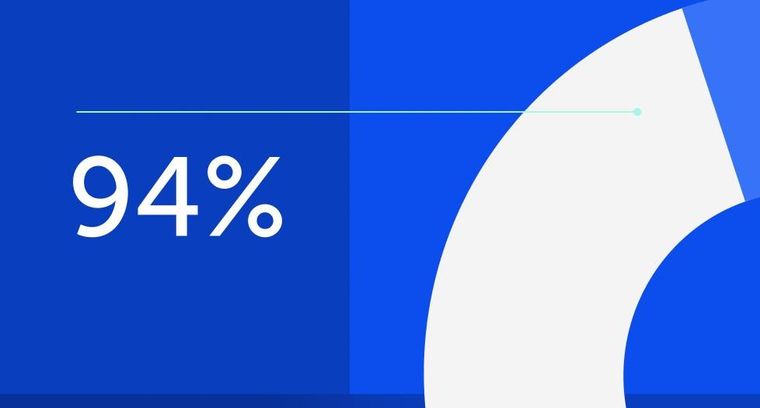
94% of researchers rate our articles as excellent or good
Learn more about the work of our research integrity team to safeguard the quality of each article we publish.
Find out more
REVIEW article
Front. Bioeng. Biotechnol., 25 August 2022
Sec. Biomechanics
Volume 10 - 2022 | https://doi.org/10.3389/fbioe.2022.937803
This article is part of the Research TopicBiomechanical and Biochemical Regulation of the Musculoskeletal SystemView all 21 articles
Abstract: Although bone tissue has the ability to heal itself, beyond a certain point, bone defects cannot rebuild themselves, and the challenge is how to promote bone tissue regeneration. Iron oxide nanoparticles (IONPs) are a magnetic material because of their excellent properties, which enable them to play an active role in bone regeneration. This paper reviews the application of IONPs in bone tissue regeneration in recent years, and outlines the mechanisms of IONPs in bone tissue regeneration in detail based on the physicochemical properties, structural characteristics and safety of IONPs. In addition, a bibliometric approach has been used to analyze the hot spots and trends in the field in order to identify future directions. The results demonstrate that IONPs are increasingly being investigated in bone regeneration, from the initial use as magnetic resonance imaging (MRI) contrast agents to later drug delivery vehicles, cell labeling, and now in combination with stem cells (SCs) composite scaffolds. In conclusion, based on the current research and development trends, it is more inclined to be used in bone tissue engineering, scaffolds, and composite scaffolds.
Orthopedic diseases are the second leading cause of disability worldwide, as living conditions improve and people live longer, skeletal diseases are becoming more frequent, and their incidence is increasing every year (Rasker, 1995; Jakob et al., 2013; Weinstein, 2016). Among them, bone defects caused by trauma, tumors, chronic inflammation, infection, osteoporosis and congenital deformities caused great physical, and emotional damage to patients and diminished their quality of life (Gao et al., 2022; Li et al., 2017; Migliorini et al., 2021; Li et al., 2022b). Bone is a living organ that can heal and remodel itself. Nonetheless, extensive defects of bone and cartilage require the necessary interventions. For osteochondral defects that are not self-repairing, autologous or allogeneic osteochondral grafts are currently used as the primary means. However, this has the disadvantages of infection, immune rejection, inflammation, and disease transmission, and these limitations have severely restricted their widespread use in clinical practice (Tang et al., 1998; Kowalczewski and Saul, 2018; Zhang et al., 2019c; Yao et al., 2019; Koons et al., 2020; Xu et al., 2021). Particularly for those with serious irregularities in bone and cartilage, their rational repair and reconstruction can be interesting and challenging (Fazzalari, 2011; Tevlin et al., 2014; Ho-Shui-Ling et al., 2018).
In recent years, magnetic nanoparticles (MNPs) have been widely used in medical research, such as magnetic resonance imaging (MRI), cell labeling, targeted drug delivery, antitumor, thermal therapy, and biosensing (Ferreira et al., 2016). Compared with other materials, MNPs have unique advantages, including low production cost, good biocompatibility, stable physicochemical properties, and low toxicity (Ozbey et al., 2015), and consequently, they are receiving increasing attention in the biomedical field. The magnetic materials currently most used in the medical field are based on iron oxide (IO), such as ferric oxide (Fe2O3) and ferroferric oxide (Fe3O4) (Ramimoghadam et al., 2014; Shokrollahi, 2017). Iron is an essential trace element in the body, and exogenous IO can be metabolized and degraded through various pathways when it enters the body, and it is relatively non-toxic (Xia et al., 2019c; Liu et al., 2020a). Because IO is more common in nature, easy to synthesise and particularly sensitive to magnetic fields, it has been researched more frequently (Paik et al., 2015; Salehiabar et al., 2018). And mainly due to the mobility in response to magnetic fields (Kitture and Ghosh, 2019), iron oxide nanoparticles (IONPs) have been approved by the FDA and are the most widely studied material in the field of nanomedicine (Bobo et al., 2016). However, due to the easy aggregation of bare iron and the potential toxicity caused by the release of iron ions, most of them are coated with biocompatible ligands, which can affect cell proliferation, adhesion, migration, differentiation, movement, and distribution under the action of an external magnetic field (Vangijzegem et al., 2019). IONPs can be visualized by MRI and can also promote cell differentiation, inhibit osteoclast formation, and enhance osteogenesis (Li et al., 2018a; Xia et al., 2018b; Liu et al., 2018). Overall, IONPs have a strong ability to promote bone regeneration, good biocompatibility and chemical stability, as well as no significant intrinsic toxicity, which is superior to other magnetic nanoparticles, and are widely used in bone regeneration therapy (Paik et al., 2015; Li et al., 2018a; Xia et al., 2018b; Salehiabar et al., 2018).
Consequently, this article summarizes some of the achievements and progress in the use of IONPs in bone regeneration in recent years and provides a summary and perspective of the research hotspots and trends. Specifically, the characteristics of IONPs and their application in bone repair, mechanism studies, and safety were discussed. Furthermore, this article adopts a bibliometric approach to analyze and discuss the hot spots and research trends. Through the above research, it is hoped that this contribution will guide the study of bone regeneration (Figure 1).
FIGURE 1. General diagram of the core content of the article. This paper contains six main contents, which are respectively bone regeneration mechanisms, iron oxide nanoparticles and bone regeneration, application of iron oxide nanoparticles in bone regeneration, application of iron oxide nanoparticles in pathological bone defects, mechanism of iron oxide nanoparticles in bone regeneration, and hot spots and trends in iron oxide nanoparticles for bone regeneration.
The skeleton is a vital organ that supports the body and remains under stress and weight for the whole life (Zhu et al., 2021). Usually, bone resorption and destruction is a dynamically balanced metabolic process. In the micro-ecological environment of bone, various cells are involved, including a large number of osteocytes, osteoclasts, and immune cells (Mohammadi et al., 2018). Osteocytes and osteoblasts play a key role in bone formation, while osteoclasts are involved in the resorption and regeneration of bone tissue (Torgbo and Sukyai, 2018). In addition, type I collagen, the inorganic minerals calcium (Ca2+), and phosphate (PO43-) are still important components of bone structure (Jang et al., 2009). Physiologically, it has a certain degree of self-healing when minor bone defects or bone destruction occurs; however, this ability is limited, especially in the case of trauma, infections, and tumours that result in bone defects worth more than 2 cm (Yang et al., 2018; Zhang W. et al., 2019a; Wagner et al., 2019). More importantly, bone defects can also lead to bone ischaemia, bone loss, and osteonecrosis, which further lead to a failure of bone healing (Loi et al., 2016). When a bone defect cannot heal itself, additional means are essential to achieve the goal of stimulating bone regeneration. How to maximize the optimal repair of bone defects has been a hot topic. The current methods used for bone regeneration consist of five main components depending on the pathogenesis of the bone injury (Figure 2): 1) external stimulation, 2) cells, 3) blood vessels, 4) biomolecules, and 5) biomaterials (Saiz et al., 2013; Hankenson et al., 2015; Lopes et al., 2018). In current studies, several methods are used in combination to enhance the effectiveness of the therapy. External stimulation such as magnetic fields promote bone regeneration (Singh et al., 2014; Zhuang et al., 2018); cellular therapy is currently focused on the use of MSCs (Jia et al., 2019); vascular regeneration is essential to ensure cellular metabolism and nutrition (Xia et al., 2018b); biomolecules include growth factors and bone morphogenetic proteins (Yu et al., 2020); and biomaterials are currently an important modality in the treatment of bone defects, and the development of various scaffolds has extended the effect of osteogenesis. For the study of scaffolds, various requirements need to be met, of which biocompatibility, absorbability, mechanical properties, non-toxic side effects, and bubble structure are the most important, as shown in Table 1.
FIGURE 2. Main schematic diagram of bone regeneration. There are five ways to promote bone repair after bone injury, which are external stimulation, cells, blood vessels, biomolecules, and biomaterials.
In response to the mechanisms of bone regeneration, current research focuses on promoting bone healing through the modification of external conditions. There are various methods of bone regeneration, but in order to enhance the effect of bone growth, a combination of methods is usually applied, and this is most noticeable in the case of biological scaffolds. If biomolecules, cells, and IONPs are mixed in a scaffold, it retains all the advantages of a scaffold but also has molecular, cellular, and nanoparticle-related characteristics (Ou et al., 2019; Yang et al., 2019; Petretta et al., 2021). Although some results have been reached, the search for the design of scaffolds with ideal loading molecules and cells with good biological effects, suitable mechanical properties, optimized bubble structures with controlled degradability, and non-toxic to tissues are still the direction being pursued.
Among the various nanoparticles, magnetic materials are more attractive to researchers because of their unique responsiveness to magnetic fields (Dhivya et al., 2015). Currently, magnetic materials commonly used in the biomedical field include iron, cobalt, titanium, and nickel metal alloys as well as IO, ferrite (BaFe12O19, CoFe2O4), and nano-magnetic hydroxyapatite, among which magnetic IONPs are the most widely studied because iron is an essential element for the human body and can be sensitive to magnetic fields (Li et al., 2016). It can be combined with growth factors, stem cells (SCs), drugs, and other biologics so that be labeled, imaged, thermally treated, and disease targeted (Ghosh et al., 2015; Accomasso et al., 2016; Rajan et al., 2020). The biomedical applications of IONPs are mainly based on their superparamagnetic properties. Superparamagnetic iron oxide nanoparticles (SPIONs) are a type of IONPs with a diameter of 10–100 nm and can generate strong magnetism in a weak magnetic field, and its magnetism can disappear with the withdrawal of the external magnetic field (Laurent et al., 2008; Kircher et al., 2011). Based on its superparamagnetic properties, IONPs are usually used in MRI examinations and targeted to specific areas under the action of an external magnetic field to achieve targeted therapeutic effects (Radeloff et al., 2020). Maria et al. (Volokhova et al. 2022) made SiO2-coated magnetic iron oxide nanoparticles into α-Fe@SiO2 with a cubic morphology, which exhibited higher MRI relaxation than iron oxide alone and could show high-performance contrast effects. Zheng et al. (2022) found that allantoin phosphate can deliver iron oxide nanoparticles precisely to bone tissue for targeted synergistic treatment of osteoporosis. At present, the preparation of IONPs primarily consists of dry and wet methods (Yu et al., 2016; Huang et al., 2018) (Figure 3). The wet methods are more commonly used and include thermal decomposition, chemical co-precipitation, sol-gel, ball milling and atomic layer deposition (Cotin et al., 2022; Kiyani et al., 2022). The co-precipitation method is more commonly used in the synthesis of MNPs and allows for better control of the size and magnetic properties of the material, but the MNPs have a tendency to aggregate due to the small particle size (Lin et al., 2017). The high-temperature pyrolysis method is mainly used to synthesis ultra-fine powders and crystals of different materials, but it cannot obtain NPs smaller than 10 nm in size (Bhavani et al., 2017). Therefore, different extraction methods have their advantages and disadvantages, and researchers should select the appropriate method for generating IONPs according to the specific situation.
FIGURE 3. Methods for the preparation of IONPs. (A) Wet method preparation process of CTS-g-PTMAAC/SC-Fe3O4 composite magnetic nanoparticles (Chen et al., 2016); (B) Images of IONPs prepared by immunoprecipitation technique under scanning electron microscope (SEM), (a) 5 nm, (b) 20 nm (Mengesha et al., 2022); (C) IONPs obtained by thermal decomposition (Cotin et al., 2022); (D) Preparation of IONPs by co-precipitation method in microchannel reactor (Lin et al., 2017); (E) Preparation of high saturation magnetic IO nanomaterials (Bhavani et al., 2017).
The physicochemical properties of IONPs include magnetic properties, chemical stability, modifiability, biocompatibility and ease of surface functionalization (Clark et al., 2005) (Figure 4). In particular, the magnetic properties make it multifunctional. IONPs have long been used for MRI contrast for its uniquely magnetic and highly biocompatible (Zhao et al., 2022). Its good biocompatibility allows it to label specific cells without causing damage (Moise et al., 2017). With their biocompatible, magnetic, small size and customised surface coating properties, SPIONs can be used for the delivery and monitoring of small molecules, drugs and cells, particularly in areas of muscle, bone or cartilage (Iyer et al., 2017).
FIGURE 4. Physicochemical properties of iron oxide nanoparticles. (A) IONPs are used for MRI examination due to their good magnetic properties and biocompatibility (Zhao et al., 2022); (B) IONPs are readily absorbed by cells and can label cells for cell tracking (Iyer et al., 2017); (C) IONPs have magnetic heating properties and can substantially promote tumor cell death in vitro (Iacoviță et al., 2021); (D) IONPs are used for targeted magnetic drug delivery (Hola et al., 2015); (E) CSLM images showing MG-63 cancer cell line stained alive/dead at 41°C (Khodaei et al., 2022); (F) IO-OA/PLGA produces mechanical stimulation in the presence of an external magnetic field and promotes osteogenic differentiation of MC3T3-E1 cells (Hao et al., 2019).
Magnetism is the most important property of IONPs. SPION can sparingly deliver drugs to specific areas under the action of an external magnetic field and can undergo magnetothermal transformation to kill tumour cells which ultimately treats tumours (Iacoviță et al., 2021; Pucci et al., 2022). Additionally, magnetic materials can have mechanical properties that can regulate cell behaviour and promote bone regeneration (Hao et al., 2019). The small particle size of IONPs allow them to overcome the biological barrier for wide distribution in the body, and they can also be excreted from the body by themselves (Hola et al., 2015). Studies have indicated that very small particles with hydrodynamic diameters up to 5 nm–8 nm can be excreted from the kidneys; while larger IONPs are readily captured by the reticuloendothelial system (RES), allowing imaging of the liver and spleen and detection of areas of inflammation; it ranging in size from about 20 to 150 nm may also accumulate in the stomach, bones and kidneys (Dadfar et al., 2019). Thus, the particle nature of the iron oxide nanoparticles is also an important characteristic to ensure its functionality.
IONPs consist primarily of an IO core, a coating, and an external modification layer (Figure 5). The core is a large amount of IO, which ensures that it has a certain magnetism. Uncovered IO is toxic because it tends to collect and react chemically, so an organic or inorganic coating needs to be applied to the surface of the core, which reduces IO oxidation, toxicity, and enhances its biocompatibility (Dadfar et al., 2019). Current coating materials include organic, inorganic, and organic-inorganic composites (Ma et al., 2013). Organic coatings include mainly dextran, chitosan, citrate, agarose, collagen, polylactic acid (PLA), polycaprolactone (PCL), polyglycolic acid (PGA), and polylactic acid-glycolic acid copolymer (PLGA) (Iyer et al., 2017; Marin et al., 2020); the inorganic coating consists mainly of silica, calcium phosphate, calcium silicate, and calcium phosphate cement complexes (Ferrage et al., 2017); organic-inorganic composites such as mineralized collagen, which retain the common properties of both while giving the scaffold good rigidity and toughness (Li et al., 2021b). The main purpose of the coating is generally to restrict particle aggregation and provide hydrophilicity, stability, and biocompatibility to the internal core (Accomasso et al., 2016; Harrison et al., 2017). In order to bind specifically to the receptor, molecular modifications can be applied outside the coating, or the therapeutic agent can be loaded so that the combination can be used for specific targeted therapy (Li et al., 2012).
FIGURE 5. Structure of IONPs. IONPs consist primarily of an IO core, a coating and an external modification layer (This diagram is drawn from (Dasari et al., 2022)).
One of the first things to ensure when IONPs are used for bone regeneration is safety (Figure 6). Studies have indicated that IONPs have superior biocompatibility and stability without significant intrinsic toxicity (Salehiabar et al., 2018). Most studies have demonstrated that low doses of IONPs are non-toxic to organisms (Pariti et al., 2014). In general, the toxicity of nanoparticles depends on their physical and chemical properties, such as particle size, surface properties, and chemical composition (Huang et al., 2017b). Therefore, before evaluating the toxicity of IONPs in vitro and in vivo, their physicochemical properties must be clarified. Various nanoparticles exhibit size-dependent toxicity in vitro and in vivo (Wu et al., 2022a). Under normal conditions, nanoparticles smaller than 10 nm are eliminated by the kidneys, while nanoparticles larger than 200 nm are phagocytosed (Rajan et al., 2020). The majority of IONPs are engulfed by hepatocytes, which on the one hand, facilitates their usefulness in liver imaging and, on the other hand, is a potential hazard to the body (Daldrup-Link, 2017). Although IONPs can stay in the body for a long time, they are biodegradable, can be metabolised from the circulation through a variety of pathways (Ventola, 2012). However, some IONPs are still non-degradable and it is not known whether there are serious effects on the body. It is also considered that IONPs can induce some toxic side effects in the body. IONPs have been found to cause significant toxicity in rats when kept in the body for long periods, causing reduced body weight, significant liver damage, and mild splenic toxicity (Verma et al., 2021). The interaction of the surface charge of IONPs between nanoparticles and biological components also substantially affects their toxic response (Woo et al., 2021). Toxic reactions are mainly manifested by impaired mitochondrial activity, membrane leakage, and morphological changes that negatively affect cell viability, proliferation, and metabolic activity, significantly weakening its therapeutic efficiency (Yang et al., 2011). Nanoparticle-treated cells may trigger immune-inflammatory reactions and other side effects if they migrate in tissues or accumulate at specific sites over time (Mahmoudi et al., 2010; Mahmoudi et al., 2012). IONPs were discovered to cause reversible damage to the reproductive system of male mice without affecting major organs (Yang et al., 2022). There are some solutions to these risks. For example, IONPs may damage neural tissue through free iron accumulation, protein aggregation, and oxidative stress, but quercetin may reduce neurotoxicity in clinical applications (Bardestani et al., 2021). The effect of IONPs on the inflammatory response is size-dependent, with smaller IONPs weakening the inflammatory response; this inflammatory response can also be attenuated by blocking actin polymerization, endoplasmic reticulum (ER) stress, or oxidative stress (Ying et al., 2022). New predictive models have also been developed as a method of toxicity screening to provide a safety profile for the clinical use of IONPs. Coccini et al. (2019) have developed a model for assessing human stem cells-based Fe3O4 NP toxicity screening and support using this approach to improve the safety of NPs to predict health outcomes with confidence. Hence, when utilizing IONPs, it is essential to understand their physicochemical properties. IO itself is toxic, but by choosing a suitable surface coating, reducing the dosage, reducing the diameter of the particles, and avoiding long-term aggregation in the same location, the toxic reaction can be effectively reduced. Measures should be taken in advance for different side effects, and extracts of some herbs can be used in synergy with IONPs to reduce toxicity. Models for predicting toxicity and safety assessment are also necessary for the rational use of nanoparticles.
FIGURE 6. Safety of IONPs. (A) MTS assay showed that different concentrations of Au-Fe3O4 nanoparticles were not significantly toxic to CHO cells (Pariti et al., 2014); (B) USPIO could induce an immune response (Daldrup-Link, 2017); (C) Flow cytometry assay showed that SPIO was incubated directly on hMSCs for a long time (72 h) and had no significant effect on ROS(Yang et al., 2011); (D) IONPs can cause reversible damage to the reproductive system of male mice without affecting major organs (Yang et al., 2022); (E) Quercetin attenuates the neurotoxicity induced by IONPs(Bardestani et al., 2021).
IONPs, as a special magnetic particle, have a multifaceted effect on bone regeneration. Its function of promoting bone regeneration is closely related to its physicochemical properties. Among them, magnetism is an important prerequisite for the action of IONPs on bone regeneration. It has been demonstrated that IONPs not only respond to external magnetic fields, but also act as a magnetic domain themselves on the nanoscale, with nanoparticles forming magnetic fields to generate a number of biochemical reactions (Xie et al., 2019). When an external magnetic field is applied to bone defects, it promotes fracture healing and tissue repair, even without the intervention of IONPs(Shan et al., 2013; Thrivikraman et al., 2014). When IONPs are used to treat bone defects, a magnetic field is applied externally, which further enhances their magnetic effect, and this magnetism stimulates osteoblast-associated cells, activating the osteogenic pathway and exerting a bone regeneration effect (Jia et al., 2019). In addition, mechanical stimulation was found to promote osteogenic differentiation of stem cells (Meng et al., 2021). IONPs are mechanically stimulated by the effect of a magnetic field, which acts on the cells to generate internal biochemical signals and enhance bone regeneration (Wang et al., 2016; Zhuang et al., 2018). Furthermore, IONPs can generate magnetothermal conversion with the intervention of an alternating magnetic field (AMF), which is delivered to deep lesions to generate heat for the treatment of bone tumours and post-operative bone defects (Guo et al., 2017; Lee et al., 2018; Albarqi et al., 2019). The production of blood vessels is still essential for the regeneration of bone tissue. IONPs were identified to improve angiogenic properties and promote the formation of new blood vessels in bone defects, which was present in the presence or absence of static magnetic field (SMF) intervention (Singh et al., 2014; Xia et al., 2018b; Hu et al., 2018). It can be absorbed by cells through endocytosis (Kolosnjaj-Tabi et al., 2013), so it is used for cell tracking and targeting (Zhang and Zhang, 2005; Shen et al., 2013). This can detect the location of cells in bone regeneration and migration of SCs(Liao et al., 2022). Cells labelled with these nanoparticles are non-toxic and easily reach the desired effect. Note that for those IONPs that are smaller in size and have a longer half-life in the blood stream, it has significant bone targeting properties (Arami et al., 2015). In particular, it can remove reactive oxygen species from the body to regulate bone metabolism and improve post-menopausal bone loss (Yu et al., 2020). It has also been found that IONPs can inhibit osteoclast production and differentiation (Zheng et al., 2022). Its multifaceted action promotes the occurrence of bone regeneration.
The multifaceted properties of IONPs have led to a wide range of research and applications in bone regeneration. The main directions of application include direct action (Figure 7), action on stem cells (differentiation, migration, homing, tracking) (Figure 8), targeted drug delivery, combined use with scaffolds, and composite scaffolds.
FIGURE 7. Mechanical stimulation of IONPs. (A) Mechanical activation of IONPs stimulates cellular receptors (Sniadecki, 2010); (B) Fe3O4/mineralized collagen coating promotes osteogenic differentiation of MC3T3-E1 cells (Zhuang et al., 2018).
FIGURE 8. Role of IONPs on stem cells. (A) Promotion of stem cell migration (Zhao et al., 2021); (B) stem cell labeling (Mehta, 2022); (C) promotion of stem cell homing (Li et al., 2019); (D) promotion of stem cell differentiation (Jiang et al., 2016).
IONPs have a direct effect on cells. Various physical forces can affect cell activation, and studies have demonstrated that magnetic and mechanical stimulation can promote SCs proliferation and differentiation (Sniadecki, 2010; Schwartz et al., 2018). Magnetic forces can be converted into mechanical forces, and when cells are mechanically stimulated, they can generate a series of biochemical signals that induce a biological response to the organism (Ingber, 2006; Martino et al., 2018). Research has revealed that a composite coating formed by IONPs with a mineralized collagen coating under a static magnetic field can promote osteogenic differentiation through mechanical stimulation (Zhuang et al., 2018). Studies have also indicated that SIONPs themselves can strengthen bone regeneration without external magnetic fields (Hu et al., 2018). Proven research has also been conducted on the effects of the state of nanoparticles on cells, finding that direct delivery of colloidal ferromagnetic fluids is more desirable than the administration of powdered particles (Uskoković et al., 2019). Evidently, IONPs can stimulate cells directly with or without external magnetic field intervention and promote osteogenesis.
SCs are an important tool in the treatment of bone defects. It holds great promise in treating many diseases and conditions (Daneshmandi et al., 2017; Česen Mazič et al., 2018). SCs are a particularly important group of cells in bone tissue engineering, which can differentiate into different cells under certain conditions. SCs are primarily derived from bone marrow, adipose tissue, muscle, umbilical cord, cord blood, placenta, Wharton’s glue, and amniotic fluid, all of which can differentiate into osteoblasts, of which the most studied are bone marrow mesenchymal stem cells (BMSCs) (Kangari et al., 2020). When bone damage occurs, MSCs migrate from the bone marrow or other adjacent tissues to the site of injury and secrete growth factors such as BMP and VEGF to promote the bone healing process (Schreivogel et al., 2019; Wasnik et al., 2019; McNeill et al., 2020). At the same time, MSCs are also involved in regulating inflammation and repairing bone damage together with progenitor cells, stromal cells and macrophages (Grayson et al., 2015; Xue et al., 2022; Yin et al., 2023). MSCs are also capable of differentiating into bone tissue and have favourable immunomodulatory properties (Safarova et al., 2020).
In recent years, SCs therapy has provided a strategy for bone regeneration, particularly in repairing large bone defects (Watanabe et al., 2016). As Hao et al. (2022) constructed microgels that can effectively promote the adhesion, proliferation, and osteogenesis of BMSCs, providing a new idea for repairing large bone defects. Liu et al. (Liu et al., 2022) also designed functional spheroids containing MSCs to create a 3D cell-directed microenvironment for the bone repair of large defects, resulting in excellent osteogenesis, angiogenesis, and bone regeneration of MSCs. For cells expanded in vitro, a stable cell phenotype should be maintained, targeting SCs to specific sites, promoting differentiation and proliferation of SCs to osteoblasts at the site of injury, maintaining local cell stability, and reducing cell loss at the defect site, all of which are necessary for bone defect repair.
The differentiation of SCs is influenced by many factors, of which magnetic conditions are an important one. Ruchita et al. (Shelat et al., 2020) used L-lysine-functionalized IONPs to interfere with bone marrow mesenchymal stem cells (BMSCs) and showed efficacy in promoting in situ cartilage regeneration and its ability to label cells and act as a contrast agent. Driven by a magnetic field, carbon quantum dot (CD)-doped SPION can also differentiate MSCs into bone and cartilage for osteochondral regeneration (Das et al., 2019). Uptake of bovine serum albumin (BSA) (Fe3O4/BSA) particles loaded with IONPs also significantly enhanced osteogenic differentiation of MSCs under a constant static magnetic field (Jiang et al., 2016). Low-temperature atmospheric nitrogen plasma positively affects the behaviour of MSCs cultured on bone scaffolds containing IO-loaded silica nanoparticle catalysts, improving SCs behavior and promoting the value-added and osteogenic differentiation (Przekora et al., 2020). IONPs can directly promote the differentiation of SCs. However, current research tends to package and combine IONPs to strengthen this impact.
SCs labeled by IOPNs can migrate and home to specific sites in response to magnetic fields. It has been disclosed that the presence of SPIONs confers special magnetic properties to PLGA microspheres, which greatly promote the proliferation, migration, and osteogenic differentiation of BMSCs under the influence of external magnetic fields (Zhao et al., 2021). Moreover, the combination of pulsed electromagnetic fields (PEMF) and SPIONs were synergistic role in promoting the directional migration and osteogenic differentiation of BMSCs (Wu et al., 2018). A further finding was that silica-encapsulated IONPs promoted migration while retaining the proliferation and differentiation capacity of BMSCs and that the labeled BMSCs were more viable and migrated better, but their osteogenic potential was not affected (Yao et al., 2020). In vitro magnetic targeting system was used to attract rabbit BMSCs. This technique significantly facilitated the penetration of IONPs-labelled cells into porous hydroxyapatite ceramics transplanted into rabbit ulnar bone defects and promoted bone formation (Chen et al., 2010). IONPs enhance the homing potential of MSCs to the site of injury and have no negative impact on MSCs function; furthermore, MSCs-loaded nanoparticles exhibit good homing and anti-inflammatory capacity in the absence of external magnetic field intervention (Li et al., 2019). The combination of IONPs with MSCs has special magnetic effects and pro-osteogenic differentiation potential, which indicates the great ability of the two to merge for bone regeneration.
IONPs can be used as nuclear magnetic resonance (NMR) contrast agents for cell tracking (Hua et al., 2015). Currently, the commonly used MRI contrast agent is gadolinium, but its toxicity has raised the level of concern. When searching for an effective alternative without significant toxic effects, IONPs-labelled MSCs were found to show positive results both in vitro and in vivo (Mehta, 2022). Similarly, in a sheep tendinitis model treated with MSCs labeled with IONPs, cells were still detectable in MRI 7 days after surgery (Scharf et al., 2015). The magnetic effect mediated by IONPs is a crucial tool for SCs tracking and a wide range of applications. It has great potential as a magnetic particle imaging (MPI) tracer advancing SCs therapy (Wang et al., 2020).
Targeted drug delivery is an important feature of IONPs (Figure 9), and IONPs complexes are commonly used as carriers for controlled drug release (Prodan et al., 2013). For instance, IONPs have been found to stimulate the corresponding drug delivery systems for targeted drug delivery in arthritis (Zhang et al., 2022). To enhance the anti-osteoporosis effect, the investigators synergized IONPs with alendronate, and the combination substantially improved bone mineral density and microarchitecture compared to the same dose of alendronate (Zheng et al., 2022). Merging bisphosphonate (Bis) and IONPs and incorporating them into osteoblasts, this composite magnetic nanoparticle has the significant anti-osteoporotic potential (Lee et al., 2016). Guo et al. (2021) constructed a PLGA-based drug delivery system, co-loaded with 17β-estradiol (E2) and iron oxide (Fe3O4) and modified with alendronate for the remote targeting of estradiol in the treatment of post-ovariectomy osteoporosis in rats, which achieved good efficacy and magnetically remote targeted drug release. Besides, IONPs can be loaded with various cytokines, genes, and targets to reach the site of bone defects for the purpose of targeting and promoting bone regeneration. Jiang et al. (Chiang et al., 2018) developed magnetic gelatin nanocapsules containing transforming growth factor (TGF)-β1 consisting of hexanoic anhydride grafted gelatin and IONPs and achieved magnetic enrichment of loaded cells through combined treatment with magnetically induced stimulation and TGF-β1. Fan et al. (2014) developed a magnetic IONPs-encapsulated biopolymer nanogel consisting of chitosan and heparin via specific nucleobase pairing for Bone morphogenetic protein 2 (BMP-2) delivery, which plays an important role in cartilage and bone regeneration. For this purpose, Sahmani et al. (2020) generated a specific biomimetic scaffold with drug delivery capacity, a porous scaffold for biomedical use, prepared from hydroxyapatite and IONPs and loaded with gelatin, in which ibuprofen was incorporated to achieve excellent pain relief at the specified site. Researchers (Wang et al., 2021) have developed IONPs containing HA and raloxifene (R-IONPs-HA), which exhibit superior biocompatibility, antibacterial activity, and osteoinduction, and have great potential for fracture repair and infection prevention. Specific composite platforms are also being investigated for targeted drug delivery (Pistone et al., 2014). It is evident that the targeted delivery of IONPs is being refined and matured, and multiple compound platforms loaded with drugs have been investigated, showing a good ability to promote osteochondral regeneration.
FIGURE 9. IONPs can be used for drug delivery. (A) Delivery of alendronate to osteoporosis (Zheng et al., 2022); (B) Remote controlled bone-targeted delivery of estradiol to treat ovariectomy-induced osteoporosis in rats (Guo et al., 2021).
In bone tissue engineering exploration, scaffolds are the most used modality because they can retain specific structural and mechanical properties while loading other factors, particles, and materials, as well as being biocompatible and offering outstanding advantages in maintaining the normal physiological structure of bone and maintaining good weight-bearing and force-bearing functions. The design of delivery platforms plays a crucial role in the activation of bone regeneration as they provide a suitable environment for cell adhesion and growth while providing a beneficial platform for delivery strategies (Bi et al., 2020). Simple biodegradable polymeric materials or ceramics have been investigated as bone tissue engineering scaffolds, but these materials have significant limitations, including insufficient mechanical strength. In contrast, nanoparticle-modified composite scaffolds offer significant promise for promoting bone regeneration. The nanomaterials incorporated into polymeric scaffolds are divided into organic and materials, and they can provide sufficient surface area and mechanical properties required for support, as well as cell adhesion, differentiation, and integration (Kim and Fisher, 2007). The scaffolds currently in use mainly consist of organic materials (lipids, liposomes, dendrimers and polymers, chitosan, gelatin, collagen) or inorganic nano-biomaterials (silica, bioceramics, bioglass, hydroxyapatite) fused (Navarro et al., 2008; Virlan et al., 2016; Kumar et al., 2020). Xia et al. (2018a) mixes IONPs with calcium phosphate cement to create a scaffold that promotes osteoinduction and bone regeneration, adheres to SCs, and promotes osteogenic differentiation. In contrast, thermoplastic polyurethane (TPU) and PLA polymers doped with IONPs exhibited significant osteogenic differentiation of MSCs in response to external magnetic fields (Marycz et al., 2020). Hydrogels have excellent biocompatibility and adjustable mechanical properties, promising biomaterials for replacing extracellular matrix (ECM) and organ regeneration (Hu et al., 2015; Huang et al., 2017a; Xue et al., 2019). Han et al. (Han et al., 2021b) developed a tunable hydrogel for the 3D culture of dental pulp stem cells (DPSCs), through which DPSCs could aggregate and generate spheroids enriched with CD146+ cell subpopulations, which exhibited significant osteogenic differentiation in response to the intervention of IONPs.
The mainstream methods currently used to synthesize scaffolds containing IONPs are electrospinning techniques, freeze drying and three-dimensional (3D) printing (Figure 10).
FIGURE 10. Three common methods for constructing scaffolds for IONPs. (A) Electrostatic spinning technique (Khalili et al., 2022); (B) freeze-drying technique (Zhang W. et al., 2019b); and (C) 3D printing technique (Shokouhimehr et al., 2021).
Electrospinning technology is a well-established scaffold fabrication method with many critical advantages, including its simple nature, high surface area to volume ratio, potential to release drugs and antimicrobial agents, controlled fiber diameter, high porosity, and permeability (Mortimer and Wright, 2017). To promote the fruitful differentiation of MSCs, a researcher prepared magnetic polycaprolactone (PCL) nanofiber scaffolds by adding third-generation dendrimer-modified SPIONs (G3-SPIONs) during electrospinning, which demonstrated promising results with favorable cytocompatibility and cell adhesion (Khalili et al., 2022). Although electrospinning technology is simple to handle and easy to control the surface properties of the support, it still has the disadvantage of low yield and low strength (Singh et al., 2014; Li et al., 2018b).
The freeze-drying technique contains both freezing and drying steps and is one of the most commonly used methods to enhance the stability of scaffolds (Rahman et al., 2010; Hassanajili et al., 2019). It is currently used as a common method for producing scaffolds in bone regeneration research. Freeze-dried scaffolds have a unique structure with the required porosity and pore interconnections for the selective movement of small molecules and closely resemble the natural bone extracellular matrix, making them optimal for bone tissue engineering studies (Singh and Dasgupta, 2022). Lu et al. (Lu et al., 2020) successfully prepared gadolinium-hyaluronic acid (Gd-HA) nanoparticles (NPs) by a simple freeze-drying method as a novel MRI contrast agent for the accurate detection of cartilage damage without toxic side effects. Wu Zhang et al. (2019b) used a combination of freeze-drying monthly physical mixing methods to prepare ultra-small superparamagnetic iron oxide (USPOI)-labeled hydroxyapatite (HA)/silk fibroin (SF) scaffolds loaded with dental pulp stem cells (DPSCs), which were physically stable and significantly promoted pulp tissue repair and regeneration. Azam et al. (Hajinasab et al., 2018) also applied freeze-drying methods to fabricate a 3D nanocomposite scaffold consisting of gelatin and hydroxyapatite (GEL/HA) loaded with Fe3O4 nanoparticles, which has great potential for drug loading and bone replacement. The flexibility of the freeze-drying technique can be seen to provide important support for the study of bone regeneration scaffolds.
3D printing methods allow the precise, reproducible, and large-scale manufacture of complex scaffold systems with tunable structural and physicomechanical properties (Filardo et al., 2019; Serpooshan and Guvendiren, 2020). 3D printing technology for bone tissue engineering also allows for specific geometric design of defective bone areas (Trombetta et al., 2017; Tomov et al., 2019). The manipulation of computer-aided design (CAD) models for printing scaffolds permits precise adjustment of the porosity, size, and geometric design of the graft to closely correspond to the target bone defect (Moreno Madrid et al., 2019). 3D printing technology offers good stability, 3D spatial structure, and high efficiency, but materials are more restricted and expensive (Zhao et al., 2014). Shokouhimehr et al. (2021) 3D printed a new generation of hyperelastic bone (HB) implants loaded with SPIONs applying a bio-ink consisting primarily of HA to scaffolds and studied their therapeutic effect on large non-healing fractures, demonstrating great potential for bone regeneration. The three scaffold fabrication methods and components are shown in Table 2.
Tissue engineering is a method of reconstructing functional tissue at the site of damage, usually including cells, growth factors, and 3D biodegradable scaffolds. With the addition of IONPs to the tissue project, it will contain four main components (Figure 11). Its complex construction is of great importance in promoting bone regeneration. In order to strengthen the effectiveness of the bone repair, extend the synergistic effect and reduce the deficiencies possessed by a single scaffold, researchers are now primarily developing composite scaffolds to reach the desired goal (Figure 12). Researchers combined aminopropyltriethoxysilane (APTES)-modified nanohydroxyapatite (nHAp) with IONPs to develop composites that significantly enhanced osteoblast activity and reduced inflammatory responses and osteoclastogenesis (Marycz et al., 2022). By coating core-shell structured HA on SPIONs (SPIO@HA), Li et al. (Li M. et al., 2021) discovered it could target osteoclastogenesis and osteogenesis. A multifunctional scaffold of filamentous protein/hydroxyapatite scaffold combined with ultra-small SPIONs (USPIO) doped with BMSCs promotes osteogenic differentiation of SCs and allows for osteogenic differentiation non-invasive monitoring of bone regeneration by quantitative MRI (Liu Q. et al., 2020). Moreover, oleic acid-modified IONPs (IO-OA NPs) and PLGA were used to produce homogeneous magnetic nanocomposites, which showed good mechanical properties and enhanced osteogenic differentiation (Hao et al., 2019). In addition, calcium phosphate cement (CPC) scaffolds doped with IONPs on SCs utilizing an external static magnetic field (SMF) have great osteoinductivity, which may be related to the physical forces generated by the magnetic field and the cell-intrinsic action of the magnetic nanoparticles released from the scaffold (Xia et al., 2019a). The relevant modification of the surface of IONPs and their subsequent application to composite scaffolds is also a way of extending their efficacy. Kartogenin (KGN), an emerging stable non-protein compound that promotes the differentiation of BMSCs into chondrocytes, was transplanted onto the surface of modified USPIO and then made into a fibrin nanocrystal/dextran hydrogel, which showed enhanced cartilage regeneration (Yang et al., 2019).
FIGURE 11. Components of tissue engineering in IONPs. When IONPs are applied to tissue engineering, this body has four main components: cells, molecules, IO and scaffold.
FIGURE 12. Application of composite scaffold in bone regeneration. IONPs are mostly found in the form of scaffolds in the treatment of bone defects. To enhance their efficacy, scaffolds can contain a variety of components that promote osteogenic differentiation of stem cells under the influence of a magnetic field.
Bone tissue-engineered scaffolds alone have many drawbacks, including lack of osteoinduction and low mechanical strength. To circumvent these limitations, the option of composite scaffolds is necessary. Hu et al. (Hu et al., 2021) used a poly (lactic acid-ethanolic acid copolymer)/polycaprolactone/β-tricalcium phosphate (PPT) scaffold, incorporating IONPs into a porous electromimetic scaffold for long-term release, and this composite scaffold was optimized to further enhance osteogenic capacity. The low mechanical strength of the scaffold can be regulated by various preparation techniques, of which electrostatic spinning is one of the most applied (Schiffman and Schauer, 2008; Jun et al., 2018). Chen et al. (2018) reported magnetic PLGA/PCL scaffolds made by electrostatic spinning technology and layer-by-layer assembly of SPIONs, which exhibit excellent biocompatibility, adjustable stiffness, physical sensing, and stimulus responsiveness. Estévez et al. (2022) were the first to combine type I collagen and SPIONs to design magnetic and biocompatible electrostatic spinning scaffolds, showing that the electrostatic spinning structures containing SPIONs could retain the magnetic properties of the nanoparticles alone and that these collagen scaffolds could promote the proliferation, adhesion, and viability of osteoblasts and HBMSCs.
3D printing technology for composite scaffolds is another commonly used method whose versatility makes it increasingly important in bone regeneration. The study found that IONPs-rich PLGA copolymer scaffolds prepared through the layer-by-layer assembly by 3D printing technology promoted cell adhesion and enhanced osteogenesis of rat BMSCs (Han L. et al., 2021). Saraiva’s group (Saraiva et al., 2021) used 3D printing to create a polylactic acid platform loaded with HA, IONPs, and an antibiotic (minocycline) with tunable properties and a multi-stimulus response. The platform was discovered to regulate the anti-biofilm dose and strengthen the ability of bone tissue regeneration. Utilizing 3D printing technology, porous titanium aluminium vanadium (Ti6Al4V, pTi) scaffolds can reconstruct large bone defects (especially in load-bearing areas). Nevertheless, the lack of osteogenic properties limits its use in clinical practice. To address this dilemma, a researcher co-deposited IONPs and polydopamine (PDA) on the surface of 3D printed pTi scaffolds, a novel magnetic coating that improved the adhesion, proliferation, and osteogenic differentiation of HBMSCs (Huang et al., 2020). Wang et al. (2018) synthesized biodegradable shape memory polyurethane (PU) as the main component of a 3D printing ink for manufacturing bone scaffolds and added SPIONPs and polyethylene oxide (PEO) or gelatin to the 3D printing ink to promote osteogenic induction, shape fixation and increase printability. This composite scaffold with its shape memory properties, biodegradability, and osteogenic effect, has the potential to become a bone substitute. Petretta et al. (2021) prepared a polycaprolactone-based (PCL) scaffold with HA and SPIONs (PCL-HAp-1% SPION) by 3D printing to improve the healing efficiency of bone and inoculated the scaffold with MSCs.
The construction of composite scaffolds gives new ideas for bone regeneration, while the addition of drug loading after the platform has been established can achieve certain therapeutic effects for specific bone diseases. As Schneider et al. (Schneider et al., 2021) used citric acid (MG@CA) coated magnetic iron oxide nanoparticles (MNPs) as a raw material for the treatment of bone diseases to construct a magnetic nanoplatform, adding raloxifene (Ral), curcumin (Cur), and methylene blue (MB) for the symptomatic treatment of bone diseases.
Mild bone injuries have some ability to heal themselves, but beyond a certain point, bone defects usually fail to reconstruct themselves. For this type of bone defect that does not heal on its own, pathological bone defects such as osteoporosis (Bai et al., 2020; Li et al., 2022a; Li et al., 2022b), rheumatoid arthritis (Liu et al., 2021), infections (Taylor and Webster, 2011) and tumors (Kesse et al., 2020) are the main causes. In contrast, IONPs have been extensively investigated in pathological bone defects. Several studies have demonstrated that IONPs can positively regulate bone metabolism in vitro and in vivo, and that they have favorable bone targeting, bone regeneration and biocompatibility properties. For instance, Zheng et al. (2022) revealed that IONPs can alleviate osteoporosis induced by ovariectomized mice (OVX) by scavenging reactive oxygen species, promoting osteogenic differentiation of BMSCs and inhibiting osteoclastogenesis in vitro. Krzysztof et al. (Marycz et al., 2022) combined aminopropyltriethoxysilane (APTES) modified nHAp with IO nanoparticles and demonstrated in vitro that this biomaterial promotes osteoblast viability and RUNX-2 expression, reduces osteoclast metabolism and inflammation, and can be used for healing of osteoporotic fractures. Another study produced a SPIO with a core-shell structure coated with HA (SPIO@HA), which was used to target osteoclastogenesis and osteogenesis. The results revealed that the material greatly prevented bone loss in OVX mice and that their mechanism may be related to TRAF6-p62-CYLD, TGF-β, PI3K-AKT and calcium signalling pathways (Li et al., 2021a). Moreover, IONPs can be used for drug delivery in the presence of magnetic fields, bone targeting for osteoporosis (Guo et al., 2021).
Rheumatoid arthritis (RA) is a systemic immune disease that is primarily characterised by joint inflammation accompanied by progressive bone and joint destruction, which can cause significant bone defects (Takeuchi et al., 2021). Magnetic nanoparticles, particularly IONPs, have been documented to be an important tool for the treatment and diagnosis of rheumatoid arthritis by targeting local lesions with additional magnetic fields and avoiding systemic side effects (Liu et al., 2021). IONPs are an effective nanoplatform for targeted drug delivery, stimulus-responsive drug release, MRI diagnostics, photothermal therapy, and magnetothermal therapy in RA treatment, and could also contribute to the development of new magnetic materials (Wu and Shen, 2019). Duan et al., (2014) synthesized polyethyleneimine-functionalized superparamagnetic iron oxide nanoparticles (PEI-SPION) in vitro and demonstrated through in vivo and in vitro experiments that PEI-SPION can be used for systemic siRNA delivery to strengthen the therapeutic effect of RA with the intervention of an external magnetic field. Furthermore, Carneiro et al. (2020) constructed gold-coated SPIO (AuSPION) for the treatment of arthritic rats by intraperitoneal injection and found that AuSPION inhibited joint inflammation and edema, redox imbalance and cytokine expression under the influence of an external magnetic field.
Infection is another major challenge for bone regeneration, but this has been made viable with the intervention of nanotechnology. Studies have indicated that SPIONs have good antimicrobial activity, magnetic properties, and bone regeneration properties, which could be used in the development of new drugs for the treatment of bone defects associated with orthopaedic infections (Taylor and Webster, 2011). Wang et al. (2021) used IONPs (R-IONPs-HA) containing HA and raloxifene for fracture repair and infection prevention which showed good biocompatibility, antibacterial activity and osteoinductive effects. Taylor et al. (Taylor and Webster, 2009) demonstrated in an in vitro study that SPION significantly inhibited the growth of Staphylococcus epidermidis and at the same concentration significantly enhanced osteoblast function, offering hope for bone repair after infection. Wei et al. (2021) synthesized IONPs-sHA-strontium@collagen (IONSs-HA-SR@C) using a co-precipitation method and evaluated its effect on the differentiation and proliferation of MC3T3-E1 cells and their morphological changes as well as its antibacterial activity by in vitro experiments. The results clearly indicate that IONSs-HA-SR@C has promising biocompatibility, bone growth promoting ability and antibacterial activity. It is evident that IONPS has great potential for bone repair and anti-infection.
The incorporation of IONPs into multifunctional biomaterials for the treatment of tumors and post-tumour bone defects has led to a number of advances and breakthroughs due to their thermal, magnetic targeting, bone healing and pro-tumour cell apoptosis properties. Pang et al. (2021) synthesized a bone-targeted SPIO that, when delivered to bone, inhibits flavoproteinase, thereby inhibiting bone resorption by osteoclasts and invasion by breast cancer cells, effectively protecting against breast cancer-induced osteolysis. Khodaei et al. (2022) developed a method for the delivery of curcumin by SPIONs-based magnetothermal nanocarriers, in which the release of curcumin was coordinated by temperature. In vitro results showed that the temperature could be stabilised at 41°C by alternating magnetic fields, and that curcumin and heat therapy could kill MG-63 osteosarcoma cells and achieve good anti-bone tumour effects. Not only that, IONPs can promote bone regeneration for the repair of bone defects after tumour surgery. Sasikala et al. (2022) developed a piezoelectric magnetic nanoparticle (PMNP) that can regulate cell behaviour and fate under ultrasound (US), magnetic field (MF) and light-responsive conditions to achieve superior bone regeneration and bone transformation after bone tumour surgery. Vergnaud et al. (2022) designed superparamagnetic bioactive nanoparticles with an IO core (γ-Fe2O3) wrapped in a bioactive glass (SiO2-CaO) shell, which demonstrated in vitro experiments its non-toxic, bone healing and thermal therapeutic properties for direct or combined scaffolding of bone defects after bone tumour resection. It is apparent that IONPs have a great platform and opportunity in the treatment of pathological bone defects and have now gained great traction.
The rational use of IONPs can better promote bone regeneration. Previous studies have focused on the construction of scaffolds, the selection of composite materials, and the integrated use of SCs and drugs but neglected their specific mechanisms. In recent years, with the development of molecular biology, the study of the mechanism has gradually applied to the field of materials; the results found that IONPs promote bone regeneration with a variety of factors and signaling pathways related (Figure 13). Proteomic analysis revealed that magnetic iron oxide/polydopamine coating (Fe3O4/PDA) promotes osteogenesis by upregulating the TGFβ-Smads pathway in a static magnetic field (Huang et al., 2020). Heat therapy is beneficial in stimulating bone regeneration. Wang et al. (2022) constructed nanoparticle-hydrogel composites by embedding Arg-Gly-Asp (RGD)-coated core-shell structured magnetic iron oxide nanoparticles (MION) (CoFe2O4@MnFe2O4) in agarose with significant magnetothermal effects. Under mild magnetothermal treatment, the expression of HSP90 was upregulated in preosteoblasts and endothelial cells, activating the PI3K/Akt pathway in preosteoblasts, thus significantly promoting osteogenesis and biomineralization; meanwhile, the cobalt in CoFe2O4@MnFe2O4 upregulated the expression of the angiogenesis-related gene HIF-1 α, further promoting the formation of new blood vessels at the injury site. Zhuang et al. (2018) incorporated IONPs into mineralized collagen coatings (MC) to promote osteogenic differentiation by initiating mechanotransduction pathways and upregulating integrin α1, integrin β1 and RhoA under static magnetic fields. Jia et al. (2019) synthesized mesoporous silica-coated magnetic (Fe3O4) nanoparticles (M-MSN), which exhibited excellent biocompatibility and promoted osteogenic differentiation of MSCs in vitro via the Wnt/β-catenin pathway. In healing osteoporosis, IONPs can inhibit osteoclastogenesis by modulating the TRAF6-p62-CYLD signaling complex (Liu et al., 2020a). Yu et al. (2020) synthesized Fe2O3@PSC nanoparticles using the biopolysaccharide-based antioxidant polyglucose-sorbitol-carboxymethyl ether (PSC) as a precursor and found that it scavenged reactive oxygen species from MC3T3-E1 and Raw 264. cells by activating the Akt-GSK-3β-β-catenin pathway, inhibiting MAPK and NF-κB pathways ultimately promoting osteoblast differentiation. IONPs, in combination with SCs or biomaterials, will exhibit multiple functions. It was discovered to promote osteogenic differentiation of hBMSCs via the MAPK pathway (Wang et al., 2016). Jiang et al. (2016) prepared bovine serum albumin (BSA) particles loaded with IONPs (Fe3O4/BSA) and found that they significantly promoted the expression of alkaline phosphatase, type I collagen, and osteocalcin at the mRNA and protein levels in MSCs under static magnetic fields. The microbiota influences the process of bone repair. By constructing a rat palatal bone model, Jia et al. (2021) found that 3D printed SPION/PLGA could have a significant antibacterial effect and could alter the oral microbiota while treating palatal bone defects. Lu et al. (2018) prepared magnetic SrFe12O19 nanoparticle-modified mesoporous bioglass (BG)/chitosan (CS) porous scaffolds (MBCS) with excellent bone regeneration and anti-tumor functions. The magnetic field generated by MBCS can promote the expression of osteogenic genes such as OCN, COL1, Runx2, and ALP by activating the BMP-2/Smad/Runx2 pathway; it can also perform photothermal conversion to promote apoptosis of tumour cells, so it is used for tumor-associated bone defects. The combination of IONPs, MSCs, and biomaterials to build a composite system is the mainstay of bone regeneration research, and some breakthroughs have been made in their mechanisms. Duan et al. (2020) constructed a human umbilical cord mesenchymal stem cell-super magnetic iron oxide nanoparticles (NPs)@polydopamine (SCIOPs) system, which was found to inhibit osteoblast apoptosis, enhance osteoblast proliferation and promote bone repair through the Akt/Bcl-2/Bad/caspase-3 signaling pathway in the presence of a magnetic field and SCs homing ability. Xia et al. (2019b) added IONPs powder to CPC powder to fabricate CPC-IONP scaffolds and investigated the effect of the novel composite on bone matrix formation and osteogenesis of human dental pulp stem cells (hDPSCs). The results indicate that the osteogenic behavior of hDPSCs may be driven by CPC-IONP via the WNT signaling pathway. The mechanism by which IONPs promote bone repair has also been explored from the perspective of RNA. It was found to upregulate lncRNA INZEB2, regulating ZB2 expression and the BMP/Smads pathway (Wang et al., 2017). As nanomaterials are increasingly used in bone regeneration, it is essential to understand their biological effects (Table 3). The ongoing exploration of their molecular mechanisms may provide a theoretical basis and biological evidence for regenerative medicine.
FIGURE 13. Mechanism of IONPs in the treatment of bone defects. (A) In the action of SMF, the Fe3O4/PDA@pTi scaffold regulates cellular function via the TGFβ-Smads signaling pathway (Huang et al., 2020); (B) IONPs promote osteogenic differentiation of HBMSCs through the MAPK pathway when they enter the cells (Wang et al., 2016); (C) IONPs + CPC can promote osteogenic differentiation of HDPSCs through the WNT signalling pathway (Xia et al., 2019b); (D) IONPs promote osteogenic differentiation of MSCs through the INZEB2 and BMP/Smads signaling pathways (Wang et al., 2017).
A bibliometric approach was employed to investigate the hotspots and trends in IONPs on bone regeneration. We searched the Web of science core database for 631 relevant primary literature and, after limiting the language of the literature to English, eventually obtained 629 publications for inclusion in the study. The literature was imported into the Carrot2 clustering analysis tool to identify the current mainstream research directions. A burst analysis of the involved literature was also carried out using Citespace software to identify hot spots and trends in recent years. The results obtained from the Carrot2 analysis revealed that the main directions of research clustering are Mesenchymal Stem Cells, Magnetic Cells, Cells Labeled, Human, Superparamagnetic Iron Oxide, and Scaffolds (Figure 14). Burst analysis disclosed that IONPs were initially applied as magnetic resonance imaging contrast agents and transfection agents and then steadily moved towards magnetic resonance and in vivo tracking. After incorporating stem cells into the field, IONPs tend to be focused on migration, cell labeling, transplantation, and drug delivery. The increasing use of biomaterials has led to the development of IONPs from in vivo tracking to in vitro studies. Recent years have seen a shift towards the scaffold, bone tissue engineering, osteogenic differentiation, hydroxyapatite, and composite scaffold (Figure 15). The results of the thematic clustering reveal that the research literature in this area focuses on three main blocks of research: stem cell research, cell marker research, and research on scaffolds. Based on the analysis of the strongest citation bursts of keywords, the development of this study can be divided into three main periods. In the early stages, research in this field concentrated on MRI and diagnostic aspects of disease; in the middle stages, it focused on the labeling of SCs and in vivo tracking; the current hotspots and trends are in scaffold research, regenerative tissue engineering of bone and osteogenesis, and the transformation of the scaffold from a single component at the beginning to a composite scaffold. It is worth mentioning that HA is the main inorganic component of bone and its excellent biocompatibility, osteoconductivity and osteoinductivity, binding similarity, and high porosity make it an important area of interest for bone regeneration research (Ramesh et al., 2018; Gomes et al., 2019). Its effects are primarily in the coating of IO, the construction of scaffolds, and the delivery of drugs (Khajuria et al., 2018). However, HA has certain drawbacks, as its fragility reduces the mechanical properties of the material, and its demonstrated difficulty in modification makes the nanoparticles prone to agglomeration (Jegatheeswaran and Sundrarajan, 2015; Alaribe et al., 2016). To address these issues, nHAp can be combined with polymers to strengthen their mechanical properties, biocompatibility, and biofunctionability, eventually improving overall regeneration (Song et al., 2016).
FIGURE 14. Pie chart of topic clustering in carrot2. The main directions of research clustering are Mesenchymal Stem Cells, Magnetic Cells, Cells Labeled, Human, Superparamagnetic Iron Oxide, and Scaffolds.
FIGURE 15. Top 25 keywords with the strongest citation bursts. This research focuses on three main blocks of research: stem cell research, cell marker research, and research on scaffolds. Based on the analysis of the strongest citation bursts of keywords, the development of this study can be divided into three main periods. In the early stages, research in this field concentrated on MRI and diagnostic aspects of disease; in the middle stages, it focused on the labeling of SCs and in vivo tracking; the current hotspots and trends are in scaffold research, regenerative tissue engineering of bone and osteogenesis, and the transformation of the scaffold from a single component at the beginning to a composite scaffold.
Although bone has the potential to heal itself, larger bone defects are irreversible; it is a serve health problem and how to promote bone regeneration remains a clinical challenge. IONPs are an important member of the nanoparticle class because their magnetic properties give them unique advantages in bone repair, including stimulating osteogenic differentiation, cell labeling, drug delivery, molecular activation, and SCs homing. The powerful effect of IONPs is inseparable from their coating. The external coating reduces the toxicity of IO and improves the overall biocompatibility and mechanical properties of the IONPs. Additionally, the external modification of the coating with cytokines, drugs, and targets can also give IONPs a targeted therapeutic effect. The bone regenerative effect of IONPs is also influenced by external magnetic fields, which can be remotely controlled to induce various biological responses. IONPs are now regularly used in combination with SCs and scaffolds to achieve good bone regeneration results. It promotes migration, adsorption, homing, labeling, and osteogenic differentiation of SCs and confers a magnetic effect on the scaffold. In composite scaffolds, good biocompatibility, mechanical properties, resorbability, osteoconductivity, and osteoinductivity are essential for bone regeneration. Although some scholars have initiated to explore the specific mechanisms by which IONPs treat bone defects, the interactions between magnetic fields, cells, and nanoparticles in composite scaffolds are still not sufficiently understood. Although basic research has identified the crucial role of bone morphogenetic proteins, vascular endothelial growth factor, osteocalcin, and collagen in bone regeneration, there is still a need to further explore and elucidate their therapeutic mechanisms. The bibliometric analysis also identified hotspots and trends in promoting bone regeneration by IONPs from MRI, cell labeling to in vitro experiments, composite scaffolds, bone tissue engineering, and hydroxyapatite. Later studies can further experiment with innovations in tissue engineering based on HA combined with IONPs, and adding other organic or inorganic material components, adding cytokines or pharmaceutical components to better accomplish the purpose of bone regeneration. It is worth mentioning that although IONPs are relatively safe for use in bone regeneration, their toxicity is influenced by some factors, and specific toxicological studies and refined interpretation protocols are areas that we will need to explore further at a later stage.
NW and YX: conceptualization, methodology, investigation, writing-original draft. ZX and ZM: conceptualization, datum analysis. RD and XL: writing-review and editing. XL and RK: Writing and revising, supervision.
This work was funded by the Natural Science Foundation of Jiangsu Province (BK20220464), National Natural Science Foundation of China (81772356), Project of National Clinical Research Base of Traditional Chinese Medicine in Jiangsu Province (JD2022SZXMS07) and Jiangsu Province Cadre Health Care Project (BJ19030). We sincerely thank Mr Zhanpeng Yan of the clinical research room of integrated traditional Chinese and Western medicine in the Affiliated Hospital of Integrated Traditional Chinese and Western Medicine, Nanjing University of Chinese Medicine for his guidance and help in writing this article.
We sincerely thank Mr Zhanpeng Yan of the clinical research room of integrated traditional Chinese and Western medicine in the Affiliated Hospital of Integrated Traditional Chinese and Western Medicine, Nanjing University of Chinese Medicine for his guidance and help in writing this article.
The authors declare that the research was conducted in the absence of any commercial or financial relationships that could be construed as a potential conflict of interest.
All claims expressed in this article are solely those of the authors and do not necessarily represent those of their affiliated organizations, or those of the publisher, the editors and the reviewers. Any product that may be evaluated in this article, or claim that may be made by its manufacturer, is not guaranteed or endorsed by the publisher.
Accomasso, L., Gallina, C., Turinetto, V., and Giachino, C. (2016). Stem cell tracking with nanoparticles for regenerative medicine purposes: An overview. Stem Cells Int. 2016, 1–23. doi:10.1155/2016/7920358
Alaribe, F. N., Manoto, S. L., and Motaung, S. (2016). Scaffolds from biomaterials: Advantages and limitations in bone and tissue engineering. Biologia 71 (4), 353–366. doi:10.1515/biolog-2016-0056
Albarqi, H. A., Wong, L. H., Schumann, C., Sabei, F. Y., Korzun, T., Li, X. N., et al. (2019). Biocompatible nanoclusters with high heating efficiency for systemically delivered magnetic hyperthermia. Acs Nano 13 (6), 6383–6395. doi:10.1021/acsnano.8b06542
Arami, H., Khandhar, A., Liggitt, D., and Krishnan, K. M. (2015). In vivo delivery, pharmacokinetics, biodistribution and toxicity of iron oxide nanoparticles. Chem. Soc. Rev. 44 (23), 8576–8607. doi:10.1039/c5cs00541h
Bai, H. T., Zhao, Y., Wang, C. Y., Wang, Z. H., Wang, J. C., Liu, H., et al. (2020). Enhanced osseointegration of three-dimensional supramolecular bioactive interface through osteoporotic microenvironment regulation. Theranostics 10 (11), 4779–4794. doi:10.7150/thno.43736
Bardestani, A., Ebrahimpour, S., Esmaeili, A., and Esmaeili, A. (2021). Quercetin attenuates neurotoxicity induced by iron oxide nanoparticles. J. Nanobiotechnology 19 (1), 327. doi:10.1186/s12951-021-01059-0
Bhavani, P., Rajababu, C. H., Arif, M. D., Reddy, I. V. S., and Reddy, N. R. (2017). Synthesis of high saturation magnetic iron oxide nanomaterials via low temperature hydrothermal method. J. Magnetism Magnetic Mater. 426, 459–466. doi:10.1016/j.jmmm.2016.09.049
Bi, X., Li, L., Mao, Z., Liu, B., Yang, L., He, W., et al. (2020). The effects of silk layer-by-layer surface modification on the mechanical and structural retention of extracellular matrix scaffolds. Biomater. Sci. 8 (14), 4026–4038. doi:10.1039/d0bm00448k
Bobo, D., Robinson, K. J., Islam, J., Thurecht, K. J., and Corrie, S. R. (2016). Nanoparticle-based medicines: A review of FDA-approved materials and clinical trials to date. Pharm. Res. 33 (10), 2373–2387. doi:10.1007/s11095-016-1958-5
Carneiro, M. F. H., Machado, A. R. T., Antunes, L. M. G., Souza, T. E., Freitas, V. A., Oliveira, L. C. A., et al. (2020). Gold-coated superparamagnetic iron oxide nanoparticles attenuate collagen-induced arthritis after magnetic targeting. Biol. Trace Elem. Res. 194 (2), 502–513. doi:10.1007/s12011-019-01799-z
Česen Mazič, M., Girandon, L., Kneževič, M., Avčin, S. L., and Jazbec, J. (2018). Treatment of severe steroid-refractory acute-graft-vs.-host disease with mesenchymal stem cells-single center experience. Front. Bioeng. Biotechnol. 6, 93. doi:10.3389/fbioe.2018.00093
Chen, H., Sun, J., Wang, Z., Zhou, Y., Lou, Z., Chen, B., et al. (2018). Magnetic cell-scaffold interface constructed by superparamagnetic IONP enhanced osteogenesis of adipose-derived stem cells. ACS Appl. Mat. Interfaces 10 (51), 44279–44289. doi:10.1021/acsami.8b17427
Chen, S., Chen, X., Geng, Z., and Su, J. (2022). The horizon of bone organoid: A perspective on construction and application. Bioact. Mat. 18, 15–25. doi:10.1016/j.bioactmat.2022.01.048
Chen, Y., Bose, A., and Bothun, G. D. (2010). Controlled release from bilayer-decorated magnetoliposomes via electromagnetic heating. ACS Nano 4 (6), 3215–3221. doi:10.1021/nn100274v
Chen, Y., Geng, J. Q., Zhuang, Y. X., Zhao, J., Chu, L. Q., Luo, X. X., et al. (2016). Preparation of the chitosan grafted poly (quaternary ammonium)/Fe3O4 nanoparticles and its adsorption performance for food yellow 3. Carbohydr. Polym. 152, 327–336. doi:10.1016/j.carbpol.2016.06.114
Chiang, C. S., Chen, J. Y., Chiang, M. Y., Hou, K. T., Li, W. M., Chang, S. J., et al. (2018). Using the interplay of magnetic guidance and controlled TGF-β release from protein-based nanocapsules to stimulate chondrogenesis. Int. J. Nanomedicine 13, 3177–3188. doi:10.2147/ijn.S156284
Clark, S. M., Prilliman, S. G., Erdonmez, C. K., and Alivisatos, A. P. (2005). Size dependence of the pressure-induced gamma to alpha structural phase transition in iron oxide nanocrystals. Nanotechnology 16 (12), 2813–2818. doi:10.1088/0957-4484/16/12/013
Coccini, T., De Simone, U., Roccio, M., Croce, S., Lenta, E., Zecca, M., et al. (2019). In vitro toxicity screening of magnetite nanoparticles by applying mesenchymal stem cells derived from human umbilical cord lining. J. Appl. Toxicol. 39 (9), 1320–1336. doi:10.1002/jat.3819
Cotin, G., Heinrich, B., Perton, F., Kiefer, C., Francius, G., Mertz, D., et al. (2022). A confinement-driven nucleation mechanism of metal oxide nanoparticles obtained via thermal decomposition in organic media. Small 18, e2200414. doi:10.1002/smll.202200414
Dadfar, S. M., Roemhild, K., Drude, N. I., von Stillfried, S., Knüchel, R., Kiessling, F., et al. (2019). Iron oxide nanoparticles: Diagnostic, therapeutic and theranostic applications. Adv. Drug Deliv. Rev. 138, 302–325. doi:10.1016/j.addr.2019.01.005
Daldrup-Link, H. E. (2017). Ten things you might not know about iron oxide nanoparticles. Radiology 284 (3), 616–629. doi:10.1148/radiol.2017162759
Daneshmandi, S., Karimi, M. H., and Pourfathollah, A. A. (2017). TGF-β engineered mesenchymal stem cells (TGF-β/MSCs) for treatment of Type 1 diabetes (T1D) mice model. Int. Immunopharmacol. 44, 191–196. doi:10.1016/j.intimp.2017.01.019
Das, B., Girigoswami, A., Dutta, A., Pal, P., Dutta, J., Dadhich, P., et al. (2019). Carbon nanodots doped super-paramagnetic iron oxide nanoparticles for multimodal bioimaging and osteochondral tissue regeneration via external magnetic actuation. ACS Biomater. Sci. Eng. 5 (7), 3549–3560. doi:10.1021/acsbiomaterials.9b00571
Dasari, A., Xue, J. Y., and Deb, S. (2022). Magnetic nanoparticles in bone tissue engineering. Nanomaterials 12 (5), 757. doi:10.3390/nano12050757
Dhivya, S., Ajita, J., and Selvamurugan, N. (2015). Metallic nanomaterials for bone tissue engineering. J. Biomed. Nanotechnol. 11 (10), 1675–1700. doi:10.1166/jbn.2015.2115
Duan, J. L., Dong, J. L., Zhang, T. T., Su, Z. Y., Ding, J., Zhang, Y., et al. (2014). Polyethyleneimine-functionalized iron oxide nanoparticles for systemic siRNA delivery in experimental arthritis. Nanomedicine 9 (6), 789–801. doi:10.2217/nnm.13.217
Duan, L., Zuo, J., Zhang, F., Li, B., Xu, Z., Zhang, H., et al. (2020). Magnetic targeting of HU-MSCs in the treatment of glucocorticoid-associated osteonecrosis of the femoral head through akt/bcl2/bad/caspase-3 pathway. Int. J. Nanomedicine 15, 3605–3620. doi:10.2147/ijn.S244453
Estévez, M., Montalbano, G., Gallo-Cordova, A., Ovejero, J. G., Izquierdo-Barba, I., González, B., et al. (2022). Incorporation of superparamagnetic iron oxide nanoparticles into collagen formulation for 3D electrospun scaffolds. Nanomater. (Basel) 12 (2), 181. doi:10.3390/nano12020181
Fan, M., Yan, J., Tan, H., Miao, Y., and Hu, X. (2014). Magnetic biopolymer nanogels via biological assembly for vectoring delivery of biopharmaceuticals. J. Mat. Chem. B 2 (47), 8399–8405. doi:10.1039/c4tb01106f
Fazzalari, N. L. (2011). Bone fracture and bone fracture repair. Osteoporos. Int. 22 (6), 2003–2006. doi:10.1007/s00198-011-1611-4
Ferrage, L., Bertrand, G., Lenormand, P., Grossin, D., and Ben-Nissan, B. (2017). A review of the additive manufacturing (3DP) of bioceramics: Alumina, zirconia (PSZ) and hydroxyapatite. J. Aust. Ceram. Soc. 53 (1), 11–20. doi:10.1007/s41779-016-0003-9
Ferreira, R. V., Silva-Caldeira, P. P., Pereira-Maia, E. C., Fabris, J. D., Cavalcante, L. C. D., Ardisson, J. D., et al. (2016). Bio-inactivation of human malignant cells through highly responsive diluted colloidal suspension of functionalized magnetic iron oxide nanoparticles. J. Nanopart. Res. 18 (4), 92. doi:10.1007/s11051-016-3400-7
Filardo, G., Petretta, M., Cavallo, C., Roseti, L., Durante, S., Albisinni, U., et al. (2019). Patient-specific meniscus prototype based on 3D bioprinting of human cell-laden scaffold. Bone Jt. Res. 8 (2), 101–106. doi:10.1302/2046-3758.82.Bjr-2018-0134.R1
Gao, J. Y., Cai, S. M., Wang, Z. J., Li, D., Ou, M. Y., Zhang, X. L., et al. (2022). The optimization of ligature/bone defect-induced periodontitis model in rats. Odontology. doi:10.1007/s10266-022-00715-7
Ghosh, S., More, P., Derle, A., Kitture, R., Kale, T., Gorain, M., et al. (2015). Diosgenin functionalized iron oxide nanoparticles as novel nanomaterial against breast cancer. J. Nanosci. Nanotechnol. 15 (12), 9464–9472. doi:10.1166/jnn.2015.11704
Gomes, D. S., Santos, A. M. C., Neves, G. A., and Menezes, R. R. (2019). A brief review on hydroxyapatite production and use in biomedicine. Cerâmica 65 (374), 282–302. doi:10.1590/0366-69132019653742706
Grayson, W. L., Bunnell, B. A., Martin, E., Frazier, T., Hung, B. P., and Gimble, J. M. (2015). Stromal cells and stem cells in clinical bone regeneration. Nat. Rev. Endocrinol. 11 (3), 140–150. doi:10.1038/nrendo.2014.234
Guo, X. M., Li, W., Luo, L. H., Wang, Z. H., Li, Q. P., Kong, F. F., et al. (2017). External magnetic field-enhanced chemo-photothermal combination tumor therapy via iron oxide nanoparticles. ACS Appl. Mat. Interfaces 9 (19), 16581–16593. doi:10.1021/acsami.6b16513
Guo, Y., Liu, Y., Shi, C., Wu, T., Cui, Y., Wang, S., et al. (2021). Remote-controllable bone-targeted delivery of estradiol for the treatment of ovariectomy-induced osteoporosis in rats. J. Nanobiotechnology 19 (1), 248. doi:10.1186/s12951-021-00976-4
Hajinasab, A., Saber-Samandari, S., Ahmadi, S., and Alamara, K. (2018). Preparation and characterization of a biocompatible magnetic scaffold for biomedical engineering. Mater. Chem. Phys. 204, 378–387. doi:10.1016/j.matchemphys.2017.10.080
Han, L., Guo, Y., Jia, L., Zhang, Q., Sun, L., Yang, Z., et al. (2021a). 3D magnetic nanocomposite scaffolds enhanced the osteogenic capacities of rat bone mesenchymal stem cells in vitro and in a rat calvarial bone defect model by promoting cell adhesion. J. Biomed. Mat. Res. A 109 (9), 1670–1680. doi:10.1002/jbm.a.37162
Han, X., Tang, S., Wang, L., Xu, X., Yan, R., Yan, S., et al. (2021b). Multicellular spheroids formation on hydrogel enhances osteogenic/odontogenic differentiation of dental pulp stem cells under magnetic nanoparticles induction. Int. J. Nanomedicine 16, 5101–5115. doi:10.2147/ijn.S318991
Hankenson, K. D., Gagne, K., and Shaughnessy, M. (2015). Extracellular signaling molecules to promote fracture healing and bone regeneration. Adv. Drug Deliv. Rev. 94, 3–12. doi:10.1016/j.addr.2015.09.008
Hao, J., Bai, B., Ci, Z., Tang, J., Hu, G., Dai, C., et al. (2022). Large-sized bone defect repair by combining a decalcified bone matrix framework and bone regeneration units based on photo-crosslinkable osteogenic microgels. Bioact. Mat. 14, 97–109. doi:10.1016/j.bioactmat.2021.12.013
Hao, L., Li, L., Wang, P., Wang, Z., Shi, X., Guo, M., et al. (2019). Synergistic osteogenesis promoted by magnetically actuated nano-mechanical stimuli. Nanoscale 11 (48), 23423–23437. doi:10.1039/c9nr07170a
Harrison, R., Markides, H., Morris, R. H., Richards, P., El Haj, A. J., and Sottile, V. (2017). Autonomous magnetic labelling of functional mesenchymal stem cells for improved traceability and spatial control in cell therapy applications. J. Tissue Eng. Regen. Med. 11 (8), 2333–2348. doi:10.1002/term.2133
Hassanajili, S., Karami-Pour, A., Oryan, A., and Talaei-Khozani, T. (2019). Preparation and characterization of PLA/PCL/HA composite scaffolds using indirect 3D printing for bone tissue engineering. Mater. Sci. Eng. C 104, 109960. doi:10.1016/j.msec.2019.109960
Ho-Shui-Ling, A., Bolander, J., Rustom, L. E., Johnson, A. W., Luyten, F. P., and Picart, C. (2018). Bone regeneration strategies: Engineered scaffolds, bioactive molecules and stem cells current stage and future perspectives. Biomaterials 180, 143–162. doi:10.1016/j.biomaterials.2018.07.017
Hola, K., Markova, Z., Zoppellaro, G., Tucek, J., and Zboril, R. (2015). Tailored functionalization of iron oxide nanoparticles for MRI, drug delivery, magnetic separation and immobilization of biosubstances. Biotechnol. Adv. 33, 1162–1176. doi:10.1016/j.biotechadv.2015.02.003
Hou, S., Niu, X., Li, L., Zhou, J., Qian, Z., Yao, D., et al. (2019). Simultaneous nano- and microscale structural control of injectable hydrogels via the assembly of nanofibrous protein microparticles for tissue regeneration. Biomaterials 223, 119458. doi:10.1016/j.biomaterials.2019.119458
Hu, K., Sun, J., Guo, Z., Wang, P., Chen, Q., Ma, M., et al. (2015). A novel magnetic hydrogel with aligned magnetic colloidal assemblies showing controllable enhancement of magnetothermal effect in the presence of alternating magnetic field. Adv. Mat. 27 (15), 2507–2514. doi:10.1002/adma.201405757
Hu, S., Chen, H., Zhou, F., Liu, J., Qian, Y., Hu, K., et al. (2021). Superparamagnetic core-shell electrospun scaffolds with sustained release of IONPs facilitating in vitro and in vivo bone regeneration. J. Mat. Chem. B 9 (43), 8980–8993. doi:10.1039/d1tb01261d
Hu, S., Zhou, Y., Zhao, Y., Xu, Y., Zhang, F., Gu, N., et al. (2018). Enhanced bone regeneration and visual monitoring via superparamagnetic iron oxide nanoparticle scaffold in rats. J. Tissue Eng. Regen. Med. 12 (4), e2085–e2098. doi:10.1002/term.2641
Hua, P., Wang, Y. Y., Liu, L. B., Liu, J. L., Liu, J. Y., Yang, Y. Q., et al. (2015). In vivo magnetic resonance imaging tracking of transplanted superparamagnetic iron oxide-labeled bone marrow mesenchymal stem cells in rats with myocardial infarction. Mol. Med. Rep. 11 (1), 113–120. doi:10.3892/mmr.2014.2649
Huang, J., Liu, W., Liang, Y., Li, L., Duan, L., Chen, J., et al. (2018). Preparation and biocompatibility of diphasic magnetic nanocomposite scaffold. Mater. Sci. Eng. C 87, 70–77. doi:10.1016/j.msec.2018.02.003
Huang, Q., Zou, Y., Arno, M. C., Chen, S., Wang, T., Gao, J., et al. (2017). Hydrogel scaffolds for differentiation of adipose-derived stem cells. Chem. Soc. Rev. 46 (20), 6255–6275. doi:10.1039/c6cs00052e
Huang, Y. W., Cambre, M., and Lee, H. J. (2017). The toxicity of nanoparticles depends on multiple molecular and physicochemical mechanisms. Int. J. Mol. Sci. 18 (12), 2702. doi:10.3390/ijms18122702
Huang, Z., He, Y., Chang, X., Liu, J., Yu, L., Wu, Y., et al. (2020). A magnetic iron oxide/polydopamine coating can improve osteogenesis of 3D-printed porous titanium scaffolds with a static magnetic field by upregulating the TGFβ-smads pathway. Adv. Healthc. Mat. 9 (14), e2000318. doi:10.1002/adhm.202000318
Iacoviță, C., Fizeșan, I., Nitica, S., Florea, A., Barbu-Tudoran, L., Dudric, R., et al. (2021). Silica coating of ferromagnetic iron oxide magnetic nanoparticles significantly enhances their hyperthermia performances for efficiently inducing cancer cells death in vitro. Pharmaceutics 13 (12), 2026. doi:10.3390/pharmaceutics13122026
Ingber, D. E. (2006). Cellular mechanotransduction: Putting all the pieces together again. FASEB J. 20 (7), 811–827. doi:10.1096/fj.05-5424rev
Iyer, S. R., Xu, S., Stains, J. P., Bennett, C. H., and Lovering, R. M. (2017). Superparamagnetic iron oxide nanoparticles in musculoskeletal biology. Tissue Eng. Part B Rev. 23 (4), 373–385. doi:10.1089/ten.TEB.2016.0437
Jakob, F., Ebert, R., Ignatius, A., Matsushita, T., Watanabe, Y., Groll, J., et al. (2013). Bone tissue engineering in osteoporosis. Maturitas 75 (2), 118–124. doi:10.1016/j.maturitas.2013.03.004
Jang, J. H., Castano, O., and Kim, H. W. (2009). Electrospun materials as potential platforms for bone tissue engineering. Adv. Drug Deliv. Rev. 61 (12), 1065–1083. doi:10.1016/j.addr.2009.07.008
Jegatheeswaran, S., and Sundrarajan, M. (2015). PEGylation of novel hydroxyapatite/PEG/Ag nanocomposite particles to improve its antibacterial efficacy. Mater. Sci. Eng. C 51, 174–181. doi:10.1016/j.msec.2015.02.012
Jia, L., Yang, Z. K., Sun, L. X., Zhang, Q., Guo, Y., Chen, Y. L., et al. (2021). A three-dimensional-printed SPION/PLGA scaffold for enhanced palate-bone regeneration and concurrent alteration of the oral microbiota in rats. Mater. Sci. Eng. C 126, 112173. doi:10.1016/j.msec.2021.112173
Jia, Y. C., Zhang, P. L., Sun, Y. C., Kang, Q. L., Xu, J., Zhang, C. F., et al. (2019). Regeneration of large bone defects using mesoporous silica coated magnetic nanoparticles during distraction osteogenesis. Nanomedicine Nanotechnol. Biol. Med. 21, 102040. doi:10.1016/j.nano.2019.102040
Jiang, P., Zhang, Y., Zhu, C., Zhang, W., Mao, Z., and Gao, C. (2016). Fe(3)O(4)/BSA particles induce osteogenic differentiation of mesenchymal stem cells under static magnetic field. Acta Biomater. 46, 141–150. doi:10.1016/j.actbio.2016.09.020
Jun, I., Han, H. S., Edwards, J. R., and Jeon, H. (2018). Electrospun fibrous scaffolds for tissue engineering: Viewpoints on architecture and fabrication. Int. J. Mol. Sci. 19 (3), 745. doi:10.3390/ijms19030745
Kangari, P., Talaei-Khozani, T., Razeghian-Jahromi, I., and Razmkhah, M. (2020). Mesenchymal stem cells: Amazing remedies for bone and cartilage defects. Stem Cell. Res. Ther. 11 (1), 492. doi:10.1186/s13287-020-02001-1
Kesse, X., Adam, A., Begin-Colin, S., Mertz, D., Larquet, E., Gacoin, T., et al. (2020). Elaboration of superparamagnetic and bioactive multicore-shell nanoparticles (gamma-Fe2O3@SiO2-CaO): A promising material for bone cancer treatment. ACS Appl. Mat. Interfaces 12 (42), 47820–47830. doi:10.1021/acsami.0c12769
Khajuria, D. K., Kumar, V. B., Gigi, D., Gedanken, A., and Karasik, D. (2018). Accelerated bone regeneration by nitrogen-doped carbon dots functionalized with hydroxyapatite nanoparticles. ACS Appl. Mat. Interfaces 10 (23), 19373–19385. doi:10.1021/acsami.8b02792
Khalili, M., Keshvari, H., Imani, R., Sohi, A. N., Esmaeili, E., and Tajabadi, M. (2022). Study of osteogenic potential of electrospun PCL incorporated by dendrimerized superparamagnetic nanoparticles as a bone tissue engineering scaffold. Polym. Adv. Technol. 33 (3), 782–794. doi:10.1002/pat.5555
Khodaei, A., Jahanmard, F., Madaah Hosseini, H. R., Bagheri, R., Dabbagh, A., Weinans, H., et al. (2022). Controlled temperature-mediated curcumin release from magneto-thermal nanocarriers to kill bone tumors. Bioact. Mat. 11, 107–117. doi:10.1016/j.bioactmat.2021.09.028
Kim, K., and Fisher, J. P. (2007). Nanoparticle technology in bone tissue engineering. J. Drug Target. 15 (4), 241–252. doi:10.1080/10611860701289818
Kircher, M. F., Gambhir, S. S., and Grimm, J. (2011). Noninvasive cell-tracking methods. Nat. Rev. Clin. Oncol. 8 (11), 677–688. doi:10.1038/nrclinonc.2011.141
Kiyani, M. M., Moghul, N. B., Butt, M. A., Rehman, H., Masood, R., Rajput, T. A., et al. (2022). Anti-hyperuricemic effect of iron oxide nanoparticles against monosodium urate crystals induced gouty arthritis in BALB/c mice. Biol. Trace Elem. Res. 200 (4), 1659–1666. doi:10.1007/s12011-021-02769-0
Kolosnjaj-Tabi, J., Wilhelm, C., Clément, O., and Gazeau, F. (2013). Cell labeling with magnetic nanoparticles: Opportunity for magnetic cell imaging and cell manipulation. J. Nanobiotechnology 11, S7. doi:10.1186/1477-3155-11-s1-s7
Koons, G. L., Diba, M., and Mikos, A. G. (2020). Materials design for bone-tissue engineering. Nat. Rev. Mat. 5, 584–603. doi:10.1038/s41578-020-0204-2
Kowalczewski, C. J., and Saul, J. M. (2018). Biomaterials for the delivery of growth factors and other therapeutic agents in tissue engineering approaches to bone regeneration. Front. Pharmacol. 9, 513. doi:10.3389/fphar.2018.00513
Kumar, P., Saini, M., Dehiya, B. S., Sindhu, A., Kumar, V., Kumar, R., et al. (2020). Comprehensive survey on nanobiomaterials for bone tissue engineering applications. Nanomater. (Basel) 10 (10), 2019. doi:10.3390/nano10102019
Laurent, S., Forge, D., Port, M., Roch, A., Robic, C., Vander Elst, L., et al. (2008). Magnetic iron oxide nanoparticles: Synthesis, stabilization, vectorization, physicochemical characterizations, and biological applications. Chem. Rev. 108 (6), 2064–2110. doi:10.1021/cr068445e
Lee, H., Thirunavukkarasu, G. K., Kim, S., and Lee, J. Y. (2018). Remote induction of in situ hydrogelation in a deep tissue, using an alternating magnetic field and superparamagnetic nanoparticles. Nano Res. 11 (11), 5997–6009. doi:10.1007/s12274-018-2114-9
Lee, M. S., Su, C. M., Yeh, J. C., Wu, P. R., Tsai, T. Y., and Lou, S. L. (2016). Synthesis of composite magnetic nanoparticles Fe3O4 with alendronate for osteoporosis treatment. Int. J. Nanomedicine 11, 4583–4594. doi:10.2147/ijn.S112415
Li, M., Fu, S., Cai, Z., Li, D., Liu, L., Deng, D., et al. (2021). Dual regulation of osteoclastogenesis and osteogenesis for osteoporosis therapy by iron oxide hydroxyapatite core/shell nanocomposites. Regen. Biomater. 8 (5), rbab027. doi:10.1093/rb/rbab027
Li, X., Wei, J., Aifantis, K. E., Fan, Y., Feng, Q., Cui, F. Z., et al. (2016). Current investigations into magnetic nanoparticles for biomedical applications. J. Biomed. Mat. Res. A 104 (5), 1285–1296. doi:10.1002/jbm.a.35654
Li, X., Wei, Z., Lv, H., Wu, L., Cui, Y., Yao, H., et al. (2019). Iron oxide nanoparticles promote the migration of mesenchymal stem cells to injury sites. Int. J. Nanomedicine 14, 573–589. doi:10.2147/ijn.S184920
Li, Y., Chen, Z. W., and Gu, N. (2012). In vitro biological effects of magnetic nanoparticles. Chin. Sci. Bull. 57 (31), 3972–3978. doi:10.1007/s11434-012-5295-8
Li, Y. J., Chen, H., Wu, J., He, Q., Li, Y. T., Yang, W. B., et al. (2018). Preparation and characterization of APTES modified magnetic MMT capable of using as anisotropic nanoparticles. Appl. Surf. Sci. 447, 393–400. doi:10.1016/j.apsusc.2018.03.230
Li, Y., Ye, D. W., Li, M. X., Ma, M., and Gu, N. (2018). Adaptive materials based on iron oxide nanoparticles for bone regeneration. Chemphyschem 19 (16), 1965–1979. doi:10.1002/cphc.201701294
Li, Z., Du, T., Ruan, C., and Niu, X. (2021). Bioinspired mineralized collagen scaffolds for bone tissue engineering. Bioact. Mat. 6 (5), 1491–1511. doi:10.1016/j.bioactmat.2020.11.004
Li, Z. H., Bai, H. T., Wang, Z. H., Liu, Y. Z., Ren, M., Wang, X. G., et al. (2022a). Ultrasound-mediated rapamycin delivery for promoting osseointegration of 3D printed prosthetic interfaces via autophagy regulation in osteoporosis. Mater. Des. 216, 110586. doi:10.1016/j.matdes.2022.110586
Li, Z. H., Zhao, Y., Wang, Z. H., Ren, M., Wang, X. G., Liu, H., et al. (2022b). Engineering multifunctional hydrogel-integrated 3D printed bioactive prosthetic interfaces for osteoporotic osseointegration. Adv. Healthc. Mat. 11 (11), 2102535. doi:10.1002/adhm.202102535
Li, Z. Y., Ba, R. K., Wang, Z. F., Wei, J. H., Zhao, Y. M., and Wu, W. (2017). Angiogenic potential of human bone marrow-derived mesenchymal stem cells in chondrocyte brick-enriched constructs promoted stable regeneration of craniofacial cartilage. Stem Cells Transl. Med. 6 (2), 601–612. doi:10.5966/sctm.2016-0050
Liao, W., Lu, J. W., Wang, Q. J., Yan, S., Li, Y., Zhang, Y. B., et al. (2022). Osteogenesis of iron oxide nanoparticles-labeled human precartilaginous stem cells in interpenetrating network printable hydrogel. Front. Bioeng. Biotechnol. 10, 872149. doi:10.3389/fbioe.2022.872149
Lin, S., Lin, K. F., Lu, D. N., and Liu, Z. (2017). Preparation of uniform magnetic iron oxide nanoparticles by co-precipitation in a helical module microchannel reactor. J. Environ. Chem. Eng. 5 (1), 303–309. doi:10.1016/j.jece.2016.12.011
Liu, L., Jin, R., Duan, J., Yang, L., Cai, Z., Zhu, W., et al. (2020). Bioactive iron oxide nanoparticles suppress osteoclastogenesis and ovariectomy-induced bone loss through regulating the TRAF6-p62-CYLD signaling complex. Acta Biomater. 103, 281–292. doi:10.1016/j.actbio.2019.12.022
Liu, Q., Feng, L., Chen, Z., Lan, Y., Liu, Y., Li, D., et al. (2020). Ultrasmall superparamagnetic iron oxide labeled silk fibroin/hydroxyapatite multifunctional scaffold loaded with bone marrow-derived mesenchymal stem cells for bone regeneration. Front. Bioeng. Biotechnol. 8, 697. doi:10.3389/fbioe.2020.00697
Liu, X., Li, L., Gaihre, B., Park, S., Li, Y., Terzic, A., et al. (2022). Scaffold-free spheroids with two-dimensional heteronano-layers (2DHNL) enabling stem cell and osteogenic factor codelivery for bone repair. ACS Nano 16 (2), 2741–2755. doi:10.1021/acsnano.1c09688
Liu, Y., Cao, F. L., Sun, B. Q., Bellanti, J. A., and Zheng, S. G. (2021). Magnetic nanoparticles: A new diagnostic and treatment platform for rheumatoid arthritis. J. Leukoc. Biol. 109 (2), 415–424. doi:10.1002/jlb.5mr0420-008rr
Liu, Y. N., Liu, Y., Zheng, C. P., Huang, N., Chen, X., Zhu, X. F., et al. (2018). Ru nanoparticles coated with gamma-Fe2O3 promoting and monitoring the differentiation of human mesenchymal stem cells via MRI tracking. Colloids Surfaces B Biointerfaces 170, 701–711. doi:10.1016/j.colsurfb.2018.05.041
Loi, F., Córdova, L. A., Pajarinen, J., Lin, T. H., Yao, Z., and Goodman, S. B. (2016). Inflammation, fracture and bone repair. Bone 86, 119–130. doi:10.1016/j.bone.2016.02.020
Lopes, D., Martins-Cruz, C., Oliveira, M. B., and Mano, J. F. (2018). Bone physiology as inspiration for tissue regenerative therapies. Biomaterials 185, 240–275. doi:10.1016/j.biomaterials.2018.09.028
Lu, J. W., Yang, F., Ke, Q. F., Xie, X. T., and Guo, Y. P. (2018). Magnetic nanoparticles modified-porous scaffolds for bone regeneration and photothermal therapy against tumors. Nanomedicine Nanotechnol. Biol. Med. 14 (3), 811–822. doi:10.1016/j.nano.2017.12.025
Lu, R., Zhang, Y., Tao, H., Zhou, L., Li, H., Chen, T., et al. (2020). Gadolinium-hyaluronic acid nanoparticles as an efficient and safe magnetic resonance imaging contrast agent for articular cartilage injury detection. Bioact. Mat. 5 (4), 758–767. doi:10.1016/j.bioactmat.2020.05.009
Ma, B., Xie, J., Jiang, J., Shuler, F. D., and Bartlett, D. E. (2013). Rational design of nanofiber scaffolds for orthopedic tissue repair and regeneration. Nanomedicine (Lond) 8 (9), 1459–1481. doi:10.2217/nnm.13.132
Mahmoudi, M., Hofmann, H., Rothen-Rutishauser, B., and Petri-Fink, A. (2012). Assessing the in vitro and in vivo toxicity of superparamagnetic iron oxide nanoparticles. Chem. Rev. 112 (4), 2323–2338. doi:10.1021/cr2002596
Mahmoudi, M., Simchi, A., Imani, M., Shokrgozar, M. A., Milani, A. S., Häfeli, U. O., et al. (2010). A new approach for the in vitro identification of the cytotoxicity of superparamagnetic iron oxide nanoparticles. Colloids Surfaces B Biointerfaces 75 (1), 300–309. doi:10.1016/j.colsurfb.2009.08.044
Marin, E., Boschetto, F., and Pezzotti, G. (2020). Biomaterials and biocompatibility: An historical overview. J. Biomed. Mat. Res. A 108 (8), 1617–1633. doi:10.1002/jbm.a.36930
Martino, F., Perestrelo, A. R., Vinarsky, V., Pagliari, S., and Forte, G. (2018). Cellular mechanotransduction: From tension to function. Front. Physiol. 9, 824. doi:10.3389/fphys.2018.00824
Marycz, K., Alicka, M., Kornicka-Garbowska, K., Polnar, J., Lis-Bartos, A., Wiglusz, R. J., et al. (2020). Promotion through external magnetic field of osteogenic differentiation potential in adipose-derived mesenchymal stem cells: Design of polyurethane/poly(lactic) acid sponges doped with iron oxide nanoparticles. J. Biomed. Mat. Res. 108 (4), 1398–1411. doi:10.1002/jbm.b.34488
Marycz, K., Kornicka-Garbowska, K., Patej, A., Sobierajska, P., Kotela, A., Turlej, E., et al. (2022). Aminopropyltriethoxysilane (APTES)-Modified nanohydroxyapatite (nHAp) incorporated with iron oxide (IO) nanoparticles promotes early osteogenesis, reduces inflammation and inhibits osteoclast activity. Mater. (Basel) 15 (6), 2095. doi:10.3390/ma15062095
McNeill, E. P., Zeitouni, S., Pan, S. M., Haskell, A., Cesarek, M., Tahan, D., et al. (2020). Characterization of a pluripotent stem cell-derived matrix with powerful osteoregenerative capabilities. Nat. Commun. 11 (1), 3025. doi:10.1038/s41467-020-16646-2
Mehta, K. J. (2022). Iron oxide nanoparticles in mesenchymal stem cell detection and therapy. Stem Cell. Rev. Rep. 1–28. doi:10.1007/s12015-022-10343-x
Meng, H. X., Chowdhury, T. T., and Gavara, N. (2021). The mechanical interplay between differentiating mesenchymal stem cells and gelatin-based substrates measured by atomic force microscopy. Front. Cell. Dev. Biol. 9, 697525. doi:10.3389/fcell.2021.697525
Mengesha, A., Hoerres, A., and Mahajan, P. (2022). Cytocompatibility of oleic acid modified iron oxide nanoparticles. Mater. Lett. 323, 132528. doi:10.1016/j.matlet.2022.132528
Migliorini, F., La Padula, G., Torsiello, E., Spiezia, F., Oliva, F., and Maffulli, N. (2021). Strategies for large bone defect reconstruction after trauma, infections or tumour excision: A comprehensive review of the literature. Eur. J. Med. Res. 26 (1), 118. doi:10.1186/s40001-021-00593-9
Mohammadi, M., Mousavi Shaegh, S. A., Alibolandi, M., Ebrahimzadeh, M. H., Tamayol, A., Jaafari, M. R., et al. (2018). Micro and nanotechnologies for bone regeneration: Recent advances and emerging designs. J. Control. Release 274, 35–55. doi:10.1016/j.jconrel.2018.01.032
Moise, S., Céspedes, E., Soukup, D., Byrne, J. M., El Haj, A. J., and Telling, N. D. (2017). The cellular magnetic response and biocompatibility of biogenic zinc- and cobalt-doped magnetite nanoparticles. Sci. Rep. 7, 39922. doi:10.1038/srep39922
Mondschein, R. J., Kanitkar, A., Williams, C. B., Verbridge, S. S., and Long, T. E. (2017). Polymer structure-property requirements for stereolithographic 3D printing of soft tissue engineering scaffolds. Biomaterials 140, 170–188. doi:10.1016/j.biomaterials.2017.06.005
Moreno Madrid, A. P., Vrech, S. M., Sanchez, M. A., and Rodriguez, A. P. (2019). Advances in additive manufacturing for bone tissue engineering scaffolds. Mater. Sci. Eng. C 100, 631–644. doi:10.1016/j.msec.2019.03.037
Mortimer, C. J., and Wright, C. J. (2017). The fabrication of iron oxide nanoparticle-nanofiber composites by electrospinning and their applications in tissue engineering. Biotechnol. J. 12 (7), 1600693. doi:10.1002/biot.201600693
Murphy, C. M., Haugh, M. G., and O'Brien, F. J. (2010). The effect of mean pore size on cell attachment, proliferation and migration in collagen-glycosaminoglycan scaffolds for bone tissue engineering. Biomaterials 31 (3), 461–466. doi:10.1016/j.biomaterials.2009.09.063
Navarro, M., Michiardi, A., Castaño, O., and Planell, J. A. (2008). Biomaterials in orthopaedics. J. R. Soc. Interface 5 (27), 1137–1158. doi:10.1098/rsif.2008.0151
Ou, L., Lan, Y., Feng, Z., Feng, L., Yang, J., Liu, Y., et al. (2019). Functionalization of SF/HAP scaffold with GO-PEI-miRNA inhibitor complexes to enhance bone regeneration through activating transcription factor 4. Theranostics 9 (15), 4525–4541. doi:10.7150/thno.34676
Ozbey, A., Karimzadehkhouei, M., Yalcin, S. E., Gozuacik, D., and Kosar, A. (2015). Modeling of ferrofluid magnetic actuation with dynamic magnetic fields in small channels. Microfluid. Nanofluidics 18 (3), 447–460. doi:10.1007/s10404-014-1442-7
Paik, S. Y. R., Kim, J. S., Shin, S. J., and Ko, S. (2015). Characterization, quantification, and determination of the toxicity of iron oxide nanoparticles to the bone marrow cells. Int. J. Mol. Sci. 16 (9), 22243–22257. doi:10.3390/ijms160922243
Pang, Y. C., Su, L., Fu, Y., Jia, F., Zhang, C. X., Cao, X. K., et al. (2021). Inhibition of furin by bone targeting superparamagnetic iron oxide nanoparticles alleviated breast cancer bone metastasis. Bioact. Mater. 6 (3), 712–720. doi:10.1016/j.bioactmat.2020.09.006
Pariti, A., Desai, P., Maddirala, S. K. Y., Ercal, N., Katti, K. V., Liang, X., et al. (2014). Superparamagnetic Au-Fe3O4 nanoparticles: One-pot synthesis, biofunctionalization and toxicity evaluation. Mat. Res. Express 1 (3), 035023. doi:10.1088/2053-1591/1/3/035023
Petretta, M., Gambardella, A., Desando, G., Cavallo, C., Bartolotti, I., Shelyakova, T., et al. (2021). Multifunctional 3D-printed magnetic polycaprolactone/hydroxyapatite scaffolds for bone tissue engineering. Polym. (Basel) 13 (21), 3825. doi:10.3390/polym13213825
Pistone, A., Iannazzo, D., Panseri, S., Montesi, M., Tampieri, A., and Galvagno, S. (2014). Hydroxyapatite-magnetite-MWCNT nanocomposite as a biocompatible multifunctional drug delivery system for bone tissue engineering. Nanotechnology 25 (42), 425701. doi:10.1088/0957-4484/25/42/425701
Prodan, A. M., Iconaru, S. L., Ciobanu, C. S., Chifiriuc, M. C., Stoicea, M., and Predoi, D. (2013). Iron oxide magnetic nanoparticles: Characterization and toxicity evaluation by in vitro and in vivo assays. J. Nanomater. 2018, 587021. doi:10.1155/2013/587021
Przekora, A., Audemar, M., Pawlat, J., Canal, C., Thomann, J. S., Labay, C., et al. (2020). Positive effect of cold atmospheric nitrogen plasma on the behavior of mesenchymal stem cells cultured on a bone scaffold containing iron oxide-loaded silica nanoparticles catalyst. Int. J. Mol. Sci. 21 (13), 4738. doi:10.3390/ijms21134738
Pucci, C., Degl'Innocenti, A., Belenli Gümüş, M., and Ciofani, G. (2022). Superparamagnetic iron oxide nanoparticles for magnetic hyperthermia: Recent advancements, molecular effects, and future directions in the omics era. Biomater. Sci. 10, 2103–2121. doi:10.1039/d1bm01963e
Radeloff, K., Radeloff, A., Ramos Tirado, M., Scherzad, A., Hagen, R., Kleinsasser, N. H., et al. (2020). Toxicity and functional impairment in human adipose tissue-derived stromal cells (hASCs) following long-term exposure to very small iron oxide particles (VSOPs). Nanomater. (Basel) 10 (4), 741. doi:10.3390/nano10040741
Rahman, Z., Zidan, A. S., and Khan, M. A. (2010). Risperidone solid dispersion for orally disintegrating tablet: Its formulation design and non-destructive methods of evaluation. Int. J. Pharm. X. 400 (1-2), 49–58. doi:10.1016/j.ijpharm.2010.08.025
Rajan, A., Sharma, M., and Sahu, N. K. (2020). Assessing magnetic and inductive thermal properties of various surfactants functionalised Fe(3)O(4) nanoparticles for hyperthermia. Sci. Rep. 10 (1), 15045. doi:10.1038/s41598-020-71703-6
Ramesh, N., Moratti, S. C., and Dias, G. J. (2018). Hydroxyapatite-polymer biocomposites for bone regeneration: A review of current trends. J. Biomed. Mat. Res. 106 (5), 2046–2057. doi:10.1002/jbm.b.33950
Ramimoghadam, D., Bagheri, S., and Hamid, S. B. A. (2014). Progress in electrochemical synthesis of magnetic iron oxide nanoparticles. J. Magnetism Magnetic Mater. 368, 207–229. doi:10.1016/j.jmmm.2014.05.015
Rasker, J. J. (1995). Rheumatology in general practice. Rheumatology 34 (6), 494–497. doi:10.1093/rheumatology/34.6.494
Safarova, Y., Umbayev, B., Hortelano, G., and Askarova, S. (2020). Mesenchymal stem cells modifications for enhanced bone targeting and bone regeneration. Regen. Med. 15 (4), 1579–1594. doi:10.2217/rme-2019-0081
Sahmani, S., Khandan, A., Saber-Samandari, S., and Mohammadi Aghdam, M. (2020). Effect of magnetite nanoparticles on the biological and mechanical properties of hydroxyapatite porous scaffolds coated with ibuprofen drug. Mater. Sci. Eng. C 111, 110835. doi:10.1016/j.msec.2020.110835
Saiz, E., Zimmermann, E. A., Lee, J. S., Wegst, U. G., and Tomsia, A. P. (2013). Perspectives on the role of nanotechnology in bone tissue engineering. Dent. Mat. 29 (1), 103–115. doi:10.1016/j.dental.2012.08.001
Salehiabar, M., Nosrati, H., Davaran, S., Danafar, H., and Manjili, H. K. (2018). Facile synthesis and characterization of L-aspartic acid coated iron oxide magnetic nanoparticles (IONPs) for biomedical applications. Drug Res. (Stuttg). 68 (5), 280–285. doi:10.1055/s-0043-120197
Saraiva, A. S., Ribeiro, I. A. C., Fernandes, M. H., Cerdeira, A. C., Vieira, B. J. C., Waerenborgh, J. C., et al. (2021). 3D-printed platform multi-loaded with bioactive, magnetic nanoparticles and an antibiotic for re-growing bone tissue. Int. J. Pharm. X. 593, 120097. doi:10.1016/j.ijpharm.2020.120097
Sasikala, A. R. K., Kaliannagounder, V. K., Alluri, N. R., Shrestha, B. K., Kim, S. J., Ali-Boucetta, H., et al. (2022). Development of self-powered multifunctional piezomagnetic nanoparticles for non-invasive post-surgical osteosarcoma theranogeneration. Nano Energy 96, 107134. doi:10.1016/j.nanoen.2022.107134
Scharf, A., Holmes, S., Thoresen, M., Mumaw, J., Stumpf, A., and Peroni, J. (2015). Superparamagnetic iron oxide nanoparticles as a means to track mesenchymal stem cells in a large animal model of tendon injury. Contrast Media Mol. Imaging 10 (5), 388–397. doi:10.1002/cmmi.1642
Schiffman, J. D., and Schauer, C. L. (2008). A review: Electrospinning of biopolymer nanofibers and their applications. Polym. Rev. 48 (2), 317–352. doi:10.1080/15583720802022182
Schneider, M. G. M., Azcona, P., Campelo, A., Massheimer, V., Agotegaray, M., and Lassalle, V. (2021). Magnetic nanoplatform with novel potential for the treatment of bone pathologies: Drug loading and biocompatibility on blood and bone cells. IEEE Trans. Nanobioscience. doi:10.1109/tnb.2021.3136525
Schreivogel, S., Kuchibhotla, V., Knaus, P., Duda, G. N., and Petersen, A. (2019). Load-induced osteogenic differentiation of mesenchymal stromal cells is caused by mechano-regulated autocrine signaling. J. Tissue Eng. Regen. Med. 13 (11), 1992–2008. doi:10.1002/term.2948
Schwartz, L., da Veiga Moreira, J., and Jolicoeur, M. (2018). Physical forces modulate cell differentiation and proliferation processes. J. Cell. Mol. Med. 22 (2), 738–745. doi:10.1111/jcmm.13417
Serpooshan, V., and Guvendiren, M. (2020). Editorial for the special issue on 3D printing for tissue engineering and regenerative medicine. Micromachines (Basel) 11 (4), 366. doi:10.3390/mi11040366
Shan, D. Y., Shi, Y. Z., Duan, S., Wei, Y., Cai, Q., and Yang, X. P. (2013). Electrospun magnetic poly(L-lactide) (PLLA) nanofibers by incorporating PLLA-stabilized Fe3O4 nanoparticles. Mater. Sci. Eng. C 33 (6), 3498–3505. doi:10.1016/j.msec.2013.04.040
Shelat, R., Bhatt, L. K., Paunipagar, B., Kurian, T., Khanna, A., and Chandra, S. (2020). Regeneration of hyaline cartilage in osteochondral lesion model using L-lysine magnetic nanoparticles labeled mesenchymal stem cells and their in vivo imaging. J. Tissue Eng. Regen. Med. 14 (11), 1604–1617. doi:10.1002/term.3120
Shen, W. B., Plachez, C., Chan, A., Yarnell, D., Puche, A. C., Fishman, P. S., et al. (2013). Human neural progenitor cells retain viability, phenotype, proliferation, and lineage differentiation when labeled with a novel iron oxide nanoparticle, Molday ION Rhodamine B. Int. J. Nanomedicine 8, 4593–4600. doi:10.2147/ijn.S53012
Shokouhimehr, M., Theus, A. S., Kamalakar, A., Ning, L., Cao, C., Tomov, M. L., et al. (2021). 3D bioprinted bacteriostatic hyperelastic bone scaffold for damage-specific bone regeneration. Polym. (Basel) 13 (7), 1099. doi:10.3390/polym13071099
Shokrollahi, H. (2017). A review of the magnetic properties, synthesis methods and applications of maghemite. J. Magnetism Magnetic Mater. 426, 74–81. doi:10.1016/j.jmmm.2016.11.033
Singh, R. K., Patel, K. D., Lee, J. H., Lee, E. J., Kim, J. H., Kim, T. H., et al. (2014). Potential of magnetic nanofiber scaffolds with mechanical and biological properties applicable for bone regeneration. PLoS One 9 (4), e91584. doi:10.1371/journal.pone.0091584
Singh, Y. P., and Dasgupta, S. (2022). Gelatin-based electrospun and lyophilized scaffolds with nano scale feature for bone tissue engineering application: Review. J. Biomater. Sci. Polym. Ed., 1–55. doi:10.1080/09205063.2022.2068943
Sniadecki, N. J. (2010). Minireview: A tiny touch: Activation of cell signaling pathways with magnetic nanoparticles. Endocrinology 151 (2), 451–457. doi:10.1210/en.2009-0932
Song, B. T., Xu, Q., Wang, C. Y., Xu, S. C., and Zhang, H. X. (2016). Fabrication of polymer/drug-loaded hydroxyapatite particle composite fibers for drug sustained release. J. Appl. Polym. Sci. 133 (3). doi:10.1002/app.42871
Takeuchi, T., Yoshida, H., and Tanaka, S. (2021). Role of interleukin-6 in bone destruction and bone repair in rheumatoid arthritis. Autoimmun. Rev. 20 (9), 102884. doi:10.1016/j.autrev.2021.102884
Tang, C. L., Mahoney, J. L., McKee, M. D., Richards, R. R., Waddell, J. P., Louie, B., et al. (1998). Donor site morbidity following vascularized fibular grafting. Microsurgery 18 (6), 383–386. doi:10.1002/(sici)1098-2752(1998)18:6<383::aid-micr8>3.0.co;2-5
Taylor, E. N., and Webster, T. J. (2011). Multifunctional magnetic nanoparticles for orthopedic and biofilm infections. Int. J. Nanotechnol. 8 (1-2), 21–35. doi:10.1504/ijnt.2011.037168
Taylor, E. N., and Webster, T. J. (2009). The use of superparamagnetic nanoparticles for prosthetic biofilm prevention. Int. J. Nanomedicine 4, 145–152. doi:10.2147/ijn.s5976
Tevlin, R., McArdle, A., Atashroo, D., Walmsley, G. G., Senarath-Yapa, K., Zielins, E. R., et al. (2014). Biomaterials for craniofacial bone engineering. J. Dent. Res. 93 (12), 1187–1195. doi:10.1177/0022034514547271
Thrivikraman, G., Madras, G., and Basu, B. (2014). Intermittent electrical stimuli for guidance of human mesenchymal stem cell lineage commitment towards neural-like cells on electroconductive substrates. Biomaterials 35 (24), 6219–6235. doi:10.1016/j.biomaterials.2014.04.018
Tomov, M. L., Cetnar, A., Do, K., Bauser-Heaton, H., and Serpooshan, V. (2019). Patient-specific 3-dimensional-bioprinted model for in vitro analysis and treatment planning of pulmonary artery atresia in tetralogy of fallot and major aortopulmonary collateral arteries. J. Am. Heart Assoc. 8 (24), e014490. doi:10.1161/jaha.119.014490
Torgbo, S., and Sukyai, P. (2018). Bacterial cellulose-based scaffold materials for bone tissue engineering. Appl. Mater. Today 11, 34–49. doi:10.1016/j.apmt.2018.01.004
Trombetta, R., Inzana, J. A., Schwarz, E. M., Kates, S. L., and Awad, H. A. (2017). 3D printing of calcium phosphate ceramics for bone tissue engineering and drug delivery. Ann. Biomed. Eng. 45 (1), 23–44. doi:10.1007/s10439-016-1678-3
Uskoković, V., Huynh, E., Tang, S., Jovanović, S., and Wu, V. M. (2019). Colloids or powders: Which nanoparticle formulations do cells like more? Colloids Surfaces B Biointerfaces 181, 39–47. doi:10.1016/j.colsurfb.2019.05.019
Vangijzegem, T., Stanicki, D., and Laurent, S. (2019). Magnetic iron oxide nanoparticles for drug delivery: Applications and characteristics. Expert Opin. Drug Deliv. 16 (1), 69–78. doi:10.1080/17425247.2019.1554647
Ventola, C. L. (2012). The nanomedicine revolution: Part 1: Emerging concepts. P Trans. 37 (9), 512–525.
Vergnaud, F., Kesse, X., Jacobs, A., Perton, F., Begin-Colin, S., Mertz, D., et al. (2022). Magnetic bioactive glass nano-heterostructures: A deeper insight into magnetic hyperthermia properties in the scope of bone cancer treatment. Biomater. Sci. 10 (14), 3993–4007. doi:10.1039/d2bm00319h
Verma, G. S., Nirmal, N. K., Gunpal, D., Gupta, H., Yadav, M., Kumar, N., et al. (2021). Intraperitoneal exposure of iron oxide nanoparticles causes dose-dependent toxicity in Wistar rats. Toxicol. Ind. Health 37 (12), 763–775. doi:10.1177/07482337211058668
Virlan, M. J., Miricescu, D., Radulescu, R., Sabliov, C. M., Totan, A., Calenic, B., et al. (2016). Organic nanomaterials and their applications in the treatment of oral diseases. Molecules 21 (2), 207. doi:10.3390/molecules21020207
Volokhova, M., Shugai, A., Tsujimoto, M., Kubo, A. L., Telliskivi, S., Nigul, M., et al. (2022). Cubic iron core-shell nanoparticles functionalized to obtain high-performance MRI contrast agents. Mater. (Basel) 15 (6), 2228. doi:10.3390/ma15062228
Wagner, J. M., Reinkemeier, F., Wallner, C., Dadras, M., Huber, J., Schmidt, S. V., et al. (2019). Adipose-derived stromal cells are capable of restoring bone regeneration after post-traumatic osteomyelitis and modulate B-cell response. Stem Cells Transl. Med. 8 (10), 1084–1091. doi:10.1002/sctm.18-0266
Wang, G., Xu, W., Zhang, J., Tang, T., Chen, J., and Fan, C. (2021). Induction of bone remodeling by raloxifene-doped iron oxide functionalized with hydroxyapatite to accelerate fracture healing. J. Biomed. Nanotechnol. 17 (5), 932–941. doi:10.1166/jbn.2021.3068
Wang, L. T., Hu, P., Jiang, H., Zhao, J. H., Tang, J., Jiang, D. J., et al. (2022). Mild hyperthermia-mediated osteogenesis and angiogenesis play a critical role in magnetothermal composite-induced bone regeneration. Nano Today 43, 101401. doi:10.1016/j.nantod.2022.101401
Wang, Q., Chen, B., Cao, M., Sun, J., Wu, H., Zhao, P., et al. (2016). Response of MAPK pathway to iron oxide nanoparticles in vitro treatment promotes osteogenic differentiation of hBMSCs. Biomaterials 86, 11–20. doi:10.1016/j.biomaterials.2016.02.004
Wang, Q., Ma, X., Liao, H., Liang, Z., Li, F., Tian, J., et al. (2020). Artificially engineered cubic iron oxide nanoparticle as a high-performance magnetic particle imaging tracer for stem cell tracking. ACS Nano 14 (2), 2053–2062. doi:10.1021/acsnano.9b08660
Wang, Q. W., Chen, B., Ma, F., Lin, S. K., Cao, M., Li, Y., et al. (2017). Magnetic iron oxide nanoparticles accelerate osteogenic differentiation of mesenchymal stem cells via modulation of long noncoding RNA INZEB2. Nano Res. 10 (2), 626–642. doi:10.1007/s12274-016-1322-4
Wang, Y. J., Jeng, U. S., and Hsu, S. H. (2018). Biodegradable water-based polyurethane shape memory elastomers for bone tissue engineering. ACS Biomater. Sci. Eng. 4 (4), 1397–1406. doi:10.1021/acsbiomaterials.8b00091
Wasnik, S., Lakhan, R., Baylink, D. J., Rundle, C. H., Xu, Y., Zhang, J. T., et al. (2019). Cyclooxygenase 2 augments osteoblastic but suppresses chondrocytic differentiation of CD90(+) skeletal stem cells in fracture sites. Sci. Adv. 5 (7), eaaw2108. doi:10.1126/sciadv.aaw2108
Watanabe, Y., Harada, N., Sato, K., Abe, S., Yamanaka, K., and Matushita, T. (2016). Stem cell therapy: Is there a future for reconstruction of large bone defects? Injury 47, S47–S51. doi:10.1016/s0020-1383(16)30012-2
Wei, X. H., Zhang, X. K., Yang, Z., Li, L., and Sui, H. T. (2021). Osteoinductive potential and antibacterial characteristics of collagen coated iron oxide nanosphere containing strontium and hydroxyapatite in long term bone fractures. Arabian J. Chem. 14 (3), 102984. doi:10.1016/j.arabjc.2020.102984
Weinstein, S. L. (2016). The burden of musculoskeletal conditions. J. Bone Jt. Surg. 98 (16), 1331. doi:10.2106/jbjs.16.00595
Williams, D. F. (2008). On the mechanisms of biocompatibility. Biomaterials 29 (20), 2941–2953. doi:10.1016/j.biomaterials.2008.04.023
Woo, S., Kim, S., Kim, H., Cheon, Y. W., Yoon, S., Oh, J. H., et al. (2021). Charge-modulated synthesis of highly stable iron oxide nanoparticles for in vitro and in vivo toxicity evaluation. Nanomater. (Basel) 11 (11), 3068. doi:10.3390/nano11113068
Wu, L., and Shen, S. (2019). What potential do magnetic iron oxide nanoparticles have for the treatment of rheumatoid arthritis? Nanomedicine 14 (8), 927–930. doi:10.2217/nnm-2019-0071
Wu, L., Wen, W., Wang, X., Huang, D., Cao, J., Qi, X., et al. (2022). Ultrasmall iron oxide nanoparticles cause significant toxicity by specifically inducing acute oxidative stress to multiple organs. Part. Fibre Toxicol. 19 (1), 24. doi:10.1186/s12989-022-00465-y
Wu, S., Yu, Q., Sun, Y., and Tian, J. (2018). Synergistic effect of a LPEMF and SPIONs on BMMSC proliferation, directional migration, and osteoblastogenesis. Am. J. Transl. Res. 10 (5), 1431–1443.
Wu, X.-D., Kang, L., Tian, J., Wu, Y., Huang, Y., Liu, J., et al. (2022). Exosomes derived from magnetically actuated bone mesenchymal stem cells promote tendon-bone healing through the miR-21-5p/SMAD7 pathway. Mat. Today Bio 15, 100319. doi:10.1016/j.mtbio.2022.100319
Xia, Y., Chen, H., Zhang, F., Wang, L., Chen, B., Reynolds, M. A., et al. (2018a). Injectable calcium phosphate scaffold with iron oxide nanoparticles to enhance osteogenesis via dental pulp stem cells. Artif. Cells Nanomed. Biotechnol. 46, 423–433. doi:10.1080/21691401.2018.1428813
Xia, Y., Chen, H., Zhao, Y., Zhang, F., Li, X., Wang, L., et al. (2019a). Novel magnetic calcium phosphate-stem cell construct with magnetic field enhances osteogenic differentiation and bone tissue engineering. Mater. Sci. Eng. C 98, 30–41. doi:10.1016/j.msec.2018.12.120
Xia, Y., Guo, Y., Yang, Z., Chen, H., Ren, K., Weir, M. D., et al. (2019b). Iron oxide nanoparticle-calcium phosphate cement enhanced the osteogenic activities of stem cells through WNT/β-catenin signaling. Mater. Sci. Eng. C 104, 109955. doi:10.1016/j.msec.2019.109955
Xia, Y., Sun, J., Zhao, L., Zhang, F., Liang, X. J., Guo, Y., et al. (2018b). Magnetic field and nano-scaffolds with stem cells to enhance bone regeneration. Biomaterials 183, 151–170. doi:10.1016/j.biomaterials.2018.08.040
Xia, Y., Zhao, Y. T., Zhang, F. M., Chen, B., Hu, X. T., Weir, M. D., et al. (2019c). Iron oxide nanoparticles in liquid or powder form enhanced osteogenesis via stem cells on injectable calcium phosphate scaffold. Nanomedicine Nanotechnol. Biol. Med. 21, 102069. doi:10.1016/j.nano.2019.102069
Xie, Y. Y., Liu, W., Zhang, B., Wang, B., Wang, L. D., Liu, S., et al. (2019). Systematic intracellular biocompatibility assessments of superparamagnetic iron oxide nanoparticles in human umbilical cord mesenchyme stem cells in testifying its reusability for inner cell tracking by MRI. J. Biomed. Nanotechnol. 15 (11), 2179–2192. doi:10.1166/jbn.2019.2845
Xu, G., Hu, X., Han, L., Zhao, Y., and Li, Z. (2021). The construction of a novel xenograft bovine bone scaffold, (DSS)6-liposome/CKIP-1 siRNA/calcine bone and its osteogenesis evaluation on skull defect in rats. J. Orthop. Transl. 28, 74–82. doi:10.1016/j.jot.2021.02.001
Xue, H., Zhang, Z. H., Lin, Z., Su, J., Panayi, A. C., Xiong, Y., et al. (2022). Enhanced tissue regeneration through immunomodulation of angiogenesis and osteogenesis with a multifaceted nanohybrid modified bioactive scaffold. Bioact. Mater. 18, 552–568. doi:10.1016/j.bioactmat.2022.05.023
Xue, K., Wang, X., Yong, P. W., Young, D. J., Wu, Y. L., Li, Z. B., et al. (2019). Hydrogels as emerging materials for translational biomedicine. Adv. Ther. (Weinh). 2 (1), 1800088. doi:10.1002/adtp.201800088
Yang, C. Y., Hsiao, J. K., Tai, M. F., Chen, S. T., Cheng, H. Y., Wang, J. L., et al. (2011). Direct labeling of hMSC with SPIO: The long-term influence on toxicity, chondrogenic differentiation capacity, and intracellular distribution. Mol. Imaging Biol. 13 (3), 443–451. doi:10.1007/s11307-010-0360-7
Yang, H. Y., Wang, H., Wen, C. H., Bai, S., Wei, P. F., Xu, B., et al. (2022). Effects of iron oxide nanoparticles as T-2-MRI contrast agents on reproductive system in male mice. J. Nanobiotechnology 20 (1), 98. doi:10.1186/s12951-022-01291-2
Yang, W., Zhu, P., Huang, H., Zheng, Y., Liu, J., Feng, L., et al. (2019). Functionalization of novel theranostic hydrogels with kartogenin-grafted USPIO nanoparticles to enhance cartilage regeneration. ACS Appl. Mat. Interfaces 11 (38), 34744–34754. doi:10.1021/acsami.9b12288
Yang, Y., Chu, L., Yang, S., Zhang, H., Qin, L., Guillaume, O., et al. (2018). Dual-functional 3D-printed composite scaffold for inhibiting bacterial infection and promoting bone regeneration in infected bone defect models. Acta Biomater. 79, 265–275. doi:10.1016/j.actbio.2018.08.015
Yao, D., Liu, N. N., and Mo, B. W. (2020). Assessment of proliferation, migration and differentiation potentials of bone marrow mesenchymal stem cells labeling with silica-coated and amine-modified superparamagnetic iron oxide nanoparticles. Cytotechnology 72 (4), 513–525. doi:10.1007/s10616-020-00397-5
Yao, S., Lin, X., Xu, Y., Chen, Y., Qiu, P., Shao, C., et al. (2019). Osteoporotic bone recovery by a highly bone-inductive calcium phosphate polymer-induced liquid-precursor. Adv. Sci. (Weinh). 6 (19), 1900683. doi:10.1002/advs.201900683
Yi, H., Ur Rehman, F., Zhao, C., Liu, B., and He, N. (2016). Recent advances in nano scaffolds for bone repair. Bone Res. 4, 16050. doi:10.1038/boneres.2016.50
Yin, X.-Y., Wang, C.-C., Du, P., Wang, X.-S., Lu, Y.-C., Sun, Y.-W., et al. (2023). Muse cells decrease the neuroinflammatory response by modulating the proportion of M1 and M2 microglia in vitro. Neural Regen. Res. 18 (1), 213–218. doi:10.4103/1673-5374.343885
Ying, H., Ruan, Y., Zeng, Z., Bai, Y., Xu, J., and Chen, S. (2022). Iron oxide nanoparticles size-dependently activate mouse primary macrophages via oxidative stress and endoplasmic reticulum stress. Int. Immunopharmacol. 105, 108533. doi:10.1016/j.intimp.2022.108533
Yu, C., Geng, J., Zhuang, Y., Zhao, J., Chu, L., Luo, X., et al. (2016). Preparation of the chitosan grafted poly (quaternary ammonium)/Fe3O4 nanoparticles and its adsorption performance for food yellow 3. Carbohydr. Polym. 152, 327–336. doi:10.1016/j.carbpol.2016.06.114
Yu, P., Zheng, L., Wang, P., Chai, S., Zhang, Y., Shi, T., et al. (2020). Development of a novel polysaccharide-based iron oxide nanoparticle to prevent iron accumulation-related osteoporosis by scavenging reactive oxygen species. Int. J. Biol. Macromol. 165, 1634–1645. doi:10.1016/j.ijbiomac.2020.10.016
Zhang, M., Hu, W., Cai, C., Wu, Y., Li, J., and Dong, S. (2022). Advanced application of stimuli-responsive drug delivery system for inflammatory arthritis treatment. Mat. Today Bio 14, 100223. doi:10.1016/j.mtbio.2022.100223
Zhang, W., Gu, J., Li, K., Zhao, J., Ma, H., Wu, C., et al. (2019a). A hydrogenated black TiO(2) coating with excellent effects for photothermal therapy of bone tumor and bone regeneration. Mater. Sci. Eng. C 102, 458–470. doi:10.1016/j.msec.2019.04.025
Zhang, W., Zheng, Y., Liu, H., Zhu, X., Gu, Y., Lan, Y., et al. (2019b). A non-invasive monitoring of USPIO labeled silk fibroin/hydroxyapatite scaffold loaded DPSCs for dental pulp regeneration. Mater. Sci. Eng. C 103, 109736. doi:10.1016/j.msec.2019.05.021
Zhang, Y., Hu, J., Zhao, X., Xie, R., Qin, T., and Ji, F. (2019). Mechanically robust shape memory polyurethane nanocomposites for minimally invasive bone repair. ACS Appl. Bio Mat. 2 (3), 1056–1065. doi:10.1021/acsabm.8b00655
Zhang, Y., and Zhang, J. (2005). Surface modification of monodisperse magnetite nanoparticles for improved intracellular uptake to breast cancer cells. J. Colloid Interface Sci. 283 (2), 352–357. doi:10.1016/j.jcis.2004.09.042
Zhao, S., Zhu, M., Zhang, J., Zhang, Y., Liu, Z., Zhu, Y., et al. (2014). Three dimensionally printed mesoporous bioactive glass and poly(3-hydroxybutyrate-co-3-hydroxyhexanoate) composite scaffolds for bone regeneration. J. Mat. Chem. B 2 (36), 6106–6118. doi:10.1039/c4tb00838c
Zhao, Y. Z., Chen, R., Xue, P. P., Luo, L. Z., Zhong, B., Tong, M. Q., et al. (2021). Magnetic PLGA microspheres loaded with SPIONs promoted the reconstruction of bone defects through regulating the bone mesenchymal stem cells under an external magnetic field. Mater. Sci. Eng. C 122, 111877. doi:10.1016/j.msec.2021.111877
Zhao, Z., Li, M., Zeng, J., Huo, L., Liu, K., Wei, R., et al. (2022). Recent advances in engineering iron oxide nanoparticles for effective magnetic resonance imaging. Bioact. Mat. 12, 214–245. doi:10.1016/j.bioactmat.2021.10.014
Zheng, L., Zhuang, Z., Li, Y., Shi, T., Fu, K., Yan, W., et al. (2022). Bone targeting antioxidative nano-iron oxide for treating postmenopausal osteoporosis. Bioact. Mat. 14, 250–261. doi:10.1016/j.bioactmat.2021.11.012
Zhu, G., Zhang, T., Chen, M., Yao, K., Huang, X., Zhang, B., et al. (2021). Bone physiological microenvironment and healing mechanism: Basis for future bone-tissue engineering scaffolds. Bioact. Mat. 6 (11), 4110–4140. doi:10.1016/j.bioactmat.2021.03.043
Keywords: iron oxide nanoparticles, tissue engineering, bone regeneration, scaffolds, stem cells
Citation: Wang N, Xie Y, Xi Z, Mi Z, Deng R, Liu X, Kang R and Liu X (2022) Hope for bone regeneration: The versatility of iron oxide nanoparticles. Front. Bioeng. Biotechnol. 10:937803. doi: 10.3389/fbioe.2022.937803
Received: 06 May 2022; Accepted: 02 August 2022;
Published: 25 August 2022.
Edited by:
Jun Pan, Chongqing University, ChinaReviewed by:
Alessandra Quarta, Department of Physical Sciences and Technologies of Matter, National Research Council (CNR), ItalyCopyright © 2022 Wang, Xie, Xi, Mi, Deng, Liu, Kang and Liu. This is an open-access article distributed under the terms of the Creative Commons Attribution License (CC BY). The use, distribution or reproduction in other forums is permitted, provided the original author(s) and the copyright owner(s) are credited and that the original publication in this journal is cited, in accordance with accepted academic practice. No use, distribution or reproduction is permitted which does not comply with these terms.
*Correspondence: Ran Kang, a2FuZ3JhbjEyNkAxMjYuY29t; Xin Liu, bGl1eGluQG5qdWNtLmVkdS5jbg==
†These authors have contributed equally to this work and share first authorship
Disclaimer: All claims expressed in this article are solely those of the authors and do not necessarily represent those of their affiliated organizations, or those of the publisher, the editors and the reviewers. Any product that may be evaluated in this article or claim that may be made by its manufacturer is not guaranteed or endorsed by the publisher.
Research integrity at Frontiers
Learn more about the work of our research integrity team to safeguard the quality of each article we publish.