- 1Department of Biomolecular Sciences, University of Urbino Carlo Bo, Urbino, Italy
- 2Department of Orthopedic Surgery, School of Medicine, Stanford University, Stanford, CA, United States
- 3Department of Molecular Medicine, University of Pavia, Pavia, Italy
- 4Department of Biomedical Engineering, Tufts University, Medford, MA, United States
Hematopoietic stem cells (HSCs) reside in a subzone of the bone marrow (BM) defined as the hematopoietic niche where, via the interplay of differentiation and self-renewal, they can give rise to immune and blood cells. Artificial hematopoietic niches were firstly developed in 2D in vitro cultures but the limited expansion potential and stemness maintenance induced the optimization of these systems to avoid the total loss of the natural tissue complexity. The next steps were adopted by engineering different materials such as hydrogels, fibrous structures with natural or synthetic polymers, ceramics, etc. to produce a 3D substrate better resembling that of BM. Cytokines, soluble factors, adhesion molecules, extracellular matrix (ECM) components, and the secretome of other niche-resident cells play a fundamental role in controlling and regulating HSC commitment. To provide biochemical cues, co-cultures, and feeder-layers, as well as natural or synthetic molecules were utilized. This review gathers key elements employed for the functionalization of a 3D scaffold that demonstrated to promote HSC growth and differentiation ranging from 1) biophysical cues, i.e., material, topography, stiffness, oxygen tension, and fluid shear stress to 2) biochemical hints favored by the presence of ECM elements, feeder cell layers, and redox scavengers. Particular focus is given to the 3D systems to recreate megakaryocyte products, to be applied for blood cell production, whereas HSC clinical application in such 3D constructs was limited so far to BM diseases testing.
Introduction
Bone marrow (BM) is a complex microenvironment where several cellular elements co-habit and interconnect with each other and the extracellular matrix (ECM) for the maintenance of stem cells (Morrison and Scadden, 2014). Within the BM, the hematopoietic niche regulates the generation of blood and immune cells balancing between hematopoietic stem cell (HSC) quiescence, differentiation (hematopoiesis), and self-renewal. Oxygen tension (pO2) is not uniform throughout the BM, varying from oxygenated areas close to the sub-bone endosteal regions to more hypoxic zones towards the sinusoids, where HSC quiescence and survival are sustained (Wielockx et al., 2019; Congrains et al., 2021).
As illustrated in Figure 1, the niche hosts a variety of cells, which are not only HSC-derived cells (megakaryocytes, macrophages, regulatory T-cells) but also non-hematopoietic ones (as mesenchymal stromal cells, endothelial cells, osteoblasts, adipocytes, Schwann cells) (Pinho and Frenette, 2019; Dolgalev and Tikhonova, 2021), making the niche challenging to faithfully be reproduced it in vitro. Although HSCs constitute less than 0.01% of the total BM population, their multipotent role in hematopoiesis marks them as the cornerstone of the niche (Huang et al., 2007). Fine-tuning of cell-intrinsic factors such as transcriptional (e.g., GATA1 and PU.1) and chromatin regulators keep HSCs in a quiescent state in combination with extrinsic signals from the niche, e.g., hypoxia and growth factors (Rodrigues et al., 2021; Mann et al., 2022). Disruption of homeostasis due to danger-associated molecular patterns (DAMPs), proteolytic and lipolytic enzymes, inflammatory cytokines, and chemokines, i.e. IFNs, G-CSF, IL-1 ROS, suppresses quiescence and enforces HSCs proliferation (Pietras, 2017; Ratajczak et al., 2018).
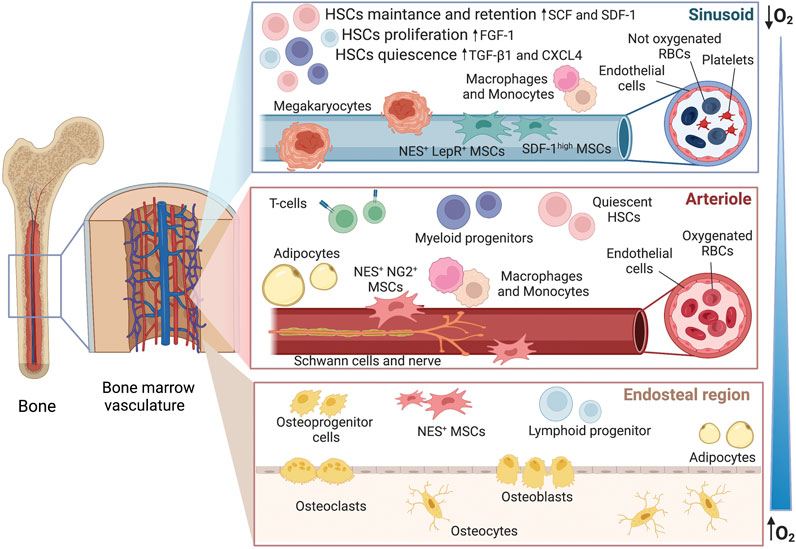
FIGURE 1. Hematopoietic niche within the bone marrow. The image offers an overview of the sub-regions with the gradient of oxygen, from hypoxic sinusoids to more oxygenated arterioles and endosteal area. In vivo, these micro-environments are all interconnected and the fate of HSCs is influenced by the secretome of the neighboring cells. As an example, megakaryocytes can release CXCR4 and TGF-β1 inducing quiescence, factors such as SDF-1 and SCF are released by the Nestin+ and SDFhigh perivascular cells leading to cell retention and self-renewal whereas FGF-1 from macrophages is conducting to proliferation. However, the action of multiple co-factors needs to be considered. CXCR4 platelet factor 4, FGF-1 fibroblast growth factor 1, SCF stem cell factor, SDF stromal cell-derived factor 1, TGF-β1 tumor growth factor-β1, NES nestin, NG2 nerve-glial antigen 2, LepR leptin receptor.
Changes in regulatory mechanisms within the niche have a prominent role in the development of hematopoietic diseases. Aging of the BM micro-environment also favors disease progression. Age-related alterations involve the increased frequency of myeloid cells parallel to a decline of lymphopoiesis, red cell abnormalities like anemia, and a higher incidence of myeloid disorders, like myeloid dysplastic syndrome and acute myeloid leukemia (Pang et al., 2011; Skulimowska et al., 2021).
Currently, the only treatment for hematologic disorders is allogenic HSC transplantation. However, the limited number of available cells isolated from peripheral blood, umbilical cord, or BM is the major obstacle to their application (Zhou et al., 2020). Therefore, HSC proliferation, blood component production, and possible drug testing/toxicology are highly desirable in an in vitro system.
The challenge for the researchers is to create a bioengineered niche able to provide the specific cues needed for HSC expansion while preserving their stem cell properties and differentiation commitment into all the blood cell lineages (Rödling et al., 2017) considering the cellular heterogenicity and the sub-compartments found in vivo (Bessy et al., 2021).
Hence, 3D cultures systems offer a better opportunity for such multi-cellular studies where a three-dimensional scaffold can provide biocompatibility (mimicking the extracellular matrix ECM), bioactivity (stimulating certain cytokines or specific gene expression), and biomechanical stiffness (comparable to the natural tissue for cell mechano-sensitivity) (Bruschi et al., 2015; Szcześ et al., 2017). Several materials have been tested; however, to achieve the required stimuli, functionalization of such scaffolds is necessary. In this review, we highlight the material properties, the modification needed to host HSCs, and further cell conditioning. A summary of all the factors involved in the modeling of a 3D niche is illustrated in Figure 2. Moreover, scaffolds for platelet generation from HSC-derived megakaryocytes will be described separately followed by the clinical outcomes of such 3D bioengineered constructs.
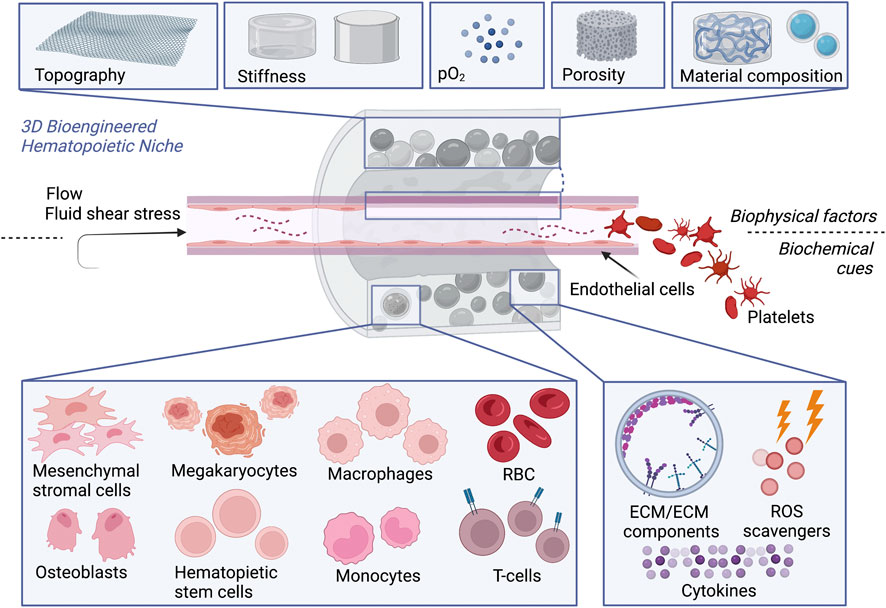
FIGURE 2. 3D bioengineered hematopoietic niche in vitro. To promote HSCs expansion and differentiation or improve platelet production by megakaryocytes, scaffolds recreating the hematopoietic niche should offer simultaneously: 1) Physical cues, given by the composition of the biomaterial, topography, stiffness, oxygen tension, porosity, fluid shear stress. 2) Biochemical cues, provided either by the presence of cell monoculture or multi-culture or cellular components as ECM or ECM components (e.g., RGD peptides) to facilitate the homing of HSCs and in perfusion devices, by blood flow where RBC can be enrolled as carries for oxygen or bioactive molecules.
Biophysical factors
The 3D architecture can be achieved via hydrogels where cells can be encapsulated, by fibrous scaffold, 3D printing, or by porous material (Lee-Thedieck and Spatz, 2012; Gaharwar et al., 2020). To mimic the natural niche, those scaffolds can be tuned with respect to: 1) topography; 2) stiffness 3) pO2 and 4) fluid shear stress (FSS).
The topography provides regulatory signals toward different cellular outcomes and the BM is influenced by the ECM (Jiang and Papoutsakis, 2013; Liu et al., 2022). The secreted ECM is mainly composed of structural proteins such as collagen I and IV, glycoproteins like fibronectin and laminin, and glycosaminoglycans (GAGs) (Matteini et al., 2021). The matrix organization varies within the niche showing a higher concentration of fibronectin and collagen type IV in the endosteal zone and a higher presence of laminin and collagen I in the perivascular region (Choi et al., 2015). ECM mimicry can be also achieved using electrospun nanofibers of nanometer size, porosity, and structure (Mahdavi and Enderami, 2022). Aside from the topography, HSC morphology is influenced by the matrix elasticity, consequentially their differentiation is strongly linked to the matrix stiffness (Zhang P. et al., 2019).
Stiffness is heterogeneous within the BM having ∼40 kPa in the endosteum closer to the bone cells, 10 kPa in marrow sinusoids, and 0.3 kPa in the central marrow (Janagama and Hui, 2020). The distribution of ECM components dictates the heterogeneity in BM stiffness. For instance, collagen type I increases the stiffness proportionally whereas collagen type III and type IV decrease it (Leiva et al., 2018).
In vitro, fibronectin-coated substrates (44 kPa) maintained the HSC myeloid progenitors and those laminin-coated (3.7 kPa) promoted differentiation towards erythroid lineages (Choi and Harley, 2017). Macrophage polarization is also affected by substrate stiffness, i.e. softer surfaces favored the shift to activated macrophages M1 whereas hard surfaces to macrophages M2 (Chen et al., 2020). Similarly, megakaryocytes cultured on soft gels (300 Pa) had higher ploidy, and greater pro-platelet formation than those cultured on stiffer gels (34 kPa) (Malara et al., 2011; Abbonante et al., 2017).
The interplay between stiffness and porous topography is linked.
Porosity (100–800 μm in diameter) and interconnectivity allow cell migration, distribution, and vascularization together with nutrient and oxygen supply within the material (Deschamps et al., 2017; Sari et al., 2021), a higher porosity increases the surface area favoring the absorption of growth factors. Zhou and co-workers designed a millimeter-scale porous scaffold for the encapsulation of feeder cells and a micron-scale for hosting HSC (Zhou et al., 2020).
Nevertheless, porosity affects the oxygen tension (pO2) within the scaffold, and HSC maintenance is increased in hypoxic conditions in vitro. In BM, the vascular zone has O2 tension of ∼12% but in the sinusoidal cavity O2 availability drops to ∼1–6% (Sayin et al., 2021).
Oxygenating biomaterials or carriers can be used to control oxygen levels and serve as 3D hypoxic environments (Fathollahipour et al., 2018). Park and Gerecht developed a hypoxia-inducible hydrogel absorbing oxygen via laccase, a multicopper oxidase that allowed pO2 levels and gradient to be tuned and predicted by reducing O2 to water molecules while concurrently oxidizing ferulic acid of the gelatin-ferulic acid-based hydrogel (Park and Gerecht, 2014). Furthermore, Zambuto and colleagues as well employed laccase to create a controllable hypoxic hydrogel (Zambuto et al., 2020). Other attempts to modulate the oxygen microenvironment involved polymeric carries with encapsulated hemoglobin or myoglobin as substitutes for RBCs (Willemen et al., 2021) However, materials with controlled hypoxia are still object of on-going investigations.
Blood perfusion can influence the activity of the cells in the niche despite not all of the cells are directly in contact with the blood flow (in sinusoids 0.2–0.8 mm/s) (Bixel et al., 2017). Fluid shear stress is absent in static culture systems despite this parameter can affect the hematopoietic niche through paracrine signaling stimulation, e.g. through Yap-1, Piezo 1 (Heck et al., 2020; Zhou et al., 2020; Li et al., 2021). Endothelial cells can convert FSS into a biochemical response for the neighboring cells, regulating cycling and quiescence of HSCs (Roux et al., 2020). FSS is necessary for megakaryocyte differentiation and maturation (Luff and Papoutsakis, 2016) as well as for the quiescence, maintenance, and apoptosis suppression of BM stromal cells (Zhang P. et al., 2019). In fact, the absence of blood circulation led to severe defects in hematopoiesis in both zebrafish and mouse embryos (Adamo et al., 2009; North et al., 2009).
Biochemical stimuli
In the BM niche, HSCs interact with MSCs, which help maintain the HSC phenotype by the secretion of cytokines such as SCF, Flt3L, IL-6, and CSF-1 (Zhang et al., 2004). Nestin+ NG2+ MSCs are located in the periarterial niche, while Nestin+ LEPR+ and SDF-1high MSCs are observed in the perisinusoidal niche (Kunisaki et al., 2013; Kandarakov et al., 2022). Therefore, typically, 3D HSC culture would contain feeder cells such as mesenchymal stromal cells (MSCs) to facilitate the creation of a conducive microenvironment for the growth and sustenance of HSCs (Cook et al., 2012; Ferreira et al., 2018). While MSCs are predominantly used as feeder cells in the 3D culture of HSCs, other stromal cell lines such as umbilical cord endothelial cells and Wharton’s jelly cells are also used (Magin et al., 2009). Thus, 3D HSC cultures make use of primary stromal cells as a feeder for the long-term culture and expansion of HSCs. Braham et al. compared the efficacy of bioactive Matrigel and bioinert alginate hydrogels with the support of different feeder cells for the expansion of cord HSC progenitors (HSPCs) (Braham et al., 2019). The authors demonstrated that a combination of feeder cells consisting of MSCs differentiated toward adipocytes and osteoblasts along with endothelial progenitor cells (EPCs) self-assemble into a hypoxic stromal network, and therefore, better support HSPCs in the bioactive hydrogel.
As previously mentioned, in the HSC niche there are also ECM proteins, such as laminins, collagens, proteoglycans, and fibronectin which provide extrinsic cues that modulate HSC proliferation, differentiation, and migration, partly by providing anchorage for growth factors and cytokines such as tumor growth factor -β, interleukins, and other proteins. Thus, recreating the HSC niche ex vivo requires not only biophysical attributes but also biochemical functionalization to resemble the native archetype.
Different from utilizing feeder cells, 3D biomaterials functionalized by incorporation of ECM molecules or cell adhesion motifs are shown to recapitulate the HSC niche. Polycaprolactone-based 3D porous scaffolds coated with fibronectin (Mousavi et al., 2018) and vitronectin (Kim et al., 2019) (Kim et al., 2019) were shown to support and maintain the HSC phenotype. Incorporation of cell adhesion ligands derived from fibronectin instead of coating the entire fibronectin protein was shown to modulate HSC functions as well (Muth et al., 2013). Fibronectin has multiple binding sides or domains in its polypeptide chain for the adhesion of different growth factors (Rahman et al., 2005; Wijelath et al., 2006). Indeed, Cuchiara et al demonstrated that functionalization of PEG hydrogels with fibronectin-derived adhesive arginine-glycine-aspartic acid-serine (RGDS), showed superior adhesion of HSCs (Cuchiara et al., 2013). Retention signals involving the interaction between CXCR4 and VLA-4 receptors on the HSC surface with perivascular stromal cells expressing SDF-1 (also known as CXCL12), and vascular cell adhesion molecule 1 (VCAM-1), retain HSCs within the BM avoiding mobilization into the peripheral blood (Ratajczak et al., 2018). Méndez-Ferrer and colleagues demonstrated that the expression of SDF-1a is also regulated through circadian noradrenaline secreted by the sympathetic nervous system (SNS) which innervates the BM. The adrenergic signal is locally delivered by nerves and transmitted to Nestin+ MSCs by the b3-adrenergic receptor, leading to the rapid downregulation of SDF-1a and HSCs egress from BM (Méndez-Ferrer et al., 2008; Maestroni, 2020). Furthermore, the incorporation of stem-cell factor (SCF) and stromal-derived factor 1α (SDF1α) into the RGDS-PEG hydrogel further enhanced the adhesion and spreading of HSCs. SCF is known to regulate the HSC niche by promoting the survival and expansion of HSCs (Doran et al., 2009). Interestingly, Mahadik et al. also incorporated SCF in methacrylamide-functionalized gelatin (GelMA) hydrogels and demonstrated that SCF immobilization on the hydrogel improved selectivity of murine HSCs whereas soluble SCF promoted differentiation of HSCs in GelMA hydrogels (Mahadik et al., 2015).
Zhang et al. described an antioxidant hydrogel to circumvent the high levels of oxidative stress generated in ex vivo 3D cultures and mimic the low reactive oxygen species (ROS) levels in the HSC niche in vivo (Zhang Y. et al., 2019). They combined carbon nanotubes (CNTs), which can scavenge free radicals, with methacrylated hyaluronic acid to form hydrogels that can scavenge the culture system for oxides and peroxides generated during the culture of HSCs. In this system with low ROS levels, the proliferation ability and pluripotency of HSCs were improved as confirmed also by other groups (Cacialli et al., 2021). Thus, the biochemical composition of the HSC niche can be a blueprint for designing novel functionalization strategies of 3D hydrogels for recreating the HSC niche in vitro.
Platelet generation
Investigations, about scaffold materials and the necessary stimuli, were performed for decades to develop efficient strategies to face the major problems related to BM engineered niches considering HSC scarce proliferation and the scale-up of blood components generation. Among the blood components, platelets are the most required because of their short lifespan which is further exacerbated by the inability to store them. Despite recent findings attributing an important contribution to platelets in inflammation and immune responses (Abbonante et al., 2020), the traditional and consolidated role of these anucleate cells concerns the maintenance of the hemostasis and thrombosis preventing bleeding by adhesion to injured vessels and thrombus formation (Thomas and Storey, 2015; Holinstat, 2017). In specific pathological or therapeutic conditions in which thrombocytopenia (low platelet count, < 150,000 per microliter of blood) occurs, the only available option to decrease mortality by bleeding is a transfusion. Unfortunately, the high demand of patients together with the platelet shortage, the donor-dependent issues, the cell quantity and functionality as well as the possible microbiological contamination, made urgent the need for platelet production (Abbonante et al., 2017; Vanzolini et al., 2022). HSCs represent suitable starting material since as a natural process, they differentiate directly in megakaryocytes which then mature in platelets. Alternatives consist of the direct differentiation of induced pluripotent stem cells (iPSCs) into megakaryocytes or their progenitors (Hansen et al., 2018; Shepherd et al., 2018; Nakamura et al., 2020) allowing the application of patient-derived cells and possible gene editing to address specific pathologies (Bruschi et al., 2021; Nakamura et al., 2021).
A 3D BM model made of silk fibroin derived from Bombyx mori silkworm cocoons was engineered to recreate the physiology of the human BM niche environment for the successful release of functional platelets into vascular tubes and study of megakaryopoiesis pathologies. Moreover, this silk platform represents a novel screening tool in the pharmaceutical industry to study the therapeutic effects of drugs on platelet number or function (Di Buduo et al., 2015). A significant improvement over the described silk model is a new miniature 3D model of human BM where megakaryocytes isolated from patient blood samples are linked to a scaffold made of silk and release platelets into an artificial bloodstream. This system proved useful to recapitulate ex vivo platelet biogenesis of patients and predict the platelet response to drugs, suggesting applicability for testing new compounds for inherited thrombocytopenia and other blood-related diseases, characterized by low platelet count and impaired hemostasis, for which effective treatment does not yet exist. (Di Buduo et al., 2021).
Current clinical application
A model that successfully mimics the BM microenvironment under homeostasis and activated conditions would have various applications in the clinical and pharmaceutical fields. Research efforts have been addressed to engineer 3D BM models to study HSCs maintenance and hematopoiesis in steady-state and disease; for expansion of HSCs for transplantation, and as a platform for drug screening and toxicity testing in particular for myelotoxic effects of chemotherapeutics (Rödling et al., 2017). Several models have been developed during the last years for different clinical applications, from cancer pharmacotherapy to the production of large quantities of lab-grown blood cells for transfusion. Some examples are highlighted in this chapter.
Nelson and colleagues developed human BM-on-a-chip, incorporating endosteal, central marrow, and perivascular areas using osteogenic differentiated MSCs for mineralization, followed by MSCs and endothelial cells seeding over a fibrin-collagen hydrogel to model a 3D microvascular network favoring maintenance CD34+ HSCs (Nelson et al., 2021). Similarly, Glaser and co-workers utilized a microfluidic device to mimic the perivascular and endosteal niche allowing CD34+ maintenance and hematopoiesis as well. This model provided drug responses comparable to the in vivo ones (Glaser et al., 2022).
Furthermore, the BM niche sheds signals and provides cell-cell interactions supporting multiple myeloma. Myeloma cells alter the BM microenvironment to support tumor proliferation, resistance to therapy, cancer cell trafficking, and homing. Hence, different models are based on the culture of primary myeloma cells in vitro, in a 3D environment mimicking the human BM. The model, in which multipotent MSCs and their osteogenic derivatives were co-cultured with endothelial progenitor cells, facilitated the survival and proliferation of primary CD138+ myeloma cells for a few days (Braham et al., 2018). Furthermore, it was successfully used to test a novel class of engineered immune cells on primary myeloma cells providing a tool to investigate the interactions of primary myeloma cells within the BM niche and novel immunotherapies.
A 3D co-culture model composed of MSCs embedded in a hydrogel system and co-cultured with primary multiple myeloma patient cells has been used to study cellular components in the myeloma niche and the role of the BM microenvironment in the pathogenesis of multiple myeloma and drug resistance. In particular, this model was useful to reveal resistance to novel and conventional agents (Jakubikova et al., 2016).
Leukemic niche models have been engineered to investigate the role of the BM niche in the survival and uncontrolled proliferation of malignant leukemic cells (Raic et al., 2019). An example is given by the 3D PEG-heparin hydrogel with endothelial cells and MSCs developed by Bray and colleagues to investigate cell-cell interactions between vascular niche and leukemic cells and the response of leukemic cells to chemotherapeutics (Bray et al., 2017). These models could favor the transition of new drugs for hematological malignancies from pre-clinical to clinical research, as the lack of a suitable tumor microenvironment and/or BM architecture often impedes the clinical employment of promising drugs (Ramasamy et al., 2012). Moreover, clinical trials often fail due to a lack of drug efficacy, and no correlations between in vitro drug efficacy and clinical outcomes in hematologic malignancies have been described (Alhallak et al., 2021).
A 3D hydrogel system for hematopoietic differentiation of iPSCs has provided a tool for hematological disease modeling of Down syndrome-associated transient myeloproliferative disorder (TMD) which is a pre-leukemic stage present in 10–20% of children with trisomy 21 possessing the mutation in the transcription factor GATA-1 (Grimm et al., 2021). The model has permitted us to conclude that, in GATA1 mutant line, the erythroid population was reduced whereas the megakaryoid and myeloid populations were significantly increased, consistent with TMD characteristics (Sidhu et al., 2021).
In conclusion, 3D BM platforms provide tools for differential applications like studying patients’ cells to investigate disease mechanisms and identify personalized treatments as well as determining precisely the safety and efficacy of new drugs.
Conclusion
Several efforts have been made to understand the intricate mechanisms within the hematopoietic niche and especially those concerning the fine-tuned homeostasis existing among HSC quiescence, self-renewal, and differentiation. Unfortunately, most of the attempts in culturing ex vivo or in reproducing in vitro the hematopoietic niche failed in being efficiently translated to the clinics. The main reason implicates the complexity of the microenvironments (endosteal, central medullary, arteriolar, and perivascular) in which HSCs harbor in the BM. These niches influence HSC behavior and fate thanks to different cues, interactions with other cell populations, and interestingly, tridimensional anatomical architecture. In particular, 3D structures seem to be essential in reproducing a system as close as possible to the original one avoiding polarization or phenotype as well as gene expression modifications, which is why they often replaced 2D cultures as study models (Jensen and Teng, 2020). Nevertheless, 2D systems are easier and more reproducible; moreover, based on the scaffold material, cell collection could be facilitated compared to tridimensional cultures. Hence, they are still preferred for large-scale production and high-throughput screenings (Bello et al., 2018; Varga et al., 2018). Moreover, some of the highly promising techniques are represented by microspheroids, organoids, and decellularized tissues (especially natural scaffolds) since they are the most realistic models and facilitate the engraftment of HSCs in vivo (Yin et al., 2016; Allenby et al., 2018; Harris et al., 2018; Janagama and Hui, 2020; Sahu et al., 2021).
Thus, understanding the behavior of HSCs in culture and their response to the cues of the artificial niche, could help with the success of their expansion ex vivo in 3D scaffolds (Tajer et al., 2019).
Author contributions
MB, TV, NS, and AF carried out the literature study and drafted the manuscript. MM and AB edited and reviewed. All authors have read and agreed to the published version of the manuscript.
Funding
This work was supported by PRIN (Bando 2017; Prot. 2017Z5LR5Z).
Acknowledgments
Figures 1 and 2 were created using BioRender.com.
Conflict of interest
The authors declare that the research was conducted in the absence of any commercial or financial relationships that could be construed as a potential conflict of interest.
Publisher’s note
All claims expressed in this article are solely those of the authors and do not necessarily represent those of their affiliated organizations, or those of the publisher, the editors and the reviewers. Any product that may be evaluated in this article, or claim that may be made by its manufacturer, is not guaranteed or endorsed by the publisher.
Abbreviations
BM bone marrow; CXCR4 platelet factor 4; ECM extracellular matrix; FGF-1 fibroblast growth factor 1; Flt3 FMS-like tyrosine kinase 3; FSS fluid shear stress; HSCs hematopoietic stem cells; IL-6 interleukin 6; iPSCs induced pluripotent stem cells; MSCs mesenchymal stromal cells; PEG polyethylene glycol; RBC red blood cells; RGDS arginine-glycine-aspartic acid-serine; ROS reactive oxygen species; SCF stem-cell factor; SDF1α stromal-derived factor 1α; TGF-β1 tumor growth factor-β1; TMD Down syndrome-associated transient myeloproliferative disorder
References
Abbonante, V., Buduo, C. A. D., Gruppi, C., Maria, C. D., Spedden, E., Acutis, A. D., et al. (2017). A new path to platelet production through matrix sensing. Haematologica 102, 1150–1160. doi:10.3324/haematol.2016.161562
Abbonante, V., Di Buduo, C. A., Malara, A., Laurent, P.-A., and Balduini, A. (2020). Mechanisms of platelet release: In vivo studies and in vitro modeling. Platelets 31, 717–723. doi:10.1080/09537104.2020.1774532
Adamo, L., Naveiras, O., Wenzel, P. L., McKinney-Freeman, S., Mack, P. J., Gracia-Sancho, J., et al. (2009). Biomechanical forces promote embryonic haematopoiesis. Nature 459, 1131–1135. doi:10.1038/nature08073
Alhallak, K., Jeske, A., de la Puente, P., Sun, J., Fiala, M., Azab, F., et al. (2021). A pilot study of 3D tissue-engineered bone marrow culture as a tool to predict patient response to therapy in multiple myeloma. Sci. Rep. 11, 19343. doi:10.1038/s41598-021-98760-9
Allenby, M. C., Tahlawi, A., Morais, J. C. F., Li, K., Panoskaltsis, N., Mantalaris, A., et al. (2018). Ceramic hollow fibre constructs for continuous perfusion and cell harvest from 3D hematopoietic organoids. Stem Cells Int. 2018, 1–14. doi:10.1155/2018/6230214
Bello, A. B., Park, H., and Lee, S.-H. (2018). Current approaches in biomaterial-based hematopoietic stem cell niches. Acta Biomater. 72, 1–15. doi:10.1016/j.actbio.2018.03.028
Bessy, T., Itkin, T., and Passaro, D. (2021). Bioengineering the bone marrow vascular niche. Front. Cell Dev. Biol. 9, 645496. doi:10.3389/fcell.2021.645496
Bixel, M. G., Kusumbe, A. P., Ramasamy, S. K., Sivaraj, K. K., Butz, S., Vestweber, D., et al. (2017). Flow dynamics and HSPC homing in bone marrow microvessels. Cell Rep. 18, 1804–1816. doi:10.1016/j.celrep.2017.01.042
Braham, M. V. J., Li Yim, A. S. P., Garcia Mateos, J., Minnema, M. C., Dhert, W. J. A., Öner, F. C., et al. (2019). A human hematopoietic niche model supporting hematopoietic stem and progenitor cells in vitro. Adv. Healthc. Mat. 8, e1801444. doi:10.1002/adhm.201801444
Braham, M. V. J., Minnema, M. C., Aarts, T., Sebestyen, Z., Straetemans, T., Vyborova, A., et al. (2018). Cellular immunotherapy on primary multiple myeloma expanded in a 3D bone marrow niche model. Oncoimmunology 7, e1434465. doi:10.1080/2162402X.2018.1434465
Bray, L. J., Binner, M., Körner, Y., von Bonin, M., Bornhäuser, M., Werner, C., et al. (2017). A three-dimensional ex vivo tri-culture model mimics cell-cell interactions between acute myeloid leukemia and the vascular niche. Haematologica 102, 1215–1226. doi:10.3324/haematol.2016.157883
Bruschi, M., Sahu, N., Singla, M., Grandi, F., Agarwal, P., Chu, C., et al. (2021). A quick and efficient method for the generation of immunomodulatory mesenchymal stromal cell from human induced pluripotent stem cell. Tissue Eng. Part A 28, 433–446. doi:10.1089/ten.TEA.2021.0172
Bruschi, M., Steinmüller-Nethl, D., Goriwoda, W., and Rasse, M. (2015). Composition and modifications of dental implant surfaces. J. Oral Implants 2015, 1–14. doi:10.1155/2015/527426
Cacialli, P., Mahony, C. B., Petzold, T., Bordignon, P., Rougemont, A.-L., Bertrand, J. Y., et al. (2021). A connexin/ifi30 pathway bridges HSCs with their niche to dampen oxidative stress. Nat. Commun. 12, 4484. doi:10.1038/s41467-021-24831-0
Chen, M., Zhang, Y., Zhou, P., Liu, X., Zhao, H., Zhou, X., et al. (2020). Substrate stiffness modulates bone marrow-derived macrophage polarization through NF-κB signaling pathway. Bioact. Mat. 5, 880–890. doi:10.1016/j.bioactmat.2020.05.004
Choi, J. S., and Harley, B. A. C. (2017). Marrow-inspired matrix cues rapidly affect early fate decisions of hematopoietic stem and progenitor cells. Sci. Adv. 3, e1600455. doi:10.1126/sciadv.1600455
Choi, J. S., Mahadik, B. P., and Harley, B. A. C. (2015). Engineering the hematopoietic stem cell niche: Frontiers in biomaterial science. Biotechnol. J. 10, 1529–1545. doi:10.1002/biot.201400758
Congrains, A., Bianco, J., Rosa, R. G., Mancuso, R. I., and Saad, S. T. O. (2021). 3D scaffolds to model the hematopoietic stem cell niche: Applications and perspectives. Mater. (Basel) 14, 569. doi:10.3390/ma14030569
Cook, M. M., Futrega, K., Osiecki, M., Kabiri, M., Kul, B., Rice, A., et al. (2012). Micromarrows--three-dimensional coculture of hematopoietic stem cells and mesenchymal stromal cells. Tissue Eng. Part C. Methods 18, 319–328. doi:10.1089/ten.TEC.2011.0159
Cuchiara, M. L., Horter, K. L., Banda, O. A., and West, J. L. (2013). Covalent immobilization of stem cell factor and stromal derived factor 1α for in vitro culture of hematopoietic progenitor cells. Acta Biomater. 9, 9258–9269. doi:10.1016/j.actbio.2013.08.012
Deschamps, I. S., Magrin, G. L., Magini, R. S., Fredel, M. C., Benfatti, C. A. M., Souza, M. J. C., et al. (2017). On the synthesis and characterization of β-tricalcium phosphate scaffolds coated with collagen or poly (D, L-lactic acid) for alveolar bone augmentation. Eur. J. Dent. 11, 496–502. doi:10.4103/ejd.ejd_4_17
Di Buduo, C. A., Laurent, P.-A., Zaninetti, C., Lordier, L., Soprano, P. M., Ntai, A., et al. (2021). Miniaturized 3D bone marrow tissue model to assess response to Thrombopoietin-receptor agonists in patients. Elife 10, e58775. doi:10.7554/eLife.58775
Di Buduo, C. A., Wray, L. S., Tozzi, L., Malara, A., Chen, Y., Ghezzi, C. E., et al. (2015). Programmable 3D silk bone marrow niche for platelet generation ex vivo and modeling of megakaryopoiesis pathologies. Blood 125, 2254–2264. doi:10.1182/blood-2014-08-595561
Dolgalev, I., and Tikhonova, A. N. (2021). Connecting the dots: Resolving the bone marrow niche heterogeneity. Front. Cell Dev. Biol. 9, 622519. doi:10.3389/fcell.2021.622519
Doran, M. R., Markway, B. D., Aird, I. A., Rowlands, A. S., George, P. A., Nielsen, L. K., et al. (2009). Surface-bound stem cell factor and the promotion of hematopoietic cell expansion. Biomaterials 30, 4047–4052. doi:10.1016/j.biomaterials.2009.04.043
Fathollahipour, S., Patil, P. S., and Leipzig, N. D. (2018). Oxygen regulation in development: Lessons from embryogenesis towards tissue engineering. Cells Tissues Organs 205, 350–371. doi:10.1159/000493162
Ferreira, J. R., Teixeira, G. Q., Santos, S. G., Barbosa, M. A., Almeida-Porada, G., Gonçalves, R. M., et al. (2018). Mesenchymal stromal cell secretome: Influencing therapeutic potential by cellular pre-conditioning. Front. Immunol. 9, 2837. doi:10.3389/fimmu.2018.02837
Gaharwar, A. K., Singh, I., and Khademhosseini, A. (2020). Engineered biomaterials for in situ tissue regeneration. Nat. Rev. Mat. 5, 686–705. doi:10.1038/s41578-020-0209-x
Glaser, D. E., Curtis, M. B., Sariano, P. A., Rollins, Z. A., Shergill, B. S., Anand, A., et al. (2022). Organ-on-a-chip model of vascularized human bone marrow niches. Biomaterials 280, 121245. doi:10.1016/j.biomaterials.2021.121245
Grimm, J., Heckl, D., and Klusmann, J.-H. (2021). Molecular mechanisms of the genetic predisposition to acute megakaryoblastic leukemia in infants with down syndrome. Front. Oncol. 11, 636633. doi:10.3389/fonc.2021.636633
Hansen, M., Varga, E., Aarts, C., Wust, T., Kuijpers, T., von Lindern, M., et al. (2018). Efficient production of erythroid, megakaryocytic and myeloid cells, using single cell-derived iPSC colony differentiation. Stem Cell Res. 29, 232–244. doi:10.1016/j.scr.2018.04.016
Harris, G. M., Raitman, I., and Schwarzbauer, J. E. (2018). Cell-derived decellularized extracellular matrices. Methods Cell Biol. 143, 97–114. doi:10.1016/bs.mcb.2017.08.007
Heck, A. M., Ishida, T., and Hadland, B. (2020). Location, location, location: How vascular specialization influences hematopoietic fates during development. Front. Cell Dev. Biol. 8. Available at: https://www.frontiersin.org/article/10.3389/fcell.2020.602617 (Accessed February 18, 2022).
Holinstat, M. (2017). Normal platelet function. Cancer Metastasis Rev. 36, 195–198. doi:10.1007/s10555-017-9677-x
Huang, X., Cho, S., and Spangrude, G. J. (2007). Hematopoietic stem cells: generation and self-renewal. Cell Death Differ 14, 1851–1859. doi:10.1038/sj.cdd.4402225
Jakubikova, J., Cholujova, D., Hideshima, T., Gronesova, P., Soltysova, A., Harada, T., et al. (2016). A novel 3D mesenchymal stem cell model of the multiple myeloma bone marrow niche: Biologic and clinical applications. Oncotarget 7, 77326–77341. doi:10.18632/oncotarget.12643
Janagama, D., and Hui, S. K. (2020). 3-D cell culture systems in bone marrow tissue and organoid engineering, and BM phantoms as in vitro models of hematological cancer therapeutics-A review. Mater. (Basel) 13, E5609. doi:10.3390/ma13245609
Jensen, C., and Teng, Y. (2020). Is it time to start transitioning from 2D to 3D cell culture? Front. Mol. Biosci. 7. Available at: https://www.frontiersin.org/article/10.3389/fmolb.2020.00033 (Accessed March 29, 2022).
Jiang, J., and Papoutsakis, E. T. (2013). Stem-cell niche based comparative analysis of chemical and nano-mechanical material properties impacting ex vivo expansion and differentiation of hematopoietic and mesenchymal stem cells. Adv. Healthc. Mat. 2, 25–42. doi:10.1002/adhm.201200169
Kandarakov, O., Belyavsky, A., and Semenova, E. (2022). Bone marrow niches of hematopoietic stem and progenitor cells. Int. J. Mol. Sci. 23, 4462. doi:10.3390/ijms23084462
Kim, J. E., Lee, E. J., Wu, Y., Kang, Y. G., and Shin, J.-W. (2019). The combined effects of hierarchical scaffolds and mechanical stimuli on ex vivo expansion of haematopoietic stem/progenitor cells. Artif. Cells Nanomed. Biotechnol. 47, 585–592. doi:10.1080/21691401.2019.1573180
Kunisaki, Y., Bruns, I., Scheiermann, C., Ahmed, J., Pinho, S., Zhang, D., et al. (2013). Arteriolar niches maintain haematopoietic stem cell quiescence. Nature 502, 637–643. doi:10.1038/nature12612
Lee-Thedieck, C., and Spatz, J. P. (2012). Artificial niches: Biomimetic materials for hematopoietic stem cell culture. Macromol. Rapid Commun. 33, 1432–1438. doi:10.1002/marc.201200219
Leiva, O., Leon, C., Ng, S. K., Mangin, P., Gachet, C., Ravid, K., et al. (2018). The role of extracellular matrix stiffness in megakaryocyte and platelet development and function. Am. J. Hematol. 93, 430–441. doi:10.1002/ajh.25008
Li, H., Luo, Q., Shan, W., Cai, S., Tie, R., Xu, Y., et al. (2021). Biomechanical cues as master regulators of hematopoietic stem cell fate. Cell. Mol. Life Sci. 78, 5881–5902. doi:10.1007/s00018-021-03882-y
Liu, B., Tao, C., Wu, Z., Yao, H., and Wang, D.-A. (2022). Engineering strategies to achieve efficient in vitro expansion of haematopoietic stem cells: Development and improvement. J. Mat. Chem. B 10, 1734–1753. doi:10.1039/d1tb02706a
Luff, S. A., and Papoutsakis, E. T. (2016). Megakaryocytic maturation in response to shear flow is mediated by the activator protein 1 (AP-1) transcription factor via mitogen-activated protein kinase (MAPK) mechanotransduction. J. Biol. Chem. 291, 7831–7843. doi:10.1074/jbc.M115.707174
Maestroni, G. J. M. (2020). Adrenergic modulation of hematopoiesis. J. Neuroimmune. Pharmacol. 15, 82–92. doi:10.1007/s11481-019-09840-7
Magin, A. S., Körfer, N. R., Partenheimer, H., Lange, C., Zander, A., Noll, T., et al. (2009). Primary cells as feeder cells for coculture expansion of human hematopoietic stem cells from umbilical cord blood--a comparative study. Stem Cells Dev. 18, 173–186. doi:10.1089/scd.2007.0273
Mahadik, B. P., Pedron Haba, S., Skertich, L. J., and Harley, B. A. C. (2015). The use of covalently immobilized stem cell factor to selectively affect hematopoietic stem cell activity within a gelatin hydrogel. Biomaterials 67, 297–307. doi:10.1016/j.biomaterials.2015.07.042
Mahdavi, M. R., and Enderami, S. E. (2022). Electrospun silk nanofibers promoted the in vitro expansion potential of CD 133+ cells derived from umbilical cord blood. Gene 809, 146005. doi:10.1016/j.gene.2021.146005
Malara, A., Gruppi, C., Rebuzzini, P., Visai, L., Perotti, C., Moratti, R., et al. (2011). Megakaryocyte-matrix interaction within bone marrow: New roles for fibronectin and factor XIII-A. Blood 117, 2476–2483. doi:10.1182/blood-2010-06-288795
Mann, Z., Sengar, M., Verma, Y. K., Rajalingam, R., and Raghav, P. K. (2022). Hematopoietic stem cell factors: Their functional role in self-renewal and clinical aspects. Front. Cell Dev. Biol. 10.
Matteini, F., Mulaw, M. A., and Florian, M. C. (2021). Aging of the hematopoietic stem cell niche: New tools to answer an old question. Front. Immunol. 12, 738204. doi:10.3389/fimmu.2021.738204
Méndez-Ferrer, S., Lucas, D., Battista, M., and Frenette, P. S. (2008). Haematopoietic stem cell release is regulated by circadian oscillations. Nature 452, 442–447. doi:10.1038/nature06685
Morrison, S. J., and Scadden, D. T. (2014). The bone marrow niche for haematopoietic stem cells. Nature 505, 327–334. doi:10.1038/nature12984
Mousavi, S. H., Abroun, S., Soleimani, M., and Mowla, S. J. (2018). 3-Dimensional nano-fibre scaffold for ex vivo expansion of cord blood haematopoietic stem cells. Artif. Cells Nanomed. Biotechnol. 46, 740–748. doi:10.1080/21691401.2017.1337026
Muth, C. A., Steinl, C., Klein, G., and Lee-Thedieck, C. (2013). Regulation of hematopoietic stem cell behavior by the nanostructured presentation of extracellular matrix components. PLoS One 8, e54778. doi:10.1371/journal.pone.0054778
Nakamura, S., Sugimoto, N., and Eto, K. (2021). Development of platelet replacement therapy using human induced pluripotent stem cells. Dev. Growth Differ. 63, 178–186. doi:10.1111/dgd.12711
Nakamura, S., Sugimoto, N., and Eto, K. (2020). Ex vivo generation of platelet products from human iPS cells. Inflamm. Regen. 40, 30. doi:10.1186/s41232-020-00139-2
Nelson, M. R., Ghoshal, D., Mejías, J. C., Rubio, D. F., Keith, E., Roy, K., et al. (2021). A multi-niche microvascularized human bone marrow (hBM) on-a-chip elucidates key roles of the endosteal niche in hBM physiology. Biomaterials 270, 120683. doi:10.1016/j.biomaterials.2021.120683
North, T. E., Goessling, W., Peeters, M., Li, P., Ceol, C., Lord, A. M., et al. (2009). Hematopoietic stem cell development is dependent on blood flow. Cell 137, 736–748. doi:10.1016/j.cell.2009.04.023
Pang, W. W., Price, E. A., Sahoo, D., Beerman, I., Maloney, W. J., and Rossi, D. J. (2011). Human bone marrow hematopoietic stem cells are increased in frequency and myeloid-biased with age. Proceedings of the National Academy of Sciences 108, 20012–20017. doi:10.1073/pnas.1116110108
Park, K. M., and Gerecht, S. (2014). Hypoxia-inducible hydrogels. Nat. Commun. 5, 4075. doi:10.1038/ncomms5075
Pietras, E. M. (2017). Inflammation: A key regulator of hematopoietic stem cell fate in health and disease. Blood 130, 1693–1698. doi:10.1182/blood-2017-06-780882
Pinho, S., and Frenette, P. S. (2019). Haematopoietic stem cell activity and interactions with the niche. Nat. Rev. Mol. Cell Biol. 20, 303–320. doi:10.1038/s41580-019-0103-9
Rahman, S., Patel, Y., Murray, J., Patel, K. V., Sumathipala, R., Sobel, M., et al. (2005). Novel hepatocyte growth factor (HGF) binding domains on fibronectin and vitronectin coordinate a distinct and amplified Met-integrin induced signalling pathway in endothelial cells. BMC Cell Biol. 6, 8. doi:10.1186/1471-2121-6-8
Raic, A., Naolou, T., Mohra, A., Chatterjee, C., and Lee-Thedieck, C. (2019). 3D models of the bone marrow in health and disease: Yesterday, today and tomorrow. MRS Commun. 9, 37–52. doi:10.1557/mrc.2018.203
Ramasamy, K., Khatun, H., Macpherson, L., Caley, M. P., Sturge, J., Mufti, G. J., et al. (2012). Fluorescence-based experimental model to evaluate the concomitant effect of drugs on the tumour microenvironment and cancer cells. Br. J. Haematol. 157, 564–579. doi:10.1111/j.1365-2141.2012.09103.x
Ratajczak, M. Z., Adamiak, M., Plonka, M., Abdel-Latif, A., Ratajczak, J., et al. (2018). Mobilization of hematopoietic stem cells as a result of innate immunity-mediated sterile inflammation in the bone marrow microenvironment—the involvement of extracellular nucleotides and purinergic signaling. Leukemia 32, 1116–1123. doi:10.1038/s41375-018-0087-z
Rödling, L., Schwedhelm, I., Kraus, S., Bieback, K., Hansmann, J., Lee-Thedieck, C., et al. (2017). 3D models of the hematopoietic stem cell niche under steady-state and active conditions. Sci. Rep. 7, 4625. doi:10.1038/s41598-017-04808-0
Rodrigues, C. P., Shvedunova, M., and Akhtar, A. (2021). Epigenetic regulators as the gatekeepers of hematopoiesis. Trends Genet. 37, 125–142. doi:10.1016/j.tig.2020.09.015
Roux, E., Bougaran, P., Dufourcq, P., and Couffinhal, T. (2020). Fluid shear stress sensing by the endothelial layer. Front. Physiol. 11, 861. doi:10.3389/fphys.2020.00861
Shepherd, J. H., Howard, D., Waller, A. K., Foster, H. R., Mueller, A., Moreau, T., et al. (2018). Structurally graduated collagen scaffolds applied to the ex vivo generation of platelets from human pluripotent stem cell-derived megakaryocytes: Enhancing production and purity. Biomaterials 182, 135–144. doi:10.1016/j.biomaterials.2018.08.019
Sahu, N., Agarwal, P., Grandi, F., Bruschi, M., Goodman, S., Amanatullah, D., et al. (2021). Encapsulated mesenchymal stromal cell microbeads promote endogenous regeneration of osteoarthritic cartilage ex vivo. Adv. Healthc. Mat. 10, e2002118. doi:10.1002/adhm.202002118
Sari, M., Hening, P., ChotimahAna, I. D., and Yusuf, Y. (2021). Bioceramic hydroxyapatite-based scaffold with a porous structure using honeycomb as a natural polymeric Porogen for bone tissue engineering. Biomater. Res. 25, 2. doi:10.1186/s40824-021-00203-z
Sayin, E., Baran, E. T., Elsheikh, A., Mudera, V., Cheema, U., Hasirci, V., et al. (2021). Evaluating oxygen tensions related to bone marrow and matrix for MSC differentiation in 2D and 3D biomimetic lamellar scaffolds. Int. J. Mol. Sci. 22, 4010. doi:10.3390/ijms22084010
Sidhu, I., Barwe, S. P., Kiick, K. L., Kolb, E. A., and Gopalakrishnapillai, A. (2021). A 3-D hydrogel based system for hematopoietic differentiation and its use in modeling down syndrome associated transient myeloproliferative disorder. Biomater. Sci. 9, 6266–6281. doi:10.1039/d1bm00442e
Skulimowska, I., Sosniak, J., Gonka, M., Szade, A., Jozkowicz, A., and Szade, K. (2021). The biology of hematopoietic stem cells and its clinical implications. FEBS J.. doi:10.1111/febs.16192
Szcześ, A., Hołysz, L., and Chibowski, E. (2017). Synthesis of hydroxyapatite for biomedical applications. Adv. Colloid Interface Sci. 249, 321–330. doi:10.1016/j.cis.2017.04.007
Tajer, P., Pike-Overzet, K., Arias, S., Havenga, M., and Staal, F. J. T. (2019). Ex vivo expansion of hematopoietic stem cells for therapeutic purposes: Lessons from development and the niche. Cells 8, 169. doi:10.3390/cells8020169
Thomas, M. R., and Storey, R. F. (2015). The role of platelets in inflammation. Thromb. Haemost. 114, 449–458. doi:10.1160/TH14-12-1067
Vanzolini, T., Bruschi, M., Rinaldi, A. C., Magnani, M., and Fraternale, A. (2022). Multitalented synthetic antimicrobial peptides and their antibacterial, antifungal and antiviral mechanisms. Int. J. Mol. Sci. 23, 545. doi:10.3390/ijms23010545
Varga, E., Hansen, M., Akker, E. v. d., and von Lindern, M. (2018). Erythropoiesis and megakaryopoiesis in a dish. IntechOpen. doi:10.5772/intechopen.80638
Wielockx, B., Grinenko, T., Mirtschink, P., and Chavakis, T. (2019). Hypoxia pathway proteins in normal and malignant hematopoiesis. Cells 8, E155. doi:10.3390/cells8020155
Wijelath, E. S., Rahman, S., Namekata, M., Murray, J., Nishimura, T., Mostafavi-Pour, Z., et al. (2006). Heparin-II domain of fibronectin is a vascular endothelial growth factor-binding domain: Enhancement of VEGF biological activity by a singular growth factor/matrix protein synergism. Circ. Res. 99, 853–860. doi:10.1161/01.RES.0000246849.17887.66
Willemen, N. G. A., Hassan, S., Gurian, M., Li, J., Allijn, I. E., Shin, S. R., et al. (2021). Oxygen-releasing biomaterials: Current challenges and future applications. Trends Biotechnol. 39, 1144–1159. doi:10.1016/j.tibtech.2021.01.007
Yin, X., Mead, B. E., Safaee, H., Langer, R., Karp, J. M., Levy, O., et al. (2016). Engineering stem cell organoids. Cell Stem Cell 18, 25–38. doi:10.1016/j.stem.2015.12.005
Zambuto, S. G., Serrano, J. F., Vilbert, A. C., Lu, Y., Harley, B. A. C., Pedron, S., et al. (2020). Response of neuroglia to hypoxia-induced oxidative stress using enzymatically crosslinked hydrogels. MRS Commun. 10, 83–90. doi:10.1557/mrc.2019.159
Zhang, P., Zhang, C., Li, J., Han, J., Liu, X., Yang, H., et al. (2019a). The physical microenvironment of hematopoietic stem cells and its emerging roles in engineering applications. Stem Cell Res. Ther. 10, 327. doi:10.1186/s13287-019-1422-7
Zhang, Y., Li, C., Jiang, X., Zhang, S., Wu, Y., Liu, B., et al. (2004). Human placenta-derived mesenchymal progenitor cells support culture expansion of long-term culture-initiating cells from cord blood CD34+ cells. Exp. Hematol. 32, 657–664. doi:10.1016/j.exphem.2004.04.001
Zhang, Y., Tong, Y., Pan, X., Cai, H., Gao, Y., Zhang, W., et al. (2019b). Promoted proliferation of hematopoietic stem cells enabled by a hyaluronic acid/carbon nanotubes antioxidant hydrogel. Macromol. Mat. Eng. 304, 1800630. doi:10.1002/mame.201800630
Keywords: hematopoietic niche, hematopoietic stem cells, megakaryocytes, platelets, 3D scaffold
Citation: Bruschi M, Vanzolini T, Sahu N, Balduini A, Magnani M and Fraternale A (2022) Functionalized 3D scaffolds for engineering the hematopoietic niche. Front. Bioeng. Biotechnol. 10:968086. doi: 10.3389/fbioe.2022.968086
Received: 13 June 2022; Accepted: 13 July 2022;
Published: 17 August 2022.
Edited by:
Antonella Motta, University of Trento, ItalyReviewed by:
Sadie Slater, University of Bristol, United KingdomAndrea Papait, Catholic University of the Sacred Heart, Italy
Copyright © 2022 Bruschi, Vanzolini, Sahu, Balduini, Magnani and Fraternale. This is an open-access article distributed under the terms of the Creative Commons Attribution License (CC BY). The use, distribution or reproduction in other forums is permitted, provided the original author(s) and the copyright owner(s) are credited and that the original publication in this journal is cited, in accordance with accepted academic practice. No use, distribution or reproduction is permitted which does not comply with these terms.
*Correspondence: Michela Bruschi, bWljaGVsYS5icnVzY2hpQHVuaXVyYi5pdA==
†ORCID: Michela Bruschi, orcid.org/0000-0002-0804-7511; Neety Sahu, orcid.org/0000-0001-5355-0514; Alessandra Fraternale, orcid.org/0000-0002-7893-3842
‡These authors have contributed equally to this work