- 1Institude of Translation Medicine, Shanghai University, Shanghai, China
- 2Department of Pharmacy, Medical Supplies Center of PLA General Hospital, Beijing, China
The past decade has become an important strategy in precision medicine for the targeted therapy of many diseases, expecially various types of cancer. As a promising targeted element, nucleic acid aptamers are single-stranded functional oligonucleotides which have specific abilities to bind with various target molecules ranging from small molecules to entire organisms. They are often named ‘chemical antibody’ and have aroused extensive interest in diverse clinical studies on account of their advantages, such as considerable biostability, versatile chemical modification, low immunogenicity and quick tissue penetration. Thus, aptamer-embedded drug delivery systems offer an unprecedented opportunity in bioanalysis and biomedicine. In this short review, we endeavor to discuss the recent advances in aptamer-based targeted drug delivery platforms for cancer therapy. Some perspectives on the advantages, challenges and opportunities are also presented.
1 Introduction
Aptamers are a special class of DNA or RNA oligonucleotides that fold up into unique three-dimensional (3D) conformations for specifically recognizing cognate molecular targets (Miao et al., 2021). Aptamers are usually screened via an in vitro iterative method named Systematic Evolution of Ligands by EXponential Enrichment (SELEX) (Tan et al., 2013), which was independently discovered by two American groups in early 1990s (Ni et al., 2011). In recent years, various aptamers have been isolated for diverse types of target molecules (Table 1), including organic and inorganic molecules, peptides, proteins, nucleic acids, bacterium and even live cells, such as EpCAM aptamer binds to epithelial cell adhesion molecules (EpCAM) and aptamer sgc8 against protein tyrosine kinase-7 (PTK-7) (Ni et al., 2021). Compared with other targeted ligands, aptamers possess many excellent properties, including high chemical stability and binding affinity, versatile chemical modification, low or even non immunogenicity, small size and quick tissue penetration (Yang et al., 2022). These remarkable advantages make aptamers widely used in the field of cancer targeted therapy (Sun et al., 2022) and diagnosis (Zhang et al., 2020). This review will predominantly provide a brief overview of recent researches on aptamer-based targeted systems for cancer therapy. The future possibilities and challenges of aptamer guided drug delivery system will also be discussed.
2 Aptamers as therapeutic agents
Aptamers, as therapeutic agents, can effectively recognize various proteins on the cell membrane or in the blood circulation to modulate their interaction with receptors and affect the corresponding biological pathways for the treatment of various diseases (Zhou and Rossi, 2017). The ongoing progresses in biomedical technology are encouraging the development of aptamers as therapeutic agents for improving human health. Over the past few decades, the number of therapeutic aptamers in clinical stages has been increasing (Nimjee1 et al., 2017). In 2004, Pegaptanib (Macugen), as the first aptamer in clinical use, was approved by the FDA to treat Age-related macular degeneration (AMD), which was known to the leading reason of blindness in many aging people (Ng et al., 2006). Vascular endothelial growth factor (VEGF) can increase vascular permeability and induce angiogenesis, leading to AMD (Ucuzian et al., 2010). Pegaptanib as an anti-VEGF antagonist aptamer can specifically block VEGF and interfere with the interaction of VEGF and its receptors to treat AMD. For increasing its in vivo biostability, 40 kDa monomethoxypolyethylene glycol (PEG) was then conjugated with pegatanib to decrease nuclease degradation. However, the antibody fragment ranibizumab (Lucentis; Genentech) has recently occupied significant market due to blocking all types of human VEGF even and the smallest VEGF121 (Martin et al., 2011).
Another famous therapeutic aptamer AS1411 has been in clinical phase II trial for the treatment of metastatic renal cell carcinoma. AS1411 composed of thymine and guanines can form special guanine-mediated quadraplex structures in solution (Ireson and Kelland, 2006). Due to this unique three-dimensional (3D) structures, AS1411 can target to nucleolin protein with high specificity, which was normally found overexpressing on the surface of cancer cells. Unlike other aptamers, AS1411 was discovered by screening antisense oligonucleotides for antiproliferation effect (Bates et al., 1999). Although the underlying mechanisms of AS1411-based antiproliferation effect have not been fully comprehended, it has showed growth-antitumor abilities against a widely range of tumor cells through multiple signaling pathways involving BCL-2 mRNA destabilization and NF-κB inhibition (Soundararajan et al., 2008). Some studies have proved that guanine deaminase is an important pathway in affecting the cell-type selectivity to the anti-proliferation function of guanine-based biomolecules (Wang et al., 2019). So this rich guanine aptamer, as one of the most promising aptamers, has great hope to be used in clinical cancer therapy owing to its outstanding safety profile and anticancer ability in some intractable tumors (Zhang et al., 2015). In addition, there were some studies about AS1411 derivatives which were obtained by chemical modification with alternative nucleobases or backbones for improving chemical and biological properties. In 2016, Fan et al. reported the first AS1411 derivative that showed excellent ability in the inhibition of DNA replication and tumor cell growth, and induced S-phase cell cycle arrest via chemical modification of 2′-deoxyinosine in AS1411 aptamer (Cai et al., 2014). Subsequently, they also developed another strategy to modify AS1411 aptamer through the use of 2′-deoxyinosine (2′-dI) and D-/l-isothymidine (D-/L-isoT) for improving the bioactivity of AS1411 aptamer (Fan, Sun, Wu, Zhang, Yang). In addition to exploring aptamers for directly inhibiting cancer cells growth, aptamers can also indirectly display anticancer abilities through modulating the immune system. In recent years, some agonistic aptamers with immunomodulatory properties have been found. It is worth noting that these aptamers recognizing 4-1BB or OX40 almost show similar or even superior immunomodulatory ability to the corresponding antibodies, followed with similar anticancer effects. Examples of this type of aptamers include multimeric aptamers that can target 4-1BB (CD137) on activated T cells and improve T cell proliferation, IL-2 secretion, survival and cytolytic activity of T cells (McNamara et al., 2008).
3 Aptamer-drug conjugates for targeted drug delivery
In addition to being therapeutic agents, aptamers have been more widely explored as targeting carriers for the therapeutic agents delivery, such as chemotherapeutics, small interfering RNAs (siRNAs), microRNAs (miRNAs), toxins and so on (Xuan et al., 2018). Traditional ApDCs are mostly comprised of aptamers attached to various potent drugs through all kinds of cleavable or non-cleavable linkers. Compared with antibody-drug conjugates (ADCs), a few of which have been applied to clinical treatment of cancer, ApDCs show many significant advantages, including relatively small size, synthesis procedure economy, chemical modification simplicity and tissue penetration speedy (Chen et al., 2017). Based on diseased-related biomarkers, ApDCs have been developed for a wide range of therapeutic modalities, such as chemotherapy, immunotherapy and so on.
Chemotherapy is one of the most fashional therapeutic modalities for cancer, but this conventional strategy suffers from serious drug toxicity in healthy tissues and various side effects. For improving therapeutic efficacy and diminishing side effects, ApDC-mediated targeted drug delivery has been studied. As an example, Tan et al. designed and synthesized a sgc8-Dox conjugate for targeted delivery of doxorubicin (Dox) (Huang et al., 2009). In this ApDCs, antitumor agent Dox was conjugated with aptamer sgc8 at a 1:1 ratio via an acid-labile hydrazone linker, such that Dox can be selectively delivered in acidic tumor environment. Zhang et al. reported a water-soluble nucleolin aptamer-paclitaxel conjugate that can specifically release PTX to the tumor site via a cathepsin B-labile valine-citrulline dipeptide linker (Li et al., 2017). Recently, a nucleolin aptamer (AS1411) loaded with BET-targeting PROTAC against breast cancer stem cells was reported by Sheng et al (He et al., 2021). Notably, the aptamer/drug ratio is essential in achieving excellent therapeutic efficacy. For maximizing drug delivery efficiency, a phosphoramidite prodrug of 5-fluorouracil (5-FU) was developed and the resulting ApDCs with multiple drug copies can be synthesized via automated nucleic acid synthesis using standard solid-phase DNA synthesis chemistry (Wang et al., 2014b). In order to control drug release, a photocleavable linker was added to the bone of phosphoramidite prodrug. As a result, the ApDCs not only were efficiently internalized into cancer cells, but also showed specific drug release in a photocontrollable manner.
Besides conjugating with chemotherapeutic drugs, aptamer can also link with therapeutic RNA or DNA. In recent years, gene therapy as a hot therapeutic modality has made great breakthrough in the treatment of cancer (Song et al., 2021). However, just like many other therapeutic drugs, most of gene therapy agents lack specific recognition ability for the disease tissues, which make it vital to specifically deliver gene therapy agents to cancer cells. As target ligands, aptamers can be utilized to improve the gene therapy safety and therapeutic efficacy (Li et al., 2013). In an early research, an ApDCs was constructed using a PSMA-targeting aptamer and a small interfering RNA (siRNA), which can silence polo-like kinase 1 (PLK1) and B-cell lymphoma 2 (BLC2) overexpressed in most human tumor cells (McNamara et al., 2006). In this study, the resulting ApDCs can not only specifically release siRNA into PSMA-positive LNCaP cells and lead to cell apoptosis, but also remarkably inhibit tumor growth in LNCaP tumor-bearing mices. Subsequently, Aptamer-siRNA conjugates have been systematically studied by PEGlation to optimize circulation half-life in the blood, by chemical modification to increase biostability, and by exploring the two-dimensional structure to improve the intracellular processing of RNA-induced genes silencing. In another research, a multiple mucin-1 aptamer was conjugated with BCL2-specific siRNA, and doxorubicin (Dox) was loaded into these conjugates through intercalation with nucleic acids (Jeong et al., 2017). These Dox-incorporated multivalent Apt-siRNA conjugates can overcome multidrug resistance into MDR cancer cells through aptamer-mediated codelivery of Dox and siRNA. Note that the 3D structure of multivalent Dox-Apt-siRNA were well defined, which is beneficial for their clinical application. Furthermore, aptamers were also successfully conjugated with other nucleic acid gene therapeutics, such as small hairpin RNA (shRNA) and microRNA (miRNA) (Soldevilla et al., 2018).
4 Aptamer-based nanomaterial system for targeted drug delivery
Nanomaterials play a crucial role in the application of bioanalysis and biomedicine (Li et al., 2021). Due to their unique physicochemical properties, including an ultra-small size, a large surface area and loading ability, nanomaterials have overcome many limitations of conventional therapeutic and diagnostic strategies (Dong et al., 2020). The key of nanomedicine development is to improve the specific recognition ability for disease tissues (Liu et al., 2021). The combination of aptamers and nanomaterials is a promising progress for targeted drug delivery (Figure 1) (Mahmoudpoura et al., 2021). In this section, several representative aptamer-based inorganic and organic nanomaterials on cancer therapy would be discussed.
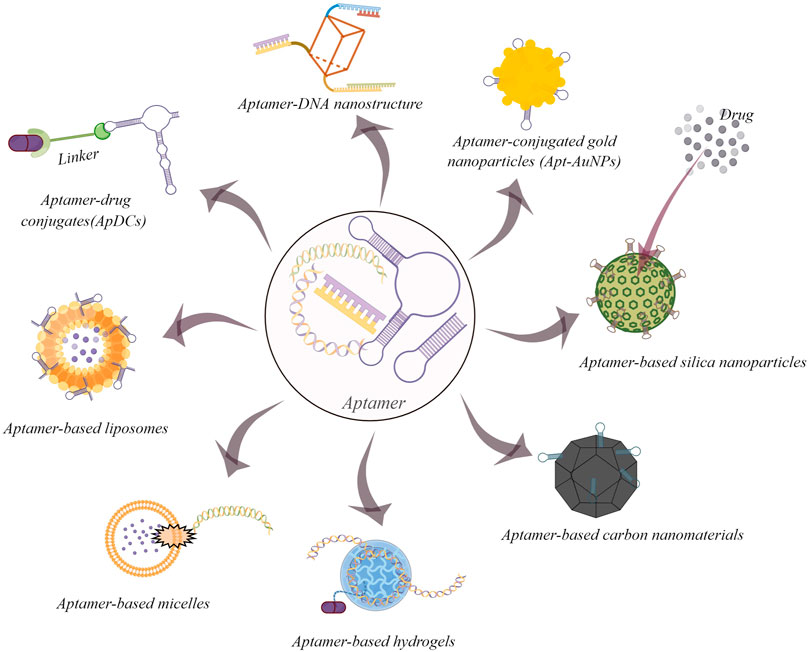
FIGURE 1. Some common examples of aptamer-based drug delivery systems for cancer therapy (By figdraw).
4.1 Aptamer-based inorganic nanomaterial systems
As an important inorganic nanomaterial, gold nanoparticles have gained considerable attentions in biomedicine as result of their high surface-to-volume ratio, low-toxicity, excellent stability and biological compatibility (Yang et al., 2015a). Aptamer-conjugated gold nanomaterials (Apt-AuNPs), which synergically possess special advantageous properties of aptamers and gold nanoparticles, have been widely utilized in the field of cancer diagnosis and therapy (Nooranian et al., 2021). A classical research from the Mirkin group employed target DNA molecules to form a polymeric network of nanoparticles for specifically detecting polynucleotide (Elghanian et al., 1997). Subsequently, there are emerging many corresponding studies, such as enzyme responsive Apt-AuNPs for mucin 1 protein (MUC1) detection (Hu et al., 2014), Apt-AuNPs combined with graphene oxide for the photothermal therapy of breast cancer (Yang et al., 2015b) and aptamer-functionalized AuNPs-Fe3O4-GS capture probe for monitoring circulating tumor cell in whole blood (Dou et al., 2019).
Among the set of inorganic nanomedicine, silica nanoparticles have become suitable carriers in drug delivery systems (Zou et al., 2020). These particles successfully provided controllable drug release in vivo and in vitro through the change in PH and temperature, photochemical reactions and certain redox reactions (Fu and Xiang, 2020). After combined with targeted elements such as aptamers, they can enhance cancer therapeutic effects with a lower dose of drug (Vandghanooni et al., 2020). As an early example, Cai’s group reported a novel Mesoporous Silica Nanoparticles (MSN)-based redox-responsive nanocontainer for triplex cancer targeted therapy. In their study, AS1411 aptamer was tailored onto the CytC-sealed MSNs (Zhang et al., 2014a). And this system can lead to the special release of Dox into the tumor cells via the breakage of S-S bonds. In 2021, Tan et al. first developed FRET-based two-photon MSNs for multiplexed intracellular imaging and targeted drug delivery (Wu et al., 2021). The MSNs can display different two-photon multicolor fluoresence by varying the doping ratio of the three dyes. Furthermore, the Dox-loaded and aptamer-capped nanosystem can be efficiently internalized into the cancer cells and release the anticancer drug Dox. In addition, aptamer-targeted MSNs have also been widely used for gene targeted delivery, which can protect gene therapy agents from degradation by nuclease (Zhang et al., 2014b).
Conventional carbon nanomaterials, including fullerene, graphene, carbon dots/nanobots/nanotubes and hybrids, exhibit unique advantages in biomedical application (Weng et al., 2018). Aptamer-functionalized carbon nanomaterials make their ideal nanoplatforms for cancer diagnostics and therapeutics (Yang et al., 2018). Recently, Wang et al. developed the multifunctional, which showed heat-stimulative and sustained release properties (Wang et al., 2015). With the introduction of MUC1 aptamers, this nanoparticle can detect targeted MCF-7 breast cells with excellent recognition ability. In addition, aptamer-based graphene nanomaterials have gained many fascinating developments in cancer gene therapy. In 2017, aptamer-based graphene quantum dots loaded with porphyrin derivatives photosensitizer were reported for fluorescence-guided photothermal/photodynamic synergetic therapy (Cao et al., 2017). This multifunctional theranostic nanomaterials displayed good feasibility for detecting intracellular cancer-related miRNA, whereas the intrinsic fluorescence could be used to distinguish cancer cells from somatic cells.
4.2 Aptamer-based organic nanomaterial systems
As the first explored drug delivery system, liposomes have many promising properties such as good biocompatibility, low toxicity, low immunogencity and excellent drug loading efficiency (Moosaviana and Sahebkar, 2019). PEGylated liposomal doxorubicin, Doxil®, is the first FDA-approved liposomal drug for the treatment of solid tumors. With the rapid development of biotechnology, liposomal systems with specific targeting ability have been synthesized successfully by the introduction of various molecular recognition elements, such as folate, peptides, antibodies, and aptamers. Among them, aptamer-based lipsomes have attracted widely attention (Zhao et al., 2019). In the early research, Tan et al. reported a therapeutic aptamer-modified liposome nanoparticle with dual-fluorophore labeling for targeted drug delivery (Kang et al., 2010). This system was conjugated with a sgc8 aptamer that showed high binding and internalization ability for targeted CEM cells. The flow cytometry and confocal imaging experiments showed sgc8-modified liposomes could deliver loaded drug to targeted cancer cells with high specificity and excellent efficiency. In recent attempts, the CRISPR/Cas9 complex were packaged into aptamer-functionalized liposomes for specific cancer gene therapy (Gong et al., 2020). For example, Liang et al. developed an aptamer-based lipopolymer for tumor-specific delivery of CRISPR/Cas9 to regulate VEGFA in osteosarcoma (Liang et al., 2017). In this system, LC09 aptamer could facilitate the selective distribution of CRISPR/Cas9 plasmids to decrease VEGFA expression, leading to inhibite orthotopic osteosarcoma malignancy and lung metastasis.
Another promising type of aptamer-based organic nanomaterial is the micelle structure. This drug delivery system displays excellent binding ability of aptamers to target due to the multivalent effect. Thus, it can be developed for numerous bioapplications (Wu et al., 2010). In 2018, Li et al. developed a cross-linked aptamer-lipid micelle system for excellent stability and specificity in target-cell recognition (Li et al., 2018a). In this facile approach, aptamer and lipid segments were linked to a methacrylamide branch via an efficient photoinduced polymerization process. In contrast to traditional aptamer-lipid micelles, this reported system provided better biostability in a cellular environment, further improving the targeting ability for imaging applications. In another fashion study, a novel aptamer-prodrug conjugate micelle was prepared by combining hydrophobic prodrug bases and bioorthogonal chemistry for hydrogen peroxide and pH-independent cancer chemodynamic therapy (Xuan et al., 2020). In depth mechanistic work reveal that, this system could be activated by intracellular Fe2+ to generate toxic C-centered free radicals self-circularly via cascading bioorthogonal reactions.
Among aptamer-based organic nanomaterial systems, target-responsive DNA hydrogels exhibited superior mechanical properties and programmable features and were widely used in biomedical and pharmaceutical applications (Li et al., 2016). In 2008, the first adenosine-responsive hydrogel was developed for potential drug release. In this work, two oligonucleotide-incorporated polyacrylamide and rationally designed cross-linking oligonucleotides were used to form the DNA nanohydrogels. The DNA linker contained the aptamer sequence for adenosine. When existing adenosine molecules, the aptamer will bind to target molecules, resulting in the breakdown of the cross-links and the dissolution of the hydrogel. Thus, this system could be explored for target-responsive drug release (Yang et al., 2008). In other elegant example, Yao et al. reported a physically cross-linked DNA network to fish bone marrow mesenchymal stem cells (BMSCs) from numerous nontarget cells (Yao et al., 2020). This nanomaterial containing a Apt19S aptamer sequence provided a 3D microenvironment to maintain excellent activity of captured stem cells.
In addition, due to the principle of complementary base pairing, aptamers can be easily integrated to prepare various DNA nanostructures for specific cancer cell recognition and subsequent applications (Seeman and Sleiman, 2018). As an important DNA-based nanostructures, DNA origami have been modified with various small molecule drugs, functional NA sequences, and nanomaterials (Bolaños Quiñones et al., 2018). In 2018, a smart DNA nanorobot was reported by Li’s group for intelligent drug delivery in cancer therapy. Because of functionalizing on the outside with aptamer AS1411, this DNA nanorobot can specifaically deliver thrombin to tumor-associated blood vessels for inhibiting the tumor growth (Li et al., 2018b). Subsquently, the aptamer-functionalized DNA Origami, named Apt-Dox-orgami-ASO, was developed by Pan’s group to co-deliver Dox and antisense oligonucleotides (ASOs) in cancer cells. This multifunctional DNA origami-based nanocarrier was precisely synthesized to adsorb Dox and load Bcl2 and P-gp ASOs for the efficient therapy of drug-resistant cancer (Pan et al., 2020). As cargo carriers, all kinds of aptamer-based DNA nanostructures have been explored By molecular engineering for targeted drug delivery in cancer therapy (Hu et al., 2018).
5 Conclusion
In the past decades, the achieved developments proved that aptamers had broad potential in the research field of cancer therapy. Multiple unique properties of aptamers attracted considerable attention in the development of aptamers as nucleic acids-functionalized alternatives to folic acid, peptides and antibodies for targeted drug delivery. This short review summarizes some recent advances of aptamer-based systems in cancer therapy. In fact, the exploration of aptamers and aptamer-drug conjugates is still in a relatively early stage. Considerable efforts should be made to overcome their bottlenecks in the clinical application. In addition, the most remarkable achievements of aptamers involved their combination with nanomaterials, enhancing the specificity of the diagnostic signal and leading to excellent target cancer cell recognition and delivery. In summary, the above description showed the versatility and therapeutic applicability of aptamers. However, multiple challenges, including poor biostability, short half-lives in vivo and unclear mechanism of endosomal escape and drug release, need to be overcome before moving forward to clinical application. Furthermore, more systematic research on organ toxicity, the safety on genomics and proteomics, the large-scale production technology and costs need to be further investigated. Despite these limitations, the rapid development of chemistry and materials encourages us to explore aptamer-based drug delivery systems with high therapeutic effects.
Author contributions
FG, JY and YC contributed equally. FG contributed to the conception. JY and YC drew the figures and wrote the article. CG revised the manuscript. All authors read and approved the submitted version.
Funding
The authors acknowledge the financial support from the National Natural Science Foundation of Shanghai (No. 21ZR1422600) and China NSFC (Nos. 2210070260).
Conflict of interest
The authors declare that the research was conducted in the absence of any commercial or financial relationships that could be construed as a potential conflict of interest.
The handling editor RW declared a past co-authorship with the author FG.
Publisher’s note
All claims expressed in this article are solely those of the authors and do not necessarily represent those of their affiliated organizations, or those of the publisher, the editors and the reviewers. Any product that may be evaluated in this article, or claim that may be made by its manufacturer, is not guaranteed or endorsed by the publisher.
References
Bates, P. J., Kahlon, J. B., Thomas, S. D., Trent, J. O., and Miller, D. M. (1999). Antiproliferative activity of G-rich oligonucleotides correlates with protein binding. J. Biol. Chem. 274, 26369–26377. doi:10.1074/jbc.274.37.26369
Bates, P. J., Laber, D. A., Miller, D. M., Thomas, S. D., and Trent, J. O. (2009). Discovery and development of the G-rich oligonucleotide AS1411 as a novel treatment for cancer. Exp. Mol. Pathol. 86, 151–164. doi:10.1016/j.yexmp.2009.01.004
Bolaños Quiñones, V. A., Zhu, H., Solovev, A. A., Mei, Y., and Gracias, D. H. (2018). Origami biosystems: 3D assembly methods for biomedical applications. Adv. Biosyst. 2, 201800230. doi:10.1002/adbi.201800230
Cai, B., Yang, X., Sun, L., Fan, X., Li, L., Jin, H., et al. (2014). Stability and bioactivity of thrombin binding aptamers modified with D-/L-isothymidine in the loop regions. Org. Biomol. Chem. 12, 8866–8876. doi:10.1039/C4OB01525H
Cao, Y., Dong, H., Yang, Z., Zhong, X., Chen, Y., Dai, W., et al. (2017). Aptamer-conjugated graphene quantum dots/porphyrin derivative theranostic agent for intracellular cancer-related MicroRNA detection and fluorescence-guided photothermal/photodynamic synergetic therapy. ACS Appl. Mat. Interfaces 9, 159–166. doi:10.1021/acsami.6b13150
Chen, C.-h. B., Chernis, G. A., Hoang, V. Q., and Landgraf, R. (2003). Inhibition of heregulin signaling by an aptamer that preferentially binds to the oligomeric form of human epidermal growth factor receptor-3. Proc. Natl. Acad. Sci. U. S. A. 100, 9226–9231. doi:10.1073/pnas.1332660100
Chen, K., Liu, B., Yu, B., Zhong, W., Lu, Y., Zhang, J. N., et al. (2017). Advances in the development of aptamer drug conjugates for targeted drug delivery. Wiley Interdiscip. Rev. Nanomed. Nanobiotechnol. 9, e1438. doi:10.1002/wnan.1438
Chi-hong, B. C., George, A. C., Van, Q. H., and Ralf, L. (2003). Inhibition of heregulin signaling by an aptamer that preferentially binds to the oligomeric form of human epidermal growth factor receptor-3. Proc. Natl. Acad. Sci. U. S. A. 100, 9226–9231. doi:10.1073/pnas.1332660100
Dong, Y., Yao, C., Zhu, Y., Yang, L., Luo, D., Yang, D., et al. (2020). DNA functional materials assembled from branched DNA: Design, synthesis, and applications. Chem. Rev. 120, 9420–9481. doi:10.1021/acs.chemrev.0c00294
Dou, B., Xu, L., Jiang, B., Yuan, R., and Xiang, Y. (2019). Aptamer-functionalized and gold nanoparticle array-decorated magnetic graphene nanosheets enable multiplexed and sensitive electrochemical detection of rare circulating tumor cells in whole blood. Anal. Chem. 91, 10792–10799. doi:10.1021/acs.analchem.9b02403
Elghanian, R., Storhoff, J. J., Mucic, R. C., Letsinger, R. L., and Mirkin, C. (1997). Selective colorimetric detection of polynucleotides based on the distance-dependent optical properties of gold nanoparticles. Science 277, 1078–1081. doi:10.1126/science.277.5329.1078
Esposito, C. L., Passaro, D., Longobardo, I., Condorelli, G., Marotta, P., Affuso, A., et al. (2011). A neutralizing RNA aptamer against EGFR causes selective apoptotic cell death. PLoS One 6, e24071. doi:10.1371/journal.pone.0024071
Fan, X., Sun, L., Wu, Y., Zhang, L., and Yang, Z. (2016). Bioactivity of 2′- deoxyinosineincorporated aptamer AS1411. Sci. Rep. 6, 25799. doi:10.1038/srep25799
Ferreira, C. S., Matthews, C. S., and Missailidi, S. (2006). DNA aptamers that bind to MUC1 tumour marker: Design and characterization of MUC1-binding single-stranded DNA aptamers. Tumor Biol. 27, 289–301. doi:10.1159/000096085
Fu, Z., and Xiang, J. (2020). Aptamer-functionalized nanoparticles in targeted delivery and cancer therapy. Int. J. Mol. Sci. 21, 9123. doi:10.3390/ijms21239123
Gefen, T., Castro, I., Muharemagic, D., Puplampu-Dove, Y., Patel, S., Gilboa, E., et al. (2017). A TIM-3 oligonucleotide aptamer enhances T cell functions and potentiates tumor immunity in mice. Mol. Ther. 25, 2280–2288. doi:10.1016/j.ymthe.2017.06.023
Gong, Y., Tian, S., Xuan, Y., and Zhang, S. (2020). Lipid and polymer mediated CRISPR/Cas9 gene editing. J. Mat. Chem. B 8, 4369–4386. doi:10.1039/D0TB00207K
Gragoudas, E. S., Adamis, A. P., Cunningham, E. T., Feinsod, M., and Guyer, D. R. (2004). Pegaptanib for neovascular age-related macular degeneration. N. Engl. J. Med. 351, 2805–2816. doi:10.1056/nejmoa042760
He, S., Gao, F., Ma, J., Ma, H., Dong, G., Sheng, C., et al. (2021). Aptamer-PROTAC conjugates (APCs) for tumor-specific targeting in breast cancer. Angew. Chem. Int. Ed. 60, 23299–23305. doi:10.1002/anie.202107347
Herrmann, A., Priceman, S. J., Swiderski, P., Kujawski, M., Xin, H., Zhang, W., et al. (2014). CTLA4 aptamer delivers STAT3 siRNA to tumor-associated and malignant T cells. J. Clin. Invest. 124, 2977–2987. doi:10.1172/JCI73174
Hu, Q., Wang, S., Wang, L., Gu, H., and Fan, C. (2018). DNA nanostructure-based systems for intelligent delivery of therapeutic oligonucleotides. Adv. Healthc. Mat. 7, 1701153. doi:10.1002/adhm.201701153
Hu, R., Wen, W., Wang, Q., Xiong, H., Zhang, X., Gu, H., et al. (2014). Novel electrochemical aptamer biosensor based on an enzyme-gold nanoparticle dual label for the ultrasensitive detection of epithelial tumour marker MUC1. Biosens. Bioelectron. X. 53, 384–389. doi:10.1016/j.bios.2013.10.015
Huang, B. T., Lai, W. Y., Chang, Y. C., Wang, J. W., Yeh, S. D., Lin, E. P. Y., et al. (2017). A CTLA-4 antagonizing DNA aptamer with antitumor effect. Mol. Ther. - Nucleic Acids 8, 520–528. doi:10.1016/j.omtn.2017.08.006
Huang, Y.-F., Shangguan, D., Liu, H., Phillips, J. A., Zhang, X., Chen, Y., et al. (2009). Molecular assembly of an aptamer–drug conjugate for targeted drug delivery to tumor cells. ChemBioChem 10, 862–868. doi:10.1002/cbic.200800805
Ireson, C. R., and Kelland, L. R. (2006). Discovery and development of anticancer aptamers. Mol. Cancer Ther. 5, 2957–2962. doi:10.1158/1535-7163.MCT-06-0172
Ishizaki, J., Nevins, J. R., and Sullenger, B. A. (1996). Inhibition of cell proliferation by an RNA ligand that selectively blocks E2F function. Nat. Med. 2, 1386–1389. doi:10.1038/nm1296-1386
Jeong, H., Lee, S. H., Hwang, Y., Yoo, H., Juang, H., Kim, S. H., et al. (2017). Multivalent aptamer–RNA conjugates for simple and efficient delivery of doxorubicin/siRNA into multidrug-resistant cells. Macromol. Biosci. 17, 1600343. doi:10.1002/mabi.201600343
Kang, H., O’Donoghue, M. B., Liu, H., and Tan, W. (2010). A liposome-based nanostructure for aptamer directed delivery. Chem. Commun. 46, 249–251. doi:10.1039/b916911c
Kruspe, S., Meyer, C., and Hahn, U. (2014). Chlorin e6 Conjugated Interleukin-6 Receptor Aptamers Selectively Kill Target Cells upon Irradiation. Mol. Ther. - Nucleic Acids 3, e143. doi:10.1038/mtna.2013.70
Lai, W. Y., Huang, B. T., Wang, J. W., Lin, P. Y., and Yang, P. C. (2016). A novel PD-L1-targeting antagonistic DNA aptamer with antitumor effects. Mol. Ther. - Nucleic Acids 5, e397. doi:10.1038/mtna.2016.102
Lebruska, L. L., and Maher, L. J. (1999). Selection and characterization of an RNA decoy for transcription factor NF-κB. Biochemistry 38, 3168–3174. doi:10.1021/bi982515x
Li, F., Lu, J., Liu, J., Liang, C., Wang, M., Wang, L., et al. (2017). A water-soluble nucleolin aptamer-paclitaxel conjugate for tumor-specific targeting in ovarian cancer. Nat. Commun. 8, 1390. doi:10.1038/s41467-017-01565-6
Li, J., Mo, L., Lu, C.-H., Fu, T., Yang, H.-H., Tan, W., et al. (2016). Functional nucleic acid-based hydrogels for bioanalytical and biomedical applications. Chem. Soc. Rev. 45, 1410–1431. doi:10.1039/c5cs00586h
Li, N., Nguyen, H. H., Byrom, M., and Ellington, A. D. (2011). Inhibition of cell proliferation by an anti-EGFR aptamer. PLoS One 6, e20299. doi:10.1371/journal.pone.0020299
Li, S., Jiang, Q., Liu, S., Zhang, Y., Tian, Y., Song, C., et al. (2018). A DNA nanorobot functions as a cancer therapeutic in response to a molecular trigger in vivo. Nat. Biotechnol. 36, 258–264. doi:10.1038/nbt.4071
Li, S. L., Jiang, P., Jiang, F. L., and Liu, Y. (2021). Recent advances in nanomaterial-based nanoplatforms for chemodynamic cancer therapy. Adv. Funct. Mat. 31, 2100243. doi:10.1002/adfm.202100243
Li, X., Figg, C. A., Wang, R., Jiang, Y., Lyu, Y., Sun, H., et al. (2018). Cross-linked aptamer–lipid micelles for excellent stability and specificity in target-cell recognition. Angew. Chem. Int. Ed. 130, 11589–11593. doi:10.1002/anie.201804682
Li, X., Zhao, Q., and Qiu, L. (2013). Smart ligand: Aptamer-mediated targeted delivery of chemotherapeutic drugs and siRNA for cancer therapy. J. Control. Release 171, 152–162. doi:10.1016/j.jconrel.2013.06.006
Liang, C., Li, F., Wang, L., Zhang, Z.-K., Wang, C., He, B., et al. (2017). Tumor cell-targeted delivery of CRISPR/Cas9 by aptamer-functionalized lipopolymer for therapeutic genome editing of VEGFA in osteosarcoma. Biomaterials 147, 68–85. doi:10.1016/j.biomaterials.2017.09.015
Liu, H., Zhang, Q., Wang, S., Weng, W., Jing, Y., Su, J., et al. (2021). Bacterial extracellular vesicles as bioactive nanocarriers for drug delivery: Advances and perspectives. Bioact. Mat. 14, 169–181. doi:10.1016/j.bioactmat.2021.12.006
Liu, Y. J., Dou, X. Q., Wang, F., Zhang, J., Wang, X. L., Xu, G. L., et al. (2017). IL-4Rα aptamer-liposome-CpG oligodeoxynucleotides suppress tumour growth by targeting the tumour microenvironment. J. Drug Target. 25, 275–283. doi:10.1080/1061186X.2016.1258569
Lozano, T., Soldevilla, M. M., Casares, N., Villanueva, H., Bendandi, M., Lasarte, J. J., et al. (2016). Targeting inhibition of foxp3 by a CD28 2'-fluro oligonucleotide aptamer conjugated to P60-peptide enhances active cancer immunotherapy. Biomaterials 91, 73–80. doi:10.1016/j.biomaterials.2016.03.007
Lupold, S. E., Hicke, B. J., Lin, Y., and Coffey, D. S. (2002). Identification and characterization of nuclease-stabilized RNA molecules that bind human prostate cancer cells via the prostate-specific membrane antigen. Cancer Res. 62, 4029–4033.
Mahmoudpoura, M., Dingd, S., Lyud, Z., Ebrahimie, G., Dud, D., Dolatabadif, J. E. N., et al. (2021). Aptamer functionalized nanomaterials for biomedical applications: Recent advances and new horizons. Nano Today 39, 101177. doi:10.1016/j.nantod.2021.101177
Mallikaratchy, P., Tang, Z., Kwame, S., Meng, L., Shangguan, D., Tan, W., et al. (2007). Aptamer directly evolved from live cells recognizes membrane bound immunoglobin heavy mu chain in burkitt's lymphoma cells. Mol. Cell. Proteomics 6, 2230–2238. doi:10.1074/mcp.M700026-MCP200
Martell, R. E., Nevins, J. R., and Sullenger, B. A. (2002). Optimizing aptamer activity for gene therapy applications using expression cassette SELEX. Mol. Ther. 6, 30–34. doi:10.1006/mthe.2002.0624
Martin, D. F., Maguire, M. G., Ying, G. s., Grunwald, J. E., Fine, S. L., and Jaffe, G. J. (2011). Ranibizumab and bevacizumab for neovascular age-related macular degeneration. N. Engl. J. Med. 364, 1897–1908. doi:10.1056/NEJMoa1102673
McNamara, J. O., Andrechek, E. R., Wang, Y., Viles, K. D., Rempel, R. E., Gilboa, E., et al. (2006). Cell type–specific delivery of siRNAs with aptamer-siRNA chimeras. Nat. Biotechnol. 24, 1005–1015. doi:10.1038/nbt1223
McNamara, J. O., Kolonias, D., Pastor, F., Mittler, R. S., Chen, L., Giangrande, P. H., et al. (2008). Multivalent 4-1BB binding aptamers costimulate CD8+ T cells and inhibit tumor growth in mice. J. Clin. Invest. 118, 376–386. doi:10.1172/JCI33365
Mi, J., Zhang, X., Giangrande, P. H., McNamara, J. O., Nimjee, S. M., Sarraf-Yazdi, S., et al. (2005). Targeted inhibition of αvβ3 integrin with an RNA aptamer impairs endothelial cell growth and survival. Biochem. Biophys. Res. Commun. 338, 956–963. doi:10.1016/j.bbrc.2005.10.043
Miao, Y., Gao, Q., Mao, M., Zhang, C., Yang, L., Yang, Y., et al. (2021). Bispecific aptamer chimeras enable targeted protein degradation on cell membranes. Angew. Chem. Int. Ed. 60, 11267–11271. doi:10.1002/anie.202102170
Moosaviana, S. A., and Sahebkar, A. (2019). Aptamer-functionalized liposomes for targeted cancer therapy. Cancer Lett. 448, 144–154. doi:10.1016/j.canlet.2019.01.045
Ng, E. W. M., Shima, D. T., Calias, P., Cunningham, E. T., Guyer, D. R., Adamis, A. P., et al. (2006). Pegaptanib, a targeted anti-VEGF aptamer for ocular vascular disease. Nat. Rev. Drug Discov. 5, 123–132. doi:10.1038/nrd1955
Ni, S., Zhuo, Z., Pan, Y., Yu, Y., Li, F., Liu, J., et al. (2021). Recent progress in aptamer discoveries and modifications for therapeutic applications. ACS Appl. Mat. Interfaces 13, 9500–9519. doi:10.1021/acsami.0c05750
Ni, X., Castanares, M., Mukherjee, A., and Lupold, S. E. (2011). Nucleic acid aptamers: Clinical applications and promising new horizons. Curr. Med. Chem. 18, 4206–4214. doi:10.2174/092986711797189600
Nimjee1, S. M., White, R. R., Becker, R. C., and Sullenger, B. A. (2017). Aptamers as therapeutics. Annu. Rev. Pharmacol. Toxicol. 57, 61–79. doi:10.1146/annurev-pharmtox-010716-104558
Nooranian, S., Mohammadinejad, A., Mohajeri, T., Aleyaghoob, G., and Oskuee, R. K. (2021). Biosensors based on aptamer-conjugated gold nanoparticles: A review. Biotechnol. Appl. Biochem., 1–18. doi:10.1002/bab.2224
Pan, Q., Nie, C., Hu, Y., Yi, J., Liu, C., Zhang, J., et al. (2020). Aptamer-functionalized DNA origami for targeted codelivery of antisense oligonucleotides and doxorubicin to enhance therapy in drug-resistant cancer cells. ACS Appl. Mat. Interfaces 12, 400–409. doi:10.1021/acsami.9b20707
Pratico, E. D., Sullenger, B. A., and Nair, S. K. (2013). Identification and characterization of an agonistic aptamer against the T cell costimulatory receptor, OX40. Nucleic Acid. Ther. 23, 35–43. doi:10.1089/nat.2012.0388
Prodeus, A., Abdul-Wahid, A., Fischer, N. W., Huang, E. H., Cydzik, M., Gariepy, J., et al. (2015). Targeting the PD-1/PD-L1 immune evasion Axis with DNA aptamers as a novel therapeutic strategy for the treatment of disseminated cancers. Mol. Ther. - Nucleic Acids 4, e237. doi:10.1038/mtna.2015.11
Seeman, N. C., and Sleiman, H. F. (2018). DNA nanotechnology. Nat. Rev. Mat. 3, 17068. doi:10.1038/natrevmats.2017.68
Shangguan, D., Cao, Z., Meng, L., Mallikaratchy, P., Sefah, K., Wang, H., et al. (2008). Cell-specific aptamer probes for membrane protein elucidation in cancer cells. J. Proteome Res. 7, 2133–2139. doi:10.1021/pr700894d
Soldevilla, M. M., de Caso, D. M. C., Menon, A. P., and Pastor, F. (2018). Aptamer-iRNAs as therapeutics for cancer treatment. Pharmaceuticals 11, 108. doi:10.3390/ph11040108
Soldevilla, M. M., Hervas, S., Villanueva, H., Lozano, T., Rabal, O., Oyarzabal, J., et al. (2017). Identification of LAG3 high affinity aptamers by HT-SELEX and conserved motif accumulation (CMA). PLoS One 12, e0185169. doi:10.1371/journal.pone.0185169
Soldevilla, M. M., Villanueva, H., Bendandi, M., Inoges, S., Lopez-Diaz de Cerio, A., and Pastor, F. (2015). 2-fluoro-RNA oligonucleotide CD40 targeted aptamers for the control of B lymphoma and bone-marrow aplasia. Biomaterials 67, 274–285. doi:10.1016/j.biomaterials.2015.07.020
Song, X. R., Liu, C., Wang, N., Huang, H., He, S. Y., Gong, C. Y., et al. (2021). Delivery of CRISPR/cas systems for cancer gene therapy and immunotherapy. Adv. Drug Deliv. Rev. 168, 158–180. doi:10.1016/j.addr.2020.04.010
Song, Y., Zhu, Z., An, Y., Zhang, W., Zhang, H., Liu, D., et al. (2013). Selection of DNA aptamers against epithelial cell adhesion molecule for cancer cell imaging and circulating tumor cell capture. Anal. Chem. 85, 4141–4149. doi:10.1021/ac400366b
Soundararajan, S., Chen, W., Spicer, E. K., Courtenay-Luck, N., and Fernandes, D. J. (2008). The nucleolin targeting aptamer AS1411 destabilizes bcl-2 messenger RNA in human breast cancer cells. Cancer Res. 68, 2358–2365. doi:10.1158/0008-5472.CAN-07-5723
Sun, S., Liu, H., Hu, Y., Wang, Y., Zhao, M., Yuan, Y., et al. (2022). Selection and identification of a novel ssDNA aptamer targeting human skeletal muscle. Bioact. Mat. 20, 166–178. doi:10.1016/j.bioactmat.2022.05.016
Tan, W., Donovan, M. J., and Jiang, J. (2013). Aptamers from cell-based selection for bioanalytical applications. Chem. Rev. 113, 2842–2862. doi:10.1021/cr300468w
Thiel, K. W., Hernandez, L. I., Dassie, J. P., Thiel, W. H., Liu, X., Stockdale, K. R., et al. (2012). Delivery of chemo-sensitizing siRNAs to her2+-breast cancer cells using RNA aptamers. Nucleic Acids Res. 40, 6319–6337. doi:10.1093/nar/gks294
Ucuzian, A. A., Gassman, A. A., East, A. T., and Greisler, H. P. (2010). Molecular mediators of angiogenesis. J. Burn Care Res. 31, 158–175. doi:10.1097/bcr.0b013e3181c7ed82
Vandghanooni, S., Barar, J., Eskandani, M., and Omidi, Y. (2020). Aptamer-conjugated mesoporous silica nanoparticles for simultaneous imaging and therapy of cancer. TrAC Trends Anal. Chem. 123, 115759. doi:10.1016/j.trac.2019.115759
Varmira, K., Hosseinimehr, S. J., Noaparast, Z., and Abedi, S. M. (2013). A HER2-targeted RNA aptamer molecule labeled with 99mTc for single-photon imaging in malignant tumors. Nucl. Med. Biol. 40, 980–986. doi:10.1016/j.nucmedbio.2013.07.004
Varmira, K., Hosseinimehr, S. J., Noaparast, Z., and Abedi, S. M. (2014). An improved RadioLabelled RNA aptamer molecule for HER2 imaging in cancers. J. Drug Target. 22, 116–122. doi:10.3109/1061186X.2013.839688
Wang, D. L., Song, Y. L., Zhu, Z., Li, X. L., Zou, Y., Yang, H. T., et al. (2014). Selection of DNA aptamers against epidermal growth factor receptor with high affinity and specificity. Biochem. Biophys. Res. Commun. 453, 681–685. doi:10.1016/j.bbrc.2014.09.023
Wang, J., Bing, T., Zhang, N., Sheng, L., He, J., Liu, X., et al. (2019). The mechanism of the selective anti-proliferation effect of guanine-based biomolecules and its compensation. ACS Chem. Biol. 14, 1164–1173. doi:10.1021/acschembio.9b00062
Wang, R., Zhu, G., Mei, L., Xie, Y., Ma, H., Ye, M., et al. (2014). Automated modular synthesis of Aptamer−Drug conjugates for targeted drug delivery. J. Am. Chem. Soc. 136, 2731–2734. doi:10.1021/ja4117395
Wang, X., Han, Q., Yu, N., Li, J., Yang, L., Yang, R., et al. (2015). Aptamer-conjugated graphene oxide-gold nanocomposites for targeted chemo-photothermal therapy of cancer cells. J. Mat. Chem. B 3, 4036–4042. doi:10.1039/c5tb00134j
Weng, W., He, S., Song, H., Li, X., Cao, L., Hu, Y., et al. (2018). Aligned carbon nanotubes reduce hypertrophic scar via regulating cell behavior. ACS Nano 12, 7601–7612. doi:10.1021/acsnano.7b07439
Wengerter, B. C., Katakowski, J. A., Rosenberg, J. M., Park, C. G., Almo, S. C., Palliser, D., et al. (2014). Aptamer-targeted antigen delivery. Mol. Ther. 22, 1375–1387. doi:10.1038/mt.2014.51
Wu, Y.-X., Zhang, D., Hu, X., Peng, R., Li, J., Zhang, X., et al. (2021). Multicolor two-photon nanosystem for multiplexed intracellular imaging and targeted cancer therapy. Angew. Chem. Int. Ed. 60, 12569–12576. doi:10.1002/anie.202103027
Wu, Y., Sefah, K., Liu, H., Wang, R., and Tan, W. (2010). DNA Aptamer−Micelle as an efficient detection/delivery vehicle toward cancer cells. Proc. Natl. Acad. Sci. U. S. A. 107, 5–10. doi:10.1073/pnas.0909611107
Xiang, D., Shigdar, S., Qiao, G., Wang, T., Kouzani, A. Z., Zhou, S. F., et al. (2015). Nucleic acid aptamer-guided cancer therapeutics and diagnostics: The next generation of cancer medicine. Theranostics 5, 23–42. doi:10.7150/thno.10202
Xuan, W., Peng, Y., Deng, Z., Peng, T., Kuai, H., Li, Y., et al. (2018). A basic insight into aptamer-drug conjugates (ApDCs). Biomaterials 182, 216–226. doi:10.1016/j.biomaterials.2018.08.021
Xuan, W., Xia, Y., Li, T., Wang, L., Liu, Y., Tan, W., et al. (2020). Molecular self-assembly of bioorthogonal aptamer-prodrug conjugate micelles for hydrogen peroxide and pH-independent cancer chemodynamic therapy. J. Am. Chem. Soc. 142, 937–944. doi:10.1021/jacs.9b10755
Yang, C., Zhao, H., Sun, Y., Wang, C., Geng, X., Wang, R., et al. (2022). Programmable manipulation of oligonucleotide–albumin interaction for elongated circulation time. Nucleic Acids Res. 50, 3083–3095. doi:10.1093/nar/gkac156
Yang, H., Liu, H., Kang, H., and Tan, W. (2008). Engineering target-responsive hydrogels based on aptamer-target interactions. J. Am. Chem. Soc. 130, 6320–6321. doi:10.1021/ja801339w
Yang, L., Tseng, Y.-T., Suo, G., Chen, L., Yu, J., and Chiu, W.-J. (2015). Photothermal therapeutic response of cancer cells to aptamer-gold nanoparticle-hybridized graphene oxide under NIR illumination. ACS Appl. Mat. Interfaces 7, 5097–5106. doi:10.1021/am508117e
Yang, X., Yang, M., Pang, B., Vara, M., and Xia, Y. (2015). Gold nanomaterials at work in biomedicine. Chem. Rev. 115, 10410–10488. doi:10.1021/acs.chemrev.5b00193
Yang, Y., Yang, X., Yang, Y., and Yuan, Q. (2018). Aptamer-functionalized carbon nanomaterials electrochemical sensors for detecting cancer relevant biomolecules. Carbon 129, 380–395. doi:10.1016/j.carbon.2017.12.013
Yao, C., Tang, H., Wu, W., Tang, J., Guo, W., Luo, D., et al. (2020). Double rolling circle amplification generates physically CrossLinked DNA network for stem cell fishing. J. Am. Chem. Soc. 142, 3422–3429. doi:10.1021/jacs.9b11001
Zhang, B., Luo, Z., Liu, J., Ding, X., Li, J., Cai, K., et al. (2014). Cytochrome c end-capped mesoporous silica nanoparticles as redox-responsive drug delivery vehicles for liver tumor-targeted triplex therapy in vitro and in vivo. J. Control. Release 192, 192–201. doi:10.1016/j.jconrel.2014.06.037
Zhang, C., Zhao, Y., Xu, X., Xu, R., Li, H., Teng, X., et al. (2020). Cancer diagnosis with DNA molecular computation. Nat. Nanotechnol. 15, 709–715. doi:10.1038/s41565-020-0699-0
Zhang, N., Bing, T., Liu, X., Qi, C., Shen, L., Wang, L., et al. (2015). Cytotoxicity of guanine-based degradation products contributes to the antiproliferative activity of guanine-rich oligonucleotides. Chem. Sci. 6, 3831–3838. doi:10.1039/C4SC03949A
Zhang, P., Cheng, F., Zhou, R., Cao, J., Li, J., Burda, C., et al. (2014). DNA-Hybrid-gated multifunctional mesoporous silica nanocarriers for dual-targeted and microRNA-responsive controlled drug delivery. Angew. Chem. Int. Ed. 53, 2371–2375. doi:10.1002/anie.201308920
Zhang, P., Zhao, N., Zeng, Z., Feng, Y., Tung, C. H., Chang, C. C., et al. (2009). Using an RNA aptamer probe for flow cytometry detection of CD30-expressing lymphoma cells. Lab. Invest. 89, 1423–1432. doi:10.1038/labinvest.2009.113
Zhao, Y., Xu, J., Le, V. M., Gong, Q., Li, S., Gao, F., et al. (2019). EpCAM aptamer-functionalized cationic LiposomeBased nanoparticles loaded with miR-139−5p for targeted therapy in colorectal cancer. Mol. Pharm. 16, 4696–4710. doi:10.1021/acs.molpharmaceut.9b00867
Zhou, J., and Rossi, J. (2017). Aptamers as targeted therapeutics: Current potential and challenges. Nat. Rev. Drug Discov. 16, 181–202. doi:10.1038/nrd.2016.199
Zhu, G., Zhang, H., Jacobson, Q., Wang, Z., Chen, H., Yang, X., et al. (2017). Combinatorial screening of DNA aptamers for molecular imaging of HER2 in cancer. Bioconjug. Chem. 28, 1068–1075. doi:10.1021/acs.bioconjchem.6b00746
Keywords: aptamers, targeted drug delivery, cancer therapy, aptamer-drug conjugates (ApDCs), aptamer-based nanomaterial system
Citation: Gao F, Yin J, Chen Y, Guo C, Hu H and Su J (2022) Recent advances in aptamer-based targeted drug delivery systems for cancer therapy. Front. Bioeng. Biotechnol. 10:972933. doi: 10.3389/fbioe.2022.972933
Received: 19 June 2022; Accepted: 12 July 2022;
Published: 16 August 2022.
Edited by:
Ruowen Wang, Shanghai Jiao Tong University, ChinaReviewed by:
Da Han, Institute of Cancer and Basic Medicine (CAS), ChinaCopyright © 2022 Gao, Yin, Chen, Guo, Hu and Su. This is an open-access article distributed under the terms of the Creative Commons Attribution License (CC BY). The use, distribution or reproduction in other forums is permitted, provided the original author(s) and the copyright owner(s) are credited and that the original publication in this journal is cited, in accordance with accepted academic practice. No use, distribution or reproduction is permitted which does not comply with these terms.
*Correspondence: Jiacan Su, ZHJzdWppYWNhbkAxNjMuY29t; Honggang Hu, SGh1NjZAc2h1LmVkdS5jbg==
†These authors have contributed equally to this work and share first authorship