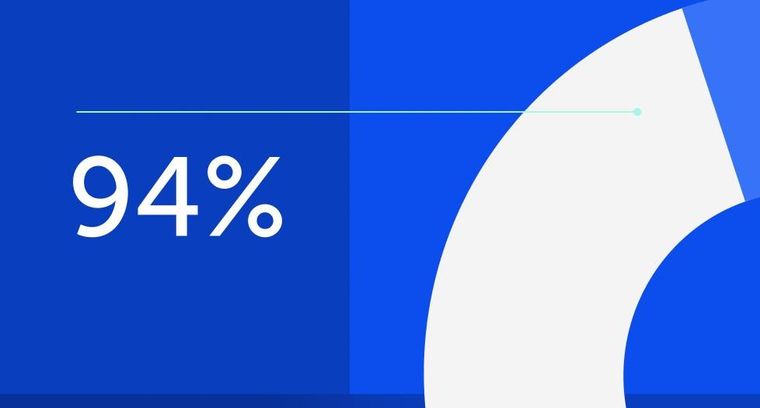
94% of researchers rate our articles as excellent or good
Learn more about the work of our research integrity team to safeguard the quality of each article we publish.
Find out more
ORIGINAL RESEARCH article
Front. Bioeng. Biotechnol., 30 June 2022
Sec. Bioprocess Engineering
Volume 10 - 2022 | https://doi.org/10.3389/fbioe.2022.937838
This article is part of the Research TopicFrom Biomass to Bio-energy and Bio-chemicals: Pretreatment, Thermochemical Conversion, Biochemical Conversion and Its Bio-Based ApplicationsView all 20 articles
The sustainability and economic viability of the bioethanol production process from lignocellulosic biomass depend on efficient and effective pretreatment of biomass. Traditional pretreatment strategies implicating the use of mineral acids, alkalis, and organic solvents release toxic effluents and the formation of inhibitory compounds posing detrimental effects on the environment and interfering with the enzymatic saccharification process, respectively. Ionic liquids (ILs) as green solvents were used to overcome this issue, but the deep eutectic solvent as an emerging class of ionic liquids performed better in terms of making the process environmentally and economically viable. The green solvent-based pretreatment strategy applied in the current research was levulinic, acid-based natural deep eutectic solvent (NADES). Three different hydrogen bond acceptors (HBAs)—acetamide, betaine, and choline chloride—in combination with levulinic acid as hydrogen bond donor (HBD) in (HBD: HBA) molar ratio 2:1, were screened for biomass pretreatment. The best deep eutectic solvent was levulinic acid: choline chloride in an optimized molar ratio of 1:0.5, resulting in 91% delignification. The physicochemical parametric optimization of saccharification exhibited maximum enzymatic hydrolysis of 25.87% with 125 mg of pretreated sawdust via simultaneous addition of three thermostable cellulases [i.e., endo-1,4-β-D-glucanase (240 U), exo-1,4-β-D-glucanase (180 U), and β-glucosidase (320 U)] for 5 h of incubation at 75°C. The reducing sugar slurry obtained from the saccharified biomass was then added to a fermentation medium for bioethanol production, and a maximum of 11.82% of production was obtained at 30°C, 72 h, and 180 rpm using a 2.5% 24 h old Saccharomyces cerevisiae seed culture. The current study revealed that the levulinic-based deep eutectic solvent exhibited remarkable delignification, which led to the efficient enzymatic hydrolysis of sawdust and hence bioethanol production. Furthermore, it will prospect new avenues in bioethanol production using a deep eutectic solvent. Deep eutectic solvent overcame the issues posed by ionic liquids: toxicity, expensive and complex preparation, and non-biodegradability.
Depletion of fossil fuel reserves and increased emission of greenhouse gases leading to climatic changes and global warming have necessitated the notion of alternative bio-based fuel to be practically implemented in the near future. The key step in shifting from a non-renewable to a renewable energy source, which is a shift from petroleum-based to bio-based fuels, is the driving phenomena of biorefineries (Cunha et al., 2020). Biorefinery is defined as the equivalent renewable of a petroleum refinery, the difference being in the starting raw material. Biorefinery converts the raw material into a wide variety of chemicals and energy carriers, which can lead to the development of the circular economy. The biorefinery concept is based on lignocellulosic materials, which produce bio-based products that are recoverable (to a certain degree) and recyclable. Biorefineries have made a fine amalgam of green chemistry, keeping in view the environmental impact of fuel. This amalgam aims to limit or minimize the use and generation of hazardous chemicals (Capolupo and Faraco, 2016). Therefore, sustainable bioethanol production can be achieved using green solvents that are deep eutectic solvents, so the end product of green biorefineries is environmentally benign and recyclable and produces minimum waste.
Lignocellulosic biomass is an inexhaustible biomaterial, mainly composed of lignin, hemicellulose, cellulose, and extractives in different proportions (Huang et al., 2022a). Sawdust from the sawmill industry is a potential, cost-efficient raw material to produce bioethanol. The use of sawdust for biofuel production promotes the local valorization of wood waste, establishing the concept of forest biorefinery (Alio et al., 2021). From the bioethanol production perspective, lignin is a barrier to enzymatic hydrolysis because it irreversibly binds to the cellulases rendering enzyme adsorption on cellulose (Takada et al., 2020; Huang et al., 2022b). Pretreatment is employed to disintegrate the cross-linked biomass fractions, enhancing the biodegradability and ease of access of hemicellulose and cellulose for enzymatic hydrolysis (Robak and Balcerek, 2020).
The choice of an appropriate pretreatment method is critical in terms of determining the sustenance and the economic viability of a project. It is evident from the literature that the previous pretreatment techniques were more concentrated on the techno-economic viability than on the sustenance of the environment (Vieira et al., 2020). The green solvent used in this research is deep eutectic solvent (DES), acknowledged as a class of ionic liquids (ILs). Deep eutectic solvents are defined as a group of large, non-symmetrical ions with low lattice energy and hence low melting points (Smith et al., 2014). They are prepared via complexation or a combination of hydrogen bond donors (HBDs) (alcohols, amides, amines, or carboxylic acids) and quaternary ammonium salts as hydrogen bond acceptors (HBA) at a moderate temperature of 60°C –80°C in a certain ratio to form a eutectic mixture (Satlewal et al., 2018).
Deep eutectic solvents and ionic liquids are alike in their physical properties but have varying chemical properties. Both exhibits low or negligible vapor pressure, non-flammability, and a wide liquid range. Ionic liquids have wide electrochemical windows and high dissolution ability, used in biomass dissolution and conversion as a solvent and a catalyst (Smith et al., 2014; Chen and Mu, 2019). The drawbacks that hold back ILs as a green solvent are their complex, expensive preparation, toxicity, and non-biodegradability. On the contrary, these deep eutectic solvents are cost-effective, easily prepared, potentially biodegradable, innocuous, and safe (Zhang et al., 2020). Deep eutectic solvents fulfill the twelve principles of green chemistry, which entails their use in the sustainable pretreatment of biomass. They have high air stability, thermal stability, low volatility, non-inflammability, and high purity (Xu et al., 2020).
The molar ratio of HBD and HBA is closely related in the context of their potential to remove lignin and hemicellulose or their dissolution ability of chemical components, that is, lignin and cellulose, subsequently influencing the saccharification process/enzymatic hydrolysis of the pretreated substrate (Xu et al., 2020). Saccharification of pretreated lignocellulosic biomass is another crucial step in the bioconversion of substrate into the desired end product, ethanol, via releasing fermentable sugars from crystalline cellulose and hemicellulose (Kucharska et al., 2020). Enzymatic saccharification/hydrolysis is usually carried out via cellulases and hemicellulases. Cellulases are commonly used to refer to the three enzymes that convert cellulose into glucose (fermentable monosaccharide): endocellulase, exocellulase, and glucosidase (Zhao et al., 2019). Different physicochemical parameters affect hydrolysis efficiency, such as incubation temperature, pH, agitation speed/rpm, incubation time, enzyme/substrate ratio, and particle size (Chavan and Gaikwad, 2021; Faizal et al., 2021).
Separate hydrolysis and fermentation (SHF) is the most studied technique in the bioethanol production process. This process enables independent optimization of the saccharification step for maximal sugar release and the fermentation step for ethanol production (Moodley and Gueguim Kana, 2019). Limitations of this strategy are inhibition of cellulases by cellobiose and glucose and the potential risk of contamination due to the prolonged duration of saccharification (Keshav et al., 2021).
Maugeri and Domı (2012) first prepared choline chloride: levulinic acid DES in equimolar concentration, but exploitation of DES for biomass pretreatment was first reported by Gunny et al. (2014). Several reports were available with choline chloride-based DES pretreatment of lignocellulosic biomass (Wang et al., 2020). However, reports regarding levulinic acid-based DES are infrequent (Ling et al., 2020). By considering the research gap, the current research work focuses on selecting HBA for levulinic acid-based DES pretreatment, optimization of the molar ratio (HBD:HBA), and physicochemical parametric optimization of saccharification and bioethanol production.
The thermophilic cellulases, endo-1,4-
Sawdust was acquired from the local furniture market of Lahore, Punjab, Pakistan. The biomass was washed, dried, and sieved with mesh size 400 to attain homogeneously sized particles.
Levulinic acid-based DES with three variable HBAs (i.e., acetamide, betaine, and choline chloride) was prepared at an initial molar ratio of 2:1 (HBD: HBA) in screw-capped reagent bottles and kept in a shaking water bath, 80°C, 120 rpm for 1–2 h till complete dissolution and appearance of clear solution (Ling et al., 2020). After selecting the appropriate HBA for DES, molar ratios of both HBA and HBD varied from 0.1 to 2.5 M.
Pretreatment was conducted for 1 g/100 ml of DES in a screw-capped reagent bottle at 121°C, 15 psi for 30 min. Upon pretreatment, the pretreated sample was separated from DES by filtration. The filtered pretreated biomass was washed to neutral pH to remove all the residual DES.
The lignin content of untreated and pretreated biomass was estimated using the following equation (Irfan et al., 2011):
The percentage delignification was calculated using the following formula:
L1 = lignin content of control (untreated substrate).
L2 = lignin content of the pretreated substrate.
Cellulosic content on a dry matter basis was estimated using the method of Gopal and Ranjhan (1980) using the following equation:
The hemicellulosic content of the pretreated and untreated substrate was determined by NDF and ADF treatment (Van Soest and Roberston, 1979):
The saccharification of pretreated sawdust was conducted by taking 100 mg of the pretreated substrate in a screw-capped reagent bottle. Cellulases, endo-1,4-
The physicochemical parameters optimized for saccharification were sequential/simultaneous addition of cellulases, incubation time (1–7 h), incubation temperature (70 °C –90°C), substrate concentration (50–200 mg), concentration of endo-1,4-
The vegetative seed inoculum of Saccharomyces cerevisiae (IIB-56) acquired from the culture bank of the Institute of Industrial Biotechnology, GC University Lahore, Pakistan, was prepared by aseptically transferring dry (granulated) baker’s yeast into a 50 ml medium containing 10 g glucose, 0.25 g yeast extract, and 0.15 g ammonium sulfate. The flask was incubated at 30 C, 180 rpm for 24 h.
The submerged fermentation for ethanol production was carried out in a fermentation medium using the reducing sugar slurry (in place of glucose) obtained after optimization. The fermentation medium was inoculated with 2.5% of 24 h seed inoculum of S. cerevisiae aseptically, incubated at 30°C, 180 rpm for 72 h. The samples were harvested at regular intervals to estimate the ethanol content during fermentation (Yuan et al., 2011).
The experiment was run in triplicate. Statistical analysis was done using SPSS version 16.00 (IBM Analytics, New York, United States). One-way ANOVA was applied on replicates to observe the significant difference with the probability (p) value. Error bars in the figures of the Results section indicated standard deviation (
The pretreatment of sawdust with levulinic acid-based DES prepared with three variable HBAs exhibited maximum delignification (80.5%, p < 0.05) and cellulosic content (60.7%, p < 0.05) for levulinic acid: choline chloride DES (2:1) compared to levulinic acid: betaine, levulinic acid: acetamide DES exhibiting 65% (p < 0.05) and 24% (p < 0.05) delignification, as shown in Figure 1.
FIGURE 1. Content estimation of sawdust pretreated with levulinic acid-based DESs with varying HBAs.
Further optimization of the molar ratio of HBA, that is, choline chloride varying from 0.1 to 2.5 M, showed maximum delignification (87.1%, p < 0.05) for 0.5 M choline chloride: 2 M levulinic acid based-DES shown in Figure 2. However, upon varying concentration of levulinic acid (0.1–2.5 M), maximum delignification (90.1%, p < 0.05) and cellulosic content (70.16%, p < 0.05) was recorded for the 1:0.5 M concentration of levulinic acid and choline chloride DES (Figure 3).
FIGURE 2. Content estimation of levulinic acid-based pretreatment with varying choline chloride concentrations (M).
Saccharification of pretreated sawdust with variable HBAs and molar ratios of DES constituents resulted in maximum saccharification (7.99%; p < 0.05) for levulinic acid: choline chloride (2:1) pretreated sawdust (Figure 4). However, upon the varying concentration of HBA of selected DES, maximum saccharification of 9.73% (p < 0.05) was recorded for levulinic acid: choline chloride (2:0.5) and 15.44% (p < 0.05) for levulinic acid: choline chloride (1:0.5), upon the varying concentration of HBD as evident from Figures 5, 6 respectively.
FIGURE 5. Saccharification of sawdust pretreated with varying choline chloride concentrations (0.1–2.5 M).
FIGURE 6. Saccharification of sawdust pretreated with varying levulinic acid concentrations (0.1–2.5 M).
The effect of cellulase addition was evaluated by adding cellulases simultaneously (all at once) and sequentially (after a regular interval of 2 h) for 6 h; at 85°C; 50 rpm. Maximum saccharification for simultaneous and sequential addition of cellulases was 15.93% (p < 0.05) and 15.23% (p < 0.05) respectively, as exhibited in Figure 7 (Figures 7A,B). Thus, the simultaneous addition of cellulases exhibited better hydrolysis than the sequential one.
The incubation time of the simultaneously added cellulase mixture for saccharification was 5 h, exhibiting maximum saccharification and total reducing sugar of 15.88% (p < 0.05) and 11.44 mg (p < 0.05), respectively (Figure 8).
For thermophilic cellulases, temperature regimes (70°C–90°C) with a regular increment of 5°C were used to evaluate the optimum saccharification of biomass. Maximum saccharification (16.62%, p < 0.05) and total reducing sugar (11.66 mg, p < 0.05) was estimated at 75°C, 5 h, 50 rpm, using 100 mg of pretreated sawdust, evident from Figure 9.
By increasing substrate concentration from 50 to 125 mg, saccharification also increased from 11.31% (p < 0.05) to 20.99% (p < 0.05). However, a further increase in biomass concentration decreased saccharification to 7.22%, but the total reducing sugar did not follow the same trend (Figure 10). Thus, substrate concentration of 125 mg was optimum with maximum saccharification (20.99%, p < 0.05) and release of total reducing sugar (18.41 mg, p < 0.05).
The units of endoglucanase varied from 40 to 290 U with a regular increment of 50 U to analyze the concentration required for optimal saccharification while keeping the units of the remaining two cellulases constant. Endoglucanase (240 U) gave maximum saccharification of 23.55% (p < 0.05), upon 5 h of incubation at 75°C using 125 mg of pretreated sawdust (Figure 11).
The units of exoglucanase varied from 30 to 230 U with a regular increment of 50 U to evaluate the optimum enzyme units for enzymatic hydrolysis. The maximum saccharification and total reducing sugar was 24.54% (p < 0.05) and 21.52 mg (p < 0.05) for 180 U of exoglucanase with 240 U of endoglucanase and 220 U of
The released reducing sugars obtained after optimized saccharification of pretreated sawdust were subjected to the final step of ethanol production by S. cerevisiae. The ethanol production was estimated by potassium dichromate reagent, which turned green from orange, indicating the presence of ethanol in the supernatant. Maximum ethanol production (11.82%, p < 0.05) was obtained after 72 h of incubation at 30°C, 180 rpm. Initially, ethanol production estimated after 24 h was not appreciable (0.647%, p < 0.05), but after 48 h, a noticeable increase in ethanol production was estimated. Upon 96 h of incubation, ethanol production (11.23%, p < 0.05) was observed to decline (Figure 14).
The sustainability and the economic viability of bioethanol production were achieved via lignocellulosic biomass (sawdust) and novel levulinic acid-based pretreatment applied in current research. Pretreatment is the key bottleneck and the costliest step in the sustenance of such projects. DES pretreatment resolved the issue. The DES used in the current research were natural deep eutectic solvents belonging to Class III, reported to be used in biomass valorization (Scelsi et al., 2021). Levulinic acid as HBD was used because it had a single carboxyl group; the second functional group was a ketonic group that interacted with lignin rather than the carboxyl group, which was responsible for the reduction in lignin solubility (Soares et al., 2017; Magalhães et al., 2021). Three hydrogen bond acceptors—acetamide, betaine, and choline chloride—were of natural origin to prepare natural deep eutectic solvent (NADES) to out rule the potential environmental hazards posed by ILs and other organic solvents used for pretreatment (Scelsi et al., 2021).
The efficient removal of lignin and hemicellulose depends on the cleavage of a covalent bond between lignin and hemicellulose containing phenyl glucoside, benzyl ester/ether groups, and cross-linking hydrogen bonds. The strong hydrogen bond in lignocellulosic biomass might be weakened by a competing hydrogen bond formed between the chloride ions from choline chloride constituting DES and the hydroxyl group of lignin and carbohydrate. Thus, breaking the lignin carbohydrate complexes and removing lignin and hemicellulose in DES during pretreatment (Liu et al., 2017; Smink et al., 2019). On this basis, levulinic acid: choline chloride DES exhibited better potential to remove lignin and hemicellulose than levulinic acid: betaine and levulinic acid: acetamide DESs. Similarly, in the literature, a few articles reported the exploitation of levulinic acid and keto-organic acid in DES preparation used for biomass valorization.
The molar ratio of HBD (i.e., levulinic acid) and selected hydrogen bond acceptor (HBA) (i.e., choline chloride) was optimized to be 1:0.5 whereas, the reported molar ratio for this combination was 2:1 by Ling et al. (2020) and 1:1 by Alvarez-Vasco et al. (2016). The molar ratio of levulinic acid (HBD) to choline chloride (HBA) was responsible for affecting the strength of the hydrogen bond in the resulting DES. The strength of the DES hydrogen bond, as a result, affects their mobility as well as the degradation potential of biomass in pretreatment (Xu et al., 2021). Therefore, maybe the molar ratio of 1:0.5 exhibited strong hydrogen bonding compared to the other variable ratios of HBD to HBA. Thus, pretreatment intensity was best at this molar ratio of HBD to HBA forming DES, that is, 91% of delignification and cellulosic content of 70.16%.
After the pretreatment process, enzymatic hydrolysis/saccharification of lignocellulosic biomass was recognized as the techno-economic bottleneck in converting lignocellulosic biomass into ethanol (Alio et al., 2020). The type of pretreatment given to the substrate was among the factors influencing saccharification (Vasić et al., 2021). This was evident from the result of saccharification for DES pretreatment with variable HBAs and varying concentrations of HBA and HBD. Chourasia et al. (2021) reported efficient saccharification of choline chloride-based DES pretreated biomass. Ling et al. (2020) also reported maximum enzymatic hydrolysis for the pretreated biomass exhibiting maximum delignification with levulinic acid: choline chloride DES. The maximum saccharification of 15.44% for the optimized levulinic acid and choline chloride molar ratio of 1:0.5 from the initial pretreatment molar ratio of 2:1 was 7.99%. Thus, the adsorption of cellulases to exposed cellulose after efficient delignification increased saccharification. The decrease in percentage saccharification might be due to the deposition of lignin and pseudo lignin droplets on the surface of pretreated biomass, due to which enzymes adsorbs unproductively on the substrate (Lin et al., 2021).
The addition of cellulases played a critical role in saccharification; three basic cellulases (endoglucanases, exoglucanases, and
Incubation time for enzymatic hydrolysis determines the extent of contact between enzyme (i.e., cellulase) and pretreated substrate (sawdust) molecules involved in the reaction, affecting the rate of product formation (i.e., reducing sugar). After 5 h of incubation, saccharification decreased due to the exhaustion of amorphous cellulose by cellulases attack in the initial stages and the deferred hydrolysis rate of crystalline cellulose (Sridevi et al., 2015).
In the current research, the thermostable cellulase sourced from Thermotoga petrophila cloned in Escherichia coli exhibited maximum enzymatic hydrolysis of 16.62% at 75°C. According to Arrhenius’s theory, the rate of enzyme-catalyzed reaction rises with increasing temperature but up to a certain limit. The kinetic energy of reacting molecules increases with the temperature rise, resulting in a higher collision rate and subsequent substrate conversion into a product. However, at elevated temperature, water that critically affects protein folding and structure are lost, due to which enzyme activity is compromised (Chavan and Gaikwad, 2021). The deviations were possibly due to the difference in the substrate used in enzyme assay and saccharification, as cellulases exhibit differential specificity and affinity for soluble and insoluble substrates (Sidar et al., 2020). Most of the thermophilic microbes did not have the potential to degrade crystalline cellulose due to the lack of cellulose-binding modules (CBMs) (Maki et al., 2009). The half-life of cellulases has been reported to be 8 h at 80°C (with optimum pH) by Wang et al. (2011), so upon increasing temperature, the half-life decreases. That is why the saccharification dropped at elevated temperatures.
The maximum substrate concentration of 125 mg exhibited the highest saccharification yield (20.99%), and a further increase in the substrate was not supportive in increasing saccharification percentage and release of reducing sugar. A similar effect of increasing substrate concentration resulting in deferred conversion rate/enzymatic hydrolysis was justifiable for the factors limiting the substrate conversion into the product as inadequate stirring for higher substrate loadings, increased viscosity, deferred seepage of cellulases to cellulose, and mass transfer limitations (Althuri and Banerjee, 2019; Ailo et al., 2020).
The saccharification process of pretreated sawdust was influenced by substrate-related factors and other physical conditions, and enzyme-related factors were also responsible for affecting the hydrolysis rate positively and negatively. In the current findings, the optimized concentration of crude cellulases (i.e., endoglucanase, exoglucanase, and
The long-chain oligosaccharides (cello-oligosaccharides) are the end product of endoglucanases, which are then processed by exoglucanases, and the resulting disaccharides (cellodextrins/cellobiose) are then cleaved by
The jamming effect was created by the overcrowding of cellobiohydrolases (CBH) in the crystalline cellulose due to the orientation of cellulose fiber and restricted movement of enzymes over it in one direction (i.e., along with the fiber). That is why upon increasing the concentration of cellulases, several molecules bound adjacent to each other over the exposed cellulosic content tend to impede each other. As a result, not all enzymes were able to move at the same pace, causing a reduction in cellulose to glucose conversion rate (Bommarius et al., 2008).
However, further increase in enzyme concentration was not supportive in increasing percentage saccharification probably due to the following reasons: source of cellulases, product inhibition, synergism among cellulases, non-specific binding, and specific activity of enzymes, as well as their processivity and compatibility with the substrate to be saccharified (Yang et al., 2011). However, other reasons were the increased rate of transglycosylation reactions, inadequate mixing, hydrodynamic instability, and slurry suspension (Alrumman, 2016).
The bioethanol production of 11.82% using S. cerevisiae, with a maximum consumption of reducing sugar slurry, was obtained within 72 h. After 72 h, a slight decrease in glucose consumption and its subsequent conversion into ethanol was observed. The decrease might be due to the depletion of essential nutrients supporting yeast growth and the accumulation of toxic metabolites. Alio et al. (2020) reported ethanol production of 16 g/L from saccharified biomass with substrate (sawmill mixed feedstock) loading of 7.5% using S. cerevisiae. However, with DES pretreated sorghum straw, Wu et al. (2021) reported 0.45 g ethanol/g glucose using S. cerevisiae. Thus, variation in results was due to the difference in the substrate, using saccharified biomass slurry of reducing sugar instead of saccharified biomass.
The raw data supporting the conclusion of this article will be made available by the authors without undue reservation.
AN, FJ, RH, and YF conceptualized the study and developed and conducted the research idea. IH and KJ helped in manuscript preparation. AN, FJ, HM, and YF helped in manuscript proofreading and statistical analysis.
The authors acknowledge the financial support of National Innovation and Entrepreneurship Training program for College Students (202213023009) and the Doctoral Scientific Research Foundation of Hangzhou Medical College (0004F1RCYJ1905).
The authors declare that the research was conducted in the absence of any commercial or financial relationships that could be construed as a potential conflict of interest.
All claims expressed in this article are solely those of the authors and do not necessarily represent those of their affiliated organizations or those of the publisher, the editors, and the reviewers. Any product that may be evaluated in this article, or claim that may be made by its manufacturer, is not guaranteed or endorsed by the publisher.
Abdou Alio, M., Marcati, A., Pons, A., and Vial, C. (2021). Modeling and Simulation of a Sawdust Mixture-Based Integrated Biorefinery Plant Producing Bioethanol. Bioresour. Technol. 325, 124650. doi:10.1016/j.biortech.2020.124650
Alio, M. A., Tugui, O.-C., Rusu, L., Pons, A., and Vial, C. (2020). Hydrolysis and Fermentation Steps of a Pretreated Sawmill Mixed Feedstock for Bioethanol Production in a Wood Biorefinery. Bioresour. Technol. 310, 123412. doi:10.1016/j.biortech.2020.123412
Alrumman, S. A. (2016). Enzymatic Saccharification and Fermentation of Cellulosic Date Palm Wastes to Glucose and Lactic Acid. Braz. J. Microbiol. 47 (1), 110–119. doi:10.1016/j.bjm.2015.11.015
Althuri, A., and Banerjee, R. (2019). Separate and Simultaneous Saccharification and Fermentation of a Pretreated Mixture of Lignocellulosic Biomass for Ethanol Production. Biofuels 10 (1), 61–72. doi:10.1080/17597269.2017.1409059
Alvarez-Vasco, C., Ma, R., Quintero, M., Guo, M., Geleynse, S., Ramasamy, K. K., et al. (2016). Unique Low-Molecular-Weight Lignin with High Purity Extracted from Wood by Deep Eutectic Solvents (DES): A Source of Lignin for Valorization. Green Chem. 18, 5133–5141. doi:10.1039/C6GC01007E
Bommarius, A. S., Katona, A., Cheben, S. E., Patel, A. S., Ragauskas, A. J., Knudson, K., et al. (2008). Cellulase Kinetics as a Function of Cellulose Pretreatment. Metab. Eng. 10 (6), 370–381. doi:10.1016/j.ymben.2008.06.008
Capolupo, L., and Faraco, V. (2016). Green Methods of Lignocellulose Pretreatment for Biorefinery Development. Appl. Microbiol. Biotechnol. 100 (22), 9451–9467. doi:10.1007/s00253-016-7884-y
Chavan, S., and Gaikwad, A. (2021). Optimization of Enzymatic Hydrolysis of Bamboo Biomass for Enhanced Saccharification of Cellulose through Taguchi Orthogonal Design. J. Environ. Chem. Eng. 9 (1), 104807. doi:10.1016/j.jece.2020.104807
Chen, Y., and Mu, T. (2019). Application of Deep Eutectic Solvents in Biomass Pretreatment and Conversion. Green Energy & Environ. 4 (2), 95–115. doi:10.1016/j.gee.2019.01.012
Chourasia, V. R., Pandey, A., Pant, K. K., and Henry, R. J. (2021). Improving Enzymatic Digestibility of Sugarcane Bagasse from Different Varieties of Sugarcane Using Deep Eutectic Solvent Pretreatment. Bioresour. Technol. 337, 125480. doi:10.1016/j.biortech.2021.125480
Cunha, J. T., Soares, P. O., Baptista, S. L., Costa, C. E., and Domingues, L. (2020). Engineered Saccharomyces cerevisiae for Lignocellulosic Valorization: a Review and Perspectives on Bioethanol Production. Bioengineered 11 (1), 883–903. doi:10.1080/21655979.2020.1801178
Faizal, A., Sembada, A. A., and Priharto, N. (2021). Production of Bioethanol from Four Species of Duckweeds (Landoltia Punctata, Lemna Aequinoctialis, Spirodela Polyrrhiza, and Wolffia Arrhiza) through Optimization of Saccharification Process and Fermentation with Saccharomyces cerevisiae. Saudi J. Biol. Sci. 28 (1), 294–301. doi:10.1016/j.sjbs.2020.10.002
Gopal, K., and Ranjhan, S. K. (1980). Laboratory Manual for Nutrition Research. India: Roland Press (India) Private Ltd, 56–60.
Gunny, A. A. N., Arbain, D., Daud, M. Z. M., and Jamel, P. (2014). A Synergistic Action of Deep Eutectic Solvents and Cellulases for Lignocellulosic Biomass Hydrolysiss. Mat. Res. Innovat. 18 sup6, S6-65-S6-67. doi:10.1179/1432891714Z.000000000933
Huang, C., Jiang, X., Shen, X., Hu, J., Tang, W., Wu, X., et al. (2022b). Lignin-enzyme Interaction: A Roadblock for Efficient Enzymatic Hydrolysis of Lignocellulosics. Renew. Sustain. Energy Rev. 154, 111822. doi:10.1016/j.rser.2021.111822
Huang, C., Xu, C., Meng, X., Wang, L., and Zhou, X. (2022a). Editorial: Isolation, Modification, and Characterization of the Constituents (Cellulose, Hemicellulose, Lignin, et al.) in Biomass and Their Bio-Based Applications. Front. Bioeng. Biotechnol. 10, 866531. doi:10.3389/fbioe.2022.866531
Irfan, M., Gulsher, M., Abbas, S., Syed, Q., Nadeem, M., and Baig, S. (2011). Effect of Various Pretreatment Conditions on Enzymatic Saccharification. Songklanakarin J. Sci. Technol. 33 (4), 397–404.
Keshav, P. K., Banoth, C., Kethavath, S. N., and Bhukya, B. (2021). Lignocellulosic Ethanol Production from Cotton Stalk: an Overview on Pretreatment, Saccharification and Fermentation Methods for Improved Bioconversion Process. Biomass Convers. biorefin. doi:10.1007/s13399-021-01468-z
Kucharska, K., Słupek, E., Cieśliński, H., and Kamiński, M. (2020). Advantageous Conditions of Saccharification of Lignocellulosic Biomass for Biofuels Generation via Fermentation Processes. Chem. Pap. 74, 1199–1209. doi:10.1007/s11696-019-00960-1
Lin, W., Yang, J., Zheng, Y., Huang, C., and Yong, Q. (2021). Understanding the Effects of Different Residual Lignin Fractions in Acid-Pretreated Bamboo Residues on its Enzymatic Digestibility. Biotechnol. Biofuels 14, 143. doi:10.1186/s13068-021-01994-y
Ling, Z., Guo, Z., Huang, C., Yao, L., and Xu, F. (2020). Deconstruction of Oriented Crystalline Cellulose by Novel Levulinic Acid Based Deep Eutectic Solvents Pretreatment for Improved Enzymatic Accessibility. Bioresour. Technol. 305, 123025. doi:10.1016/j.biortech.2020.123025
Liu, Y., Chen, W., Xia, Q., Guo, B., Wang, Q., Liu, S., et al. (2017). Efficient Cleavage of Lignin-Carbohydrate Complexes and Ultrafast Extraction of Lignin Oligomers from Wood Biomass by Microwave-Assisted Treatment with Deep Eutectic Solvent. ChemSusChem 10 (8), 1692–1700. doi:10.1002/cssc.201601795
Magalhães, S., Filipe, A., Melro, E., Fernandes, C., Vitorino, C., Alves, L., et al. (2021). Lignin Extraction from Waste Pine Sawdust Using a Biomass Derived Binary Solvent System. Polymers 13 (7), 1090. doi:10.3390/polym13071090
Maki, M., Leung, K. T., and Qin, W. (2009). The Prospects of Cellulase-Producing Bacteria for the Bioconversion of Lignocellulosic Biomass. Int. J. Biol. Sci. 5 (5), 500–516. doi:10.7150/ijbs.5.500
Malgas, S., Rose, S. H., van Zyl, W. H., and Pletschke, B. I. (2020). Enzymatic Hydrolysis of Softwood Derived Paper Sludge by an In Vitro Recombinant Cellulase Cocktail for the Production of Fermentable Sugars. Catalysts 10 (7), 775. doi:10.3390/catal10070775
Maugeri, Z., and Domı, P. (2012). RSC Advances Novel Choline-Chloride-Based Deep-Eutectic-Solvents with Renewable Hydrogen Bond Donors: Levulinic Acid and Sugar-Based Polyols. RSC Advanc. 421, C425. doi:10.1039/C1RA00630D
Miller, G. L. (1959). Use of Dinitrosalicylic Acid Reagent for Determination of Reducing Sugar. Anal. Chem. 31 (3), 426–428. doi:10.1021/ac60147a030
Moodley, P., and Gueguim Kana, E. B. (2019). Bioethanol Production from Sugarcane Leaf Waste: Effect of Various Optimized Pretreatments and Fermentation Conditions on Process Kinetics. Biotechnol. Rep. 22, e00329. doi:10.1016/j.btre.2019.e00329
Obeng, E. M., Ongkudon, C. M., Budiman, C., Maas, R., and Jose, J. (2018). An Optimal Blend of Single Autodisplayed Cellulases for Cellulose Saccharification - a Proof of Concept. J. Chem. Technol. Biotechnol. 93 (9), 2719–2728. doi:10.1002/jctb.5628
Robak, K., and Balcerek, M. (2020). Current State-Of-The-Art in Ethanol Production from Lignocellulosic Feedstocks. Microbiol. Res. 240, 126534. doi:10.1016/j.micres.2020.126534
Satlewal, A., Agrawal, R., Bhagia, S., Sangoro, J., and Ragauskas, A. J. (2018). Natural Deep Eutectic Solvents for Lignocellulosic Biomass Pretreatment: Recent Developments, Challenges and Novel Opportunities. Biotechnol. Adv. 36 (8), 2032–2050. doi:10.1016/j.biotechadv.2018.08.009
Scelsi, E., Angelini, A., and Pastore, C. (2021). Deep Eutectic Solvents for the Valorisation of Lignocellulosic Biomasses towards Fine Chemicals. Biomass 1, 29–59. doi:10.3390/biomass1010003
Sidar, A., Albuquerque, E. D., Voshol, G. P., Ram, A. F. J., Vijgenboom, E., and Punt, P. J. (2020). Carbohydrate Binding Modules: Diversity of Domain Architecture in Amylases and Cellulases from Filamentous Microorganisms. Front. Bioeng. Biotechnol. 8, 871–5206. doi:10.3389/fbioe.2020.00871
Smink, D., Juan, A., Schuur, B., and Kersten, S. R. A. (2019). Understanding the Role of Choline Chloride in Deep Eutectic Solvents Used for Biomass Delignification. Ind. Eng. Chem. Res. 58 (36), 16348–16357. doi:10.1021/acs.iecr.9b03588
Smith, E. L., Abbott, A. P., and Ryder, K. S. (2014). Deep Eutectic Solvents (DESs) and Their Applications. Chem. Rev. 114 (21), 11060–11082. doi:10.1021/cr300162p
Soares, B., Tavares, D. J. P., Amaral, J. L., Silvestre, A. J. D., Freire, C. S. R., and Coutinho, J. A. P. (2017). Enhanced Solubility of Lignin Monomeric Model Compounds and Technical Lignins in Aqueous Solutions of Deep Eutectic Solvents. ACS Sustain. Chem. Eng. 5 (5), 4056–4065. doi:10.1021/acssuschemeng.7b00053
Sridevi, A., Narasimha, G., Ramanjaneyulu, G., Dileepkumar, K., Reddy, B. R., and Devi, P. S. (2015). Saccharification of Pretreated Sawdust by Aspergillus niger Cellulase. 3 Biotech. 5 (6), 883–892. doi:10.1007/s13205-015-0284-7
Takada, M., Chandra, R., Wu, J., and Saddler, J. N. (2020). The Influence of Lignin on the Effectiveness of Using a Chemithermomechanical Pulping Based Process to Pretreat Softwood Chips and Pellets Prior to Enzymatic Hydrolysis. Bioresour. Technol. 302, 122895. doi:10.1016/j.biortech.2020.122895
Vallander, L., and Eriksson, K.-E. (1987). Enzyme Recirculation in Saccharification of Lignocellulosic Materials. Enzyme Microb. Technol. 9 (12), 714–720. doi:10.1016/0141-0229(87)90030-5
Van Soest, P., and Robertson, J. (1979). “Systems of Analysis for Evaluating Fibrous Feeds in Standardization of Analytical Methodology for Feeds,” in Proceedings IDRC, Ottawa, ON, Canada, March 12–14, 1979 (IDRC).
Vasić, K., Knez, Ž., and Leitgeb, M. (2021). Bioethanol Production by Enzymatic Hydrolysis from Different Lignocellulosic Sources. Molecules 26 (3), 753. doi:10.3390/molecules26030753
Vieira, S., Barros, M. V., Sydney, A. C. N., Piekarski, C. M., de Francisco, A. C., Vandenberghe, L. P. D. S., et al. (2020). Sustainability of Sugarcane Lignocellulosic Biomass Pretreatment for the Production of Bioethanol. Bioresour. Technol. 299, 122635. doi:10.1016/j.biortech.2019.122635
Wang, H., Squina, F., Segato, F., Mort, A., Lee, D., Pappan, K., et al. (2011). High-Temperature Enzymatic Breakdown of Cellulose. Appl. Environ. Microbiol. 77 15, 5199–5206. doi:10.1128/AEM.00199-11
Wang, Z. H., Li, X., Lin, T., Tang, J., Chen, J., Mo, R., et al. (2020). Bioresource Technology Novel Recyclable Deep Eutectic Solvent Boost Biomass Ppretreatment for Enzymatic Hydrolysis. Bioresour. Technol. 307, 123237. doi:10.1016/j.biortech.2020.123237
Wu, M., Gong, L., Ma, C., and He, Y.-C. (2021). Enhanced Enzymatic Saccharification of Sorghum Straw by Effective Delignification via Combined Pretreatment with Alkali Extraction and Deep Eutectic Solvent Soaking. Bioresour. Technol. 340, 125695. doi:10.1016/j.biortech.2021.125695
Xu, H., Kong, Y., Peng, J., Song, X., Liu, Y., Su, Z., et al. (2021). Comprehensive Analysis of Important Parameters of Choline Chloride-Based Deep Eutectic Solvent Pretreatment of Lignocellulosic Biomass. Bioresour. Technol. 319, 124209. doi:10.1016/j.biortech.2020.124209
Xu, H., Peng, J., Kong, Y., Liu, Y., Su, Z., Li, B., et al. (2020). Key Process Parameters for Deep Eutectic Solvents Pretreatment of Lignocellulosic Biomass Materials: A Review. Bioresour. Technol. 310, 123416. doi:10.1016/j.biortech.2020.123416
Yang, B., Dai, Z., Ding, S.-Y., and Wyman, C. E. (2011). Enzymatic Hydrolysis of Cellulosic Biomass. Biofuels 2 (4), 421–449. doi:10.4155/bfs.11.116
Yuan, D., Rao, K., Relue, P., and Varanasi, S. (2011). Fermentation of Biomass Sugars to Ethanol Using Native Industrial Yeast Strains. Bioresour. Technol. 102 (3), 3246–3253. doi:10.1016/j.biortech.2010.11.034
Zhang, H., Lang, J., Lan, P., Yang, H., Lu, J., and Wang, Z. (2020). Study on the Dissolution Mechanism of Cellulose by ChCl-Based Deep Eutectic Solvents. Materials 13 (2), 278. doi:10.3390/ma13020278
Keywords: deep eutectic solvent, enzymatic saccharification, green biorefinery, levulinic acid, ionic liquids, bioethanol
Citation: Nawaz A, Huang R, Junaid F, Feng Y, Haq IU, Mukhtar H and Jiang K (2022) Sustainable Production of Bioethanol Using Levulinic Acid Pretreated Sawdust. Front. Bioeng. Biotechnol. 10:937838. doi: 10.3389/fbioe.2022.937838
Received: 06 May 2022; Accepted: 20 May 2022;
Published: 30 June 2022.
Edited by:
Caoxing Huang, Nanjing Forestry University, ChinaReviewed by:
Xiaojun Shen, Dalian Institute of Chemical Physics (CAS), ChinaCopyright © 2022 Nawaz, Huang, Junaid, Feng, Haq, Mukhtar and Jiang. This is an open-access article distributed under the terms of the Creative Commons Attribution License (CC BY). The use, distribution or reproduction in other forums is permitted, provided the original author(s) and the copyright owner(s) are credited and that the original publication in this journal is cited, in accordance with accepted academic practice. No use, distribution or reproduction is permitted which does not comply with these terms.
*Correspondence: Ali Nawaz, YWxpLm5hd2F6QGdjdS5lZHUucGs=; Kankan Jiang, amlhbmdrYW5rYW5AMTI2LmNvbQ==
†These authors have contributed equally to this work
Disclaimer: All claims expressed in this article are solely those of the authors and do not necessarily represent those of their affiliated organizations, or those of the publisher, the editors and the reviewers. Any product that may be evaluated in this article or claim that may be made by its manufacturer is not guaranteed or endorsed by the publisher.
Research integrity at Frontiers
Learn more about the work of our research integrity team to safeguard the quality of each article we publish.