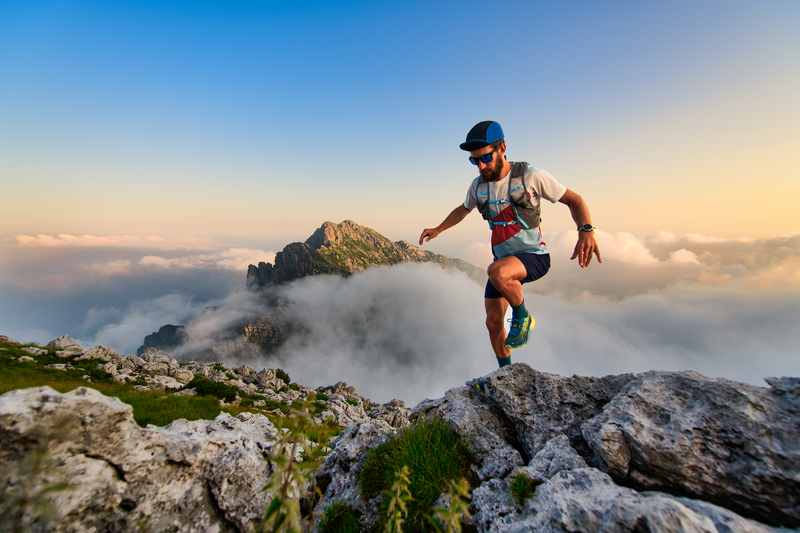
95% of researchers rate our articles as excellent or good
Learn more about the work of our research integrity team to safeguard the quality of each article we publish.
Find out more
ORIGINAL RESEARCH article
Front. Bioeng. Biotechnol. , 18 July 2022
Sec. Synthetic Biology
Volume 10 - 2022 | https://doi.org/10.3389/fbioe.2022.946117
This article is part of the Research Topic Exploration of Highly Active Enzymes, Performance Enhancement and Enzymatic Processing Techniques View all 7 articles
Candida rugosa lipase (CRL) was activated with surfactants (sodium dodecyl sulfate [SDS]) and covalently immobilized onto a nanocomposite (Fe3O4-CS-DAC) fabricated by combining magnetic nanoparticles Fe3O4 with chitosan (CS) using polysaccharide macromolecule dialdehyde cellulose (DAC) as the cross-linking agent. Fourier transform infrared spectroscopy, transmission electron microscope, thermogravimetric analysis, and X-ray diffraction characterizations confirmed that the organic–inorganic nanocomposite support modified by DAC was successfully prepared. Enzymology experiments confirmed that high enzyme loading (60.9 mg/g) and 1.7 times specific enzyme activity could be obtained under the optimal immobilization conditions. The stability and reusability of immobilized CRL (Fe3O4-CS-DAC-SDS-CRL) were significantly improved simultaneously. Circular dichroism analysis revealed that the active conformation of immobilized CRL was maintained well. Results demonstrated that the inorganic–organic nanocomposite modified by carbohydrate polymer derivatives could be used as an ideal support for enzyme immobilization.
Enzymes are a class of biological macromolecules with catalytic activity and specific spatial conformation in organisms, and they are widely used in chemical industry, medicine, food, and energy (Ismail et al., 2021; Sherawat et al., 2021). Candida rugosa lipase (CRL) is a lipase with important application value, which has high activity and specificity and can be widely used in chemical, cosmetic, food, and other applications (Barriuso et al., 2016; Vanleeuw et al., 2019). However, free CRL is very susceptible to external conditions and has the disadvantages of poor stability and difficult to reuse, which limit the application of CRL in large-scale production.
Immobilization can improve the operational stabilization and activity of enzymes and sometimes can even modulate the selectivity of enzymes by immobilization on functional supports (Mateo et al., 2007; Rodrigues et al., 2011; Barbosa et al., 2013). At the same time, the immobilized enzymes can be easily separated from products, which reduce the protein contamination in the product and are conducive to the continuity of production (Xiang et al., 2017; Liang et al., 2020). In recent years, organic–inorganic composites based on Fe3O4 and natural organic polymers with the characteristics of excellent biocompatibility, high mechanical stability, and good recyclability have received more and more attention and obtained good applications in enzyme immobilization (Faraji et al., 2021; Matveeva et al., 2021). Chitosan (CS) is an inexpensive and available natural polysaccharide. Owing to its excellent biocompatibility, nontoxicity, and good biodegradability, CS has been valued in the research of modification of supports for enzyme immobilization (Nunes et al., 2021). Wang et al. (2015) fabricated composites (GO-CS-Fe3O4) that consisted of magnetic graphene oxide–chitosan (GO-CS) and magnetite nanoparticles (Fe3O4) and that was activated with glutaraldehyde (GA) to immobilize CRL. The immobilized CRL exhibited excellent properties such as improved stability, pH, and temperature tolerance. However, the activity recovery was only 60.2% with a moderate enzyme loading obtained (48.5 mg/g). Ziegler-Borowska et al. (2017) coated CS and rat tail collagen onto the surface of magnetic nanoparticles Fe3O4, and then the support was condensed with CRL by the function of EDC/NHS to prepare the immobilized CRL. The activity recovery rate of the immobilized CRL was as high as 112.0%, and the thermal stability was also enhanced, while the support had a low enzyme loading (27.0 mg/g). Hence, organic–inorganic nanocomposites are still challenging to greatly improve the enzymatic properties of activity, stability, and reusability and obtain high enzyme loading at the same time.
In general, GA is widely used to immobilize enzymes by way of covalent cross-linking. However, this immobilization process is relatively violent, and its high toxicity often results in the loss of enzyme activity (Leung et al., 2001). Starch and cellulose are the most common natural polysaccharides, which are inexpensive, available, and biocompatible. Dialdehyde starch (DAS) and dialdehyde cellulose (DAC) are easy to be prepared by selective oxidation of starch and cellulose. Owing to the abundant aldehyde and hydroxyl functional groups, DAS and DAC have been successfully used as new efficient covalent cross-linking reagents in the process of enzyme immobilization (Sharma et al., 2014; Ruan et al., 2018; Tamaddon et al., 2019). Qiao et al. (2022) prepared magnetic dialdehyde cellulose and used it for the immobilization of bacterial laccase. The pH and inhibitor tolerance, activity, and stability of immobilized laccase were obviously improved. In recent times, a study from our group has verified that DAS could be firmly combined with laccase onto Fe3O4 modified by amino-functionalized ionic liquid to stabilize its three-dimensional conformation; compared with those cross-linked by GA, the enzymatic properties of laccase were improved by a larger extent (Qiu et al., 2021).
The active center of lipase is usually covered by a “lid” structure (Verger et al., 1997; Schmid et al., 1998). In addition to the strategy of immobilizing lipase on a hydrophobic carrier to open its lid structure and stabilize its active conformation, addition of surfactants is also a commonly used method to activate lipase by stabilization of the open form of lipase (Lopez et al., 2002; Manoel et al., 2015; De Oliveira et al., 2018). Calvo et al. (1996) studied the effect of SDS and Triton X-100 surfactants on the hydrolysis activity of isoenzymes (CRL-A and CRL-B). Compared with nonionic-type surfactant Triton X-100, anionic surfactant SDS showed less toxic effects and had better stability to lipases (Calvo et al., 1996). Betancor et al. (2006) found that the addition of surfactants could further improve the enzymatic properties of CRL covalently immobilized on aminated Fe3O4 support by restraining the aggregation of lipase and decreasing the formation of dimer of lipase to maintain enzymatic activity. In the previous research of our laboratory, surfactants improved the activity and stability of CRL encapsulated in sol–gel materials, and the interface-activated immobilized CRL was successfully applied to catalyze the synthesis of vitamin E succinate (Hu et al., 2013).
Herein, this study intends to expand the support design and enzyme immobilization methods developed by our research group in the early stage, using biological macromolecule DAC as the cross-linking agent and surfactant as the interface activator to prepare an immobilized CRL based on magnetic CS nanocomposites. Under the premise of high enzyme loading, the activity, stability, and reusability of the enzymes will be greatly improved; thus, a new method of highly efficient enzyme immobilization will be developed.
CRL (500.0 U/g) was purchased from Sigma-Aldrich; FeCl3·6H2O (99.0%), FeCl2·4H2O (99.0%), microcrystalline cellulose (>90 um), chitosan (78% degree of deacetylation), sodium dodecyl sulfate (>88.0%), glutaraldehyde (70% in water), sodium periodate (>98%), Triton X-100 (>98.0%), and Tween-80 (AR) were purchased from Sinopharm Chemical Reagent Co., Ltd. Other chemicals were of analytical grade.
Transmission electron microscopy (TEM) was carried out on a Tecnai G2 F20 (USA) and Talos F200x (USA). Fourier transform infrared (FT-IR) spectroscopy analysis was carried out using a Nicolet iS5 FT-IR spectrometer (Thermo Nicolet IS5, USA) in a wavelength range of 450–4,000 cm−1. Thermogravimetric analysis was conducted on a TG209F3 instrument by heating from 20 to 800°C under N2 with a heating rate of 20°C/min. X-ray diffraction (XRD) analysis was performed on a Bruker D8 Advance instrument (Germany). The circular dichroism characterization was performed on the J-1500CD spectrometer (Japan), the scanning speed was 30 nm/min, and the wavelength range was 190–260 nm.
The synthesis of Fe3O4 nanoparticles was referred to the previously reported literature with a slight modification (Suo et al., 2018). The specific process was as follows: FeCl3·6H2O (5.5 g) and FeCl2·4H2O (2.1 g) were dissolved in purified water (100 ml) under magnetic stirring, and the reaction solution was heated to 70°C under nitrogen atmosphere. Then, ammonium hydroxide (28%) was used to adjust the pH until the solution was reddish brown, and the temperature was raised to 80°C for 1.5 h. At last, Fe3O4 nanoparticles were separated by a magnet after cooling to room temperature and washed with purified water until neutral.
DAC was prepared using a method similar to the conventional procedure (Varma et al., 2002). First, 150 ml of distilled water was added to a light-proof round bottom flask, and 1% hydrochloric acid was used to adjust the pH to 3. Then cellulose (3.0 g) and sodium periodate (6.0 g) were added into the flask and stirred at 35°C for 3 h. Next, 2 ml ethylene glycol was added into the flask and kept for 2 h to remove excess sodium periodate. At last, the resulting suspension was suction filtered to obtain a filter cake. The filter cake (DAC) was washed with distilled water several times and dried at 50°C.
The synthesis of supports Fe3O4-CS, Fe3O4-CS-GA, and Fe3O4-CS-DAC were referred to the previously reported literature with a minor modification (Kim et al., 2017; Zhao et al., 2018).
First, CS (0.3 g) was dispersed in 50 ml acetic acid solution (1%, v/v) and sonicated for 15 min at room temperature. At the same time, Fe3O4 (1.5 g) was added to 50 ml acetic acid solution (1%, v/v) and stirred to obtain Fe3O4 acetic acid suspension. Then, the Fe3O4 acetic acid suspension was slowly dropped into the CS/acetic acid solution and stirred for 30 min at room temperature. Next, NaOH (1%, v/v) was added to adjust the pH to neutral. At last, Fe3O4-CS nanocomposites was separated by the magnet and washed with pure water several times. The resulting support was denoted as Fe3O4-CS.
At first, CS (0.2 g) was dispersed in 50 ml acetic acid solution (1%, v/v) and sonicated for 15 min. Then, GA (2 ml) or DAC (0.5 g) was added to the CS/acetic acid solution, and NaOH (1%, v/v) was added to adjust the pH to 7.0. Next, the system was stirred at 30°C for 4 h. At last, CS-GA or CS-DAC was filtered and washed with pure water several times. Fe3O4 (1.0 g) was dispersed to 25 ml acetic acid solution (1%, v/v), and the 1.5 g CS-GA or CS-DAC was added to this suspension. Next, the system was stirred for 30 min at room temperature. Then, NaOH (1%, v/v) was added to adjust the pH to neutral. At last, nanocomposites was separated by a magnet and washed with pure water several times. The resulting supports were denoted as Fe3O4-CS-GA or Fe3O4-CS-DAC.
Anionic surface-active (SDS) and nonionic surface-active agents (Tween 80, Triton X-100) were selected to investigate the effects of different types of surfactants on the immobilization of CRL. Different surfactants (0.02 mol) were added to the phosphate buffer solution (PBS) (5 ml, pH 7.0, 0.2 M). Then, CRL (40 mg) was added to the solution and stirred at 10°C for 2 h. Next, Fe3O4-CS-DAC (0.1 g) was dispersed in the PBS (5 ml, pH7.0, 0.2 M), and the system was stirred at 30°C for 4 h. At last, the prepared immobilized CRL was separated by the magnet and washed several times with PBS (0.2 M, pH7.0). The prepared immobilized CRL was designed as Fe3O4-CS-DAC-SDS-CRL. Other supports were referred to the above method to immobilize CRL and named as Fe3O4-CS-SDS-CRL and Fe3O4-CS-GA-SDS-CRL. The support (Fe3O4-CS, Fe3O4-CS-GA or Fe3O4-CS-DAC) with CRL without surfactants were immobilized according to the above method, and the prepared immobilized CRL was designed as Fe3O4-CS-CRL, Fe3O4-CS-GA-CRL, or Fe3O4-CS-DAC-CRL (Supplementary Figures S1–S3).
The preparation of immobilized support and immobilized CRL is shown in Figure 1. The BCA method was used to detect the protein content in the supernatant, and the following formula was used to calculate CRL loading and immobilization efficiency (Walker et al., 1994). Each result was measured three times, and the average value was taken.
where
FIGURE 1. Schematic diagram of preparation of immobilized support and immobilized Candida rugosa lipase (CRL).
The activity of free and immobilized CRL were determined using the olive oil hydrolysis method (Monier et al., 2010; Li et al., 2015). Olive oil (50 ml) and gum arabic solution (50 ml, 14%, w/v) were mixed into a substrate solution. Thereafter, the substrate solution (5 ml) and PBS (5 ml, 0.2 M) were put into an Erlenmeyer flask and incubated at their optimum temperature and pH for 10 min. Then, free or immobilized CRL were added to the substrate solution and reacted 10 min. At last, acetone/ethanol (10 ml, 1/1, v/v) was added to terminate the hydrolysis reaction. The NaOH solution (0.05 mol/L) was used to titrate the fatty acid produced by hydrolysis. One unite (1 U) was defined as the amount of enzymes required to catalyze the hydrolysis of 1 μmol of acid per minute. The following formulas were used for the activity recovery rate, apparent activity, and specific activity of the enzymes. Each result was measured three times, and the average value was taken.
where
To study optimal pH conditions for the activity assay, free and immobilized CRL activity was measured under different pH values (pH6.0–8.0) at 40°C. The optimum temperature values were obtained by examining the activity of free and immobilized CRL at 30–50°C under respective optimal pH conditions. With the relative activity as an index, the maximum activity was defined as 100%.
In order to determine the thermal stability, the free and immobilized CRL were stored at 55°C for a period of time, and then the enzyme activity was measured every 30 min. The free and immobilized CRL were stored at 4°C in the refrigerator for 1 month to determine the storage stability, and then the enzyme activity was checked every 5 days. The enzyme activity was measured under the optimum conditions, and the initial enzyme activity was defined as 100%.
Kinetic parameters are important indexes for evaluating immobilized CRL. We adopted Michaelis–Menten model to evaluate the kinetic properties of free CRL and immobilized CRL, the same amount of immobilized CRL were added to triacetin emulsification solution and reacted for 3 min at respective optimum pH and temperature. Calculate the Km and Vmax values through lin-burkpolt plotting according to the following formula:
where
The reusability of immobilized enzymes were investigated through the determination of the residual in the olive oil hydrolysis under their respective optimal hydrolysis conditions. After completing a reaction cycle, the immobilized enzymes were separated by magnetic separation and washed with PBS. Then the recovered immobilized enzymes was put back into fresh reaction medium for the next cycle, and their activity was tested after each cycle.
Figure 2 displays the FT-IR spectrum of DAC, Fe3O4-CS, and Fe3O4-CS-DAC. In the DAC spectrum, the infrared image of DAC shows the characteristic absorption peaks of the aldehyde group (–CHO) at 1,747 and 884 cm−1. The former is assigned to the C=O stretching of free aldehyde and the latter to the hemiacetal structure (Li et al., 2011; Yang et al., 2019). In the Fe3O4-CS spectrum, 595 cm−1 belongs to the characteristic peak of Fe3O4, and the spectra at 2,873, 1,563, 1,415, and 1,088 cm−1 are characteristic peaks of CS (Qiu et al., 2019; Suo et al., 2019). Those confirm that CS is successfully coated on Fe3O4. In the Fe3O4-CS-DAC spectrum, it contains the characteristic peak of Fe3O4. The absorption peak at 1,620 cm−1 is considered to be the C=N functional group formed by the Schiff base reaction between the aldehyde group of DAC and the amino group of CS (Qiu et al., 2021). At the same time, the absorption peaks at 1742 and 878 cm−1 confirm that there are still aldehyde groups on the surface of the nanoparticles, which can be further covalently bound to CRL.
XRD characterization can determine the crystal structure of the material. Figure 3A shows the XRD characterization of Fe3O4, Fe3O4-CS, and Fe3O4-CS-DAC nanoparticles. The peaks appearing at 2θ = 32.6°, 35.5°, 43.2°, 53.5°, 57.0°, and 63.0° are consistent with the characteristic peaks of the crystal structure of magnetic nanoparticles Fe3O4 (Nguyen et al., 2017), which proves that the crystal structure of Fe3O4 is not damaged.
FIGURE 3. X-ray diffraction characterization (A) and thermogravimetric analysis curve (B) of support.
The weight loss of Fe3O4, Fe3O4-CS, and Fe3O4-CS-DAC are shown in Figure 3B. The weight of supports dropping before 150°C may be mainly due to the loss of water adsorbed on the nanoparticles. The weight loss after 150°C may have been caused by CS and DAC on the surface of the supports. Therefore, the results can indicate that DAC modified by CS was successfully coated on the surface of Fe3O4.
Figure 4 shows the TEM images of Fe3O4-CS and Fe3O4-CS-DAC. From Figures 4A and C, it can be seen that the boundary structure becomes blurred owing to the CS covering the surface of Fe3O4. From Figures 4B and D, it can be seen that after introducing macromolecule DAC with abundant aldehyde and hydroxy groups, which could prevent particle agglomeration through electrostatic repulsive force between particles, the agglomeration phenomenon of the support is reduced. The surface of the support is further blurred, and the particle size is significantly larger than that of Fe3O4-CS; those phenomena indicate the successful connection of DAC.
Table 1 shows the effect of different surfactants on the immobilization of CRL to Fe3O4-CS-DAC and the effect of using SDS as surfactants to immobilize CRL onto other supports. The results show that the immobilization efficiency of physical adsorption (Fe3O4-CS) and covalent cross-linking (Fe3O4-CS-GA and Fe3O4-CS-DAC) do not change; obviously, those supports all obtain high enzyme loading. The high loading is because organic–inorganic nanocomposites may provide more interaction sites for the immobilization of CRL. Among those immobilized CRLs, the activity of Fe3O4-CS-DAC-CRL is significantly improved. Compared with traditional cross-linking agents such as GA, DAC with good biocompatibility can effectively reduce the mass transfer limitation of the substrate and improve the affinity of the immobilized CRL to the substrate (Nguyen et al., 2017). Wong et al. (2020) coated the surface of Fe3O4 with SiO2 and modified it with 3-aminopropyltri ethoxysilane, and then they used GA as the cross-linking agent to covalently immobilize CRL, while the loading capacity was only 14.7 mg/g. Ji et al. (2021) reported a one-pot strategy to achieve the successful encapsulation of CRL and Fe3O4 nanoparticles into ZIF-8. The immobilized CRL only had 20.9 mg/g enzyme loading. Adikwu et al. (2021) used GA as the cross-linking agent to immobilize CRL onto the amino-functionalized SiO2/Fe3O4/GO ternary composite, but the enzyme loading was only 24.7 mg/g, and the specific activity was only 65.2 U/g. Compared with that in the above documents, it is worth mentioned that higher enzyme loading and activity could be obtained using the novel support (Fe3O4-CS-DAC).
The results show that the immobilization efficiency is slightly improved after adding three types of surfactants and the anionic surfactant SDS has the best effect especially. The results also show that the effect of using SDS as the surfactant to immobilize CRL onto different supports change slightly. However, the specific activities of immobilized CRLs are all improved to a certain extent, and Fe3O4-CS-DAC-SDS shows the best immobilization of CRL. Compared with nonionic surfactants, anionic surfactants have a stronger hydrophobic effect (Holmberg et al., 2018; Ozyilmaz et al., 2020). Zhang et al. (2018) employed molecular docking to provide an insight into the interactions of CRL and SDS. They found that the principal binding region of SDS and CRL is close to the hydrophobic force–dominated cavity, which can keep the active conformation of CRL. In addition, SDS changes the microenvironment polarity of CRL and combines with CRL through hydrophobic force, hydrogen bonding force. and electrostatic force; thus, it will partially or completely unfold the tertiary structure of CRL. These effects transfer CRL from the relatively closed form to the more open form and increases its activity (Biasutti et al., 2008; Gabriele et al., 2018; Katiyar et al., 2021).
Figure 5A shows the effect of pH (6.0–8.0) on the activity of free and immobilized CRL at 40°C. The sensitivity of immobilized CRL to pH is slightly reduced compared with that of free CRL. Optimal pH value and better acid/alkaline resistance may indicate that DAC or GA provides multiple attachment sites for CRL to maintain its conformation and increase its resistance to environmental changes (Nguyen et al., 2017).
It can be seen from Figure 5B that the sensitivity of immobilized CRL to temperature is reduced. The optimum temperature of Fe3O4-CS-GA-SDS-CRL and Fe3O4-CS-DAC-SDS-CRL is higher than that of free CRL. The optimum temperature of immobilized CRL moves to a higher direction; however, as the temperature further increases, the structure of CRL will be destroyed, resulting in a decrease in CRL activity. In the process of immobilization, the electrostatic and hydrogen bond interaction between CRL and CS or the cross-linking agent, especially the covalent attachment of CRL with DAC-modified CS, may lead to the retention of the biologically active conformation of CRL, which increases the rigidity of the immobilized CRL and the stability of the structure of CRL (Zhang et al., 2018).
The thermal stability results are shown in Figure 6A. Although the high temperature easily destroys the secondary and tertiary structures of CRL, the activity of Fe3O4-CS-DAC-SDS-CRL is still retained at 52.3% after 3.5 h, which is much higher than that of free CRL and other immobilized CRLs. DAC has a similar chemical structure to CS, so the CS composite material with DAC has better biocompatibility. At the same time, DAC is rich in aldehyde group sites, which can increase the rigid structure and improve the thermal stability of immobilized CRL. Therefore, the introduction of DAC increases the stability of CRL to structural changes and improves the thermal stability of Fe3O4-CS-DAC-SDS-CRL (Qiu et al., 2019).
Figure 6B shows the effect of storage time on the activities of free CRL and different immobilized CRL. It can be seen that after immobilization, the support has a certain protective effect on CRL. After 30 days, Fe3O4-CS-DAC-SDS-CRL retained about 80% of the initial activity, which is much higher than those of other immobilized CRLs and free CRL. It may be that DAC as the cross-linking agent can form the stable C=N bond by Schiff base reaction, and the multiple interactions including van der Waals force, hydrogen bond, and electrostatic force between CRL and the support might be enhanced with the incorporation of DAC; therefore, the storage stability of immobilized CRL could be improved (Rashid et al., 2018).
As showed in Figure 6C, Fe3O4-CS-DAC-SDS-CRL still retains 81% activity after eight operation times, which is higher than those of other immobilized CRLs. It may be attributed to the reason that the interaction between CRL and Fe3O4-CS-DAC is more stable. The stability of CRL is enhanced and the leakage of CRL from the support is reduced when using DAC with good biocompatibility and multiple functions as the cross-linking agent (Para et al., 2002; Zhang et al., 2018). Compared with many methods reported in the literature, the reusability of immobilized CRL (Fe3O4-CS-DAC-SDS-CRL) showed better performance. Zhu et al. (2015) covalently fixed CRL on polyethylene imide–modified magnetic Fe3O4 using GA as the cross-linking agent, and the immobilized CRL retained only 75.0% of its initial activity after repeated use for eight times. Guo et al. (2021) used DAC as the cross-linking agent to immobilize Rhizopus lipase onto aminated Fe3O4 and found that the activity of immobilized lipase only retained 52.1% after six runs.
By drawing the Lineweaver–Burk diagram of immobilized CRL, the apparent Michaelis constant (Km), the maximum enzymatic reaction rate (Vmax), and the catalytic efficiency (Km/Vmax) can be calculated, which are listed in Table 2. The kinetic parameters show that compared with Fe3O4-CS-SDS-CRL, the introduction of DAC significantly reduces the Km value of the support. In general, the decrease in Km value means that the affinity of reaction substrate and enzyme is enhanced, which may be more favorable for the substrate to enter the active domain of enzyme. The decrease of Vmax may be attributed to the increase of steric hindrance caused by the introduction of DAC macromolecules. In general, the Vmax/Km value is increased, which is conducive to the increase of hydrolysis activity (Xia et al., 2016; Qiu et al., 2020).
Circular dichroism has been widely used in the analysis of the secondary structure of protein molecules. The secondary structure composition of free and immobilized CRL is listed in the Table 3. These results indicate that the content of α-helix and β-sheet in the three vectors has changed. The decrease of α-helix indicates that CRL opens a higher conformation and the active site of CRL is more exposed, which makes the substrate more accessible and thus increases the enzymatic activity. The increase of β-folding could be related to the improvement of the stability of immobilized enzymes (Zhu et al., 2015; Suo et al., 2019). The β-folding ratio of CRL increased significantly after covalent fixation, which may be due to the strong interaction between CRL and the magnetic CS carrier activated by the aldehyde group, so that the structure of CRL becomes more rigidity, which is beneficial to maintaining the active conformation of CRL and enhancing the stability of CRL. This is consistent with the previous enzymological data.
In this study, a novel magnetic organic–inorganic nanocomposite support was prepared by combining Fe3O4, CS, and DAC with good biocompatibility and abundant functional groups through Schiff base reaction; then, CRL was covalently immobilized onto the support through the multifunctional DAC, which is first applied to immobilize CRL. The addition of anionic surfactant SDS can further increase the enzyme loading and specific activity of immobilized CRL, and the immobilized CRL exhibited strong pH and temperature tolerance, good thermal stability, and storage stability. Reusability study showed that after eight operation times, the hydrolysis activity of Fe3O4-CS-DAC-SDS-CRL could still be retained at 81.3%. Studies have confirmed that the use of macromolecular cross-linking agents and the addition of suitable surfactants can enhance the interaction between the supports and CRL, stabilize the active conformation of the enzymes, and thus enhance the activity and stability of CRL. The study of rationally analyzing the mechanism of changes in enzymatic properties of immobilized enzymes at the molecular level through methods using molecular simulation and spectroscopic structural characterization is currently under search.
The original contributions presented in the study are included in the article/Supplementary Material, and further inquiries can be directed to the corresponding authors.
SW: conceptualization, methodology, formal analysis, investigation, data curation, writing–original draft, and writing–review and editing. SL: methodology, investigation. RL: methodology and investigation. WZ: methodology and investigation. HX: conceptualization, methodology, funding acquisition, and supervision. YH: funding acquisition, supervision, and writing–review and editing.
This research was financially supported by National Natural Science Foundation of China (No. 22178174), National key R&D program of China (No.2021YFC2103802) and The Jiangsu Synergetic Innovation Center for Advanced Bio-Manufacture (XTC2206).
The authors declare that the research was conducted in the absence of any commercial or financial relationships that could be construed as a potential conflict of interest.
All claims expressed in this article are solely those of the authors and do not necessarily represent those of their affiliated organizations, or those of the publisher, the editors, and the reviewers. Any product that may be evaluated in this article, or claim that may be made by its manufacturer, is not guaranteed or endorsed by the publisher.
The Supplementary Material for this article can be found online at: https://www.frontiersin.org/articles/10.3389/fbioe.2022.946117/full#supplementary-material
Adikwu, G. J., Wahab, R. A., and Mahat, N. A. (2021). Ternary Biogenic Silica/magnetite/graphene Oxide Composite for the Hyperactivation of Candida Rugosa Lipase in the Esterification Production of Ethyl Valerate. Enzyme Microb. Technol. 148, 109807. doi:10.1016/j.enzmictec.2021.109807
Barbosa, O., Torres, R., Ortiz, C., Berenguer-Murcia, Á., Rodrigues, R. C., and Fernandez-Lafuente, R. (2013). Heterofunctional Supports in Enzyme Immobilization: From Traditional Immobilization Protocols to Opportunities in Tuning Enzyme Properties. Biomacromolecules 14 (8), 2433–2462. doi:10.1021/bm400762h
Barriuso, J., Vaquero, M. E., Prieto, A., and Martínez, M. J. (2016). Structural Traits and Catalytic Versatility of the Lipases from the Candida Rugosa-like Family: A Review. Biotechnol. Adv. 34 (5), 874–885. doi:10.1016/j.biotechadv.2016.05.004
Betancor, L., López-Gallego, F., Hidalgo, A., Alonso-Morales, N., Mateo, G. D.-O. C., Fernández-Lafuente, R., et al. (2006). Different Mechanisms of Protein Immobilization on Glutaraldehyde Activated Supports: Effect of Support Activation and Immobilization Conditions. Enzyme Microb. Technol. 39 (4), 877–882. doi:10.1016/j.enzmictec.2006.01.014
Biasutti, M. A., Abuin, E. B., Silber, J. J., Correa, N. M., and Lissi, E. A. (2008). Kinetics of Reactions Catalyzed by Enzymes in Solutions of Surfactants. Adv. Colloid Interface Sci. 136 (1/2), 1–24. doi:10.1016/j.cis.2007.07.001
Calvo, M. V., Plou, F. J., and Ballesteros, A. (1996). Effect of Surfactants on Activity and Stability of Native and Chemically Modified Lipases A and B from Candida Rugosa. Biocatal. Biotransformation 13 (4), 271–285. doi:10.3109/10242429609003605
De Oliveira, U. M. F., Lima de Matos, L. J. B., De Souza, M. C. M., Pinheiro, B. B., Dos Santos, J. C. S., and Gonçalves, L. R. B. (2018). Effect of the Presence of Surfactants and Immobilization Conditions on Catalysts' Properties of Rhizomucor Miehei Lipase onto Chitosan. Appl. Biochem. Biotechnol. 184 (4), 1263–1285. doi:10.1007/s12010-017-2622-1
Faraji, M., Shirani, M., and Rashidi-Nodeh, H. (2021). The Recent Advances in Magnetic Sorbents and Their Applications. Trac-Trends Anal. Chem. 141, 116302. doi:10.1016/j.trac.2021.116302
Gabriele, F., Spreti, N., Del Giacco, T., Germani, R., and Tiecco, M. (2018). Effect of Surfactant Structure on the Superactivity of Candida Rugosa Lipase. Langmuir 34 (38), 11510–11517. doi:10.1021/acs.langmuir.8b02255
Guo, H., Lei, B., Yu, J., Chen, Y., and Qian, J. (2021). Immobilization of Lipase by Dialdehyde Cellulose Crosslinked Magnetic Nanoparticles. Int. J. Biol. Macromol. 185, 287–296. doi:10.1016/j.ijbiomac.2021.06.073
Holmberg, K. (2018). Interactions between Surfactants and Hydrolytic Enzymes. Colloids Surfaces B Biointerfaces 168, 169–177. doi:10.1016/j.colsurfb.2017.12.002
Hu, Y., Jiang, X., Wu, S., Jiang, L., and Huang, H. (2013). Synthesis of Vitamin E Succinate by Interfacial Activated Candida Rugosa Lipase Encapsulated in Sol-Gel Materials. Chin. J. Catal. 34 (8), 1608–1616. doi:10.1016/S1872-2067(12)60628-7
Ismail, A. R., Kashtoh, H., and Baek, K.-H. (2021). Temperature-resistant and Solvent-Tolerant Lipases as Industrial Biocatalysts: Biotechnological Approaches and Applications. Int. J. Biol. Macromol. 187, 127–142. doi:10.1016/j.ijbiomac.2021.07.101
Ji, Y., Wu, Z., Zhang, P., Qiao, M., Hu, Y., Shen, B., et al. (2021). Enzyme-functionalized Magnetic Framework Composite Fabricated by One-Pot Encapsulation of Lipase and Fe3O4 Nanoparticle into Metal-Organic Framework. Biochem. Eng. J. 169, 107962. doi:10.1016/j.bej.2021.107962
Katiyar, M., Abida, K., and Ali, A. (2021). Candida Rugosa Lipase Immobilization over SBA-15 to Prepare Solid Biocatalyst for Cotton Seed Oil Transesterification. Mater. Today Proc. 36 (3), 763–768. doi:10.1016/j.matpr.2020.06.061
Kim, U.-J., Lee, Y. R., Kang, T. H., Choi, J. W., Kimura, S., and Wada, M. (2017). Protein Adsorption of Dialdehyde Cellulose-Crosslinked Chitosan with High Amino Group Contents. Carbohydr. Chem. 163, 34–42. doi:10.1016/j.carbpol.2017.01.052
Leung, H.-W. (2001). Ecotoxicology of Glutaraldehyde: Review of Environmental Fate and Effects Studies. Ecotoxicol. Environ. Saf. 49 (1), 26–39. doi:10.1006/eesa.2000.2031
Li, H., Wu, B., Mu, C., and Lin, W. (2011). Concomitant Degradation in Periodate Oxidation of Carboxymethyl Cellulose. Carbohydr. Polym. 84 (3), 881–886. doi:10.1016/j.carbpol.2010.12.026
Li, X., Zhang, C., Li, S., Huang, H., and Hu, Y. (2015). Improving Catalytic Performance of Candida Rugosa Lipase by Chemical Modification with Polyethylene Glycol Functional Ionic Liquids. Ind. Eng. Chem. Res. 54 (33), 8072–8079. doi:10.1021/acs.iecr.5b01881
Liang, S., Wu, X.-L., Xiong, J., Zong, M.-H., and Lou, W.-Y. (2020). Metal-organic Frameworks as Novel Matrices for Efficient Enzyme Immobilization: An Update Review. Coord. Chem. Rev. 406, 213149. doi:10.1016/j.ccr.2019.213149
López-Serrano, P., Cao, L., van Rantwijk, F., and Sheldon, R. A. (2002). Cross-linked Enzyme Aggregates with Enhanced Activity: Application to Lipases. Biotechnol. Lett. 24 (16), 1379–1383. doi:10.1023/a:1019863314646
Manoel, E. A., dos Santos, J. C. S., Freire, D. M. G., Rueda, N., and Fernandez-Lafuente, R. (2015). Immobilization of Lipases on Hydrophobic Supports Involves the Open Form of the Enzyme. Enzyme Microb. Technol. 71, 53–57. doi:10.1016/j.enzmictec.2015.02.001
Mateo, C., Palomo, J. M., Fernandez-Lorente, G., Guisan, J. M., and Fernandez-Lafuente, R. (2007). Improvement of Enzyme Activity, Stability and Selectivity via Immobilization Techniques. Enzyme Microb. Technol. 40 (6), 1451–1463. doi:10.1016/j.enzmictec.2007.01.018
Matveeva, V. G., and Bronstein, L. M. (2021). Magnetic Nanoparticle-Containing Supports as Carriers of Immobilized Enzymes: Key Factors Influencing the Biocatalyst Performance. Nanomaterials 11 (9), 2257. doi:10.3390/nano11092257
Monier, M., Wei, Y., and Sarhan, A. A. (2010). Evaluation of the Potential of Polymeric Carriers Based on Photo-Crosslinkable Chitosan in the Formulation of Lipase from Candida Rugosa Immobilization. J. Mol. Catal. B Enzym. 63 (1-2), 93–101. doi:10.1016/j.molcatb.2009.12.015
Nguyen, X. S., Zhang, G., and Yang, X. (2017). Mesocrystalline Zn-Doped Fe3O4 Hollow Submicrospheres: Formation Mechanism and Enhanced Photo-Fenton Catalytic Performance. ACS Appl. Mat. Interfaces 9 (10), 8900–8909. doi:10.1021/acsami.6b16839
Nunes, Y. L., de Menezes, F. L., de Sousa, I. G., Cavalcante, A. L. G., Cavalcante, F. T. T., da Silva Moreira, K., et al. (2021). Chemical and Physical Chitosan Modification for Designing Enzymatic Industrial Biocatalysts: How to Choose the Best Strategy? Int. J. Biol. Macromol. 181, 1124–1170. doi:10.1016/j.ijbiomac.2021.04.004
Ozyilmaz, E., and Eski, F. (2020). Effect of Cyclic and Acyclic Surfactants on the Activity of Candida Rugosa Lipase. Bioprocess Biosyst. Eng. 43 (11), 2085–2093. doi:10.1007/s00449-020-02397-3
Para, A., and Karolczyk-Kostuch, S. (2002). Metal Complexes of Starch Dialdehyde Dithiosemicarbazone. Carbohydr. Polym. 50 (2), 151–158. doi:10.1016/S0144-8617(02)00011-5
Qiao, W., Zhang, Z., Qian, Y., Xu, L., and Guo, H. (2022). Bacterial Laccase Immobilized on a Magnetic Dialdehyde Cellulose without Cross-Linking Agents for Decolorization. Colloids Surfaces A Physicochem. Eng. Aspects 632, 127818. doi:10.1016/j.colsurfa.2021.127818
Qiu, X., Qin, J., Xu, M., Kang, L., and Hu, Y. (2019). Organic-inorganic Nanocomposites Fabricated via Functional Ionic Liquid as the Bridging Agent for Laccase Immobilization and its Application in 2,4-dichlorophenol Removal. Colloids Surfaces B Biointerfaces 179, 260–269. doi:10.1016/j.colsurfb.2019.04.002
Qiu, X., Wang, S., Miao, S., Suo, H., Xu, H., and Hu, Y. (2021). Co-immobilization of Laccase and ABTS onto Amino-Functionalized Ionic Liquid-Modified Magnetic Chitosan Nanoparticles for Pollutants Removal. J. Hazard. Mater. 401, 123353. doi:10.1016/j.jhazmat.2020.123353
Qiu, X., Wang, Y., Xue, Y., Li, W., and Hu, Y. (2020). Laccase Immobilized on Magnetic Nanoparticles Modified by Amino-Functionalized Ionic Liquid via Dialdehyde Starch for Phenolic Compounds Biodegradation. Chem. Eng. J. 391, 123564. doi:10.1016/j.cej.2019.123564
Rashid, R., Anwar, Z., Zafar, M., Rashid, T., and Butt, I. (2018). Chitosan-alginate Immobilized Lipase Based Catalytic Constructs: Development, Characterization and Potential Applications. Int. J. Biol. Macromol. 119, 992–1001. doi:10.1016/j.ijbiomac.2018.07.192
Rodrigues, R. C., Berenguer-Murcia, Á., and Fernandez-Lafuente, R. (2011). Coupling Chemical Modification and Immobilization to Improve the Catalytic Performance of Enzymes. Adv. Synth. Catal. 353 (13), 2216–2238. doi:10.1002/adsc.201100163
Ruan, C.-Q., Strømme, M., and Lindh, J. (2018). Preparation of Porous 2,3-dialdehyde Cellulose Beads Crosslinked with Chitosan and Their Application in Adsorption of Congo Red Dye. Carbohydr. Polym. 181, 200–207. doi:10.1016/j.carbpol.2017.10.072
Schmid, R. D., and Verger, R. (1998). Lipases: Interfacial Enzymes with Attractive Applications. Angew. Chem. Int. Ed. Engl. 37 (12), 1608–1633. doi:10.1002/(SICI)1521-3773(19980703)37:12<1608::AID-ANIE1608>3.0.CO;2-V
Sharma, P. R., and Varma, A. J. (2014). Thermal Stability of Cellulose and Their Nanoparticles: Effect of Incremental Increases in Carboxyl and Aldehyde Groups. Carbohydr. Polym. 114, 339–343. doi:10.1016/j.carbpol.2014.08.032
Sherawat, M., Rahi, R. K., Gupta, V., Neelam, D., and DevkiSain, D. (2021). Prevention and Control of Food Spoilage: An Overview (Review Article). ijpbs 11 (1), 124–130. doi:10.1016/j.carbpol.2014.08.03210.21276/ijpbs.2021.11.1.16
Suo, H., Gao, Z., Xu, L., Xu, C., Yu, D., Xiang, X., et al. (2019). Synthesis of Functional Ionic Liquid Modified Magnetic Chitosan Nanoparticles for Porcine Pancreatic Lipase Immobilization. Mater. Sci. Eng. C Mater. Biol. Appl. 96, 356–364. doi:10.1016/j.msec.2018.11.041
Suo, H., Xu, L., Xu, C., Chen, H., Yu, D., Gao, Z., et al. (2018). Enhancement of Catalytic Performance of Porcine Pancreatic Lipase Immobilized on Functional Ionic Liquid Modified Fe3O4-Chitosan Nanocomposites. Int. J. Biol. Macromol. 119, 624–632. doi:10.1016/j.ijbiomac.2018.07.187
Tamaddon, F., and Arab, D. (2019). Urease Covalently Immobilized on Cotton-Derived Nanocellulose-Dialdehyde for Urea Detection and Urea-Based Multicomponent Synthesis of Tetrahydro-Pyrazolopyridines in Water. RSC Adv. 9 (71), 41893–41902. doi:10.1039/C9RA05240B
Vanleeuw, E., Winderickx, S., Thevissen, K., Lagrain, B., Dusselier, M., Cammue, B. P. A., et al. (2019). Substrate-specificity of Candida Rugosa Lipase and its Industrial Application. ACS Sustain. Chem. Eng. 7 (19), 15828–15844. doi:10.1021/acssuschemeng.9b03257
Varma, A. J., and Kulkarni, M. P. (2002). Oxidation of Cellulose under Controlled Conditions. Polym. Degrad. Stab. 77 (1), 25–27. doi:10.1016/S0141-3910(02)00073-3
Verger, R. (1997). 'Interfacial Activation' of Lipases: Facts and Artifacts. Trends Biotechnol. 15 (1), 32–38. doi:10.1016/S0167-7799(96)10064-0
Walker, J. M. (1996). The Bicinchoninic Acid (BCA) Assay for Protein Quantitation. Methods Mol. Biol. 32, 11–14. doi:10.1007/978-1-60327-259-9_3
Wang, J., Zhao, G., Jing, L., Peng, X., and Li, Y. (2015). Facile Self-Assembly of Magnetite Nanoparticles on Three-Dimensional Graphene Oxide-Chitosan Composite for Lipase Immobilization. Biochem. Eng. J. 98, 75–83. doi:10.1016/j.bej.2014.11.013
Wong, W. K. L., Wahab, R. A., and Onoja, E. (2020). Chemically Modified Nanoparticles from Oil Palm Ash Silica-Coated Magnetite as Support for Candida Rugosa Lipase-Catalysed Hydrolysis: Kinetic and Thermodynamic Studies. Chem. Pap. 74 (4), 1253–1265. doi:10.1007/s11696-019-00976-7
Xia, T.-T., Liu, C.-Z., Hu, J.-H., and Guo, C. (2016). Improved Performance of Immobilized Laccase on Amine-Functioned Magnetic Fe 3 O 4 Nanoparticles Modified with Polyethylenimine. Chem. Eng. J. 295, 201–206. doi:10.1016/j.cej.2016.03.044
Xiang, X. R., Huang, H., and Hu, Y. (2017). Research Progress on Enzyme Immobilized on Nanocomposites. Chin. J. Inorg. Chem. 33 (1), 1–15. doi:10.11862/CJIC.2017.016
Yang, X., Chen, Y., Yao, S., Qian, J., Guo, H., and Cai, X. (2019). Preparation of Immobilized Lipase on Magnetic Nanoparticles Dialdehyde Starch. Carbohydr. Polym. 218, 324–332. doi:10.1016/j.carbpol.2019.05.012
Zhang, R., Liu, Y., Huang, X., Xu, M., Liu, R., and Zong, W. (2018). Interaction of a Digestive Protease, Candida Rugosa Lipase, with Three Surfactants Investigated by Spectroscopy, Molecular Docking and Enzyme Activity Assay. Sci. Total Environ. 622-623, 306–315. doi:10.1016/j.scitotenv.2017.11.305
Zhao, H., and Heindel, N. D. (1991). Determination of Degree of Substitution of Formyl Groups in Polyaldehyde Dextran by the Hydroxylamine Hydrochloride Method. Pharm. Res. 08 (3), 400–402. doi:10.1023/A:1015866104055
Zhao, K., Chen, B., Li, C., Li, X. F., Li, K. B., and Shen, Y. H. (2018). Immobilization of Candida Rugosa Lipase on Glutaraldehyde-Activated Fe 3 O 4 @Chitosan as a Magnetically Separable Catalyst for Hydrolysis of Castor Oil. Eur. J. Lipid Sci. Technol. 120 (1), 1700373. doi:10.1002/ejlt.201700373
Zhu, W., Li, Y., Zeng, F., Yin, H., Wang, L., and Zhu, H. (2015). Superparamagnetic Fe3O4nanoparticles Modified by Water-Soluble and Biocompatible Polyethylenimine for Lipase Immobilization with Physical and Chemical Mechanisms. RSC Adv. 5 (29), 23039–23045. doi:10.1039/c4ra15832f
Ziegler-Borowska, M., Chelminiak-Dudkiewicz, D., Siódmiak, T., Sikora, A., Wegrzynowska-Drzymalska, K., Skopinska-Wisniewska, J., et al. (2017). Chitosan-collagen Coated Magnetic Nanoparticles for Lipase Immobilization-New Type of "Enzyme Friendly" Polymer Shell Crosslinking with Squaric Acid. Catalysts 7 (1), 26–14. doi:10.3390/catal7010026
Keywords: Candida rugosa lipase, lipase immobilization, dialdehyde cellulose, magnetic nanoparticles, interface activation
Citation: Wang S, Li S, Liu R, Zhang W, Xu H and Hu Y (2022) Immobilization of Interfacial Activated Candida rugosa Lipase Onto Magnetic Chitosan Using Dialdehyde Cellulose as Cross-Linking Agent. Front. Bioeng. Biotechnol. 10:946117. doi: 10.3389/fbioe.2022.946117
Received: 17 May 2022; Accepted: 01 June 2022;
Published: 18 July 2022.
Edited by:
Zheng Mingming, Oil Crops Research Institute (CAAS), ChinaReviewed by:
Gang Xu, Zhejiang University, ChinaCopyright © 2022 Wang, Li, Liu, Zhang, Xu and Hu. This is an open-access article distributed under the terms of the Creative Commons Attribution License (CC BY). The use, distribution or reproduction in other forums is permitted, provided the original author(s) and the copyright owner(s) are credited and that the original publication in this journal is cited, in accordance with accepted academic practice. No use, distribution or reproduction is permitted which does not comply with these terms.
*Correspondence: Huajin Xu, MjAxOTEwMDA2NjYwQG5qdGVjaC5lZHUuY24=; Yi Hu, aHV5aUBuanRlY2guZWR1LmNu
Disclaimer: All claims expressed in this article are solely those of the authors and do not necessarily represent those of their affiliated organizations, or those of the publisher, the editors and the reviewers. Any product that may be evaluated in this article or claim that may be made by its manufacturer is not guaranteed or endorsed by the publisher.
Research integrity at Frontiers
Learn more about the work of our research integrity team to safeguard the quality of each article we publish.