosion wear of Fe1.5 and Fe2 alloys after sliding in PBS solution.
FIGURE 18. (A, B) Comparison of frictional properties of MoTaWNbV high entropy alloy and Inconel 718 conventional high-temperature alloy, using aluminum oxide and steel balls as counter body materials (Poulia et al., 2016). C–I SEM images of worn-scar surfaces of the Ti-Zr-Hf-Nb-Fex HEAs under PBS wet wear conditions (Wang et al., 2022c).
In conclusion, Bio-HEAs have potential applications in the biomedical field but are still in the laboratory stage. The immature preparation process, high cost, and clinical experiments have not yet been realized, making it difficult for Bio-HEAs to be widely used. The design theory, mechanical properties, and biocompatibility of Bio-HEAs are summarized, and their future directions are partially discussed as follows:
(1). There are currently relatively systematic design theories for HEAs, including semi-empirical guidelines for characteristic quantities such as mixing entropy, valence electron concentration, atomic radius difference, mixing enthalpy, and electronegativity. However, a great deal of validation work is still required for Bio-HEAs to design novel alloys. A simulation is an essential approach for the composition design of Bio-HEAs to predict material properties from electronic and atomic scales. Combined with machine learning, it can significantly reduce the time and cost of designing Bio-HEAs with new compositions.
(2). The phase structure of Bio-HEAs is dominated by the single phase of BCC, and the second phases of multi-phase Bio-HEAs include FCC, HCP, Laves, B2, and IC phases. Still, not all of these precipitated phases exhibit beneficial effects on the alloy, and excessive doping will destroy the continuity of the matrix. It is necessary to verify the reinforcement mechanism of precipitated relative Bio-HEAs through continuous exploratory experiments to achieve the goal of multiphase synergistic reinforcement.
(3). In the biomedical field, the application trend of Bio-HEAs is biased towards metal implants, which requires rigorous experimental to demonstrate the biosafety and service life of Bio-HEAs, and the continuous efforts of several research teams.
JF mainly wrote this review paper, YT and JL provided writing ideas for the article, PZ and LW provided guidance and revisions for the article, and CL provided writing suggestions for the article.
The authors thankfully acknowledge the financial support listed as below: National Natural Science Foundation of China under (Grant Nos.51831011, 52011530181, 52075317), Shanghai Science and Technology Commission under Grant No. 20S31900100, Science Foundation of Institute of Plasma Physics Chinese Academy of Sciences (No. DSJJ-18-01), Guangxi Science and Technology Program [Guike Jizi (2020) No. 198], Guangxi key R & D Project (Guike AB18050008).
The authors declare that the research was conducted in the absence of any commercial or financial relationships that could be construed as a potential conflict of interest.
All claims expressed in this article are solely those of the authors and do not necessarily represent those of their affiliated organizations, or those of the publisher, the editors and the reviewers. Any product that may be evaluated in this article, or claim that may be made by its manufacturer, is not guaranteed or endorsed by the publisher.
Akdim, B., Woodward, C., Rao, S., and Antillon, E. (2021). Predicting core structure variations and spontaneous partial kink formation for ½ screw dislocations in three BCC NbTiZr alloys. Scr. Mater. 199, 113834. doi:10.1016/j.scriptamat.2021.113834
Al-Hafidh, N. N., Al-Khatib, A. R., and Al-Hafidh, N. N. (2020). Assessment of the cortical bone thickness by CT-scan and its association with orthodontic implant position in a young adult eastern mediterranean population: A cross sectional study. Int. Orthod. 18, 246–257. doi:10.1016/J.ORTHO.2020.02.001
Arif, Z. U., Khalid, M. Y., Al Rashid, A., ur Rehman, E., and Atif, M. (2022). Laser deposition of high-entropy alloys: A comprehensive review. Opt. Laser Technol. 145, 107447. doi:10.1016/J.OPTLASTEC.2021.107447
Arif, Z. U., Khalid, M. Y., ur Rehman, E., Ullah, S., Atif, M., and Tariq, A. (2021). A review on laser cladding of high-entropy alloys, their recent trends and potential applications. J. Manuf. Process. 68, 225–273. doi:10.1016/J.JMAPRO.2021.06.041
Bai, L., Hu, Y., Liang, X., Tong, Y., Liu, J., Zhang, Z., et al. (2021). Titanium alloying enhancement of mechanical properties of NbTaMoW refractory high-Entropy alloy: First-principles and experiments perspective. J. Alloys Compd. 857, 157542. doi:10.1016/J.JALLCOM.2020.157542
Bakr, M., Syarif, J., and Hashem, I. A. T. (2022). Prediction of phase and hardness of HEAs based on constituent elements using machine learning models. Mater. Today Commun. 31, 103407. doi:10.1016/J.MTCOMM.2022.103407
Bao, L., Zheng, M., Zhou, Q., Gao, P., Xu, Y., and Jiang, H. (2022). Multi-objective optimization of partition temperature of steel sheet by NSGA-II using response surface methodology. Case Stud. Therm. Eng. 31, 101818. doi:10.1016/J.CSITE.2022.101818
Basu, B., Gowtham, N. H., Xiao, Y., Kalidindi, S. R., and Leong, K. W. (2022). Biomaterialomics: Data science-driven pathways to develop fourth-generation biomaterials. Acta Biomater. 143, 1–25. doi:10.1016/J.ACTBIO.2022.02.027
Bhandari, U., Zhang, C., Zeng, C., Guo, S., Adhikari, A., and Yang, S. (2021). Deep learning-based hardness prediction of novel refractory high-entropy alloys with experimental validation. Crystals 11, 46. doi:10.3390/CRYST11010046
Biermair, F., Razumovskiy, V. I., and Ressel, G. (2022). Influence of alloying on thermodynamic properties of AlCoCrFeNiTi high entropy alloys from DFT calculations. Comput. Mater. Sci. 202, 110952. doi:10.1016/J.COMMATSCI.2021.110952
Braic, V., Balaceanu, M., Braic, M., Vladescu, A., Panseri, S., and Russo, A. (2012). Characterization of multi-principal-element (TiZrNbHfTa)N and (TiZrNbHfTa)C coatings for biomedical applications. J. Mech. Behav. Biomed. Mater. 10, 197–205. doi:10.1016/J.JMBBM.2012.02.020
Cann, J. L., De Luca, A., Dunand, D. C., Dye, D., Miracle, D. B., Oh, H. S., et al. (2021). Sustainability through alloy design: Challenges and opportunities. Prog. Mater. Sci. 117, 100722. doi:10.1016/J.PMATSCI.2020.100722
Chanda, B., Jana, P. P., and Das, J. (2021). A tool to predict the evolution of phase and Young's modulus in high entropy alloys using artificial neural network. Comput. Mater. Sci. 197, 110619. doi:10.1016/J.COMMATSCI.2021.110619
Che Ghani, S. A., Mohamed, S. R., Sha’ban, M., Wan Harun, W. S., and Md Noar, N. A. Z. (2020). Experimental investigation of biological and mechanical properties of CoCrMo based selective laser melted metamaterials for bone implant manufacturing. Procedia CIRP 89, 79–91. doi:10.1016/J.PROCIR.2020.05.122
Chen, C., Chen, J., Yuan, S., Li, W., Wang, W., Li, X., et al. (2022a). Microstructure, mechanical properties, corrosion resistance and anti-bacterial behavior of novel Co-free high entropy alloys. J. Alloys Compd. 902, 163714. doi:10.1016/J.JALLCOM.2022.163714
Chen, H., Deng, T., Du, T., Chen, B., Skibniewski, M. J., and Zhang, L. (2022b). An RF and LSSVM-NSGA-II method for the multi-objective optimization of high-performance concrete durability. Cem. Concr. Compos. 129, 104446. doi:10.1016/J.CEMCONCOMP.2022.104446
Chen, H., Kauffmann, A., Gorr, B., Schliephake, D., Seemüller, C., Wagner, J. N., et al. (2016). Microstructure and mechanical properties at elevated temperatures of a new Al-containing refractory high-entropy alloy Nb-Mo-Cr-Ti-Al. J. Alloys Compd. 661, 206–215. doi:10.1016/J.JALLCOM.2015.11.050
Chen, S. H., Zhang, J. S., Guan, S., Li, T., Liu, J. Q., Wu, F. F., et al. (2022c). Microstructure and mechanical properties of WNbMoTaZrx (x = 0.1, 0.3, 0.5, 1.0) refractory high entropy alloys. Mater. Sci. Eng. A 835, 142701. doi:10.1016/J.MSEA.2022.142701
Chen, S. M., Ma, Z. J., Qiu, S., Zhang, L. J., Zhang, S. Z., Yang, R., et al. (2022d). Phase decomposition and strengthening in HfNbTaTiZr high entropy alloy from first-principles calculations. Acta Mater. 225, 117582. doi:10.1016/J.ACTAMAT.2021.117582
Chen, X., Lu, Q., Gao, Y., Tian, W., Wang, H., Zhou, H., et al. (2022e). Bidirectional improvement of strength and ductility of CoCrFeNiTi (Co40Cr16Fe35Ni8Ti1) high-entropy alloys suitable for coronary stents. J. Mater. Res. Technol. 18, 1934–1946. doi:10.1016/J.JMRT.2022.03.084
Chen, Y., Li, Y., Cheng, X., Xu, Z., Wu, C., Cheng, B., et al. (2018). Interstitial strengthening of refractory ZrTiHfNb0.5Ta0.5Ox (x = 0.05, 0.1, 0.2) high-entropy alloys. Mater. Lett. 228, 145–147. doi:10.1016/J.MATLET.2018.05.123
Chen, Z. W., Chen, L. X., Gariepy, Z., Yao, X., and Singh, C. V. (2022f). High-throughput and machine-learning accelerated design of high entropy alloy catalysts. Trends Chem. 4, 577–579. doi:10.1016/J.TRECHM.2022.03.010
Cheng, Q., Shi, H., Jiang, Q., Yu, Z., Zhang, P., and Ye, X. (2022). Effect of phase composition on microstructure and wear resistance of (Al16.80Co20.74Cr20.49Fe21.28Ni20.70)99.5Ti0.5 high-entropy alloy coatings. Mater. Today Commun. 31, 103765. doi:10.1016/J.MTCOMM.2022.103765
Chiba, A., Kumagai, K., Nomura, N., and Miyakawa, S. (2007). Pin-on-disk wear behavior in a like-on-like configuration in a biological environment of high carbon cast and low carbon forged Co-29Cr-6Mo alloys. Acta Mater. 55, 1309–1318. doi:10.1016/J.ACTAMAT.2006.10.005
Choi-Yim, H., and Johnson, W. L. (1997). Bulk metallic glass matrix composites. Appl. Phys. Lett. 71, 3808–3810. doi:10.1063/1.120512
Chopra, I. (2007). The increasing use of silver-based products as antimicrobial agents: A useful development or a cause for concern? J. Antimicrob. Chemother. 59, 587–590. doi:10.1093/JAC/DKM006
Cieslak, J., Tobola, J., Przewoznik, J., Berent, K., Dahlborg, U., Cornide, J., et al. (2019). Multi-phase nature of sintered vs. arc-melted CrxAlFeCoNi high entropy alloys - experimental and theoretical study. J. Alloys Compd. 801, 511–519. doi:10.1016/J.JALLCOM.2019.06.121
Cohen, A., Hostyk, J., Baugh, E. H., Buchovecky, C. M., Aggarwal, V. S., Recker, R. R., et al. (2022). Whole exome sequencing reveals potentially pathogenic variants in a small subset of premenopausal women with idiopathic osteoporosis. Bone 154, 116253. doi:10.1016/J.BONE.2021.116253
Cui, Y., Shen, J., Geng, K., and Hu, S. (2021). Fabrication of FeCoCrNiMnAl0.5-FeCoCrNiMnAl gradient HEA coating by laser cladding technique. Surf. Coatings Technol. 412, 127077. doi:10.1016/J.SURFCOAT.2021.127077
Cui, Y. W., Chen, L. Y., Qin, P., Li, R., Zang, Q., Peng, J., et al. (2022). Metastable pitting corrosion behavior of laser powder bed fusion produced Ti-6Al-4V in Hank's solution. Corros. Sci. 203, 110333. doi:10.1016/J.CORSCI.2022.110333
Davis, R., Singh, A., Jackson, M. J., Coelho, R. T., Prakash, D., Charalambous, C. P., et al. (2022). A comprehensive review on metallic implant biomaterials and their subtractive manufacturing. Int. J. Adv. Manuf. Technol. 120, 1473–1530. doi:10.1007/S00170-022-08770-8
Diao, H. Y., Feng, R., Dahmen, K. A., and Liaw, P. K. (2017). Fundamental deformation behavior in high-entropy alloys: An overview. Curr. Opin. Solid State Mater. Sci. 21, 252–266. doi:10.1016/J.COSSMS.2017.08.003
Dirras, G., Lilensten, L., Djemia, P., Laurent-Brocq, M., Tingaud, D., Couzinié, J. P., et al. (2016). Elastic and plastic properties of as-cast equimolar TiHfZrTaNb high-entropy alloy. Mater. Sci. Eng. A 654, 30–38. doi:10.1016/j.msea.2015.12.017
Du, C., Hu, L., Ren, X., Li, Y., Zhang, F., Liu, P., et al. (2021). Cracking mechanism of brittle FeCoNiCrAl HEA coating using extreme high-speed laser cladding. Surf. Coatings Technol. 424, 127617. doi:10.1016/J.SURFCOAT.2021.127617
Duesbery, M. S., and Vitek, V. (1998). Plastic anisotropy in b.c.c. transition metals. Acta Mater. 46, 1481–1492. doi:10.1016/S1359-6454(97)00367-4
Dvurečenskij, A., Cigáň, A., Lobotka, P., Radnóczi, G., Škrátek, M., Benyó, J., et al. (2022). Colloids of HEA nanoparticles in an imidazolium-based ionic liquid prepared by magnetron sputtering: Structural and magnetic properties. J. Alloys Compd. 896, 163089. doi:10.1016/J.JALLCOM.2021.163089
Fazakas, E., Zadorozhnyy, V., Varga, L. K., Inoue, A., Louzguine-Luzgin, D. V., Tian, F., et al. (2014). Experimental and theoretical study of Ti20Zr20Hf20Nb20X20 (X = V or Cr) refractory high-entropy alloys. Int. J. Refract. Metals Hard Mater. 47, 131–138. doi:10.1016/j.ijrmhm.2014.07.009
Feng, H., Li, H.-B., Dai, J., Han, Y., Qu, J.-D., Jiang, Z.-H., et al. (2022a). Why CoCrFeMnNi HEA could not passivate in chloride solution? - a novel strategy to significantly improve corrosion resistance of CoCrFeMnNi HEA by N-alloying. Corros. Sci. 204, 110396. doi:10.1016/J.CORSCI.2022.110396
Feng, J., Zhang, P., Jia, Z., Yu, Z., Fang, C., Yan, H., et al. (2021). Microstructures and mechanical properties of reduced activation ferritic/martensitic steel fabricated by laser melting deposition. Fusion Eng. Des. 173, 112865. doi:10.1016/J.FUSENGDES.2021.112865
Feng, J., Zhang, P., Jia, Z., Yu, Z., Fang, C., Yan, H., et al. (2022b). Laser additive manufacturing and post-heat treatment on microstructure and mechanical properties of 9Cr steel. Int. J. Press. Vessels Pip. 198, 104681. doi:10.1016/J.IJPVP.2022.104681
Feuerbacher, M., Heidelmann, M., and Thomas, C. (2015). Plastic deformation properties of Zr-Nb-Ti-Ta-Hf high-entropy alloys. Philos. Mag. 95, 1221–1232. doi:10.1080/14786435.2015.1028506
Gaffuri, F., Cossellu, G., Maspero, C., Lanteri, V., Ugolini, A., Rasperini, G., et al. (2021). Correlation between facial growth patterns and cortical bone thickness assessed with cone-beam computed tomography in young adult untreated patients. Saudi Dent. J. 33, 161–167. doi:10.1016/J.SDENTJ.2020.01.009
Gao, X., Wang, L., Guo, N., Luo, L., Zhu, G., Shi, C., et al. (2021). In-situ development of MB2 and their effect on microstructure and mechanical properties of refractory Hf0.5Mo0.5NbTiZr high entropy alloy matrix composites. Int. J. Refract. Metals Hard Mater. 96, 105473. doi:10.1016/J.IJRMHM.2021.105473
Geetha, M., Singh, A. K., Asokamani, R., and Gogia, A. K. (2009). Ti based biomaterials, the ultimate choice for orthopaedic implants - a review. Prog. Mater. Sci. 54, 397–425. doi:10.1016/J.PMATSCI.2008.06.004
George, E. P., Curtin, W. A., and Tasan, C. C. (2020). High entropy alloys: A focused review of mechanical properties and deformation mechanisms. Acta Mater. 188, 435–474. doi:10.1016/J.ACTAMAT.2019.12.015
George, E. P., Raabe, D., and Ritchie, R. O. (2019). High-entropy alloys. Nat. Rev. Mat. 4, 515–534. doi:10.1038/S41578-019-0121-4
Grischke, J., Eberhard, J., and Stiesch, M. (2016). Antimicrobial dental implant functionalization strategies -A systematic review. Dent. Mat. J. 35, 545–558. doi:10.4012/DMJ.2015-314
Gross, C., Bergfeldt, T., Fretwurst, T., Rothweiler, R., Nelson, K., and Stricker, A. (2020). Elemental analysis of commercial zirconia dental implants - is "metal-free" devoid of metals? J. Mech. Behav. Biomed. Mater. 107, 103759. doi:10.1016/J.JMBBM.2020.103759
Gu, M., Gong, Y., Wu, X., Dong, Y., and Wang, G. L. (2022a). Surface polarization of BiOI to boost photoelectrochemical signal transduction for high-performance bioassays. Chem. Commun. 58, 4651–4654. doi:10.1039/D2CC00019A
Gu, P., Qi, T., Chen, L., Ge, T., and Ren, X. (2022b). Manufacturing and analysis of VNbMoTaW refractory high-entropy alloy fabricated by selective laser melting. Int. J. Refract. Metals Hard Mater. 105, 105834. doi:10.1016/J.IJRMHM.2022.105834
Güler, S., Alkan, E. D., and Alkan, M. (2022). Vacuum arc melted and heat treated AlCoCrFeNiTiX based high-entropy alloys: Thermodynamic and microstructural investigations. J. Alloys Compd. 903, 163901. doi:10.1016/J.JALLCOM.2022.163901
Guo, L., Ataollah Naghavi, S., Wang, Z., Nath Varma, S., Han, Z., Yao, Z., et al. (2022). On the design evolution of hip implants: A review. Mater. Des. 216, 110552. doi:10.1016/J.MATDES.2022.110552
Guo, N. N., Wang, L., Luo, L. S., Li, X. Z., Chen, R. R., Su, Y. Q., et al. (2016). Microstructure and mechanical properties of refractory high entropy (Mo 0.5 NbHf 0.5 ZrTi) BCC/M 5 Si 3 in-situ compound. J. Alloys Compd. 660, 197–203. doi:10.1016/j.jallcom.2015.11.091
Guo, N. N., Wang, L., Luo, L. S., Li, X. Z., Su, Y. Q., Guo, J. J., et al. (2015). Microstructure and mechanical properties of refractory MoNbHfZrTi high-entropy alloy. Mater. Des. 81, 87–94. doi:10.1016/j.matdes.2015.05.019
Guo, S., Hu, Q., Ng, C., and Liu, C. T. (2013). More than entropy in high-entropy alloys: Forming solid solutions or amorphous phase. Intermetallics 41, 96–103. doi:10.1016/J.INTERMET.2013.05.002
Gurel, S., Nazarahari, A., Canadinc, D., Cabuk, H., and Bal, B. (2021). Assessment of biocompatibility of novel TiTaHf-based high entropy alloys for utility in orthopedic implants. Mater. Chem. Phys. 266, 124573. doi:10.1016/J.MATCHEMPHYS.2021.124573
Han, Z. D., Luan, H. W., Liu, X., Chen, N., Li, X. Y., Shao, Y., et al. (2018). Microstructures and mechanical properties of Ti NbMoTaW refractory high-entropy alloys. Mater. Sci. Eng. A 712, 380–385. doi:10.1016/J.MSEA.2017.12.004
Hassan, M. A., Ghayad, I. M., Mohamed, A. S. A., El-Nikhaily, A. E., and Elkady, O. A. (2021). Improvement ductility and corrosion resistance of CoCrFeNi and AlCoCrFeNi HEAs by electroless copper technique. J. Mater. Res. Technol. 13, 463–485. doi:10.1016/J.JMRT.2021.04.083
He, M. Y., Shen, Y. F., Jia, N., and Liaw, P. K. (2021). C and N doping in high-entropy alloys: A pathway to achieve desired strength-ductility synergy. Appl. Mater. Today 25, 101162. doi:10.1016/J.APMT.2021.101162
Hou, S., Sun, M., Bai, M., Lin, D., Li, Y., and Liu, W. (2022). A hybrid prediction frame for HEAs based on empirical knowledge and machine learning. Acta Mater. 228, 117742. doi:10.1016/J.ACTAMAT.2022.117742
Hu, S., Li, T., Su, Z., Meng, S., Jia, Z., and Liu, D. (2021). A novel TiZrNb medium entropy alloy (MEA) with appropriate elastic modulus for biocompatible materials. Mater. Sci. Eng. B 270, 115226. doi:10.1016/J.MSEB.2021.115226
Hu, X., Liu, X., Yan, D., and Li, Z. (2022). A high-density non-equiatomic WTaMoNbV high-entropy alloy: Alloying behavior, microstructure and mechanical properties. J. Alloys Compd. 894, 162505. doi:10.1016/J.JALLCOM.2021.162505
Huang, H., Wu, Y., He, J., Wang, H., Liu, X., An, K., et al. (2017). Phase-transformation ductilization of brittle high-entropy alloys via metastability engineering. Adv. Mat. 29, 1701678. doi:10.1002/ADMA.201701678
Huang, K., Peng, D., Yao, Z., Xia, J., Zhang, B., Liu, H., et al. (2021). Cathodic plasma driven self-assembly of HEAs dendrites by pure single FCC FeCoNiMnCu nanoparticles as high efficient electrocatalysts for OER. Chem. Eng. J. 425, 131533. doi:10.1016/J.CEJ.2021.131533
Huang, S., and Vitos, L. (2022). High entropy alloys: Elastic parameters and trends. Encycl. Mat. Metall. Alloy. 2, 427–434. doi:10.1016/B978-0-12-803581-8.11714-X
Huang, W., Wang, X., Qiao, J., and Wu, Y. (2022). Microstructures and mechanical properties of TiZrHfNbTaWx refractory high entropy alloys. J. Alloys Compd. 914, 165187. doi:10.1016/J.JALLCOM.2022.165187
Huo, W., Zhou, H., Fang, F., Zhou, X., Xie, Z., and Jiang, J. (2018). Microstructure and properties of novel CoCrFeNiTax eutectic high-entropy alloys. J. Alloys Compd. 735, 897–904. doi:10.1016/j.jallcom.2017.11.075
Hwang, E. H., Park, J. S., Seong, H. G., and Kim, S. J. (2019). Analysis on surface film formed on high-strength carbon steels in acidic phosphate solution and its relationship with localized corrosion in a 3.5% NaCl solution. J. Mat. Res. Technol. 8, 1419–1426. doi:10.1016/J.JMRT.2018.10.007
Iijima, Y., Nagase, T., Matsugaki, A., Wang, P., Ameyama, K., and Nakano, T. (2021). Design and development of Ti-Zr-Hf-Nb-Ta-Mo high-entropy alloys for metallic biomaterials. Mater. Des. 202, 109548. doi:10.1016/J.MATDES.2021.109548
Iki, M., Fujimori, K., Nakatoh, S., Tamaki, J., Ishii, S., Okimoto, N., et al. (2022). Delayed initiation of anti-osteoporosis medications increases subsequent hip and vertebral fractures in patients on long-term glucocorticoid therapy: A nationwide health insurance claims database study in Japan. Bone 160, 116396. doi:10.1016/J.BONE.2022.116396
Ishida, K. (2017). Schaeffler-type phase diagram of Ti-based alloys. Metall Mat Trans A 48, 4990–4998. doi:10.1007/S11661-017-4218-3
Jiang, Q., Zhang, P., Tan, J., Yu, Z., Tian, Y., Ma, S., et al. (2022a). Influence of the microstructure on mechanical properties of SLM additive manufacturing Fe-based bulk metallic glasses. J. Alloys Compd. 894, 162525. doi:10.1016/J.JALLCOM.2021.162525
Jiang, Q., Zhang, P., Yu, Z., Tian, Y., and Ma, S. (2022b). AlCoCrFeNi high entropy alloy fabricated via selective laser melting reinforced by Fe-based metallic glass. Mater. Lett. 307, 130994. doi:10.1016/J.MATLET.2021.130994
Jin, Z.-Z., Zha, M., Wang, S.-Q., Wang, S.-C., Wang, C., Jia, H.-L., et al. (2022). Alloying design and microstructural control strategies towards developing Mg alloys with enhanced ductility. J. Magnesium Alloys 10, 1191–1206. doi:10.1016/J.JMA.2022.04.002
Juan, C. C., Tsai, M. H., Tsai, C. W., Lin, C. M., Wang, W. R., Yang, C. C., et al. (2015). Enhanced mechanical properties of HfMoTaTiZr and HfMoNbTaTiZr refractory high-entropy alloys. Intermetallics 62, 76–83. doi:10.1016/j.intermet.2015.03.013
Juan, C. C., Tseng, K. K., Hsu, W. L., Tsai, M. H., Tsai, C. W., Lin, C. M., et al. (2016). Solution strengthening of ductile refractory HfMo x NbTaTiZr high-entropy alloys. Mater. Lett. 175, 284–287. doi:10.1016/j.matlet.2016.03.133
Kazemi, M., Ahangarani, S., Esmailian, M., and Shanaghi, A. (2020). Investigation on the corrosion behavior and biocompatibility of Ti-6Al-4V implant coated with HA/TiN dual layer for medical applications. Surf. Coatings Technol. 397, 126044. doi:10.1016/J.SURFCOAT.2020.126044
Khodaei, M., Nejatidanesh, F., Shirani, M. J., Iyengar, S., Sina, H., Valanezhad, A., et al. (2020). Optimum temperature and chlorine ion concentration for hydrogen peroxide treatment of titanium dental implant material. J. Mater. Res. Technol. 9, 13312–13319. doi:10.1016/J.JMRT.2020.09.040
Klimenko, D. N., Yurchenko, N. Y., Stepanov, N. D., and Zherebtsov, S. V. (2021). Prediction of strength characteristics of high-entropy alloys Al-Cr-Nb-Ti-V-Zr systems. Mater. Today Proc. 38, 1535–1540. doi:10.1016/J.MATPR.2020.08.145
Kumar, D., Gangwar, N., Rathore, A. S., and Ramteke, M. (2021). Multi-objective optimization of monoclonal antibody production in bioreactor. Chem. Eng. Process. - Process Intensif. 13, 108720. doi:10.1016/J.CEP.2021.108720
Lai, J. J., Lin, Y. S., Chang, C. H., Wei, T. Y., Huang, J. C., Liao, Z. X., et al. (2018). Promising Ta-Ti-Zr-Si metallic glass coating without cytotoxic elements for bio-implant applications. Appl. Surf. Sci. 427, 485–495. doi:10.1016/J.APSUSC.2017.08.065
Laplanche, G., Gadaud, P., Perrière, L., Guillot, I., and Couzinié, J. P. (2019). Temperature dependence of elastic moduli in a refractory HfNbTaTiZr high-entropy alloy. J. Alloys Compd. 799, 538–545. doi:10.1016/j.jallcom.2019.05.322
LaRosa, C. R., Shih, M., Varvenne, C., and Ghazisaeidi, M. (2019). Solid solution strengthening theories of high-entropy alloys. Mater. Charact. 151, 310–317. doi:10.1016/J.MATCHAR.2019.02.034
Lei, J., Liu, M. Q., Yap, A. U. J., and Fu, K. Y. (2013). Condylar subchondral formation of cortical bone in adolescents and young adults. Br. J. Oral Maxillofac. Surg. 51, 63–68. doi:10.1016/J.BJOMS.2012.02.006
Lei, Z., Liu, X., Wu, Y., Wang, H., Jiang, S., Wang, S., et al. (2018). Enhanced strength and ductility in a high-entropy alloy via ordered oxygen complexes. Nature 563, 546–550. doi:10.1038/s41586-018-0685-y
Li, C., Li, X., Shi, Z., Wu, P., Fu, J., Tang, J., et al. (2022). Exosomes from LPS-preconditioned bone marrow MSCs accelerated peripheral nerve regeneration via M2 macrophage polarization: Involvement of TSG-6/NF-κB/NLRP3 signaling pathway. Exp. Neurol. 356, 114139. doi:10.1016/J.EXPNEUROL.2022.114139
Li, J., Meng, X., Wan, L., and Huang, Y. (2021a). Welding of high entropy alloys: Progresses, challenges and perspectives. J. Manuf. Process. 68, 293–331. doi:10.1016/J.JMAPRO.2021.05.042
Li, T., Wu, J., and Frankel, G. S. (2021b). Localized corrosion: Passive film breakdown vs. Pit growth stability, Part VI: Pit dissolution kinetics of different alloys and a model for pitting and repassivation potentials. Corros. Sci. 182, 109277. doi:10.1016/J.CORSCI.2021.109277
Li, W., Xie, D., Li, D., Zhang, Y., Gao, Y., and Liaw, P. K. (2021c). Mechanical behavior of high-entropy alloys. Prog. Mater. Sci. 118, 100777. doi:10.1016/J.PMATSCI.2021.100777
Liang, C., Wang, C., Zhang, K., Tan, H., Liang, M., Xie, Y., et al. (2022). Mechanical and tribological properties of (FeCoNi)88-x(AlTi)12Mox high-entropy alloys. Int. J. Refract. Metals Hard Mater. 105, 105845. doi:10.1016/j.ijrmhm.2022.105845
Lilensten, L., Couzinié, J. P., Perrière, L., Hocini, A., Keller, C., Dirras, G., et al. (2018). Study of a bcc multi-principal element alloy: Tensile and simple shear properties and underlying deformation mechanisms. Acta Mater. 142, 131–141. doi:10.1016/j.actamat.2017.09.062
Lin, C. L., Lee, J. L., Kuo, S. M., Li, M. Y., Gan, L., Murakami, H., et al. (2021a). Investigation on the thermal expansion behavior of FeCoNi and Fe30Co30Ni30Cr10-xMnx high entropy alloys. Mater. Chem. Phys. 271, 124907. doi:10.1016/j.matchemphys.2021.124907
Lin, C. M., Juan, C. C., Chang, C. H., Tsai, C. W., and Yeh, J. W. (2015). Effect of Al addition on mechanical properties and microstructure of refractory AlxHfNbTaTiZr alloys. J. Alloys Compd. 624, 100–107. doi:10.1016/j.jallcom.2014.11.064
Lin, Y. C., Chao, C. M., Wang, D. K., Liu, K. M., and Tseng, H. H. (2021b). Enhancing the antifouling properties of a PVDF membrane for protein separation by grafting branch-like zwitterions via a novel amphiphilic SMA-HEA linker. J. Membr. Sci. 624, 119126. doi:10.1016/J.MEMSCI.2021.119126
Liu, B., Lei, S., Li, Y., Shan, A., and Dong, B. (2021). Deep periocular recognition method via multi-angle data augmentation. Int. J. Adv. Netw. Monit. Control 6, 11–17. doi:10.21307/IJANMC-2021-002
Liu, C., Liu, J., Yang, C., Tang, Y., Lin, Z., Li, L., et al. (2022a). Computer vision-aided 2D error assessment and correction for helix bioprinting. Int. J. Bioprint 8, 174–186. doi:10.18063/IJB.V8I2.547
Liu, C., Wang, L., Lu, W., Liu, J., Yang, C., Fan, C., et al. (2022b). Computer vision-aided bioprinting for bone research. Bone Res. 10, 21. doi:10.1038/S41413-022-00192-2
Liu, C., Yang, Y., and Xia, Z. (2020). Deformation mechanism in Al0.1CoCrFeNi Σ3(111)[110] high entropy alloys - molecular dynamics simulations. RSC Adv. 10, 27688–27696. doi:10.1039/D0RA01885F
Liu, Y., Zhang, Y., Zhang, H., Wang, N., Chen, X., Zhang, H., et al. (2017). Microstructure and mechanical properties of refractory HfMo0.5NbTiV0.5Six high-entropy composites. J. Alloys Compd. 694, 869–876. doi:10.1016/j.jallcom.2016.10.014
Liu, Z., Zhao, D., Wang, P., Yan, M., Yang, C., Chen, Z., et al. (2022c). Additive manufacturing of metals: Microstructure evolution and multistage control. J. Mater. Sci. Technol. 100, 224–236. doi:10.1016/J.JMST.2021.06.011
Long, M., and Rack, H. J. (1998). Titanium alloys in total joint replacement-a materials science perspective. Biomaterials 19, 1621–1639. doi:10.1016/S0142-9612(97)00146-4
Lv, Y., Liu, G., Wang, B., Tang, Y., Lin, Z., Liu, J., et al. (2022). Pore strategy design of a novel NiTi-Nb biomedical porous scaffold based on a triply periodic minimal surface. Front. Bioeng. Biotechnol. 10, 910475. doi:10.3389/FBIOE.2022.910475
Ma, N., Liu, S., Liu, W., Xie, L., Wei, D., Wang, L., et al. (2020a). Research progress of titanium-based high entropy alloy: Methods, properties, and applications. Front. Bioeng. Biotechnol. 8, 1303. doi:10.3389/FBIOE.2020.603522/BIBTEX
Ma, Y., Liu, X., Dong, W., Li, R., Zhang, Y., Lu, Y., et al. (2020b). Interstitial carbide synergistically strengthening high-entropy alloy CoCrFeNiV0.5C. Mater. Sci. Eng. A 792, 139802. doi:10.1016/j.msea.2020.139802
Mao, C., Yu, W., Jin, M., Wang, Y., Shang, X., Lin, L., et al. (2022). Mechanobiologically optimized Ti-35Nb-2Ta-3Zr improves load transduction and enhances bone remodeling in tilted dental implant therapy. Bioact. Mater. 16, 15–26. doi:10.1016/J.BIOACTMAT.2022.03.005
Mattia, L., Davis, S., Mark-Wagstaff, C., Abrahamsen, B., Peel, N., Eastell, R., et al. (2022). Utility of PINP to monitor osteoporosis treatment in primary care, the POSE study (PINP and Osteoporosis in Sheffield Evaluation). Bone 158, 116347. doi:10.1016/J.BONE.2022.116347
Meloni, M., Balzaretti, S., and Ceriotti, L. (2019). Medical devices biocompatibility assessment on HCE: Evidences of delayed cytotoxicity of preserved compared to preservative free eye drops. Regul. Toxicol. Pharmacol. 106, 81–89. doi:10.1016/J.YRTPH.2019.04.022
Miracle, D. B., Miller, J. D., Senkov, O. N., Woodward, C., Uchic, M. D., and Tiley, J. (2014). Exploration and development of high entropy alloys for structural applications. Entropy 16, 494–525. doi:10.3390/E16010494
Moazzen, P., Toroghinejad, M. R., Zargar, T., and Cavaliere, P. (2022). Investigation of hardness, wear and magnetic properties of NiCoCrFeZrx HEA prepared through mechanical alloying and spark plasma sintering. J. Alloys Compd. 892, 161924. doi:10.1016/J.JALLCOM.2021.161924
Moghadasi, K., Mohd Isa, M. S., Ariffin, M. A., Mohd jamil, M. Z., Raja, S., Wu, B., et al. (2022). A review on biomedical implant materials and the effect of friction stir based techniques on their mechanical and tribological properties. J. Mater. Res. Technol. 17, 1054–1121. doi:10.1016/J.JMRT.2022.01.050
Mohd Pauzi, S. S., Harun, M. K., and Talari, M. K. (2016). Effect of Ta addition on microstructure and hardness of FeCrNiMnCoTax and Al0.5FeCrNiMnCoTax high-entropy alloys. Msf 846, 13–19. doi:10.4028/WWW.SCIENTIFIC.NET/MSF.846.13
Motallebzadeh, A., Peighambardoust, N. S., Sheikh, S., Murakami, H., Guo, S., and Canadinc, D. (2019). Microstructural, mechanical and electrochemical characterization of TiZrTaHfNb and Ti1.5ZrTa0.5Hf0.5Nb0.5 refractory high-entropy alloys for biomedical applications. Intermetallics 113, 106572. doi:10.1016/J.INTERMET.2019.106572
Motallebzadeh, A., Yagci, M. B., Bedir, E., Aksoy, C. B., and Canadinc, D. (2018). Mechanical properties of TiTaHfNbZr high-entropy alloy coatings deposited on NiTi shape memory alloy substrates. Metall Mat Trans A 49, 1992–1997. doi:10.1007/S11661-018-4605-4
Muftah, W., Allport, J., and Vishnyakov, V. (2021). Corrosion performance and mechanical properties of FeCrSiNb amorphous equiatomic HEA thin film. Surf. Coatings Technol. 422, 127486. doi:10.1016/J.SURFCOAT.2021.127486
Nagase, T., Iijima, Y., Matsugaki, A., Ameyama, K., and Nakano, T. (2020). Design and fabrication of Ti-Zr-Hf-Cr-Mo and Ti-Zr-Hf-Co-Cr-Mo high-entropy alloys as metallic biomaterials. Mater. Sci. Eng. C 107, 110322. doi:10.1016/J.MSEC.2019.110322
Nan, L., and Yang, K. (2016). Effect of Cu addition on antibacterial property of type 200 stainless steel. Mater. Technol. 31, 44–47. doi:10.1179/1753555715Y.0000000025
Nong, Z. S., Lei, Y. N., and Zhu, J. C. (2018). Wear and oxidation resistances of AlCrFeNiTi-based high entropy alloys. Intermetallics 101, 144–151. doi:10.1016/j.intermet.2018.07.017
Ocak, B. C., and Goller, G. (2021). Investigation the effect of FeNiCoCrMo HEA addition on properties of B4C ceramic prepared by spark plasma sintering. J. Eur. Ceram. Soc. 41, 6290–6301. doi:10.1016/J.JEURCERAMSOC.2021.06.003
Ostovari Moghaddam, A., Shaburova, N. A., Samodurova, M. N., Abdollahzadeh, A., and Trofimov, E. A. (2021). Additive manufacturing of high entropy alloys: A practical review. J. Mater. Sci. Technol. 77, 131–162. doi:10.1016/j.jmst.2020.11.029
Ostovari Moghaddam, A., and Trofimov, E. A. (2021). Toward expanding the realm of high entropy materials to platinum group metals: A review. J. Alloys Compd. 851, 156838. doi:10.1016/J.JALLCOM.2020.156838
Peker, A., and Johnson, W. L. (1993). A highly processable metallic glass: Zr41.2Ti13.8Cu12.5Ni10.0Be22.5. Appl. Phys. Lett. 63, 2342–2344. doi:10.1063/1.110520
Peng, S., Xu, J., Xie, Z. H., and Munroe, P. (2022). Titanium bipolar plates augmented by nanocrystalline TiZrHfMoW coatings for application in proton exchange membrane fuel cells. Appl. Surf. Sci. 591, 153200. doi:10.1016/J.APSUSC.2022.153200
Poulia, A., Georgatis, E., Lekatou, A., and Karantzalis, A. E. (2016). Microstructure and wear behavior of a refractory high entropy alloy. Int. J. Refract. Metals Hard Mater. 57, 50–63. doi:10.1016/J.IJRMHM.2016.02.006
Pradeep, K. G., Tasan, C. C., Yao, M. J., Deng, Y., Springer, H., and Raabe, D. (2015). Non-equiatomic high entropy alloys: Approach towards rapid alloy screening and property-oriented design. Mater. Sci. Eng. A 648, 183–192. doi:10.1016/J.MSEA.2015.09.010
Qiao, D. X., Jiang, H., Chang, X. X., Lu, Y. P., and Li, T. J. (2017). Microstructure and mechanical properties of VTaTiMoAlx refractory high entropy alloys. Msf 898, 638–642. doi:10.4028/WWW.SCIENTIFIC.NET/MSF.898.638
Radi, A., Asghari-Rad, P., Kim, H. S., and Yapici, G. G. (2022). On the development of a novel multi-phase high entropy alloy with transformation-induced plasticity effect. J. Alloys Compd. 905, 164014. doi:10.1016/J.JALLCOM.2022.164014
Raturi, A., Biswas, K., and Gurao, N. P. (2022). Elastic and plastic anisotropy in a refractory high entropy alloy utilizing combinatorial instrumented indentation and electron backscatter diffraction. J. Alloys Compd. 896, 162902. doi:10.1016/J.JALLCOM.2021.162902
Ren, G., Huang, L., Hu, K., Li, T., Lu, Y., Qiao, D., et al. (2022). Enhanced antibacterial behavior of a novel Cu-bearing high-entropy alloy. J. Mater. Sci. Technol. 117, 158–166. doi:10.1016/J.JMST.2022.02.001
Roy, A., Babuska, T., Krick, B., and Balasubramanian, G. (2020). Machine learned feature identification for predicting phase and Young's modulus of low-, medium- and high-entropy alloys. Scr. Mater. 185, 152–158. doi:10.1016/J.SCRIPTAMAT.2020.04.016
Sarell, C. J., Wilkinson, S. R., and Viles, J. H. (2010). Substoichiometric levels of Cu2+ ions accelerate the kinetics of fiber formation and promote cell toxicity of amyloid-β from alzheimer disease. J. Biol. Chem. 285, 41533–41540. doi:10.1074/JBC.M110.171355
Schönecker, S., Li, X., Wei, D., Nozaki, S., Kato, H., Vitos, L., et al. (2022). Harnessing elastic anisotropy to achieve low-modulus refractory high-entropy alloys for biomedical applications. Mater. Des. 215, 110430. doi:10.1016/J.MATDES.2022.110430
Schopphoven, T., Gasser, A., Wissenbach, K., and Poprawe, R. (2016). Investigations on ultra-high-speed laser material deposition as alternative for hard chrome plating and thermal spraying. J. Laser Appl. 28, 022501. doi:10.2351/1.4943910
Senkov, O. N., Wilks, G. B., Miracle, D. B., Chuang, C. P., and Liaw, P. K. (2010). Refractory high-entropy alloys. Intermetallics 18, 1758–1765. doi:10.1016/J.INTERMET.2010.05.014
Senkov, O. N., Wilks, G. B., Scott, J. M., and Miracle, D. B. (2011). Mechanical properties of Nb25Mo25Ta25W25 and V20Nb20Mo20Ta20W20 refractory high entropy alloys. Intermetallics 19, 698–706. doi:10.1016/J.INTERMET.2011.01.004
Serda, M. (2013). Synteza i aktywność biologiczna nowych analogów tiosemikarbazonowych chelatorów żelaza. Uniw. śląski 3, 343–354. doi:10.2/JQUERY.MIN.JS
Sheikh, S., Shafeie, S., Hu, Q., Ahlström, J., Persson, C., Veselý, J., et al. (2016). Alloy design for intrinsically ductile refractory high-entropy alloys. J. Appl. Phys. 120, 164902. doi:10.1063/1.4966659
Shipilova, O. I., Gorbunov, S. P., Paperny, V. L., Chernykh, A. A., Dresvyansky, V. P., Martynovich, E. F., et al. (2020). Fabrication of metal-dielectric nanocomposites using a table-top ion implanter. Surf. Coatings Technol. 393, 125742. doi:10.1016/J.SURFCOAT.2020.125742
Soto, A. O., Salgado, A. S., and Niño, E. B. (2020). Thermodynamic analysis of high entropy alloys and their mechanical behavior in high and low-temperature conditions with a microstructural approach - a review. Intermetallics 124, 106850. doi:10.1016/J.INTERMET.2020.106850
Spataru, M. C., Butnaru, M., Sandu, A. V., Vulpe, V., Vlad, M. D., Baltatu, M. S., et al. (2021). In-depth assessment of new Ti-based biocompatible materials. Mater. Chem. Phys. 258, 123959. doi:10.1016/J.MATCHEMPHYS.2020.123959
Squitti, R., Tecchio, F., and Ventriglia, M. (2015). The role of copper in human diet and risk of dementia. Curr. Nutr. Rep. 4, 114–125. doi:10.1007/S13668-015-0121-Y
Stepanov, N. D., Yurchenko, N. Y., Skibin, D. V., Tikhonovsky, M. A., and Salishchev, G. A. (2015). Structure and mechanical properties of the AlCrxNbTiV (x = 0, 0.5, 1, 1.5) high entropy alloys. J. Alloys Compd. 652, 266–280. doi:10.1016/j.jallcom.2015.08.224
Takeuchi, A., Amiya, K., Wada, T., and Yubuta, K. (2015). Alloy design for high-entropy alloys based on Pettifor map for binary compounds with 1:1 stoichiometry. Intermetallics 66, 56–66. doi:10.1016/J.INTERMET.2015.06.014
Tancret, F. (2022). Materials design: Computational alloy design by combinatorial optimization. Encycl. Mat. Metall. Alloy., 596–608. doi:10.1016/B978-0-12-819726-4.00061-2
Thebelt, A., Tsay, C., Lee, R. M., Sudermann-Merx, N., Walz, D., Tranter, T., et al. (2022). Multi-objective constrained optimization for energy applications via tree ensembles. Appl. Energy 306, 118061. doi:10.1016/J.APENERGY.2021.118061
Tian, F., Varga, L. K., Chen, N., Shen, J., and Vitos, L. (2014). Ab initio design of elastically isotropic TiZrNbMoV high-entropy alloys. J. Alloys Compd. 599, 19–25. doi:10.1016/j.jallcom.2014.01.237
Tian, F., Varga, L. K., Chen, N., Shen, J., and Vitos, L. (2015). Empirical design of single phase high-entropy alloys with high hardness. Intermetallics 58, 1–6. doi:10.1016/J.INTERMET.2014.10.010
Tie, D., Feyerabend, F., Feyerabend, W.-D., Müller, R., Schade, K., Liefeith, K., et al. (2013). Antibacterial biodegradable Mg-Ag alloys. eCM 25, 284–298. doi:10.22203/ECM.V025A20
Todai, M., Nagase, T., Hori, T., Matsugaki, A., Sekita, A., and Nakano, T. (2017). Novel TiNbTaZrMo high-entropy alloys for metallic biomaterials. Scr. Mater. 129, 65–68. doi:10.1016/J.SCRIPTAMAT.2016.10.028
Tong, Y., Bai, L., Liang, X., Chen, Y., Zhang, Z., Liu, J., et al. (2020). Influence of alloying elements on mechanical and electronic properties of NbMoTaWX (X = Cr, Zr, V, Hf and Re) refractory high entropy alloys. Intermetallics 126, 106928. doi:10.1016/J.INTERMET.2020.106928
Troparevsky, M. C., Morris, J. R., Kent, P. R. C., Lupini, A. R., and Stocks, G. M. (2015). Criteria for predicting the formation of single-phase high-entropy alloys. Phys. Rev. X 5, 011041. doi:10.1103/PHYSREVX.5.011041/FIGURES/2/MEDIUM
Uppal, G., Thakur, A., Chauhan, A., and Bala, S. (2022). Magnesium based implants for functional bone tissue regeneration - a review. J. Magnesium Alloys 10, 356–386. doi:10.1016/J.JMA.2021.08.017
Wang, B., Huang, X., Fu, A., Liu, Y., and Liu, B. (2018a). Serration behavior and microstructure of high entropy alloy CoCrFeMnNi prepared by powder metallurgy. Mater. Sci. Eng. A 726, 37–44. doi:10.1016/j.msea.2018.04.071
Wang, G., Liu, Q., Yang, J., Li, X., Sui, X., Gu, Y., et al. (2019). Synthesis and thermal stability of a nanocrystalline MoNbTaTiV refractory high-entropy alloy via mechanical alloying. Int. J. Refract. Metals Hard Mater. 84, 104988. doi:10.1016/J.IJRMHM.2019.104988
Wang, J. C., Liu, Y. J., Liang, S. X., Zhang, Y. S., Wang, L. Q., Sercombe, T. B., et al. (2022a). Comparison of microstructure and mechanical behavior of Ti-35Nb manufactured by laser powder bed fusion from elemental powder mixture and prealloyed powder. J. Mater. Sci. Technol. 105, 1–16. doi:10.1016/J.JMST.2021.07.021
Wang, L., Wang, Y., Huang, W., Liu, J., Tang, Y., Zhang, L., et al. (2020). Tensile and superelastic behaviors of Ti-35Nb-2Ta-3Zr with gradient structure. Mater. Des. 194, 108961. doi:10.1016/J.MATDES.2020.108961
Wang, L., Xie, L., Lv, Y., Zhang, L. C., Chen, L., Meng, Q., et al. (2017). Microstructure evolution and superelastic behavior in Ti-35Nb-2Ta-3Zr alloy processed by friction stir processing. Acta Mater. 131, 499–510. doi:10.1016/J.ACTAMAT.2017.03.079
Wang, L., Xie, L., Zhang, L. C., Chen, L., Ding, Z., Lv, Y., et al. (2018b). Microstructure evolution and superelasticity of layer-like NiTiNb porous metal prepared by eutectic reaction. Acta Mater. 143, 214–226. doi:10.1016/J.ACTAMAT.2017.10.021
Wang, Q., Dong, C., and Liaw, P. K. (2015). Structural stabilities of β-Ti alloys studied using a new Mo equivalent derived from [β/(α + β)] phase-boundary slopes. Metall Mat Trans A 46, 3440–3447. doi:10.1007/S11661-015-2923-3/FIGURES/3
Wang, S., Li, Y., Zhang, D., Yang, Y., Marwana Manladan, S., and Luo, Z. (2022b). Microstructure and mechanical properties of high strength AlCoCrFeNi2.1 eutectic high entropy alloy prepared by selective laser melting (SLM). Mater. Lett. 310, 131511. doi:10.1016/J.MATLET.2021.131511
Wang, S. P., and Xu, J. (2017). TiZrNbTaMo high-entropy alloy designed for orthopedic implants: As-cast microstructure and mechanical properties. Mater. Sci. Eng. C 73, 80–89. doi:10.1016/J.MSEC.2016.12.057
Wang, W., Yang, K., Wang, Q., Dai, P., Fang, H., Wu, F., et al. (2022c). Novel Ti-Zr-Hf-Nb-Fe refractory high-entropy alloys for potential biomedical applications. J. Alloys Compd. 906, 164383. doi:10.1016/J.JALLCOM.2022.164383
Wang, X., Xu, S., Zhou, S., Xu, W., Leary, M., Choong, P., et al. (2016). Topological design and additive manufacturing of porous metals for bone scaffolds and orthopaedic implants: A review. Biomaterials 83, 127–141. doi:10.1016/J.BIOMATERIALS.2016.01.012
Waseem, O. A., and Ryu, H. J. (2017). Powder metallurgy processing of a WxTaTiVCr high-entropy alloy and its derivative alloys for fusion material applications. Sci. Rep. 7, 1926. doi:10.1038/S41598-017-02168-3
Wei, D., Gong, W., Wang, L., Tang, B., Kawasaki, T., Harjo, S., et al. (2022a). Strengthening of high-entropy alloys via modulation of cryo-pre-straining-induced defects. J. Mater. Sci. Technol. 129, 251–260. doi:10.1016/J.JMST.2022.04.055
Wei, D., Wang, L., Zhang, Y., Gong, W., Tsuru, T., Lobzenko, I., et al. (2022b). Metalloid substitution elevates simultaneously the strength and ductility of face-centered-cubic high-entropy alloys. Acta Mater. 225, 117571. doi:10.1016/J.ACTAMAT.2021.117571
Wei, X., Zhang, P., Yu, Z., Yan, H., Wu, D., Shi, H., et al. (2021). Effect of phase transformation on mechanical properties of Al16.80Co20.74Cr20.49Fe21.28Ni20.70 high entropy alloy coatings processed by laser cladding. J. Alloys Compd. 862, 158563. doi:10.1016/J.JALLCOM.2020.158563
Wen, C., Zhang, Y., Wang, C., Xue, D., Bai, Y., Antonov, S., et al. (2019). Machine learning assisted design of high entropy alloys with desired property. Acta Mater. 170, 109–117. doi:10.1016/J.ACTAMAT.2019.03.010
Wu, D., Isaksson, P., Ferguson, S. J., and Persson, C. (2018). Young's modulus of trabecular bone at the tissue level: A review. Acta Biomater. 78, 1–12. doi:10.1016/J.ACTBIO.2018.08.001
Wu, H., and Fan, G. (2020). An overview of tailoring strain delocalization for strength-ductility synergy. Prog. Mater. Sci. 113, 100675. doi:10.1016/J.PMATSCI.2020.100675
Wu, Y. D., Cai, Y. H., Chen, X. H., Wang, T., Si, J. J., Wang, L., et al. (2015). Phase composition and solid solution strengthening effect in TiZrNbMoV high-entropy alloys. Mater. Des. 83, 651–660. doi:10.1016/j.matdes.2015.06.072
Wu, Z., Li, B., Chen, M., Yang, Y., Zheng, R., Yuan, L., et al. (2022). Tailoring magnetic property and corrosion resistance of FeCoNiCuAl high-entropy alloy with Ce additive. J. Alloys Compd. 901, 163665. doi:10.1016/J.JALLCOM.2022.163665
Xie, J., Zhang, S., Sun, Y., Hao, Y., An, B., Li, Q., et al. (2020). Microstructure and mechanical properties of high entropy CrMnFeCoNi alloy processed by electopulsing-assisted ultrasonic surface rolling. Mater. Sci. Eng. A 795, 140004. doi:10.1016/j.msea.2020.140004
Xin, Y., Li, S., Qian, Y., Zhu, W., Yuan, H., Jiang, P., et al. (2020). High-entropy alloys as a platform for catalysis: Progress, challenges, and opportunities. ACS Catal. 10, 11280–11306. doi:10.1021/ACSCATAL.0C03617/ASSET/IMAGES/LARGE/CS0C03617_0015
Xing, F., Li, S., Yin, D., Xie, J., Rommens, P. M., Xiang, Z., et al. (2022). Recent progress in Mg-based alloys as a novel bioabsorbable biomaterials for orthopedic applications. J. Magnesium Alloys 10, 1428–1456. doi:10.1016/J.JMA.2022.02.013
Yadav, S., Zhang, Q., Behera, A., Haridas, R. S., Agrawal, P., Gong, J., et al. (2021). Role of binder phase on the microstructure and mechanical properties of a mechanically alloyed and spark plasma sintered WC-FCC HEA composites. J. Alloys Compd. 877, 160265. doi:10.1016/J.JALLCOM.2021.160265
Yang, C. H., Wang, Y. T., Tsai, W. F., Ai, C. F., Lin, M. C., and Huang, H. H. (2011). Effect of oxygen plasma immersion ion implantation treatment on corrosion resistance and cell adhesion of titanium surface. Clin. Oral Implants Res. 22, 1426–1432. doi:10.1111/J.1600-0501.2010.02132.X
Yang, F., Wang, J., Zhang, Y., Wu, Z., Zhang, Z., Zhao, F., et al. (2022a). Recent progress on the development of high entropy alloys (HEAs) for solid hydrogen storage: A review. Int. J. Hydrogen Energy 47, 11236–11249. doi:10.1016/J.IJHYDENE.2022.01.141
Yang, W., Liu, Y., Pang, S., Liaw, P. K., and Zhang, T. (2020). Bio-corrosion behavior and in vitro biocompatibility of equimolar TiZrHfNbTa high-entropy alloy. Intermetallics 124, 106845. doi:10.1016/J.INTERMET.2020.106845
Yang, W., Pang, S., Liu, Y., Wang, Q., Liaw, P. K., and Zhang, T. (2022b). Design and properties of novel Ti-Zr-Hf-Nb-Ta high-entropy alloys for biomedical applications. Intermetallics 141, 107421. doi:10.1016/J.INTERMET.2021.107421
Yang, X., and Zhang, Y. (2012). Prediction of high-entropy stabilized solid-solution in multi-component alloys. Mater. Chem. Phys. 132, 233–238. doi:10.1016/J.MATCHEMPHYS.2011.11.021
Ye, Y. F., Wang, Q., Lu, J., Liu, C. T., and Yang, Y. (2015). Design of high entropy alloys: A single-parameter thermodynamic rule. Scr. Mater. 104, 53–55. doi:10.1016/J.SCRIPTAMAT.2015.03.023
Ye, Y. F., Wang, Q., Lu, J., Liu, C. T., and Yang, Y. (2016). High-entropy alloy: Challenges and prospects. Mater. Today 19, 349–362. doi:10.1016/J.MATTOD.2015.11.026
Yeh, J. W. (2013). Alloy design strategies and future trends in high-entropy alloys. JOM 65, 1759–1771. doi:10.1007/S11837-013-0761-6
Yeh, J. W., Chen, S. K., Lin, S. J., Gan, J. Y., Chin, T. S., Shun, T. T., et al. (2004). Nanostructured high-entropy alloys with multiple principal elements: Novel alloy design concepts and outcomes. Adv. Eng. Mat. 6, 299–303. doi:10.1002/ADEM.200300567
Yeh, J. W. (2006). Recent progress in high-entropy alloys. Ann. Chim. Sci. Mat. 31, 633–648. doi:10.3166/ACSM.31.633-648
Yu, P., Feng, R., Du, J., Shinzato, S., Chou, J. P., Chen, B., et al. (2019). Phase transformation assisted twinning in a face-centered-cubic FeCrNiCoAl high entropy alloy. Acta Mater. 181, 491–500. doi:10.1016/J.ACTAMAT.2019.10.012
Yurchenko, N. Y., Stepanov, N. D., Zherebtsov, S. V., Tikhonovsky, M. A., and Salishchev, G. A. (2017). Structure and mechanical properties of B2 ordered refractory AlNbTiVZrx (x = 0-1.5) high-entropy alloys. Mater. Sci. Eng. A 704, 82–90. doi:10.1016/J.MSEA.2017.08.019
Zhang, E. L., Fu, S., Wang, R. X., Li, H. X., Liu, Y., Ma, Z. Q., et al. (2019). Role of Cu element in biomedical metal alloy design. Rare Metall. 38, 476–494. doi:10.1007/S12598-019-01245-Y
Zhang, E., Zhao, X., Hu, J., Wang, R., Fu, S., and Qin, G. (2021). Antibacterial metals and alloys for potential biomedical implants. Bioact. Mater. 6, 2569–2612. doi:10.1016/J.BIOACTMAT.2021.01.030
Zhang, M., Wang, D., He, L., Ye, X., Ouyang, W., Xu, Z., et al. (2022). Microstructure and elevated temperature wear behavior of laser-cladded AlCrFeMnNi high-entropy alloy coating. Opt. Laser Technol. 149, 107845. doi:10.1016/J.OPTLASTEC.2022.107845
Zhang, M., Zhou, X., and Li, J. (2017). Microstructure and mechanical properties of a refractory CoCrMoNbTi high-entropy alloy. J. Materi Eng Perform 26, 3657–3665. doi:10.1007/S11665-017-2799-Z
Zhang, W., Liaw, P. K., and Zhang, Y. (2018). Science and technology in high-entropy alloys. Sci. China Mat. 61, 2–22. doi:10.1007/S40843-017-9195-8
Zhang, Y., Yang, X., and Liaw, P. K. (2012). Alloy design and properties optimization of high-entropy alloys. JOM 64, 830–838. doi:10.1007/S11837-012-0366-5
Zhang, Y., Zhou, Y. J., Lin, J. P., Chen, G. L., and Liaw, P. K. (2008). Solid-solution phase formation rules for multi-component alloys. Adv. Eng. Mat. 10, 534–538. doi:10.1002/ADEM.200700240
Zhang, Y., Zuo, T. T., Tang, Z., Gao, M. C., Dahmen, K. A., Liaw, P. K., et al. (2014). Microstructures and properties of high-entropy alloys. Prog. Mater. Sci. 61, 1–93. doi:10.1016/J.PMATSCI.2013.10.001
Zhao, S. (2020). Defect properties in a VTaCrW equiatomic high entropy alloy (HEA) with the body centered cubic (bcc) structure. J. Mater. Sci. Technol. 44, 133–139. doi:10.1016/J.JMST.2019.10.025
Zheng, S., Li, J., Zhang, J., Jiang, K., Liu, X., Chang, C., et al. (2018). Effect of Si addition on the electrochemical corrosion and passivation behavior of Fe-Cr-Mo-C-B-Ni-P metallic glasses. J. Non-Crystalline Solids 493, 33–40. doi:10.1016/J.JNONCRYSOL.2018.04.036
Zhou, J., Cheng, Y., Chen, Y., and Liang, X. (2022). Composition design and preparation process of refractory high-entropy alloys: A review. Int. J. Refract. Metals Hard Mater. 105, 105836. doi:10.1016/J.IJRMHM.2022.105836
Zhou, Y. J., Zhang, Y., Wang, Y. L., and Chen, G. L. (2007). Solid solution alloys of AlCoCrFeNiTix with excellent room-temperature mechanical properties. Appl. Phys. Lett. 90, 181904. doi:10.1063/1.2734517
Zhu, M., Zhao, B., Yuan, Y., Guo, S., and Wei, G. (2021). Study on corrosion behavior and mechanism of CoCrFeMnNi HEA interfered by AC current in simulated alkaline soil environment. J. Electroanal. Chem. 882, 115026. doi:10.1016/J.JELECHEM.2021.115026
Keywords: biological high-entrogy, compositon design, mechanical properties, implant, biocompatibility
Citation: Feng J, Tang Y, Liu J, Zhang P, Liu C and Wang L (2022) Bio-high entropy alloys: Progress, challenges, and opportunities. Front. Bioeng. Biotechnol. 10:977282. doi: 10.3389/fbioe.2022.977282
Received: 24 June 2022; Accepted: 01 August 2022;
Published: 08 September 2022.
Edited by:
Daixiu Wei, Tohoku University, JapanReviewed by:
Yuting Lv, Shandong University of Science and Technology, ChinaCopyright © 2022 Feng, Tang, Liu, Zhang, Liu and Wang. This is an open-access article distributed under the terms of the Creative Commons Attribution License (CC BY). The use, distribution or reproduction in other forums is permitted, provided the original author(s) and the copyright owner(s) are credited and that the original publication in this journal is cited, in accordance with accepted academic practice. No use, distribution or reproduction is permitted which does not comply with these terms.
*Correspondence: Yujin Tang, dGFuZ3l1amluQHltY24uZWR1LmNu; Jia Liu, bGl1amlhQHltY24uZWR1LmNu; Peilei Zhang, cGVpbGVpQHN1ZXMuZWR1LmNu
Disclaimer: All claims expressed in this article are solely those of the authors and do not necessarily represent those of their affiliated organizations, or those of the publisher, the editors and the reviewers. Any product that may be evaluated in this article or claim that may be made by its manufacturer is not guaranteed or endorsed by the publisher.
Research integrity at Frontiers
Learn more about the work of our research integrity team to safeguard the quality of each article we publish.