- Department of Anatomy, College of Basic Medicine Sciences, Jilin University, Changchun, China
Nanogels have come out as a great potential drug delivery platform due to its prominently high colloidal stability, high drug loading, core-shell structure, good permeation property and can be responsive to environmental stimuli. Such nanoscopic drug carriers have more excellent abilities over conventional nanomaterials for permeating to brain parenchyma in vitro and in vivo. Nanogel-based system can be nanoengineered to bypass physiological barriers via non-invasive treatment, rendering it a most suitable platform for the management of neurological conditions such as neurodegenerative disorders, brain tumors, epilepsy and ischemic stroke, etc. Therapeutics of central nervous system (CNS) diseases have shown marked limited site-specific delivery of CNS by the poor access of various drugs into the brain, due to the presences of the blood-brain barrier (BBB) and blood-cerebrospinal fluid barrier (BCSFB). Hence, the availability of therapeutics delivery strategies is considered as one of the most major challenges facing the treatment of CNS diseases. The primary objective of this review is to elaborate the newer advances of nanogel for CNS drugs delivery, discuss the early preclinical success in the field of nanogel technology and highlight different insights on its potential neurotoxicity.
Introduction
Neurological diseases and disorders are considered significant challenges to the human health. According to global statistics, more than 1.5 billion people, arguably a quarter of the world’s population suffer from CNS diseases (Palmer, 2010; Srikanth and Kessler, 2012; Soni et al., 2016a). A wide spectrum of therapeutic agents, e.g., nucleic acids, polypeptides, proteins and antisense drugs, have been promoted to alleviate CNS diseases, but the majority of these agents are unable to enter the brain parenchyma noninvasively due to a series of challenges. Site-specific delivery of the drugs is one of the most significant challenges due to restrictions imposed by two biochemical barriers, primarily by BBB. To treat CNS diseases, the most lethal and disabling diseases in the world, researchers have attempted to explore the novel treatment strategies using multiple perspectives ranging from more established efforts, such as the disruption of the BBB, the alteration of BBB permeability, and lipidization of water-soluble drugs, to the newer methods such as the development of nanoenabled drug delivery systems.
Nanotechnology is one of the strategies that provide a greater possibility to meet the requirements associated with high doses of the CNS drugs. Nanocarriers for the management of CNS diseases mainly include polymeric nanoparticles, solid lipid nanoparticles (SLNs), lipid nanocapsules, albumin nanoparticles, liposomes, dendrimers, nanoemulsions, hydrogels and nanogels, etc. (Date et al., 2007; Sun et al., 2012; Cojocaru et al., 2020; Bhia et al., 2021) However, these agents have the following inherent limitations in preclinical applications: 1) liposomes have poor stability and dispersibility; 2) polylactic acid-glycolic acid copolymer NPs but suffer from burst release problems; 3) chitosan nanoparticles have poor dispersibility when used in humans and potential biotoxicity; 5) inorganic-based carriers still cannot achieve biocompatibility; and 6) superparamagnetic iron oxide that contain choline has been mainly been fabricated for in vivo imaging studies. These limitations may render drugs unable to treat CNS diseases with ideal effects. Table 1 summarizes several classes of the drug nanocarriers and their limitations for CNS therapy.
Among these nanotechnologies, the transition from hydrogels to nanogels offers new opportunities to achieve a systemic controlled-release drug delivery platform at the cellular level. Traditional hydrogels exhibit limited BBB penetration ability due to their microstructure, but this obstacle can be overcome by designing nanogels as nanosized colloidal particles (Figure 1). (Chen et al., 2021) Nanogels are the nanoscale hydrogel materials consisting of crosslinked polymer networks, which can be easily synthesized by chemical or physical routes (Figure 2). (Yin et al., 2008; Merino et al., 2015; Neamtu et al., 2017) Such nanocarrier systems are characterized by excellent ability to cross the BBB and accomplish site-specific delivery of drugs due to high water retention capacity of the hydrogels, their tunable shape, amphiphilic behavior, surface modifiability, and especially to their biodegradability and safety. Currently, nanogel technologies are mainly manifested in the fields of disease diagnosis (medical imaging) and drug delivery. The latter application faces more challenges, especially for therapeutic drug delivery for brain diseases, due to complex physiological responses in vivo (Debele et al., 2016; Ma et al., 2017; Neamtu et al., 2017; Ali et al., 2021).
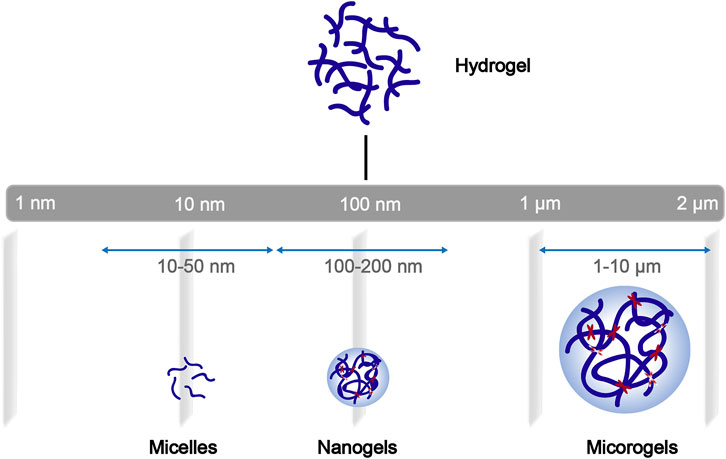
FIGURE 1. General schematization of the network construction of hydrogels and derivatives of different sizes.
The present review provides insights into nanogels as an effective carrier system for CNS delivery in preclinical applications, the strategies to improve the BBB penetration, and the approaches that are close to clinical applications. The present article considers the major challenges for the nanogels, which remain despite certain advances in the design of CNS nanocarrier, to provide ideal plans for the clinical therapies of CNS diseases.
Biological Barriers for CNS Drug Delivery
The Blood-Brain Barrier
CNS homeostasis is strictly protected by two peripheral barriers, termed blood-brain barrier (BBB) and blood-cerebrospinal fluid barrier (BCSFB), which strictly regulate a series of the transport and metabolic processes to protect the brain from the periphery (Erickson and Banks, 2018; Ghersi-Egea et al., 2018; Kadry et al., 2020). These biological barriers, particularly the BBB pose the largest obstacle to nanocarriers for the delivery of various drugs into the CNS. In the absence of effective drug carriers, most macromolecules, such as proteins, oligonucleotides, nucleoside analogs, macromolecular drugs and more than 90% of small-molecule drugs cannot enter the brain through the BBB, which is the key bottleneck in CNS disease treatment (Pardridge, 2005; Wohlfart et al., 2012). These issues are the key bottleneck in the treatment of CNS diseases. Additionally, rapid drug clearance and the failure to achieve a steady release of the drugs in the brain tissue remain among the challenges for the treatment of this group of diseases (Misra et al., 2003; Furtado et al., 2018; Jain, 2020).
The BBB was defined for the first time by Paul Ehrlich in 1885 and is composed of unique CNS microvasculature, including tight junctions (TJs) and adherent junctions, which strictly regulate the metabolism of immune surveillance cells and the entry process of xenobiotics/endogenous materials (Hawkins and Davis, 2005; Engelhardt and Sorokin, 2009; Tietz and Engelhardt, 2015). The functional unit of the BBB is not only constituted by brain capillary endothelial cells (ECs), but also has close interactions with pericytes, perivascular astrocytes and nerve cells. In particular, the tight junction proteins claudins and occludin, which are expressed in brain microvascular cells, account for extremely high transendothelial electrical resistance (TEER, approximately 1,500–2,000 Ω cm2) of the BBB thus limiting the entry of the neurotherapeutic agents (Baeten and Akassoglou, 2011; Sá-Pereira et al., 2012; Molino et al., 2014; Girolamo et al., 2021).
In addition to impermeable cell barrier, there is a selective membrane-bound barrier regulated by ion channels, receptors, and transporters specifically expressed at the BBB. These molecules include ATP-binding cassette (ABC) transporters expressed in brain ECs, such as multiple drug resistance protein 1 (MDR1), permeability glycoprotein (P-gp), multiple resistance-associated protein 4 (MRP4), and breast cancer resistance protein (BCRP); these protein largely limit the permeability of the neurotherapeutic agents (e.g., anticancer drugs or kinins) through the BBB (Ueno et al., 2010; Alyautdin et al., 2014; Aday et al., 2016; Begicevic and Falasca, 2017; Gil-Martins et al., 2020). The transport of the polypeptides and proteins across the BBB requires the assistance of a series of receptor-binding molecules, such as insulin, insulin-like growth factors (IGF-I and IGF-II), angiotensin, and transferrin (Tf), which undergo receptor-mediated endocytosis (Wang et al., 2009; Soni et al., 2010). Another metabolic barrier is driven jointly by the complex and widely expressed influx/efflux transporters in the BBB. It is well known that P-glycoprotein (P-gp) and selective multidrug resistance protein-1 (MRP-1) are expressed at the high levels in the BBB and act as the efflux channels to confine the therapeutic drugs in combination with metabolic enzymes expressed by ECs (Lee et al., 2001; T. Ronaldson and P. Davis, 2012). Thus, the efficiency of the transport of most cargos from the blood circulation into the brain through the BBB is regulated by various transport systems.
Mounting evidence indicates that the permeability of the BBB is pathologically altered in several CNS diseases, such as neurodegenerative diseases, resulting in the aberrant expression of pivotal carrier/receptor-mediated transporters (e.g., P-gp and neuropeptides) and BBB efflux proteins (Zatta et al., 2009; Meairs, 2015; Logan et al., 2019; Bonsack et al., 2020). Therefore, insight into the pathophysiological characteristics of CNS barriers is essential for the development of a safe and efficient carrier system. The regulation of these transporter proteins and CNS barriers may provide new strategies for targeting of the brain by for neuroprotection and therapy.
Blood-Cerebrospinal Fluid Barrier (BCSFB)
The BCSFB is formed by a rich vascular network surrounding choroid plexus epithelial cells, located in the choroid plexus and meninges, which secrete cerebrospinal fluid into the ventricular system. where it is secreted into the ventricular system by choroid plexus epithelial cells. Similar to many other secretory epithelial cells, but unlike the endothelial cells of the brain capillaries that form the BBB, the endothelial cells of the choroid plexus capillaries are fenestrated. The barrier is composed of choroid plexus epithelial cells and their TJs and restricts the movement of small polar molecules. Thus, the BCSFB also regulates the permeability to nutrients or xenobiotics.
Notably, similar to the BBB, the BCSFB not only acts as both a physical barriers and an enzymatic barrier via endothelial or epithelial cells. These cells express not only a series of cytoplasmic and membrane-related enzymes that can effectively metabolize biologically active drugs, but also express many transport proteins and ion channels. These characteristic enzymatic reactions and polarized expression of proteins are clearly of considerable concern for the design of drug carriers targeting the brain.
Strategies Across the Brain Barriers
A variety of strategies have been developed to overcome the challenge of transporting the drugs across the BBB; however, the discoveries of most of strategies have not resulted in significant advances. Table 2 describes the strategies to improve the BBB penetration of active drugs/agents. New advances in nanotechnology have produced various opportunities in the field of CNS disorders because nanocarrier systems have been shown to load poorly distributed drugs in the brain, traverse the cellular/metabolic barrier regions of the BBB, and efficiently deliver the drugs into the brain parenchyma (Poovaiah et al., 2018; Prasanna and Upadhyay, 2021; Wang et al., 2021). For example, Harbi et al. designed sertraline (Ser-HCl)-loaded pegylated and glycosylated liposomes. The results of analysis of the transport in endothelial polyoma cells of the mouse brain showed that glycosylated liposomes have a greater ability to target the cerebellum than PEGylated liposomes (Harbi et al., 2016).
The transport of drug nanocarrier systems across the BBB mainly involves the following mechanisms: 1) receptor-mediated endocytosis; 2) adsorptive-mediated endocytosis; 3) carrier-mediated transport; 4) passive diffusion; 5) efflux pump inhibition; and 6) the transient opening of the TJs of BBB. Surface functionalization of nanocarriers is a potential strategy to facilitate crossing of the BBB, enabling their entry into the brain via a transcellular pathway due to their specific targeting. Thus, the development of optimal drug delivery systems for CNS diseases should consider not only the ability to cross the BBB but also the ability to target and accumulate the drugs. Nanoscale particles can traverse the smallest capillaries, previous studies have demonstrated that after intravenous administration, the particles in the range of 5–10 nm are rapidly removed by the kidney, whereas the particles ranging from 10 to 50 nm are small enough to traverse the capillaries. Additionally, the particles ranging from 50 to 100 nm in size have the longest cycle lifetime, and the particles with a diameter larger than 100 nm are usually blocked by the spleen and removed by phagocytosis, resulting in a short blood circulation time (Vinogradov et al., 2002; De Jong et al., 2008; Kreyling et al., 2014).
Modifying the carrier system with targeting ligands showed better trans-BBB efficiency. The surface of polyamidoamine (PAMAM) dendrimers was conjugated to transferrin (Tf) for improved drug delivery to the brain. The results demonstrated that surface-modified dendrimers show better BBB transport ability in physiological environments.
Emergence of the Nanogel as a Nanocarrier System
Recently developed available drug nanocarriers for the management of CNS diseases, range from more conventional formulations (e.g., liposomes, solid lipid nanoparticles, polymeric nanoparticles) to advanced formulations (e.g., nanocapsules, albumin, dendrimers (Vigani et al., 2020), and nanogels). Among them, nanogels have been shown to achieve a suitable drug pharmacokinetic profile and higher efficacy and safety compared with other drug nanocarriers.
Properties of the Nanogel
Vinogradov et al. initially introduced the term “NanoGel” in 1999 to describe the particles of a hydrophilic polymer network (PEG-PEI) obtained by crosslinking polyethylene glycol (PEG) and polyethyleneimine (PEI) that were able to deliver antisense oligonucleotides (Zhao et al., 2015). These hydrogel nanoparticles are generally defined as three-dimensional colloidal hydrogel nanoparticles obtained by physical or chemical crosslinking of the polymers with a diameter < 200 nm, thus possessing both the advantages of a hydrogel and characteristics of a nanocarrier system (Goldberg et al., 2007; Zhang et al., 2021a). Traditionally, nanogels have been classified as physically or chemically covalently cross-linked according to the synthesis method. Nanogels can also be classified based on the network structure including hollow, core-shell, hairy, multilayered, and core-shell core cross-linking. In addition, according to their responses to environmental stimuli, they can also be divided into response and nonresponse types.
Similar to the hydrogels, the hydrophilic groups in the polymeric structure of nanogels provide for a high water retention capacity (Amoli-Diva et al., 2017; Mauri et al., 2021; Stawicki et al., 2021). Nanogels have unique advantages in CNS drug delivery, especially in increasing the penetration of the drugs through the BBB, enhancing the stability of the bioactive molecules against enzymatic degradation, and reducing the cytotoxic side effects. Compared with other nanocarriers, nanogels have the following unique characteristics (Figure 3):1) Tunable nanosize: nanogels have a large specific surface area and, more importantly, can be engineered with an adapted nanosize based on the target tissue/organ, enabling them to efficiently cross cellular and biological barriers. 2) Colloidal stability: nanogels possess higher stability in physiological environments. 3) Swelling behavior: swelling/deswelling is one of the most important properties of nanogels and can be controlled by their design with suitable parameters (such as polymers, cross-linking forms, and functional structures). Furthermore, these behavior properties can be altered by responding to external stimuli. 4) Drug loading and easy surface modification: the diversity of polymeric materials and simple modification of their physical or chemical characteristics enables the creation of nanogels with versatile formulations. Depending on the crosslinked polymer network, such as the hydrophilic/lipophilic groups of the monomers, surfactants, surface charge, and crosslinking agents, various types of nanogels can deliver almost all types of therapeutic agents, including active biomacromolecules (DNA and SiRNA), hydrophobic/hydrophilic drugs, proteins, vaccines and even immunetherapeutics (Coviello et al., 2007; Lombardo et al., 2020; Chander et al., 2021; Tang et al., 2022). 5) Active targeting and controlled release: nanogels can be synthesized by crosslinking natural (e.g., alginate, dextran, and hyaluronic acid) or artificial polymers (e.g., methylcellulose, chitosan, and cyclodextrin). These polymers are nontoxic and stable and ensure high cell viability in the studies, demonstrating that nanogels are inherently biocompatible and biodegradable, which can avoid excessive accumulation in the tissues (Soni et al., 2016b; Mathew et al., 2018). 6) Non immune response: due to their high water-holding capacity a which enables them to absorb large amounts of nonimmunoreactive liquids, usually nanogel formulations do not produce any immune response; 7) Biodegradability: nanogels are synthesized from natural materials or polymers, which can be degraded in a nontoxic manner in living organisms and thus avoid organ accumulation.
Progress of Nanogel Drug Carrier Systems
Nanogels can be accurately modified with respect to their shape, charge, and surface function in response to various internal stimuli (changes in pH, redox conditions, or enzymes), which are usually associated with the majority of physiological conditions in vivo because most pathological processes generally induce certain changes in pH, redox levels, or specific complementary ligand expression levels. A continuous increase in the availability of the functional and macromolecular monomers can expand the response range of these nanogels (Stuart et al., 2010; Sun et al., 2014). Furthermore, comparison with traditional nanomaterial-based controlled release systems indicates that stimulus-responsive nanogels can react to external stimuli, such as light, electricity, and magnetism, which can control drug release by reversible expansion or contraction of the gels (Zhang et al., 2020). Zhang et al. formed ultra-pH-sensitive nanogels through the self-assembly of an ultra-pH-sensitive hydrogel from the chiral peptide derivative ferrocene-diphenylalanine (FC-FF). The material precisely responds to the changes in pH over a very narrow range (pH 5.7–5.9) (Liu et al., 2020a). Polypeptide, a promising biomedical polymer with biodegradability and biocompatibility, was approved in 1906 α- Ring opening polymerization (ROP) of amino acid n-carboxylic anhydride (NCA) was synthesized for the first time. At present, peptide based nano gel has been applied to the targeted delivery of therapeutic drugs for various diseases (Shi et al., 2017). Previously, our team synthesized the dual-response nanogels, NG/DOX, which respond simultaneously to a low pH level and a high GSH level. After intravenous injection of NG/DOX into tumor-bearing mice, the drug release from nanogel is triggered at a low pH level and a high GSH level. The antitumor effect of NG/DOX is far superior to that of free DOX hydrochloride, and nanogel has extremely low cytotoxicity (Figure 4).. (Huang et al., 2015; Suhail et al., 2019)
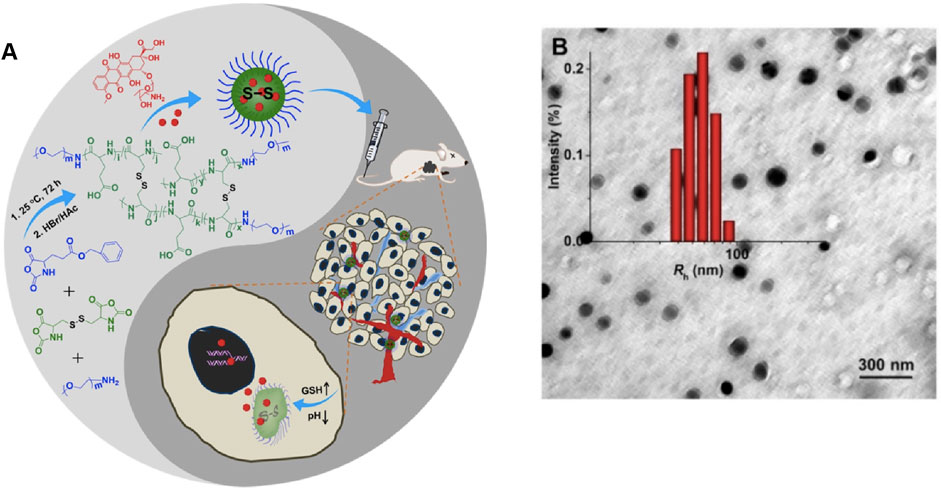
FIGURE 4. (A) Synthetic pathway for mPEG−P (LG-co-LC) nanogel, illustration of DOX encapsulation by nanogel, and its circulation, intratumoral accumulation, endocytosis, and targeted intracellular DOX release after intravenous injection (Huang et al., 2015). (B) Typical TEM micrographs and Rh NG/DOX. Copyright 2017 Ivyspring International Publisher.
Ion-induced gelation has attracted considerable attention due to environmentally friendly and time-controlled properties (Lee et al., 2021). Recently, researchers have developed stimuli-responsive DNA noncationic nanogels that can be used for targeted delivery of combination cancer therapeutics with high biocompatibility (Oh et al., 2007). Degradable nanogels loaded with rhodamine B isothiocyanate dextran (RITC DX) were shown to be degraded into a polymeric sol in a reducing environment, thus releasing the encapsulated carbohydrate drugs (Vinogradov, 2010). Based on nanogel technology, many therapeutic strategies for the delivery/release of therapeutic drugs to the brain have been explored to achieve active targeting: 1) by attaching functionalized ligands that recognize homologous receptors on the target organs or tissues, 2) due to noninvasive responsiveness to magnetic field or ultrasound to disrupt the BBB, and 3) due to shutter peptide-mediated BBB crossing (Figure 5). (Ganguly et al., 2014; Chaurasiya et al., 2016; Wei et al., 2021) Furthermore, nanogels with encapsulated drugs can be delivered by a variety of methods, such as intravenous or intraperitoneal injection, oral administration, and nasal and intraocular drug delivery (Verma et al., 2011; Zhao et al., 2021). Reports have suggested that PEGylation renders nanogel surface more hydrophilic, shields the drugs, and provides steric hindrance to avoid the interactions with serum proteins, endowing nanogels with a “stealth” feature (Kamaly et al., 2012; Sun et al., 2014).
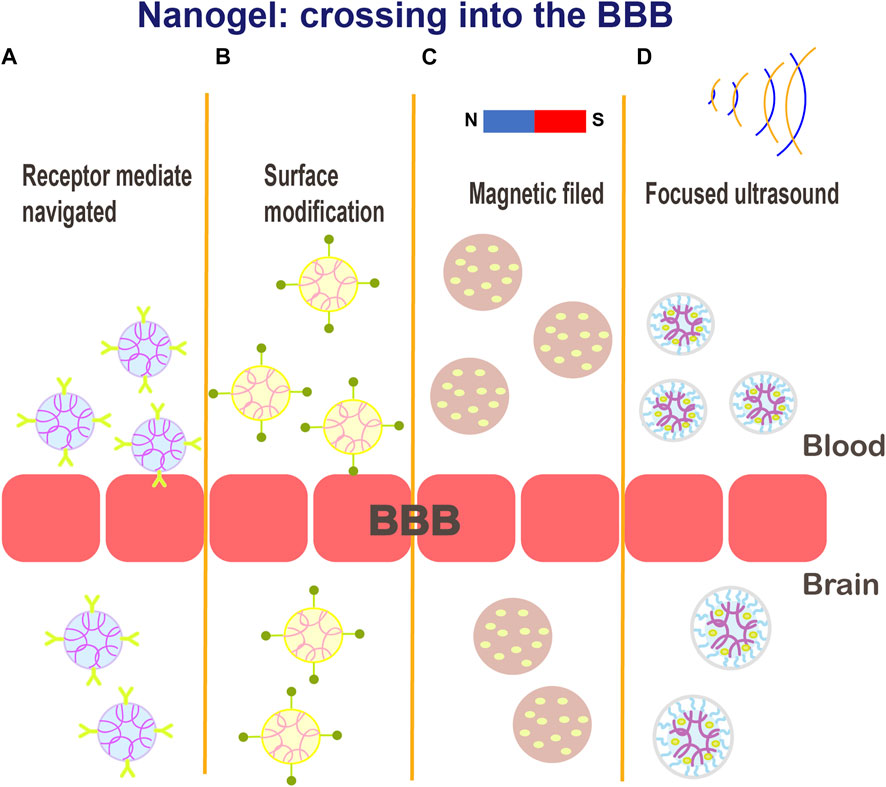
FIGURE 5. Schematic illustration of the various potential mechanisms for crossing the BBB: (A) receptor-mediated endocytosis; (B) functionalized ligands that recognize cognate receptors on target organs or tissues; (C–D) magnetic field or ultrasound mediated delivery.
The modulation of nanogels (e.g., ligands complementary to receptors) can direct them to the affected tissues with lesions, which differentially express the corresponding receptors, thereby facilitating the uptake and retention of the drugs at a target site (Saha et al., 2021). Vinogradov et al. synthesized a new system based on a nanogel network of crosslinked PEG and PEI, which is capable of efficiently delivery of ODNs to the brain across the BBB. The transport efficiency was further improved when the surface of nanogels was modified with Tf or insulin. The results of distribution studies in a mouse model showed that the accumulation of ODN in the brain was increased by more than 15-fold after 1 h of intravenous injection compared with the accumulation of unincorporated ODN, and the accumulation of free ODN in the liver and spleen was reduced by 2-fold, implying that clearance of ODN from the blood was not accelerated (Vinogradov et al., 2004). Zwitterionic-based nanogels have greatly broadened the applications of nanogels in drug delivery by virtue of their characteristics such as superhydrophilicity (Peng et al., 2020). In this context, zwitterionic polysulfamide nanogels (PMEDAPA) modified with transferrin (Tf) were synthesized as drug carriers that effectively respond to hyperthermia. These nanogels have their tumor-targeting features shielded at normal temperature; at high temperature, tumor targeting is achieved to enhance the accumulation of chemotherapeutic drugs in the tumor. These finding provides an exciting rationale to achieve tumor targeting of nanogels and to enable on-demand drug release in microwave heating-assisted cancer therapy in a clinical setting (Muresanu et al., 2019).
Overall, nanogels are more novel and advanced nanocarrier that are characterized by superior efficacy, bioavailability, and favorable drug pharmacokinetics, render their the potential side effects are greatly reduced.
Applications of the Nanogels for CNS Drug Delivery
The advantages of simple and stable synthetic routes, controllable drug release, and high targeting efficiency make nanogel drug carriers one of the preferred options for the treatment of various CNS diseases, such as stroke, neurodegenerative disorders, epilepsy, traumatic brain injury, and brain tumors. Table 3 summarizes several major studies related to the design of nanogels used for CNS drug delivery.
Ischemic Stroke
Ischemic stroke occurs when a blood clot or embolus locally blocks the middle brain artery, which accounts for 85% of all types of stroke (Houng et al., 2014). Currently, the thrombus-dissolving agents, such as tissue plasminogen activator (tPA), are available for the treatment of ischemic stroke; however, a narrow time window for the use of these agents and cerebral ischemia/reperfusion injury often cause serious pathological reactions, which produce unsatisfactory results of these conventional treatment approached for ischemic stroke (Mihalko et al., 2022). Nanogels are expected to expand the arsenal of ischemic stroke treatment strategies by achieving brain targeting of the drugs and local controlled release.
Mihalko et al. designed a fibrin-specific nanogel (FSN) that can be used for the targeted delivery of tPA. In vivo experiments confirmed that tPA-FSNs can modulate fibrin/fibrinogen and platelets in thrombi. The distribution of both FSN and tPA-FSNs showed potential clearance and very low toxicity after 24 h. (Cui et al., 2016) A recent study suggested that a new form of urokinase (United Kingdom)-containing PEG-conjugated nanogel with pH-sensitive properties (PEG-UK) was designed to release the payload at a certain pH value. PEG-UKs were detected at the regions of microcirculation with low pH in a rat model of ischemic stroke. Wei Cui at el. demonstrated that the administration of PEG-UKs reduces the infarct volume of ischemic stroke, and this effect protects the BBB, inhibits apoptosis, and decreases neurotoxicity (Kleindorfer et al., 2005). However, high incidence of hemorrhagic events and failure to hospitalize in time usually limit the application of thrombolytic drugs. Statistical data show that very few patients receive thrombolytic therapy within 3 h after ischemic stroke (He et al., 2021). Multiple findings have shown that the generation of reactive oxygen species (ROS) or reactive nitrogen species (RONS) associated with cerebral ischemia and reperfusion worsen the conditions in patients. To reduce ischemic injury caused by oxidative stress, Zhang et al. developed artificial nanogel-zymes with multiple enzyme activities, which were able to provide neuroprotection against ischemic stroke by scavenging RONS. In a rat model of ischemic stroke, RONS levels were significantly reduced, and side effects were minimal (Liu et al., 2021).
The latest studies have shown that administration of microRNAs (miRNAs) can promote blood vessel growth and help restore the function of damaged tissues. Liu et al. encapsulated miRNAs in a nanocapsule platform, which systematically and effectively delivered miRNAs (Davis, 2016). However, the delivery of miRNA by nanogel delivery platform against cerebral ischemia has yet to be investigated. Moreover, future work should assess in detail the ability of these nanoformulations to be translated to the clinic.
Brain Tumors
Specific targeted drug delivery is the future of brain tumor therapy. Gliomas are the most prevalent and malignant CNS tumors, with a median patient survival of less than 15 months despite aggressive use of surgery combined with radiotherapy and chemotherapy (Kim et al., 2018). The blood-brain tumor barrier (BBTB) forms with tumor progression, which blocks almost all small-molecule chemotherapeutics and biomacromolecules. In addition to the limitations of the barriers, most therapeutic agents for glioma are the substrates of biological barrier efflux transporters, and a combination of these obstacles contribute to a high rate of treatment failure (Agarwal et al., 2011; Feng et al., 2019). Gliomas, similar to other solid tumors, have a special tumor microenvironment (TME) characterized by hypoxia, low pH, and chronic inflammation. Intriguingly, the extracellular glutathione concentrations of gliomas are up to 1,000-fold higher than the extracellular glutathione concentrations in the normal tissues, and the pH values in the vicinity of tumor cells are significantly lower (Marí et al., 2009; Wen et al., 2019).
Based on specific characteristics of the tumor tissue, various stimulus-responsive nanogel drug delivery networks have been developed for antitumor drug delivery. Methotrexate, doxorubicin, rituximab, and temozolomide are routine chemotherapeutic agents toxic for glioma cells, which have been formulated as nanomedicines to significantly improve the efficiency of the BBB crossing. The membrane protein connexin 43 (Cx43) and brain-specific anion transporter (BSAT1) are characteristically expressed in gliomas and the adjacent tissues. Recently, cisplatin was loaded into nanogels conjugated to Cx43 and BSAT1 monoclonal antibodies. MRI analysis of tumor-bearing rats showed that the nanoformulation achieved targeted antitumor effects (Gadhave et al., 2021). A recent study demonstrated successful loading of teriflunomide into a nanolipid-based (NLC) carbopol-gellan gum nanogel (TNLCGHG) for the treatment of brain glioma via intranasal administration. These gels were demonstrated to prolong blood circulation and showed significant tumor-suppressive effects (Zhang et al., 2021b). Nanogel formulations have been developed as promising contrast agents. Jiang et al. developed Cy5.5-Lf-MPNA nanogels by labeling lactoferrin (Lf) with Cy5.5. This preparation can target tumor tissue and achieve MR/fluorescence imaging with high sensitivity and specificity in the acidic environment of glioma tissue (Cui et al., 2016). Similarly, Lf/phenylboronic acid (PBA)-reduction-sensitive dual-target nanogels (Lf-DOX/PBNG) were developed for the delivery to deliver doxorubicin (DOX) for glioma therapy. The effective accumulation of Lf-DOX/PBNG was 12.37 times greater than that of free DOX solution (Rizzi et al., 2014).
Alzheimer’s Disease
Alzheimer’s disease (AD) is the most common cause of dementia, with more than 50 million people worldwide currently living with dementia (DeTure and Dickson, 2019). Clinical therapeutic approaches for AD focus on lowering the levels of the toxic forms of the amyloid beta (Aβ) peptide and τ protein to effectively delay the progression of the disease (Giacobini and Gold, 2013; Yang et al., 2021). A range of limitations account for poor efficacy of most therapeutic drugs, such as hydrophobicity, poor BBB permeability, rapid metabolism, and strong tissue toxicity. Various nanogel technologies have enabled the treatment of neurodegenerative diseases (Jiang et al., 2018).
The development of the dual inhibitor nanosystems is expected to effectively target the inhibition of Aβ aggregation and cytotoxicity induced by drug delivery systems. The biocompatible nanogels of cholesterol-bearing pullulan (CHP) were shown to be able to significantly inhibit the formation of Aβ fibrils. Epigallocatechin-3-gallate (EGCG) and curcumin are able to effectively inhibit Aβ aggregation. These two inhibitors were linked via a modification of hyaluronic acid (HA). The results showed that the EGCG and curcumin dual-modified nanogels (CEHA), which were synthesized by self-assembly, induced 69 and 55% higher inhibition than EGCG- or EHA-loaded single-modified nanogels, respectively. The results of an in vitro toxicity assay showed that CEHA significantly improved the viability of SH-SY5Y cells (Zhang et al., 2021c). Another approach to AD therapy involves scavenging of excess ROS induced by mitochondrial dysfunction and inflammatory factor that are overactivated in the brain (McNaught et al., 2002). Oxytocin-loaded angiopep-2-modified AOC NGs were developed to cross the BBB via the transcytosis of a surface-loaded ligand (ANG) for enrichment in the AD lesions. AOC NGs can block the ERK/p38 MAPK and COX-2/iNOS NF-κB signaling pathways, showing the ability to effectively inhibit microglial activation and reduce inflammatory cytokine levels.
Advances in nanotechnology have enabled new prospects for clinical therapeutic strategies for AD; however, the field of AD nanotherapeutics faces several challenges. AD may lead to a variety of clinical complications; hence, the design of the nanogel drugs with multiple drug candidates to achieve a synergistic effect may enhance the benefits of AD treatment.
Parkinson’s Disease
Parkinson’s disease (PD) is the second most common neurodegenerative disorder characterized by the death of dopaminergic neurons in the substantia nigra and the formation of Lewy bodies (Hawthorne et al., 2016). Dopamine therapy is the primary treatment option for PD; however, the disease is currently considered incurable. The demands for nanomedical studies of PD are mainly focused on achieving a stable concentration of dopamine in the brain (Jiang et al., 2018; Khan et al., 2018).
PEGylated nanogels were engineered to load dopamine and modified ligands of the transferrin receptor. The results of in vivo experiments demonstrated that the concentration of dopamine in the brain obtained using this nanogel was nine times higher than that obtained in a rat model treated with free dopamine. The removal of Lewy bodies is another effective strategy for the treatment of PD (Liu et al., 2020b). A study developed the Lewy body antagonist NanoCA using a self-assembly reaction. NanoCA targets the brain, releases its cargo in a controlled manner, and protects the neurons from the neurotoxicity of PD inducers in the animal models. In PD animal models, the nanomaterials are administered by local injection or transdermal absorption. Ropinirole nanogels were proved to enhance the efficacy of transdermal absorption, thus increasing the bioavailability up to two-fold compared with transdermal methods of delivery of the unincorporated free drug (Jafarieh et al., 2015). Jafarieh et al. prepared ropinirole hydrochloride (RH)-loaded chitosan nanoparticles (RH-CSNPs) by the ionic gel method. RH-CSNPs with nasal mucosal absorbability continuously released their cargo for 18 h, and the RH concentration in the brain was significantly increased after intranasal administration (Leoni and Caccia, 2014). The first-line treatment for patients with Parkinson’s disease is natural oral levodopa, which has shown higher efficiency than synthetic levodopa. C Chittasupho et al. encapsulated native levodopa from M. pruriens seed extract into nanogels for incorporated into a jelly as a functional food for patients with Parkinson’s disease.
Finally, although the prospects of using nanogel technology to treat neurodegenerative diseases are very attractive, the actual studies performed to date remain only experimental.
Huntington’s Disease
Huntington’s disease (HD) is an autosomal dominant neurodegenerative disorder (Arrasate and Finkbeiner, 2012). The known molecular mechanism of HD involves a single mutation of the huntingtin (HTT) gene exon 1, which leads to polyQ expansion, resulting in the misfolding and aggregation of the huntingtin protein in the brain (Li et al., 2020). However, the molecular mechanism by which HTT mutation causes neuronal death remains unclear. At present, many studies have demonstrated that short interfering RNAs (siRNAs) can silence the expression of the mutant proteins, and this application is one of the most recent and promising therapeutic strategies (Godinho et al., 2013).
Godinho et al. developed modified amphiphilic β-cyclodextrin (CD) oligosaccharide molecules as novel neuronal siRNA vectors. The results showed that CD nanoparticles are stable in artificial cerebrospinal fluid. The nanocarrier complex reduces HTT gene expression in ST14A-Htt120q rat striatum cells and primary human HD fibroblasts. A single injection of the CD-siRNA nanoparticles significantly reduces HTT expression in the striatum in a mouse HD model, and multiple injections can alleviate the motor dysfunction in HD mice. In addition, low toxicity of CD-siRNA nanoparticles has been observed in vitro toxicity experiments (Löscher et al., 2020). Numerous efforts have demonstrated the potential efficacy of the nanogel drug delivery systems; however, limited data on in vivo toxicity suggest a need to determine potential long-term systemic toxicity.
Epilepsy
Epilepsy is the second most frequent chronic disease of the CNS. Approximately 30% of patients with epilepsy are characterized by poorly controlled drug release and drug resistance during clinical treatment (Hanada, 2014; Shringarpure et al., 2021). Nanogel technology promises to revolutionize the treatment strategies for epilepsy using unique advantages due to the ability to cross the BBB without toxic effects to the brain and other tissues.
Lamotrigine is a broad-spectrum antiepileptic. Only a small amount of potent lamotrigine can exert its antiepileptic effects through the BBB after oral absorption (Xu et al., 2022). The group of Xu designed a polymer hydrogel that specifically responded to electromagnetic radiation. The results of intravital imaging of rats showed that fluorescently labeled lamotrigine nanogels are enriched in the rat brain 3 h after intravenous injection, indicating that lamotrigine nanogels are characterized by enhanced BBB penetration. Comparison with free lamotrigine indicated that the seizures of rats in the lamotrigine nanogel group were continuously and significantly decreased (Wang et al., 2016).
Recently, Wang et al. designed electroresponsive hydrogel nanoparticles (eRHNPs) modified with brain-targeting angiopep-2 (ANG) to facilitate the delivery of the antiepileptic drug phenytoin (PHT) and subsequently developed PHT-loaded ANG-eRHNPs. These formulations achieved a high distribution in the brain and an electrical response in a rat epilepsy model, resulting in a strong release of PHT from nanogels during seizures and achieving better antiepileptic effects (Ying et al., 2014). Brain-targeted ANG peptide-modified electro-responsive nanogels have been confirmed to achieve high-efficiency BBB penetration and enhance the efficacy of alleviating epilepsy (Wilson et al., 2017).
These studies will enable the generation of safe and effective seizure therapeutics. Future attention should also be paid to human clinical trials to evaluate and select precise nanodosage forms and concentrations for subsequent clinical applications.
Traumatic Brain Injury
Traumatic brain injury (TBI) is the main cause of death and disability in young individuals under 45 years of age and a known risk factor for chronic neurodegenerative diseases, such as AD and PD. To date, effective clinical treatments for TBI are lacking (McKee and Robinson, 2014; Gong et al., 2022).
The application of the bioactive scaffold materials is a promising method for tissue regeneration and repair (Marçal et al., 2012). Bladder stroma extracted from the porcine bladder tissue (UBM) showed good performance in promoting and supporting neural cell growth in vitro experiments (Zhang et al., 2013). To improve the biocompatibility of UBM in the brain and the effect of UBM on the function after TBI, a hydrogel-based form of UBM was developed. The results showed that the UBM-hydrogel had weak toxic effects on the healthy brain. After TBI, the application of the nanoscale UBM-hydrogel reduced the volume of the lesions and alleviated myelin sheath breakage induced by traumatic injury (Xu et al., 2019). Unexpectedly, the treatment of TBI using the UBM-hydrogel significantly improved neurobehavioral and vestibulomotor functions. The application of nanogels not only greatly improved the biocompatibility of UBM but also revealed a protective effect on the injured brain tissue. These observations provide valuable insight into the potential efficacy of nanogels in structurally damaged brain tissue.
Recently, Xu et al. successfully demonstrated that nanocapsules loaded with nerve growth factor (NGF) enabled nerve recovery and tissue remodeling in mice with spinal cord injury (Teng et al., 2018). It is suggested that nanogel technology may provide a better approach for TBI and CNS tissue regeneration engineering, which needs to be more explored in the future.
Potential Neurotoxicity
The CNS strongly protects itself from any xenobiotics (pathogens, toxins, and foreign bodies) through strict barrier structures (Nance et al., 2014). Nanocarriers can effectively circumvent the BBB and deliver unevenly distributed drugs to the brain parenchyma (Xiao et al., 2015); however, this delivery also leads to overexposure to the nanomaterials, making the application of the nanomaterials for CNS therapy regarded as a dual-edged sword. Therefore, it is crucial to systematically investigate the potential toxicity of the nanomaterials, which is one of the biggest challenges for the clinical translation of the nanodrug delivery systems.
Existing neurotoxicity studies have shown that the nanocarriers have neurotoxic effects both in vitro and in vivo induced by the nanoparticles composed mostly of inorganic materials, whereas complete assessment of potential toxicity of nanogels in humans has not been reported (Wong et al., 2012). The present review analyzed the main reasons associated with neurotoxicity of nanogels. Chemically crosslinked nanogels may release toxic monomers from the matrix during their expected chemical degradation (Lee et al., 2021). Inflammation is one of the main mechanisms of neurotoxicity caused by the nanomaterials. Inflammation is known to eliminate foreign substances from the body and is a protective response; however, overactivation of the inflammatory response will induce substantial damage. Exposure of the brain to nanogels may stimulate glial cells, causing a strong inflammatory response of neuronal mitochondria or other organelles (Trompetero et al., 2018; Wu and Tang, 2018). The mechanism of brain inflammation induced by nanogels needs additional research, and only a few relevant studies of this subject have been performed.
In addition, neuronal exposure to the nanomaterials may induce neurodegenerative diseases. The accumulation of some fibrin aggregates or misfolded proteins is the pathological mechanism of some neurodegenerative diseases (AD, PD, and HD). Plausible interactions between the CNS and nanomaterials may exacerbate the accumulation or misfolding of these protein aggregates (Pichla et al., 2020). The results reported by Alvarez et al. suggest that the induction of a neurodegenerative disease may depend on the size and concentration of the nanoparticles and on their biocompatibility (Oberdörster, 2010). Current data of the assessments of the toxicity of the nanomaterials suggest that rigorous in vitro, in vivo, and clinical toxicity studies are needed before the clinical transformation of the nanomaterials can be achieved (Wang et al., 2022). Emphasis on detailed toxicity studies of nanogels is needed to provide evolutionary insight into the risks associated with efficient applications of these promising targeting approaches.
Discussion
Overall, nanogel technology can provide the possibilities for advanced treatment in the field of CNS diseases (Sarkar et al., 2017). The BBB is the major anatomical and physiological dynamic barrier, representing one of the narrowest bottlenecks for successful treatment of CNS diseases; hence, the development of safer and more effective targeted nanomedicines has become a major research direction for numerous nanoformulation studies. Continuous development of new nanogel technologies, such as surface modification of the receptors/ligands and magnetic structures, enables various nanogel-based approaches to overcome the BBB to achieve targeted drug delivery. Nanogel-based technologies have been implicated in the development of the nanocarriers of various neuroprotective drugs, including nucleic acids, small-molecule peptides, nerve growth factors, and free radical scavengers (e.g., edaravone). However, the nanogel drug delivery platforms are still in their infancy (Jogani et al., 2008; Kumar et al., 2017; Patel et al., 2021). Therefore, additional in-depth studies on nanogels are required to address several issues before these agents can be widely used in the clinic.
Specific targeted delivery is a future research direction for nanogel studies. Recent discoveries of several specific peptides or receptor-targeting drugs, such as Tf, ANG and human insulin receptor, can facilitate the development of effective nanogels for brain-targeted drug delivery. However, these receptors are not specific; thus, organ/tissue specificity should also be considered in the future studies to provide more specific and rational therapeutic strategies. Due to the outstanding properties associated with the suppression of reactive oxygen species and protein misaggregation, the application of nanogels have been eagerly explored for the treatment of neurological diseases, such as ischemic stroke, AD, PD, brain tumors, and epilepsy (Liu et al., 2018). However, the efficacy of these carrier systems is hindered by the involvement of various pharmacological factors, such as drug loading, controlled release, safety, and biocompatibility in vivo. Furthermore, considering the complex structure and unique microenvironment of the brain, additional polymeric materials should be developed to enhance biodegradability and biocompatibility by preparing nanogels with completely eliminated possibilities of potential toxicity.
Most CNS diseases require long-term drug therapy; thus, toxicological studies of the organs, including the kidney, liver, and spleen, should be performed using nanogel formulations (Picone et al., 2018). In the future, advanced diagnostic techniques, such as magnetic resonance imaging, positron emission tomography, and computed tomography, aphy, will be needed to assess nanocarrier-related CNS toxicity.
Author Contributions
HL and YZ contributed to conception and design of the study. YZ wrote the first draft of the manuscript. All authors contributed to manuscript revision, read, and approved the submitted version.
Funding
This study was funded by National Natural Science Foundation of China (No. 81971174).
Conflict of Interest
TH is employed by Evergreen Therapeutics, Inc. The author declares that the research was conducted in the absence of any commercial or financial relationships that could be construed as a potential conflict of interest.
Publisher’s Note
All claims expressed in this article are solely those of the authors and do not necessarily represent those of their affiliated organizations, or those of the publisher, the editors and the reviewers. Any product that may be evaluated in this article, or claim that may be made by its manufacturer, is not guaranteed or endorsed by the publisher.
References
Aday, S., Cecchelli, R., Hallier-Vanuxeem, D., Dehouck, M. P., and Ferreira, L. (2016). Stem Cell-Based Human Blood-Brain Barrier Models for Drug Discovery and Delivery. Trends Biotechnol. 34 (5), 382–393. doi:10.1016/j.tibtech.2016.01.001
Agarwal, S., Sane, R., Oberoi, R., Ohlfest, J. R., and Elmquist, W. F. (2011). Delivery of Molecularly Targeted Therapy to Malignant Glioma, a Disease of the Whole Brain. Expert Rev. Mol. Med. 13, e17. doi:10.1017/s1462399411001888
Ali, E. S., Sharker, S. M., Islam, M. T., Khan, I. N., Shaw, S., Rahman, M. A., et al. (2021). Targeting Cancer Cells with Nanotherapeutics and Nanodiagnostics: Current Status and Future Perspectives. Seminars Cancer Biol. 69, 52–68. doi:10.1016/j.semcancer.2020.01.011
Alyautdin, R., Khalin, I., Nafeeza, M. I., Haron, M. H., and Kuznetsov, D. (2014). Nanoscale Drug Delivery Systems and the Blood-Brain Barrier. Int. J. Nanomedicine 9, 795–811. doi:10.2147/IJN.S52236
Amoli-Diva, M., Sadighi-Bonabi, R., and Pourghazi, K. (2017). Switchable On/off Drug Release from Gold Nanoparticles-Grafted Dual Light- and Temperature-Responsive Hydrogel for Controlled Drug Delivery. Mater. Sci. Eng. C 76, 242–248. doi:10.1016/j.msec.2017.03.038
Arrasate, M., and Finkbeiner, S. (2012). Protein Aggregates in Huntington's Disease. Exp. Neurol. 238 (1), 1–11. doi:10.1016/j.expneurol.2011.12.013
Bae, K. H., Lee, J. Y., Lee, S. H., Park, T. G., and Nam, Y. S. (2013). Optically Traceable Solid Lipid Nanoparticles Loaded with siRNA and Paclitaxel for Synergistic Chemotherapy with In Situ Imaging. Adv. Healthc. Mater. 2 (4), 576–584. doi:10.1002/adhm.201200338
Baeten, K. M., and Akassoglou, K. (2011). Extracellular Matrix and Matrix Receptors in Blood-Brain Barrier Formation and Stroke. Devel Neurobio 71 (11), 1018–1039. doi:10.1002/dneu.20954
Bajracharya, R., Song, J. G., Back, S. Y., and Han, H.-K. (2019). Recent Advancements in Non-invasive Formulations for Protein Drug Delivery. Comput. Struct. Biotechnol. J. 17, 1290–1308. doi:10.1016/j.csbj.2019.09.004
Baroud, M., Lepeltier, E., Thepot, S., El-Makhour, Y., and Duval, O. (2021). The Evolution of Nucleosidic Analogues: Self-Assembly of Prodrugs into Nanoparticles for Cancer Drug Delivery. Nanoscale Adv. 3 (8), 2157–2179. doi:10.1039/d0na01084g
Battaglia, L., Gallarate, M., Peira, E., Chirio, D., Muntoni, E., Biasibetti, E., et al. (2014). Solid Lipid Nanoparticles for Potential Doxorubicin Delivery in Glioblastoma Treatment: Preliminary In Vitro Studies. J. Pharm. Sci. 103 (7), 2157–2165. doi:10.1002/jps.24002
Begicevic, R. R., and Falasca, M. (2017). ABC Transporters in Cancer Stem Cells: Beyond Chemoresistance. Int. J. Mol. Sci. 18 (11), 2362. doi:10.3390/ijms18112362
Bhia, M., Motallebi, M., Abadi, B., Zarepour, A., Pereira-Silva, M., Saremnejad, F., et al. (2021). Naringenin Nano-Delivery Systems and Their Therapeutic Applications. Pharmaceutics 13 (2), 291. doi:10.3390/pharmaceutics13020291
Bonsack, B., Corey, S., Shear, A., Heyck, M., Cozene, B., Sadanandan, N., et al. (2020). Mesenchymal Stem Cell Therapy Alleviates the Neuroinflammation Associated with Acquired Brain Injury. CNS Neurosci. Ther. 26 (6), 603–615. doi:10.1111/cns.13378
Buyukkoroglu, G., Senel, B., Basaran, E., Yenilmez, E., and Yazan, Y. (2016). Preparation and In Vitro Evaluation of Vaginal Formulations Including siRNA and Paclitaxel-Loaded SLNs for Cervical Cancer. Eur. J. Pharm. Biopharm. 109, 174–183.
Chander, S., Kulkarni, G. T., Dhiman, N., and Kharkwal, H. (2021). Protein-Based Nanohydrogels for Bioactive Delivery. Front. Chem. 9, 573748. doi:10.3389/fchem.2021.573748
Chaurasiya, B., Mahanty, A., Roy, D., Shen, Y., Tu, J., and Sun, C. (2016). Influence of Tumor Microenvironment on the Distribution and Elimination of Nano-Formulations. Cdm 17 (8), 783–798. doi:10.2174/1389200217666160607093347
Chen, Z., Lv, Z., Zhang, Z., Weitz, D. A., Zhang, H., Zhang, Y., et al. (2021). Advanced Microfluidic Devices for Fabricating Multi‐structural Hydrogel Microsphere. Exploration 1 (3), 1. doi:10.1002/exp.20210036
Cojocaru, F. D., Botezat, D., Gardikiotis, I., Uritu, C. M., Dodi, G., Trandafir, L., et al. (2020). Nanomaterials Designed for Antiviral Drug Delivery Transport across Biological Barriers. Pharmaceutics 12 (2), 171. doi:10.3390/pharmaceutics12020171
Coviello, T., Matricardi, P., Marianecci, C., and Alhaique, F. (2007). Polysaccharide Hydrogels for Modified Release Formulations. J. Control. Release 119 (1), 5–24. doi:10.1016/j.jconrel.2007.01.004
Cui, W., Liu, R., Jin, H., Lv, P., Sun, Y., Men, X., et al. (2016). pH Gradient Difference Around Ischemic Brain Tissue Can Serve as a Trigger for Delivering Polyethylene Glycol-Conjugated Urokinase Nanogels. J. Control. Release 225, 53–63. doi:10.1016/j.jconrel.2016.01.028
Date, A., Joshi, M., and Patravale, V. (2007). Parasitic Diseases: Liposomes and Polymeric Nanoparticles versus Lipid Nanoparticles☆. Adv. Drug Deliv. Rev. 59 (6), 505–521. doi:10.1016/j.addr.2007.04.009
Davis, M. E. (2016). Glioblastoma: Overview of Disease and Treatment. Clin. J. Oncol. Nurs. 20 (5 Suppl. l), S2–S8. doi:10.1188/16.CJON.S1.2-8
De Jong, W. H., Hagens, W. I., Krystek, P., Burger, M. C., Sips, A. J. A. M., and Geertsma, R. E. (2008). Particle Size-dependent Organ Distribution of Gold Nanoparticles after Intravenous Administration. Biomaterials 29 (12), 1912–1919. doi:10.1016/j.biomaterials.2007.12.037
de Souza, M. L., Dos Santos, W. M., de Sousa, A. L. M. D., de Albuquerque Wanderley Sales, V., Nóbrega, F. P., de Oliveira, M. V. G., et al. (2020). Lipid Nanoparticles as a Skin Wound Healing Drug Delivery System: Discoveries and Advances. Cpd 26 (36), 4536–4550. doi:10.2174/1381612826666200417144530
Debele, T. A., Mekuria, S. L., and Tsai, H.-C. (2016). Polysaccharide Based Nanogels in the Drug Delivery System: Application as the Carrier of Pharmaceutical Agents. Mater. Sci. Eng. C 68, 964–981. doi:10.1016/j.msec.2016.05.121
DeTure, M. A., and Dickson, D. W. (2019). The Neuropathological Diagnosis of Alzheimer's Disease. Mol. Neurodegener. 14 (1), 32. doi:10.1186/s13024-019-0333-5
Dowling, J., Isbister, G. K., Kirkpatrick, C. M. J., Naidoo, D., and Graudins, A. (2008). Population Pharmacokinetics of Intravenous, Intramuscular, and Intranasal Naloxone in Human Volunteers. Ther. Drug Monit. 30 (4), 490–496. doi:10.1097/ftd.0b013e3181816214
Dutta, T., and Jain, N. K. (2007). Targeting Potential and Anti-HIV Activity of Lamivudine Loaded Mannosylated Poly (Propyleneimine) Dendrimer. Biochimica Biophysica Acta (BBA) - General Subj. 1770 (4), 681–686. doi:10.1016/j.bbagen.2006.12.007
Engelhardt, B., and Sorokin, L. (2009). The Blood-Brain and the Blood-Cerebrospinal Fluid Barriers: Function and Dysfunction. Semin. Immunopathol. 31 (4), 497–511. doi:10.1007/s00281-009-0177-0
Erickson, M. A., and Banks, W. A. (2018). Neuroimmune Axes of the Blood-Brain Barriers and Blood-Brain Interfaces: Bases for Physiological Regulation, Disease States, and Pharmacological Interventions. Pharmacol. Rev. 70 (2), 278–314. doi:10.1124/pr.117.014647
Falagas, M. E., Bliziotis, I. A., and Tam, V. H. (2007). Intraventricular or Intrathecal Use of Polymyxins in Patients with Gram-Negative Meningitis: a Systematic Review of the Available Evidence. Int. J. Antimicrob. Agents 29 (1), 9–25. doi:10.1016/j.ijantimicag.2006.08.024
Fassas, A., and Anagnostopoulos, A. (2005). The Use of Liposomal Daunorubicin (DaunoXome) in Acute Myeloid Leukemia. Leukemia Lymphoma 46 (6), 795–802. doi:10.1080/10428190500052438
Feng, X., Xu, W., Li, Z., Song, W., Ding, J., and Chen, X. (2019). Immunomodulatory Nanosystems. Adv. Sci. 6 (17), 1900101. doi:10.1002/advs.201900101
Francischi, J. N., Pereira, L. S. M., and Castro, M. S. (1997). Cyclosporin Inhibits Hyperalgesia and Edema in Arthritic Rats: Role of the Central Nervous System. Braz J. Med. Biol. Res. 30 (1), 101–111. doi:10.1590/s0100-879x1997000100016
Furtado, D., Björnmalm, M., Ayton, S., Bush, A. I., Kempe, K., and Caruso, F. (2018). Overcoming the Blood-Brain Barrier: The Role of Nanomaterials in Treating Neurological Diseases. Adv. Mater 30 (46), e1801362. doi:10.1002/adma.201801362
Gadhave, D., Rasal, N., Sonawane, R., Sekar, M., and Kokare, C. (2021). Nose-to-brain Delivery of Teriflunomide-Loaded Lipid-Based Carbopol-Gellan Gum Nanogel for Glioma: Pharmacological and In Vitro Cytotoxicity Studies. Int. J. Biol. Macromol. 167, 906–920. doi:10.1016/j.ijbiomac.2020.11.047
Ganguly, K., Chaturvedi, K., More, U. A., Nadagouda, M. N., and Aminabhavi, T. M. (2014). Polysaccharide-based Micro/nanohydrogels for Delivering Macromolecular Therapeutics. J. Control. Release 193, 162–173. doi:10.1016/j.jconrel.2014.05.014
Gerson, T., Makarov, E., Senanayake, T. H., Gorantla, S., Poluektova, L. Y., and Vinogradov, S. V. (2014). Nano-NRTIs Demonstrate Low Neurotoxicity and High Antiviral Activity against HIV Infection in the Brain. Nanomedicine Nanotechnol. Biol. Med. 10 (1), 177–185. doi:10.1016/j.nano.2013.06.012
Ghersi-Egea, J.-F., Strazielle, N., Catala, M., Silva-Vargas, V., Doetsch, F., and Engelhardt, B. (2018). Molecular Anatomy and Functions of the Choroidal Blood-Cerebrospinal Fluid Barrier in Health and Disease. Acta Neuropathol. 135 (3), 337–361. doi:10.1007/s00401-018-1807-1
Giacobini, E., and Gold, G. (2013). Alzheimer Disease Therapy-Moving from Amyloid-β to Tau. Nat. Rev. Neurol. 9 (12), 677–686. doi:10.1038/nrneurol.2013.223
Gil-Martins, E., Barbosa, D. J., Silva, V., Remião, F., and Silva, R. (2020). Dysfunction of ABC Transporters at the Blood-Brain Barrier: Role in Neurological Disorders. Pharmacol. Ther. 213, 107554. doi:10.1016/j.pharmthera.2020.107554
Girolamo, F., de Trizio, I., Errede, M., Longo, G., d’Amati, A., and Virgintino, D. (2021). Neural Crest Cell-Derived Pericytes Act as Pro-angiogenic Cells in Human Neocortex Development and Gliomas. Fluids Barriers CNS 18 (1), 14. doi:10.1186/s12987-021-00242-7
Godinho, B. M. D. C., Ogier, J. R., Darcy, R., O’Driscoll, C. M., and Cryan, J. F. (2013). Self-assembling Modified β-Cyclodextrin Nanoparticles as Neuronal siRNA Delivery Vectors: Focus on Huntington's Disease. Mol. Pharm. 10 (2), 640–649. doi:10.1021/mp3003946
Goldberg, M., Langer, R., and Jia, X. (2007). Nanostructured Materials for Applications in Drug Delivery and Tissue Engineering. J. Biomaterials Sci. Polym. Ed. 18 (3), 241–268. doi:10.1163/156856207779996931
Gong, B., Zhang, X., Zahrani, A. A., Gao, W., Ma, G., Zhang, L., et al. (2022). Neural Tissue Engineering: From Bioactive Scaffolds and In Situ Monitoring to Regeneration. Exploration 1, 1. doi:10.1002/EXP.20210035
Grassin-Delyle, S., Buenestado, A., Naline, E., Faisy, C., Blouquit-Laye, S., Couderc, L.-J., et al. (2012). Intranasal Drug Delivery: An Efficient and Non-invasive Route for Systemic Administration. Pharmacol. Ther. 134 (3), 366–379. doi:10.1016/j.pharmthera.2012.03.003
Guo, D., Lou, C., Wang, N., Chen, M., Zhang, P., Wu, S., et al. (2017). Poly (Styrene-divinyl Benzene-Glycidylmethacrylate) Stationary Phase Grafted with Poly Amidoamine (PAMAM) Dendrimers for Rapid Determination of Phenylene Diamine Isomers in HPLC. Talanta 168, 188–195. doi:10.1016/j.talanta.2017.03.053
Hanada, T. (2014). The Discovery and Development of Perampanel for the Treatment of Epilepsy. Expert Opin. Drug Discov. 9 (4), 449–458. doi:10.1517/17460441.2014.891580
Harbi, I., Aljaeid, B., El-Say, K. M., and Zidan, A. S. (2016). Glycosylated Sertraline-Loaded Liposomes for Brain Targeting: QbD Study of Formulation Variabilities and Brain Transport. AAPS PharmSciTech 17 (6), 1404–1420. doi:10.1208/s12249-016-0481-7
Hawkins, B. T., and Davis, T. P. (2005). The Blood-Brain Barrier/neurovascular Unit in Health and Disease. Pharmacol. Rev. 57 (2), 173–185. doi:10.1124/pr.57.2.4
Hawthorne, G. H., Bernuci, M. P., Bortolanza, M., Tumas, V., Issy, A. C., and Del-Bel, E. (2016). Nanomedicine to Overcome Current Parkinson's Treatment Liabilities: A Systematic Review. Neurotox. Res. 30 (4), 715–729. doi:10.1007/s12640-016-9663-z
He, Q., Liu, J., Liang, J., Liu, X., Li, W., Liu, Z., et al. (2018). Towards Improvements for Penetrating the Blood-Brain Barrier-Recent Progress from a Material and Pharmaceutical Perspective. Cells 7 (4), 24. doi:10.3390/cells7040024
He, W., Zhang, Z., and Sha, X. (2021). Nanoparticles-mediated Emerging Approaches for Effective Treatment of Ischemic Stroke. Biomaterials 277, 121111. doi:10.1016/j.biomaterials.2021.121111
Heldt, T., Zoerle, T., Teichmann, D., and Stocchetti, N. (2019). Intracranial Pressure and Intracranial Elastance Monitoring in Neurocritical Care. Annu. Rev. Biomed. Eng. 21, 523–549. doi:10.1146/annurev-bioeng-060418-052257
Houng, A. K., Wang, D., and Reed, G. L. (2014). Reversing the Deleterious Effects of α2-antiplasmin on Tissue Plasminogen Activator Therapy Improves Outcomes in Experimental Ischemic Stroke. Exp. Neurol. 255, 56–62. doi:10.1016/j.expneurol.2014.02.009
Huang, K., Shi, B., Xu, W., Ding, J., Yang, Y., Liu, H., et al. (2015). Reduction-responsive Polypeptide Nanogel Delivers Antitumor Drug for Improved Efficacy and Safety. Acta Biomater. 27, 179–193. doi:10.1016/j.actbio.2015.08.049
Huo, T., Barth, R. F., Yang, W., Nakkula, R. J., Koynova, R., Tenchov, B., et al. (2012). Preparation, Biodistribution and Neurotoxicity of Liposomal Cisplatin Following Convection Enhanced Delivery in Normal and F98 Glioma Bearing Rats. PLoS One 7 (11), e48752. doi:10.1371/journal.pone.0048752
Jafari, S., Derakhshankhah, H., Alaei, L., Fattahi, A., Varnamkhasti, B. S., and Saboury, A. A. (2019). Mesoporous Silica Nanoparticles for Therapeutic/diagnostic Applications. Biomed. Pharmacother. 109, 1100–1111. doi:10.1016/j.biopha.2018.10.167
Jafarieh, O., Md, S., Ali, M., Baboota, S., Sahni, J. K., Kumari, B., et al. (2015). Design, Characterization, and Evaluation of Intranasal Delivery of Ropinirole-Loaded Mucoadhesive Nanoparticles for Brain Targeting. Drug Dev. Industrial Pharm. 41 (10), 1674–1681. doi:10.3109/03639045.2014.991400
Jain, K. K. (2020). An Overview of Drug Delivery Systems. Methods Mol. Biol. 2059, 1–54. doi:10.1007/978-1-4939-9798-5_1
Jain, S., Malinowski, M., Chopra, P., Varshney, V., and Deer, T. R. (2019). Intrathecal Drug Delivery for Pain Management: Recent Advances and Future Developments. Expert Opin. Drug Deliv. 16 (8), 815–822. doi:10.1080/17425247.2019.1642870
Javed, B., Zhao, X., Cui, D., Curtin, J., and Tian, F. (2021). Enhanced Anticancer Response of Curcumin- and Piperine-Loaded Lignin-G-P (NIPAM-Co-DMAEMA) Gold Nanogels against U-251 MG Glioblastoma Multiforme. Biomedicines 9 (11), 1516. doi:10.3390/biomedicines9111516
Jiang, Z., Dong, X., Yan, X., Liu, Y., Zhang, L., and Sun, Y. (2018). Nanogels of Dual Inhibitor-Modified Hyaluronic Acid Function as a Potent Inhibitor of Amyloid β-protein Aggregation and Cytotoxicity. Sci. Rep. 8 (1), 3505. doi:10.1038/s41598-018-21933-6
Jin, J., Bae, K. H., Yang, H., Lee, S. J., Kim, H., Kim, Y., et al. (2011). In Vivo specific Delivery of C-Met siRNA to Glioblastoma Using Cationic Solid Lipid Nanoparticles. Bioconjugate Chem. 22 (12), 2568–2572. doi:10.1021/bc200406n
Jogani, V., Jinturkar, K., Vyas, T., and Misra, A. (2008). Recent Patents Review on Intranasal Administration for CNS Drug Delivery. Recent Pat. Drug Deliv. Formul. 2 (1), 25–40. doi:10.2174/187221108783331429
Johanson, C., Stopa, E., McMillan, P., Roth, D., Funk, J., and Krinke, G. (2011). The Distributional Nexus of Choroid Plexus to Cerebrospinal Fluid, Ependyma and Brain. Toxicol. Pathol. 39 (1), 186–212. doi:10.1177/0192623310394214
Kadry, H., Noorani, B., and Cucullo, L. (2020). A Blood-Brain Barrier Overview on Structure, Function, Impairment, and Biomarkers of Integrity. Fluids Barriers CNS 17 (1), 69. doi:10.1186/s12987-020-00230-3
Kamaly, N., Xiao, Z., Valencia, P. M., Radovic-Moreno, A. F., and Farokhzad, O. C. (2012). Targeted Polymeric Therapeutic Nanoparticles: Design, Development and Clinical Translation. Chem. Soc. Rev. 41 (7), 2971–3010. doi:10.1039/c2cs15344k
Kazkayasi, I., Telli, G., Nemutlu, E., and Uma, S. (2022). Intranasal Metformin Treatment Ameliorates Cognitive Functions via Insulin Signaling Pathway in ICV-STZ-Induced Mice Model of Alzheimer's Disease. Life Sci. 299, 120538. doi:10.1016/j.lfs.2022.120538
Khan, A. R., Yang, X., Fu, M., and Zhai, G. (2018). Recent Progress of Drug Nanoformulations Targeting to Brain. J. Control. Release 291, 37–64. doi:10.1016/j.jconrel.2018.10.004
Khan, O., and Chaudary, N. (2020). The Use of Amikacin Liposome Inhalation Suspension (Arikayce) in the Treatment of Refractory Nontuberculous Mycobacterial Lung Disease in Adults. Dddt 14, 2287–2294. doi:10.2147/dddt.s146111
Kim, M., Kizilbash, S. H., Laramy, J. K., Gampa, G., Parrish, K. E., Sarkaria, J. N., et al. (2018). Barriers to Effective Drug Treatment for Brain Metastases: A Multifactorial Problem in the Delivery of Precision Medicine. Pharm. Res. 35 (9), 177. doi:10.1007/s11095-018-2455-9
Kleindorfer, D. O., Khatri, P., and Katzan, I. (2005). Reasons for Exclusion from Thrombolytic Therapy Following Acute Ischemic Stroke. Neurology 65 (11), 1844. doi:10.1212/01.wnl.0000200031.41939.ac
Kreyling, W. G., Hirn, S., Möller, W., Schleh, C., Wenk, A., Celik, G., et al. (2014). Air-blood Barrier Translocation of Tracheally Instilled Gold Nanoparticles Inversely Depends on Particle Size. ACS Nano 8 (1), 222–233. doi:10.1021/nn403256v
Kumar, A., Tan, A., Wong, J., Spagnoli, J. C., Lam, J., Blevins, B. D., et al. (2017). Nanotechnology for Neuroscience: Promising Approaches for Diagnostics, Therapeutics and Brain Activity Mapping. Adv. Funct. Mater 27 (39), 1700489. doi:10.1002/adfm.201700489
Kuo, Y.-C., and Shih-Huang, C.-Y. (2013). Solid Lipid Nanoparticles Carrying Chemotherapeutic Drug across the Blood-Brain Barrier through Insulin Receptor-Mediated Pathway. J. Drug Target. 21 (8), 730–738. doi:10.3109/1061186x.2013.812094
Lee, G., Dallas, S., Hong, M., and Bendayan, R. (2001). Drug Transporters in the Central Nervous System: Brain Barriers and Brain Parenchyma Considerations. Pharmacol. Rev. 53 (4), 569–596.
Lee, K., Kim, T., Kim, Y. M., Yang, K., Choi, I., and Roh, Y. H. (2021). Multifunctional DNA Nanogels for Aptamer-Based Targeted Delivery and Stimuli-Triggered Release of Cancer Therapeutics. Macromol. Rapid Commun. 42 (2), e2000457. doi:10.1002/marc.202000457
Leoni, V., and Caccia, C. (2014). Study of Cholesterol Metabolism in Huntington′s Disease. Biochem. Biophysical Res. Commun. 446 (3), 697–701. doi:10.1016/j.bbrc.2014.01.188
Li, D., Mastaglia, F. L., Fletcher, S., and Wilton, S. D. (2020). Progress in the Molecular Pathogenesis and Nucleic Acid Therapeutics for Parkinson's Disease in the Precision Medicine Era. Med. Res. Rev. 40 (6), 2650–2681. doi:10.1002/med.21718
Liu, C., Wen, J., Li, D., Qi, H., Nih, L., Zhu, J., et al. (2021). Systemic Delivery of microRNA for Treatment of Brain Ischemia. Nano Res. 14 (9), 3319–3328. doi:10.1007/s12274-021-3413-8
Liu, J., Liu, C., Zhang, J., Zhang, Y., Liu, K., Song, J.-X., et al. (2020). A Self-Assembled α-Synuclein Nanoscavenger for Parkinson's Disease. ACS Nano 14 (2), 1533–1549. doi:10.1021/acsnano.9b06453
Liu, Y., Li, D., Ding, J., and Chen, X. (2020). Controlled Synthesis of Polypeptides. Chin. Chem. Lett. 31 (12), 3001–3014. doi:10.1016/j.cclet.2020.04.029
Liu, Z., Qiao, J., Nagy, T., and Xiong, M. P. (2018). ROS-Triggered Degradable Iron-Chelating Nanogels: Safely Improving Iron Elimination In Vivo. J. Control. Release 283, 84–93. doi:10.1016/j.jconrel.2018.05.025
Logan, S., Arzua, T., Canfield, S. G., Seminary, E. R., Sison, S. L., Ebert, A. D., et al. (2019). Studying Human Neurological Disorders Using Induced Pluripotent Stem Cells: From 2D Monolayer to 3D Organoid and Blood Brain Barrier Models. Compr. Physiol. 9 (2), 565–611. doi:10.1002/cphy.c180025
Lombardo, D., Calandra, P., Pasqua, L., and Magazù, S. (2020). Self-assembly of Organic Nanomaterials and Biomaterials: The Bottom-Up Approach for Functional Nanostructures Formation and Advanced Applications. Mater. (Basel) 13 (5), 1048. doi:10.3390/ma13051048
Löscher, W., Potschka, H., Sisodiya, S. M., and Vezzani, A. (2020). Drug Resistance in Epilepsy: Clinical Impact, Potential Mechanisms, and New Innovative Treatment Options. Pharmacol. Rev. 72 (3), 606–638. doi:10.1124/pr.120.019539
Ma, Y., Ge, Y., and Li, L. (2017). Advancement of Multifunctional Hybrid Nanogel Systems: Construction and Application in Drug Co-delivery and Imaging Technique. Mater. Sci. Eng. C 71, 1281–1292. doi:10.1016/j.msec.2016.11.031
Mahajan, H. S., Mahajan, M. S., Nerkar, P. P., and Agrawal, A. (2014). Nanoemulsion-based Intranasal Drug Delivery System of Saquinavir Mesylate for Brain Targeting. Drug Deliv. 21 (2), 148–154. doi:10.3109/10717544.2013.838014
Mao, L., Wang, H., Tan, M., Ou, L., Kong, D., and Yang, Z. (2012). Conjugation of Two Complementary Anti-cancer Drugs Confers Molecular Hydrogels as a Co-delivery System. Chem. Commun. 48 (3), 395–397. doi:10.1039/c1cc16250k
Marçal, H., Ahmed, T., Badylak, S. F., Tottey, S., and Foster, L. J. R. (2012). A Comprehensive Protein Expression Profile of Extracellular Matrix Biomaterial Derived from Porcine Urinary Bladder. Regen. Med. 7 (2), 159–166. doi:10.2217/rme.12.6
Marí, M., Morales, A., Colell, A., García-Ruiz, C., and Fernández-Checa, J. C. (2009). Mitochondrial Glutathione, a Key Survival Antioxidant. Antioxidants Redox Signal. 11 (11), 2685–2700. doi:10.1089/ars.2009.2695
Mathew, A. P., Uthaman, S., Cho, K.-H., Cho, C.-S., and Park, I.-K. (2018). Injectable Hydrogels for Delivering Biotherapeutic Molecules. Int. J. Biol. Macromol. 110, 17–29. doi:10.1016/j.ijbiomac.2017.11.113
Mauri, E., Giannitelli, S. M., Trombetta, M., and Rainer, A. (2021). Synthesis of Nanogels: Current Trends and Future Outlook. Gels 7 (2), 36. doi:10.3390/gels7020036
McAteer, J. A., and Evan, A. P. (2008). The Acute and Long-Term Adverse Effects of Shock Wave Lithotripsy. Seminars Nephrol. 28 (2), 200–213. doi:10.1016/j.semnephrol.2008.01.003
McKee, A. C., and Robinson, M. E. (2014). Military-related Traumatic Brain Injury and Neurodegeneration. Alzheimers Dement. 10 (3 Suppl. l), S242–S253. doi:10.1016/j.jalz.2014.04.003
McNaught, K. S. P., Belizaire, R., Jenner, P., Olanow, C. W., and Isacson, O. (2002). Selective Loss of 20S Proteasome α-subunits in the Substantia Nigra Pars Compacta in Parkinson's Disease. Neurosci. Lett. 326 (3), 155–158. doi:10.1016/s0304-3940(02)00296-3
Meairs, S. (2015). Facilitation of Drug Transport across the Blood-Brain Barrier with Ultrasound and Microbubbles. Pharmaceutics 7 (3), 275–293. doi:10.3390/pharmaceutics7030275
Medina, S. H., and El-Sayed, M. E. H. (2009). Dendrimers as Carriers for Delivery of Chemotherapeutic Agents. Chem. Rev. 109 (7), 3141–3157. doi:10.1021/cr900174j
Meng, X.-y., Li, J.-j., Ni, T.-j., Xiao-tong, L., He, T., Men, Z.-n., et al. (2020). Electro-responsive Brain-Targeting Mixed Micelles Based on Pluronic F127 and D-α-Tocopherol Polyethylene Glycol Succinate–Ferrocene. Colloids Surfaces A Physicochem. Eng. Aspects 601, 124986. doi:10.1016/j.colsurfa.2020.124986
Merino, S., Martín, C., Kostarelos, K., Prato, M., and Vázquez, E. (2015). Nanocomposite Hydrogels: 3D Polymer-Nanoparticle Synergies for On-Demand Drug Delivery. ACS Nano 9 (5), 4686–4697. doi:10.1021/acsnano.5b01433
Mihalko, E. P., Nellenbach, K., Krishnakumar, M., Moiseiwitsch, N., Sollinger, J., Cooley, B. C., et al. (2022). Fibrin-specific poly(N-Isopropylacrylamide) Nanogels for Targeted Delivery of Tissue-type Plasminogen Activator to Treat Thrombotic Complications Are Well Tolerated In Vivo. Bioeng. Transl. Med. 7 (2), e10277. doi:10.1002/btm2.10277
Misra, A., Ganesh, S., Shahiwala, A., and Shah, S. P. (2003). Drug Delivery to the Central Nervous System: a Review. J. Pharm. Pharm. Sci. 6 (2), 252–273.
Molino, Y., Jabès, F., Lacassagne, E., Gaudin, N., and Khrestchatisky, M. (2014). Setting-up an In Vitro Model of Rat Blood-Brain Barrier (BBB): a Focus on BBB Impermeability and Receptor-Mediated Transport. J. Vis. Exp. 88, e51278. doi:10.3791/51278
Muresanu, D. F., Strilciuc, S., and Stan, A. (2019). Current Drug Treatment of Acute Ischemic Stroke: Challenges and Opportunities. CNS Drugs 33 (9), 841–847. doi:10.1007/s40263-019-00663-x
Nance, E., Zhang, C., Shih, T.-Y., Xu, Q., Schuster, B. S., and Hanes, J. (2014). Brain-penetrating Nanoparticles Improve Paclitaxel Efficacy in Malignant Glioma Following Local Administration. ACS Nano 8 (10), 10655–10664. doi:10.1021/nn504210g
Nau, R., Sörgel, F., and Eiffert, H. (2021). Central Nervous System Infections and Antimicrobial Resistance: an Evolving Challenge. Curr. Opin. Neurol. 34 (3), 456–467. doi:10.1097/wco.0000000000000931
Neamtu, I., Rusu, A. G., Diaconu, A., Nita, L. E., and Chiriac, A. P. (2017). Basic Concepts and Recent Advances in Nanogels as Carriers for Medical Applications. Drug Deliv. 24 (1), 539–557. doi:10.1080/10717544.2016.1276232
Oberdörster, G. (2010). Safety Assessment for Nanotechnology and Nanomedicine: Concepts of Nanotoxicology. J. Intern Med. 267 (1), 89–105. doi:10.1111/j.1365-2796.2009.02187.x
Oh, J. K., Siegwart, D. J., and Matyjaszewski, K. (2007). Synthesis and Biodegradation of Nanogels as Delivery Carriers for Carbohydrate Drugs. Biomacromolecules 8 (11), 3326–3331. doi:10.1021/bm070381+
Palmer, A. M. (2010). The Role of the Blood-CNS Barrier in CNS Disorders and Their Treatment. Neurobiol. Dis. 37 (1), 3–12. doi:10.1016/j.nbd.2009.07.029
Pappu, S., Lerma, J., and Khraishi, T. (2016). Brain CT to Assess Intracranial Pressure in Patients with Traumatic Brain Injury. J. Neuroimaging 26 (1), 37–40. doi:10.1111/jon.12289
Pardridge, W. M. (2003). Blood-brain Barrier Drug Targeting: the Future of Brain Drug Development. Mol. Interv. 3 (2), 9051–9105. doi:10.1124/mi.3.2.90
Pardridge, W. M. (2005). The Blood-Brain Barrier: Bottleneck in Brain Drug Development. Neurotherapeutics 2 (1), 3–14. doi:10.1602/neurorx.2.1.3
Pargoo, E. M., Aghasadeghi, M. R., Parivar, K., Nikbin, M., Rahimi, P., and Ardestani, M. S. (2021). Lamivudine‐conjugated and Efavirenz‐loaded G2 Dendrimers: Novel Anti‐retroviral Nano Drug Delivery Systems. IET Nanobiotechnol. 15 (7), 627–637. doi:10.1049/nbt2.12060
Pastor-Maldonado, C. J., Suárez-Rivero, J. M., Povea-Cabello, S., Álvarez-Córdoba, M., Villalón-García, I., Munuera-Cabeza, M., et al. (2020). Coenzyme Q10: Novel Formulations and Medical Trends. Int. J. Mol. Sci. 21 (22), 8432. doi:10.3390/ijms21228432
Patel, V., Chavda, V., and Shah, J. (2021). Nanotherapeutics in Neuropathologies: Obstacles, Challenges and Recent Advancements in CNS Targeted Drug Delivery Systems. Curr. Neuropharmacol. 19 (5), 693–710. doi:10.2174/1570159x18666200807143526
Peng, S., Wang, H., Zhao, W., Xin, Y., Liu, Y., Yu, X., et al. (2020). Zwitterionic Polysulfamide Drug Nanogels with Microwave Augmented Tumor Accumulation and On‐Demand Drug Release for Enhanced Cancer Therapy. Adv. Funct. Mater. 30 (23), 1. doi:10.1002/adfm.202001832
Pichla, M., Bartosz, G., and Sadowska-Bartosz, I. (2020). The Antiaggregative and Antiamyloidogenic Properties of Nanoparticles: A Promising Tool for the Treatment and Diagnostics of Neurodegenerative Diseases. Oxid. Med. Cell Longev. 2020, 3534570. doi:10.1155/2020/3534570
Picone, P., Sabatino, M. A., Ditta, L. A., Amato, A., San Biagio, P. L., Mulè, F., et al. (2018). Nose-to-brain Delivery of Insulin Enhanced by a Nanogel Carrier. J. Control. Release 270, 23–36. doi:10.1016/j.jconrel.2017.11.040
Poovaiah, N., Davoudi, Z., Peng, H., Schlichtmann, B., Mallapragada, S., Narasimhan, B., et al. (2018). Treatment of Neurodegenerative Disorders through the Blood-Brain Barrier Using Nanocarriers. Nanoscale 10 (36), 16962–16983. doi:10.1039/c8nr04073g
Pottoo, F. H., Sharma, S., Javed, M. N., Barkat, M. A., Harshita, , Alam, M. S., et al. (2020). Lipid-based Nanoformulations in the Treatment of Neurological Disorders. Drug Metab. Rev. 52 (1), 185–204. doi:10.1080/03602532.2020.1726942
Pourtalebi Jahromi, L., Moghaddam Panah, F., Azadi, A., and Ashrafi, H. (2019). A Mechanistic Investigation on Methotrexate-Loaded Chitosan-Based Hydrogel Nanoparticles Intended for CNS Drug Delivery: Trojan Horse Effect or Not? Int. J. Biol. Macromol. 125, 785–790. doi:10.1016/j.ijbiomac.2018.12.093
Pourtalebi Jahromi, L., Mohammadi-Samani, S., Heidari, R., and Azadi, A. (2018). In Vitro- and In Vivo Evaluation of Methotrexate-Loaded Hydrogel Nanoparticles Intended to Treat Primary CNS Lymphoma via Intranasal Administration. J. Pharm. Pharm. Sci. 21 (1), 305–317. doi:10.18433/jpps29496
Prasanna, P., and Upadhyay, A. (2021). Flavonoid-Based Nanomedicines in Alzheimer's Disease Therapeutics: Promises Made, a Long Way to Go. ACS Pharmacol. Transl. Sci. 4 (1), 74–95. doi:10.1021/acsptsci.0c00224
Rizzi, L., Rosset, I., and Roriz-Cruz, M. (2014). Global Epidemiology of Dementia: Alzheimer's and Vascular Types. Biomed. Res. Int. 2014, 908915. doi:10.1155/2014/908915
Sá-Pereira, I., Brites, D., and Brito, M. A. (2012). Neurovascular Unit: a Focus on Pericytes. Mol. Neurobiol. 45 (2), 327–347. doi:10.1007/s12035-012-8244-2
Saha, P., Ganguly, R., Li, X., Das, R., Singha, N. K., and Pich, A. (2021). Zwitterionic Nanogels and Microgels: An Overview on Their Synthesis and Applications. Macromol. Rapid Commun. 42 (13), e2100112. doi:10.1002/marc.202100112
Sari, N. D., Baltali, S., Serin, I., and Antar, V. (2021). Evaluation of Intraventricular/Intrathecal Antimicrobial Therapy in the Treatment of Nosocomial Meningitis Caused by Multidrug-Resistant Gram-Negative Bacteria after Central Nervous System Surgery. Can. J. Infect. Dis. Med. Microbiol. 2021, 9923015. doi:10.1155/2021/9923015
Sarkar, A., Fatima, I., Mohammad Sajid Jamal, Q., Sayeed, U., Kalim A. Khan, M., Akhtar, S., et al. (2017). Nanoparticles as a Carrier System for Drug Delivery across Blood Brain Barrier. Cdm 18 (2), 129–137. doi:10.2174/1389200218666170113125132
Shi, B., Huang, K., Ding, J., Xu, W., Yang, Y., Liu, H., et al. (2017). Intracellularly Swollen Polypeptide Nanogel Assists Hepatoma Chemotherapy. Theranostics 7 (3), 703–716. doi:10.7150/thno.16794
Shringarpure, M., Gharat, S., Momin, M., and Omri, A. (2021). Management of Epileptic Disorders Using Nanotechnology-Based Strategies for Nose-To-Brain Drug Delivery. Expert Opin. Drug Deliv. 18 (2), 169–185. doi:10.1080/17425247.2021.1823965
Soni, K. S., Desale, S. S., and Bronich, T. K. (2016). Nanogels: An Overview of Properties, Biomedical Applications and Obstacles to Clinical Translation. J. Control. Release 240, 109–126. doi:10.1016/j.jconrel.2015.11.009
Soni, S., Ruhela, R. K., and Medhi, B. (2016). Nanomedicine in Central Nervous System (CNS) Disorders: A Present and Future Prospective. Adv. Pharm. Bull. 6 (3), 319–335. doi:10.15171/apb.2016.044
Soni, V., Jain, A., Khare, P., Gulbake, A., and Jain, S. (2010). Potential Approaches for Drug Delivery to the Brain: Past, Present, and Future. Crit. Rev. Ther. Drug Carr. Syst. 27 (3), 187–236. doi:10.1615/critrevtherdrugcarriersyst.v27.i3.10
Srikanth, M., and Kessler, J. A. (2012). Nanotechnology-novel Therapeutics for CNS Disorders. Nat. Rev. Neurol. 8 (6), 307–318. doi:10.1038/nrneurol.2012.76
Stawicki, B., Schacher, T., and Cho, H. (2021). Nanogels as a Versatile Drug Delivery System for Brain Cancer. Gels 7 (2), 63. doi:10.3390/gels7020063
Stuart, M. A. C., Huck, W. T. S., Genzer, J., Müller, M., Ober, C., Stamm, M., et al. (2010). Emerging Applications of Stimuli-Responsive Polymer Materials. Nat. Mater 9 (2), 101–113. doi:10.1038/nmat2614
Suhail, M., Rosenholm, J. M., Minhas, M. U., Badshah, S. F., Naeem, A., Khan, K. U., et al. (2019). Nanogels as Drug-Delivery Systems: a Comprehensive Overview. Ther. Deliv. 10 (11), 697–717. doi:10.4155/tde-2019-0010
Sun, M., Su, X., Ding, B., He, X., Liu, X., Yu, A., et al. (2012). Advances in Nanotechnology-Based Delivery Systems for Curcumin. Nanomedicine 7 (7), 1085–1100. doi:10.2217/nnm.12.80
Sun, T., Zhang, Y. S., Pang, B., Hyun, D. C., Yang, M., and Xia, Y. (2014). Engineered Nanoparticles for Drug Delivery in Cancer Therapy. Angew. Chem. Int. Ed. Engl. 53 (46), 12320–12364. doi:10.1002/anie.201403036
Tang, L., Zhang, M., and Liu, C. (2022). Advances in Nanotechnology-Based Immunotherapy for Glioblastoma. Front. Immunol. 13, 882257. doi:10.3389/fimmu.2022.882257
Tangpong, J., Cole, M. P., Sultana, R., Estus, S., Vore, M., St. Clair, W., et al. (2007). Adriamycin-mediated Nitration of Manganese Superoxide Dismutase in the Central Nervous System: Insight into the Mechanism of Chemobrain. J. Neurochem. 100 (1), 191–201. doi:10.1111/j.1471-4159.2006.04179.x
Tedford, C. E., DeLapp, S., Jacques, S., and Anders, J. (2015). Quantitative Analysis of Transcranial and Intraparenchymal Light Penetration in Human Cadaver Brain Tissue. Lasers Surg. Med. 47 (4), 312–322. doi:10.1002/lsm.22343
Teng, Y., Jin, H., Nan, D., Li, M., Fan, C., Liu, Y., et al. (2018). In Vivo evaluation of Urokinase-Loaded Hollow Nanogels for Sonothrombolysis on Suture Embolization-Induced Acute Ischemic Stroke Rat Model. Bioact. Mater. 3 (1), 102–109. doi:10.1016/j.bioactmat.2017.08.001
Tian, X. H., Lin, X. N., Wei, F., Feng, W., Huang, Z. C., Wang, P., et al. (2011). Enhanced Brain Targeting of Temozolomide in Polysorbate-80 Coated Polybutylcyanoacrylate Nanoparticles. Int. J. Nanomedicine 6, 445–452. doi:10.2147/IJN.S16570
Tietz, S., and Engelhardt, B. (2015). Brain Barriers: Crosstalk between Complex Tight Junctions and Adherens Junctions. J. Cell Biol. 209 (4), 493–506. doi:10.1083/jcb.201412147
Trompetero, A., Gordillo, A., Del Pilar, M. C., Cristina, V. M., and Bustos Cruz, R. H. (2018). Alzheimer's Disease and Parkinson's Disease: A Review of Current Treatment Adopting a Nanotechnology Approach. Cpd 24 (1), 22–45. doi:10.2174/1381612823666170828133059
T. Ronaldson, P., and P. Davis, T. (2012). Blood-brain Barrier Integrity and Glial Support: Mechanisms that Can Be Targeted for Novel Therapeutic Approaches in Stroke. Curr. Pharm. Des. 18 (25), 3624–3644. doi:10.2174/138161212802002625
Ueno, M., Nakagawa, T., Wu, B., Onodera, M., Huang, C.-l., Kusaka, T., et al. (2010). Transporters in the Brain Endothelial Barrier. Cmc 17 (12), 1125–1138. doi:10.2174/092986710790827816
Verma, S., Domb, A. J., and Kumar, N. (2011). Nanomaterials for Regenerative Medicine. Nanomedicine 6 (1), 157–181. doi:10.2217/nnm.10.146
Vigani, B., Rossi, S., Sandri, G., Bonferoni, M. C., Caramella, C. M., and Ferrari, F. (2020). Recent Advances in the Development of In Situ Gelling Drug Delivery Systems for Non-parenteral Administration Routes. Pharmaceutics 12 (9), 859. doi:10.3390/pharmaceutics12090859
Vinogradov, S. V., Batrakova, E. V., and Kabanov, A. V. (2004). Nanogels for Oligonucleotide Delivery to the Brain. Bioconjugate Chem. 15 (1), 50–60. doi:10.1021/bc034164r
Vinogradov, S. V., Bronich, T. K., and Kabanov, A. V. (2002). Nanosized Cationic Hydrogels for Drug Delivery: Preparation, Properties and Interactions with Cells. Adv. Drug Deliv. Rev. 54 (1), 135–147. doi:10.1016/s0169-409x(01)00245-9
Vinogradov, S. V. (2010). Nanogels in the Race for Drug Delivery. Nanomedicine 5 (2), 165–168. doi:10.2217/nnm.09.103
Vinogradov, S. V., Poluektova, L. Y., Makarov, E., Gerson, T., and Senanayake, M. T. (2010). Nano-NRTIs: Efficient Inhibitors of HIV Type-1 in Macrophages with a Reduced Mitochondrial Toxicity. Antivir. Chem. Chemother. 21 (1), 1–14. doi:10.3851/imp1680
Wang, D., and Wu, L.-P. (2017). Nanomaterials for Delivery of Nucleic Acid to the Central Nervous System (CNS). Mater. Sci. Eng. C 70 (Pt 2), 1039–1046. doi:10.1016/j.msec.2016.04.011
Wang, J., Ni, Q., Wang, Y., Zhang, Y., He, H., Gao, D., et al. (2021). Nanoscale Drug Delivery Systems for Controllable Drug Behaviors by Multi-Stage Barrier Penetration. J. Control. Release 331, 282–295. doi:10.1016/j.jconrel.2020.08.045
Wang, L., Lian, W., Liu, B., Lv, H., Zhang, Y., Wu, X., et al. (2022). Transparent, High-Performance and Stable Sb2 S3 Photoanode Enabled by Heterojunction Engineering with Conjugated Polycarbazole Frameworks for Unbiased Photoelectrochemical Overall Water Splitting Devices. Adv. Mater 1, e2200723. doi:10.1002/adma.202200723
Wang, X., Parvathaneni, V., Shukla, S. K., Kulkarni, N. S., Muth, A., Kunda, N. K., et al. (2020). Inhalable Resveratrol-Cyclodextrin Complex Loaded Biodegradable Nanoparticles for Enhanced Efficacy against Non-small Cell Lung Cancer. Int. J. Biol. Macromol. 164, 638–650. doi:10.1016/j.ijbiomac.2020.07.124
Wang, Y.-Y., Lui, P. C., and Li, J. Y. (2009). Receptor-mediated Therapeutic Transport across the Blood-Brain Barrier. Immunotherapy 1 (6), 983–993. doi:10.2217/imt.09.75
Wang, Y., Ying, X., Chen, L., Liu, Y., Wang, Y., Liang, J., et al. (2016). Electroresponsive Nanoparticles Improve Antiseizure Effect of Phenytoin in Generalized Tonic-Clonic Seizures. Neurotherapeutics 13 (3), 603–613. doi:10.1007/s13311-016-0431-9
Warren, G., Makarov, E., Lu, Y., Senanayake, T., Rivera, K., Gorantla, S., et al. (2015). Amphiphilic Cationic Nanogels as Brain-Targeted Carriers for Activated Nucleoside Reverse Transcriptase Inhibitors. J. Neuroimmune Pharmacol. 10 (1), 88–101. doi:10.1007/s11481-014-9576-7
Wei, P., Cornel, E. J., and Du, J. (2021). Ultrasound-responsive Polymer-Based Drug Delivery Systems. Drug Deliv. Transl. Res. 11 (4), 1323–1339. doi:10.1007/s13346-021-00963-0
Wen, J., Wu, D., Qin, M., Liu, C., Wang, L., Xu, D., et al. (2019). Sustained Delivery and Molecular Targeting of a Therapeutic Monoclonal Antibody to Metastases in the Central Nervous System of Mice. Nat. Biomed. Eng. 3 (9), 706–716. doi:10.1038/s41551-019-0434-z
Wilson, L., Stewart, W., Dams-O'Connor, K., Diaz-Arrastia, R., Horton, L., Menon, D. K., et al. (2017). The Chronic and Evolving Neurological Consequences of Traumatic Brain Injury. Lancet Neurology 16 (10), 813–825. doi:10.1016/s1474-4422(17)30279-x
Wohlfart, S., Gelperina, S., and Kreuter, J. (2012). Transport of Drugs across the Blood-Brain Barrier by Nanoparticles. J. Control. Release 161 (2), 264–273. doi:10.1016/j.jconrel.2011.08.017
Wong, H. L., Wu, X. Y., and Bendayan, R. (2012). Nanotechnological Advances for the Delivery of CNS Therapeutics. Adv. Drug Deliv. Rev. 64 (7), 686–700. doi:10.1016/j.addr.2011.10.007
Wu, T., and Tang, M. (2018). The Inflammatory Response to Silver and Titanium Dioxide Nanoparticles in the Central Nervous System. Nanomedicine 13 (2), 233–249. doi:10.2217/nnm-2017-0270
Xiao, Y., Liu, F., Yang, J., Zhong, M., Zhang, E., Li, Y., et al. (2015). Over-activation of TLR5 Signaling by High-Dose Flagellin Induces Liver Injury in Mice. Cell Mol. Immunol. 12 (6), 729–742. doi:10.1038/cmi.2014.110
Xu, C., Gong, Y., Wang, Y., and Chen, Z. (2022). New Advances in Pharmacoresistant Epilepsy towards Precise Management-From Prognosis to Treatments. Pharmacol. Ther. 233, 108026. doi:10.1016/j.pharmthera.2021.108026
Xu, D., Wu, D., Qin, M., Nih, L. R., Liu, C., Cao, Z., et al. (2019). Efficient Delivery of Nerve Growth Factors to the Central Nervous System for Neural Regeneration. Adv. Mater 31 (33), e1900727. doi:10.1002/adma.201900727
Yang, J., Wang, L., Huang, L., Che, X., Zhang, Z., Wang, C., et al. (2021). Receptor‐targeting Nanomaterials Alleviate Binge Drinking‐induced Neurodegeneration as Artificial Neurotrophins. Exploration 1 (1), 61–74. doi:10.1002/exp.20210004
Yin, L., Ding, J., Fei, L., He, M., Cui, F., Tang, C., et al. (2008). Beneficial Properties for Insulin Absorption Using Superporous Hydrogel Containing Interpenetrating Polymer Network as Oral Delivery Vehicles. Int. J. Pharm. 350 (1-2), 220–229. doi:10.1016/j.ijpharm.2007.08.051
Ying, X., Wang, Y., Liang, J., Yue, J., Xu, C., Lu, L., et al. (2014). Angiopep-conjugated Electro-Responsive Hydrogel Nanoparticles: Therapeutic Potential for Epilepsy. Angew. Chem. Int. Ed. Engl. 53 (46), 12436–12440. doi:10.1002/anie.201403846
Zain-ul-Abdin, A., Wang, L., Yu, H., Saleem, M., Akram, M., Khalid, H., et al. (2017). Synthesis of Ethylene Diamine-Based Ferrocene Terminated Dendrimers and Their Application as Burning Rate Catalysts. J. Colloid Interface Sci. 487, 38–51. doi:10.1016/j.jcis.2016.10.001
Zatta, P., Drago, D., Bolognin, S., and Sensi, S. L. (2009). Alzheimer's Disease, Metal Ions and Metal Homeostatic Therapy. Trends Pharmacol. Sci. 30 (7), 346–355. doi:10.1016/j.tips.2009.05.002
Zhang, C., Pan, D., Luo, K., She, W., Guo, C., Yang, Y., et al. (2014). Peptide Dendrimer-Doxorubicin Conjugate-Based Nanoparticles as an Enzyme-Responsive Drug Delivery System for Cancer Therapy. Adv. Healthc. Mat. 3 (8), 1299–1308. doi:10.1002/adhm.201300601
Zhang, G., Zhang, L., Rao, H., Wang, Y., Li, Q., Qi, W., et al. (2020). Role of Molecular Chirality and Solvents in Directing the Self-Assembly of Peptide into an Ultra-pH-sensitive Hydrogel. J. Colloid Interface Sci. 577, 388–396. doi:10.1016/j.jcis.2020.05.087
Zhang, L., Zhang, F., Weng, Z., Brown, B. N., Yan, H., Ma, X. M., et al. (2013). Effect of an Inductive Hydrogel Composed of Urinary Bladder Matrix upon Functional Recovery Following Traumatic Brain Injury. Tissue Eng. Part A 19 (17-18), 1909–1918. doi:10.1089/ten.TEA.2012.0622
Zhang, M., Asghar, S., Tian, C., Hu, Z., Ping, Q., Chen, Z., et al. (2021). Lactoferrin/phenylboronic Acid-Functionalized Hyaluronic Acid Nanogels Loading Doxorubicin Hydrochloride for Targeting Glioma. Carbohydr. Polym. 253, 117194. doi:10.1016/j.carbpol.2020.117194
Zhang, P., Hu, L., Yin, Q., Zhang, Z., Feng, L., and Li, Y. (2012). Transferrin-conjugated Polyphosphoester Hybrid Micelle Loading Paclitaxel for Brain-Targeting Delivery: Synthesis, Preparation and In Vivo Evaluation. J. Control. Release 159 (3), 429–434. doi:10.1016/j.jconrel.2012.01.031
Zhang, Y., Yang, H., Wei, D., Zhang, X., Wang, J., Wu, X., et al. (2021). Mitochondria‐targeted Nanoparticles in Treatment of Neurodegenerative Diseases. Exploration 1 (3), 1. doi:10.1002/exp.20210115
Zhang, Z., Ji, Y., Lin, C., and Tao, L. (2021). Thermosensitive Hydrogel-Functionalized Gold Nanorod/mesoporous MnO2 Nanoparticles for Tumor Cell-Triggered Drug Delivery. Mater. Sci. Eng. C 131, 112504. doi:10.1016/j.msec.2021.112504
Zhao, F., Yao, D., Guo, R., Deng, L., Dong, A., and Zhang, J. (2015). Composites of Polymer Hydrogels and Nanoparticulate Systems for Biomedical and Pharmaceutical Applications. Nanomaterials 5 (4), 2054–2130. doi:10.3390/nano5042054
Zhao, Q., Zhang, S., Wu, F., Li, D., Zhang, X., Chen, W., et al. (2021). Rational Design of Nanogels for Overcoming the Biological Barriers in Various Administration Routes. Angew. Chem. Int. Ed. 60 (27), 14760–14778. doi:10.1002/anie.201911048
Keywords: nanotechnology, nanogel, CNS diseases, blood-brain barrier, smart drug release
Citation: Zhang Y, Zou Z, Liu S, Miao S and Liu H (2022) Nanogels as Novel Nanocarrier Systems for Efficient Delivery of CNS Therapeutics. Front. Bioeng. Biotechnol. 10:954470. doi: 10.3389/fbioe.2022.954470
Received: 27 May 2022; Accepted: 20 June 2022;
Published: 19 July 2022.
Edited by:
Zhanzhan Zhang, Tianjin Medical University, ChinaReviewed by:
Meng Zheng, Henan University, ChinaChaoyong Liu, Beijing University of Chemical Technology, China
Xiuli Zhuang, Changchun Institute of Applied Chemistry (CAS), China
Copyright © 2022 Zhang, Zou, Liu, Miao and Liu. This is an open-access article distributed under the terms of the Creative Commons Attribution License (CC BY). The use, distribution or reproduction in other forums is permitted, provided the original author(s) and the copyright owner(s) are credited and that the original publication in this journal is cited, in accordance with accepted academic practice. No use, distribution or reproduction is permitted which does not comply with these terms.
*Correspondence: Haiyan Liu, aGFpeWFuQGpsdS5lZHUuY24=