- 1Science and Technology Division, Jilin Medical University, Jilin, China
- 2Department of Materials Science and Engineering, Changchun University of Science and Technology, Jilin, China
- 3Jilin Vocational College of Industry and Technology, Jilin, China
- 4Division of Biotechnology and Macromolecular Chemistry, Graduate School of Engineering, Hokkaido University, Sapporo, Japan
A series of thermo- and light-responsive copolymers of poly (N-isopropylacrylamide) (PNIPAM) and 6-[4-(4-methoxy phenyl azo)-phenoxyl-hexyl methacrylate) (AzoMA) (PNIPAM-b-PAzoMA) were synthesized via reversible addition–fragmentation chain transfer (RAFT) radical polymerization. The resulting copolymers had a narrow molecular weight distribution range of 1.06–1.24, in which Mn changed regularly with the monomer concentration. Subsequently, the diblock copolymers were successfully modified on the surface of iron oxide nanoparticles through the interaction between the chemical bonds to prepare Fe3O4@(PNIPAM-b-PAzoMA) nanoparticles. The size of fabricated nanoparticles with excellent thermo-sensitivity and photo-sensitivity was controlled at about 40–50 nm. Cell viability assays suggested that the nanoparticles showed no significant cytotoxicity and potential drug delivery in the tumor microenvironment.
1 Introduction
The cancer burden had risen to 19.3 million new cases and 10.0 million cancer deaths in 2020 (IARC, 2020). Cancer is characterized by highly metastatic and recurrent, traditional treatments such as surgery, chemotherapy, and radiation and is difficult to cure completely (Couzin-Frankel, 2013; Gotwals et al., 2017). Therefore, continuous endeavors by the researchers are being made to develop new low-cost and effective solutions for cancer treatments with few side effects, high specificity, and anti-metastasis (Lu et al., 2020; Baggiolini et al., 2021; Eberhardt et al., 2021). Polymeric nanomedicine has become crucial in clinical application with its precise and efficient targeting therapy of tumors (Farokhzad and Langer, 2006; Hawkins et al., 2008; Girase et al., 2019; Chen et al., 2021). A few drug-carrying polymeric nanoparticles (NPs) have been used in preclinical oncology treatment (Tong et al., 2012; Li et al., 2018; He et al., 2019). Stimulus-responsive NP drug delivery systems have attracted extensive attention because of their specificity, selectivity, and efficacy in tumor tissues and biocompatibility for normal cells (Karimi et al., 2016; Jia et al., 2018). The endogenous and exogenous (or external) stimuli could induce drug release from polymer vesicles. External stimulus-responsive factors included pH, magnetism, temperature, ultrasound, and light (Satarkar and Hilt, 2008; Brazel, 2009; Schroeder et al., 2009; Ge et al., 2012; Huo et al., 2014; Liu et al., 2014; Shanmugam et al., 2014; Olejniczak et al., 2015; Rwei et al., 2015; Alimoradi et al., 2016). Slight changes in the external environment could trigger the release of the polymer vesicles to the drug. The integration of multiple functions and various chemical nature of compounds to improve their functionalities was an innovative approach to obtaining nanoscale drug carriers.
Light-responsive nanocarriers have attracted extensive attention in the field of drug delivery (Olejniczak et al., 2015; Rwei et al., 2015). Using light as a trigger has many beneficial advantages. Light is easy to manipulate and make tiny adjustments. The photo-responsive nano-carrier acts as a biofriendly material, which could achieve accurate control of the location, time, and dose of released species (Sortino, 2012). The first example is azobenzene units, which could be handily transformed between trans- and cis-configuration to release encapsulated antineoplastic drugs. There are so many distinctions of azobenzene derivatives in physical, chemical, optical, and biological properties which are caused by E-to-Z isomerization. It is the reason that azobenzene compounds could be utilized to tune the characteristics of host materials. A growing number of studies were using azobenzene derivatives covalently linked to polymers as photoactivated drug delivery systems in NPs formed via self-assembly of polymers (Shibaev et al., 2003; Wang et al., 2015; Zhang et al., 2019; Shi et al., 2021a; Yao et al., 2021). It was reasonable to expect that as soon as the hydrophobic forces and π-π interactions are removed under UV irradiation, the vesicles with drugs dominated by electrostatic forces would expand rapidly to open the “gate” to achieve drug release (Yao et al., 2021).
Fe3O4 NPs are excellent nanoparticles for cancer therapy, which have been approved by the U.S. Food and Drug Administration (FDA) for the clinical treatment due to magnetic targeting and T2-weighted magnetic resonance imaging (MRI) (Ge et al., 2018). However, individual Fe3O4 NPs have no antitumor effect. Thus, composite materials based on ions have emerged, especially anti-tumor composites. In recent years, many substances have been used to combine with Fe3O4 to prepare antitumor nanoparticles, such as oxide-graphene (Lin et al., 2014), poly (ethylene glycol)-block-poly (lactic-co-glycolic acid) copolymer-encapsulated (Liu et al., 2022), and polymers (Swain et al., 2015; Du et al., 2017; Shi et al., 2021b; Ndebele et al., 2021). These composite Fe3O4 NPs showed great potential for cancer therapy. In the past few decades, the temperature sensitivity of poly (N-isopropylacrylamide) (PNIPAM) has been utilized to successfully synthesize so many drug nanocarriers with temperature responsiveness and high loading capacity. PNIPAM could respond to changes in the microenvironment temperature, which caused changes in the carrier structure and interactions with cells to achieve temperature-sensitive drug delivery and release (Liu et al., 2015; Bathfield et al., 2016; Nguyen et al., 2016). In our previous study, a series of biomaterials containing PNIPAM were also synthesized, and their lower critical solution temperature (LCST) was close to human body temperature (Cui G. et al., 2020; Cui G. H. et al., 2020). In this work, we took advantage of the thermo- and light-responsive properties of the polymer to synthesize polymer-coated magnetic nanoparticles, which may be used for drug delivery in the tumor microenvironment.
2 Materials and Methods
2.1 Materials and Instrumentation
N- isopropylacrylamide (Aldrich, 98%) was recrystallized twice from a hexane/benzene mixture (3/2, v/v). 2,2′-Azobis (2-methylpropionitrile) (AIBN) (J&K chemical, 98%), 1,3,5-trioxane (Sigma-Aldrich, 99%), 1-dodecanethiol (Sigma-Aldrich, ≥98%), tetrabutylammonium bromide (Sigma-Aldrich, 99%), carbon disulfide (Sigma-Aldrich, 99.9%), p-anisidine (Aladdin, 99%), 1,6-dibromohexane (Aladdin, 99%), phenol (Aladdin, 99%), methacrylic acid (J&K chemical, 98%), and MTT (3-[4,5-dimethylthiazol-2-yl]-2,5-diphenyltetrazolium were purchased from Acros. Human breast cancer cell (MCF-7) culture and NCTC clone 929 (L-929) were purchased from the Type Culture Collection of the Chinese Academy of Sciences, Shanghai.
The 1H nuclear magnetic resonance (NMR) spectra of polymers in CDCl3 were obtained on a Varian Unity 400 NMR spectrometer. The molecular weights (Mn) and polydispersity (Mw/M n) were measured by gel permeation chromatography (GPC) using a Waters 510 pump and a Model 410 differential refractometer at 25°C. The LCSTs of the polymer solutions were determined by turbidimetry, using a Shimadzu-2600 UV-Vis spectrophotometer with a heating rate of 0.1°C·min−1. FTIR spectra were recorded on a Shimadzu IR-8400S spectrometer. The average particle size and size distribution of the nanoparticles were characterized by dynamic light scattering (DLS) with a Malvern470 instrument at a fixed scattering angle of 90°, after being filtered by 0.45-μm Millipore filters. The scanning electron microscopy (SEM) images were obtained with JEOL JSM-6701SF. The morphologies of the nanoparticles were determined by transmission electron microscopy (TEM) with JEOL JEM-2100. Powder X-Ray Diffraction (XRD) was performed by using an X′-Pert PRO Philips X-ray diffractometer using Cu radiation (wavelength λ is 1.514056
2.2 General Procedure for the Thermo- and Light-Responsive Block Polymer Synthesis
2.2.1 Synthesis of Dual-responsive Block Polymers
The procedure of AzoMA synthesis is shown in Supplementary Scheme S1. NaNO2 (3.0094 g, 43.6 mmol) was dissolved in deionized water (20 ml). Then, it was added to the hydrochloric acid solution of p-anisidine (5.0984 g and 41.4 mmol) solution and stirred at 0°C for 2 h. Miscible liquids were shifted to sodium hydroxide solution (4 g and 0.1 mol) of phenol (4.0902 g and 43.4 mmol) and stirred at 0°C for 2 h, and the pH was adjusted to neutral to obtain 4-hydroxy-4′-methoxy-azobenzene. The aforementioned product (2.43 g and 10 mmol) and 1, 6-dibromohexane (14.67 g and 53.6 mmol) and anhydrous K2CO3 (7.3922 g and 53.6 mmol) were added to acetone (100 ml) and stirred at 75°C for 24 h. The mixture was precipitated in petroleum ether (30∼60°C) and recrystallized by thermal filtration in ethyl acetate twice to obtain 1-bromo-6 -(4-methoxyazobenzene-4′-oxygen) hexane.
Methacrylic acid (0.2004 g, 2 mmol) and KHCO3(0.1002 g and 1 mmol) were dissolved in N,N-dimethylformamide(10 ml); then, 1-bromo-6-(4-methoxyazobenzene-4′-oxygen) hexane(0.3912 g, 1.09 mmol) was added dropwise under whisk and stirred at 65°C for 4 h. The pure AzoMA was acquired by column chromatography (the results are shown in Supplementary Figures S1, S2).
2.2.2 Synthesis of PNIPAM-b-PAzoMA
PNIPAM-b-PAzoMA was synthesized using NIPAM and AzoMA as monomers by RAFT, as shown in Scheme 1. There were three steps for the synthesis of block copolymers. First, S-1-dodecyl-S´-(α,α′-dimethyl-α´´-acetic acid) trithio-carbonate (DMP) (1) was synthesized as a chain transfer agent (CTA). DMP was synthesized by a method derived from Lai et al. (2002), and we have reported in previous research (Cui G. et al., 2020). Subsequently, the synthesis of macromolecular chain transfer agents 2) via reversible addition–fragmentation chain transfer (RAFT) was mentioned in our study (Cui G. et al., 2020).
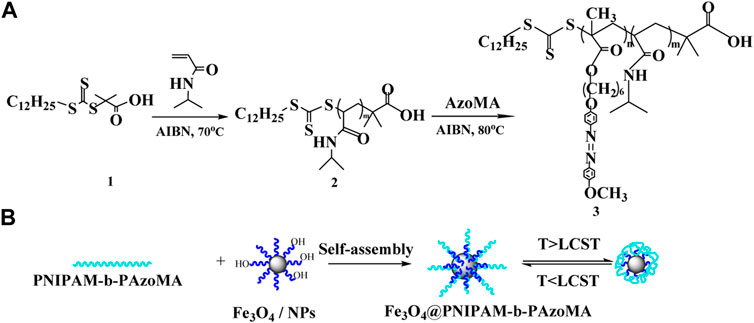
SCHEME 1. Fabrication strategy for thermo- and light-responsive polymer-coated magnetic nanoparticles.
Finally, for the synthesis of PNIPAM-b-PAzoMA, a mixture of AzoMA (0.132 g and 0.5 mmol), macro CTA (152.7 mg and 0.015 mmol), 1,3,5-trioxane (50.44 mg and 0.56 mmol), and AIBN (1.70 mg and 0.01 mmol) was added to anhydrous DMF: H2O (7.5 ml and 95:5, v/v) and was sealed on the middle side of an H-shaped ampoule glass and stirred. Nitrogen was bubbled through both mixtures for 20 min to remove any oxygen. Three freeze-pump-thaw cycles were performed to degas the solutions. The ampoule was placed at 80°C for 24 h. Polymerization was quenched by exposing the solution to air. The solution was concentrated under a vacuum, and the polymer was precipitated into petroleum ether (30∼60°C) thrice; then, the product was dried under a vacuum.
2.3 Synthesis of Polymer-Coated Magnetic Nanoparticles
Fe3O4 nanoparticles were synthesized by chemical coprecipitation (Wu et al., 2012). The details are mentioned in our report (Cui G. H. et al., 2020). The Fe3O4 nanoparticles were dissolved in N, N-dimethylformamide (20 ml) and dispersed by ultrasonic concussion. Then PNIPAM-b-PAzoMA (0.3 mmol), EDC·HCl (10 mg and 0.052 mmol), and DMAP (7.1 mg and 0.058 mmol) were added successively under stirring and stirred at 80°C in an oil bath for 24 h. The compound was washed continuously with excess methanol in order to remove the unreacted polymer after magnetic separation and dried under vacuum to obtain polymer-coated magnetic nanoparticles.
2.4 Biocompatibility Study
The cell viabilities of macro-CTA, PNIPAM-b-PAzoMA, and Fe3O4@PNIPAM-b-PAzoMA nanoparticles were preliminarily investigated by using NCTC clone 929 (L-929) and human breast cancer cell (MCF-7) culture. Specific details were referred to in our previous studies (Cui G. H. et al., 2020). Six replicate wells were used for the control and test concentrations for each sample. The optical density was measured using a microplate reader at 492 nm. The cell viability (%) was calculated according to the following Eq. 1:
where Asample was the absorbance of the cells incubated in DMEM and mixture, and Acontrol was the absorbance of the cells incubated in DMEM.
3 Results and Discussion
3.1 Architecture Analysis
The morphologies of Fe3O4@PNIPAM-b-PAzoMA nanoparticles were investigated by SEM (Figure 1A) and TEM (Figure 1B). The diameter of the Fe3O4@PNIPAM-b-PAzoMA nanoparticles ranged from 40 to 50 nm. The size was basically identical to the intensity-average hydrodynamic radius distribution f (Rh) which was measured by DLS in Figure 2A. The nanoparticles had an almost spherical shape since the polymer shells of Fe3O4@PNIPAM-b-PAzoMA nanoparticles were mostly formed by esterification.
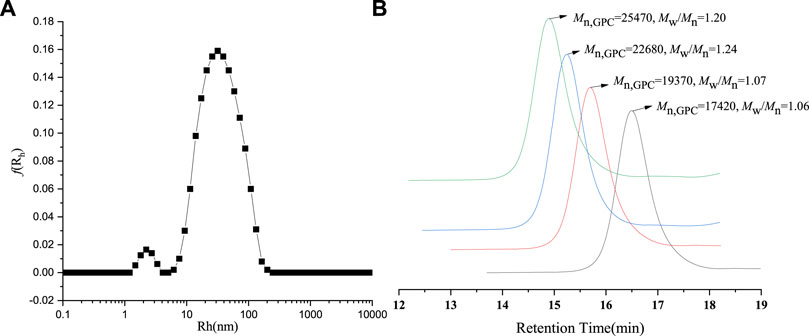
FIGURE 2. (A) DLS spectrogram for Fe3O4@ PNIPAM-b-PAzoMA nanoparticles. (B) GPC traces of PNIPAM-b-PAzoMA.
The PNIPAM-b-PAzoMA was synthesized by the different feed ratios of AzoMA/macro-CTA/AIBN, which could obtain products that had a narrow molecular weight distribution range of 1.06–1.20, as shown in Figure 2B and Supplementary Table S2. The GPC traces of the block copolymer demonstrated a clean shift toward a lower elution volume. The macro-CTA was a polymer containing 132 PNIPAM units because it had the highest LCST among many macromolecular chain initiators prepared by our study.
The architecture of the PNIPAM-b-PAzoMA and Fe3O4@PNIPAM-b-PAzoMA nanoparticles was authenticated by FT-IR spectra and 1HNMR. From Figure 3A, compared with AzoMA (Supplementary Figure S1), a characteristic signal of PNIPAM representing nitrogen atoms was shifted to 6.53 ppm. The peaks at 7.85, 6.98, 3.99, and 3.89 ppm were the characteristic signals of AzoMA. It was seen clearly that the new characteristic peak of PNIPAM at 3,286 cm−1 was assigned to the stretching vibration (νN–H) of the acylamino group in Figure 3B. The band at 1,645 fcm−1was ascribed to amide I [mainly the carbonyl stretching vibration (νC=O)], and the band at 1,544cm−1 was ascribed to amide II [mainly the N–H bending vibration (δN–H)], which has overridden the characteristic absorption peaks of AzoMA because of its high content of PNIPAM in the polymer. Two characteristic peaks appeared in the Fe3O4@PNIPAM-b-PAzoMA nanoparticles, which were located in 1,080 cm−1 and 583cm−1, and possessed the ester acid bond and Fe-O band.
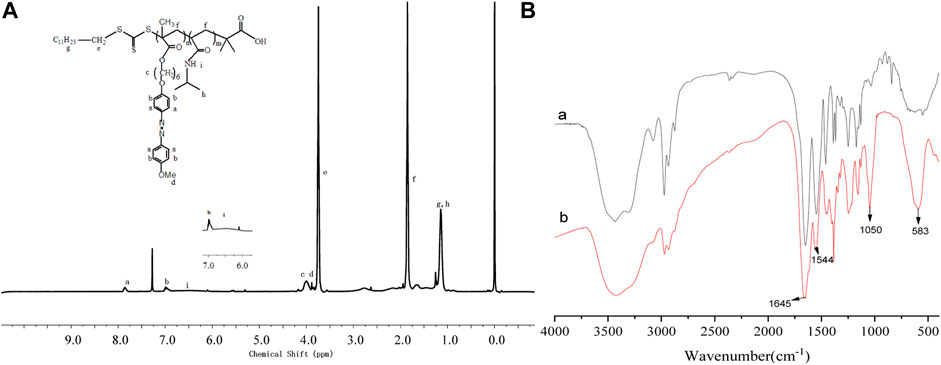
FIGURE 3. 1HNMR spectra of PNIPAM-b-PAzoMA (A), FT-IR spectra of PNIPAM-b-PAzoMA, and Fe3O4@PNIPAM-b-PAzoMA nanoparticles (B).
XPS and XRD spectra were used to further investigate the composition of the nanoparticles. Figure 4A showed the XRD pattern of the bare Fe3O4 and Fe3O4@PNIPAM-b-PAzoMA nanoparticles. The characteristic diffraction peaks for Fe3O4 (2θ = 18.07, 30.32, 35.68, 43.32, 53.78, 57.38, 62.89, and 74.56) marked by their indices on (111), (220), (311), (400), (422), (511), (440), and (533) crystal planes can be observed. All the peak positions were basically consistent with the standard data of the Fe3O4 structure (JCPDS card file No. 85–1,436) (Cullity, 1978). The peak position did no’t change, but the peak intensity changed differently. The dispersion of nanoparticles in the solution was different because of the effect of the surface polymer. In Figure 4B, peaks at the binding energies (BEs) of 284.39, 403, and 533.23eV were corresponding to C1s, N1s, and O1s, which were from the copolymer. The BE values of about 55.86eV and 688eV were ascribed to Fe3p and Fe2p. These results indicated that the copolymer was successfully grafted onto the exterior surface of the Fe3O4 nanoparticles.
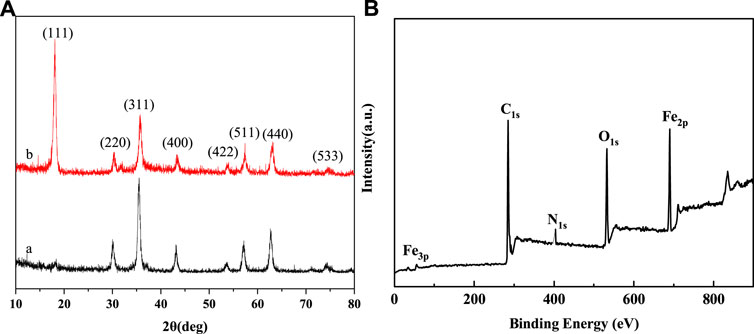
FIGURE 4. (A) XRD spectra of (a) bare Fe3O4 (b) Fe3O4@PNIPAM-b-PAzoMA nanoparticles and (B) XPS spectra of Fe3O4@PNIPAM-b-PAzoMA nanoparticles.
3.2 Thermo- and Light- Responsivity of Nanoparticles
It was found that the LCST of macro-CTA was 33.9°C in Figure 5A. It was higher than the homopolymer PNIPAM due to the hydrophilic carboxyl group of the macro-CTA. The LCSTs of all Fe3O4@PNIPAM-b-PAzoMA nanoparticles were lower than those of macro-CTA. This might be caused by the hydrophobic interaction of AzoMA in the nanoparticles. Also, the content of AzoMA in nanoparticles was inversely proportional to LCST, as shown in Supplementary Figure S3.
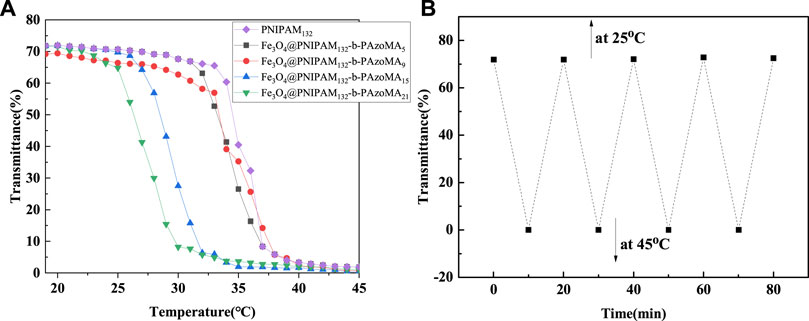
FIGURE 5. (A) Cloud point curve for the aqueous solution of macro-CTA and Fe3O4@PNIPAM-b-PAzoMA nanoparticles. (B) Plots of optical transmittance as a function of temperature for Fe3O4@(PNIPAM-b-PAzoMA) aqueous solution with the concentration of 1 mg/ml (10 min for each temperature four heating/cooling cycles between 25 and 45°C).
The effect of AzoMA’s content on the LCST of the polymer was also reflected in the change of LCST of nanoparticles before and after UV irradiation, as shown in Table 1. The reason for this phenomenon was that the azo group of AzoMA was in a low dipole moment of the trans configuration without UV irradiation, and the polarity of AzoMA was weak, and this resulted in the strong hydrophobicity of AzoMA, and the LCST of the nanoparticles was lower than that of the macro-CTA. After UV irradiation, the azo group underwent a trans-cis transition. Compared with the trans configuration, the dipole moment of the azo group in the cis configuration was much higher, and the polarity of the AzoMA was also increased so that the hydrophilicity of nanoparticles was significantly enhanced. Another possible reason was that the azo of AzoMA in the trans configuration was a planar configuration. Compared with the cis structure, the superposition between molecules was more likely to occur so as to enhance the hydrophobic association ability, which was manifested by the increased phase transition temperature of the product after UV irradiation. After the aqueous solution of the sample irradiated by UV light was placed in the shade or exposed to visible light, its LCST returned to the temperature before UV irradiation. This was due to a cis-trans configuration transition of the azo group in Figure 5B.
Figure 6 showed the UV spectrum changes of Fe3O4@PNIPAM-b-PAzoMA aqueous solutions with different contents of AzoMA that were irradiated by UV light. In the Figure 6A, the absorbance was proportional to the content of AzoMA. The sample showed a strong absorption peak at 358 nm, while the absorption peak at 450 nm was relatively weak. This was because AzoMA mainly with its trans configuration under such conditions, and the trans configuration of azobenzene was relatively low. Therefore, this study focused on Fe3O4@PNIPAM-b-PAzoMA nanoparticles with high AzoMA contents. However, in Figure 6B, the characteristic absorption peak of AzoMA’s cis configuration at 450 nm increased with the extension of UV irradiation time. This was because the azo groups in AzoMA gradually changed from trans configuration to cis configuration with the extension of UV irradiation time.
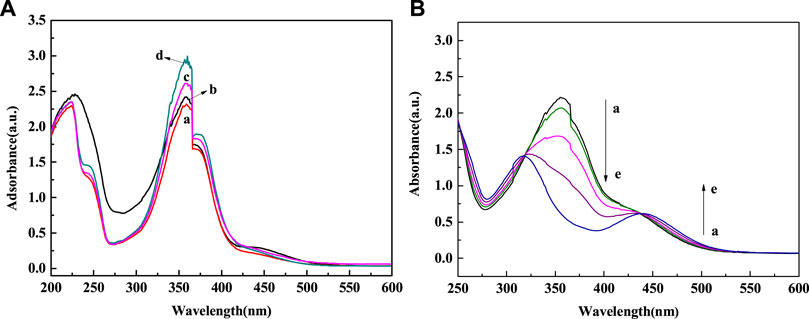
FIGURE 6. (A) Spectral changes of Fe3O4@PNIPAM-b-PAzoMA in aqueous solution during UV irradiation (365 nm, 8 W) (a) Fe3O4@PNIPAM132-b-PAzoMA5, (b) Fe3O4@PNIPAM132-b-PAzoMA9, (c) Fe3O4@PNIPAM132-b-PAzoMA15, and (d)Fe3O4@PNIPAM132-b-PAzoMA21. (B) Spectral changes of Fe3O4@PNIPAM132-b-PAzoMA21 under irradiation time: (a) 0 min, (b) 5 min, (c) 10 min, (d) 15 min, and (e) 20 min.
3.3 Thermostability and Magnetism of Nanoparticles
The thermogravimetric analysis (TG) is shown in Figure 7. We could clearly determine that below 600°C, after grafting PNIPAM-b-PAzoMA onto the surface of the Fe3O4@PNIPAM-b-PAzoMA nanoparticles, the weight loss was about 56%, while only about 7% of weight loss for bare Fe3O4 nanoparticles. Compared with bare Fe3O4 nanoparticles, the weight loss of Fe3O4@PNIPAM-b-PAzoMA is generally concentrated in three stages which were 70°C∼150°C, 150°C∼300, and 300°C∼500°C. In the first phase, the quality loss was about 5%, which was roughly consistent with the loss in 1), and this value was identified as the loss of Fe3O4. The mass loss in the second phase was mainly due to the thermal decomposition of PAzoMA. The weight loss in the third period was relatively large, especially due to the thermal decomposition of PNIPAM in the polymer, which was basically consistent with the data measured by GPC.
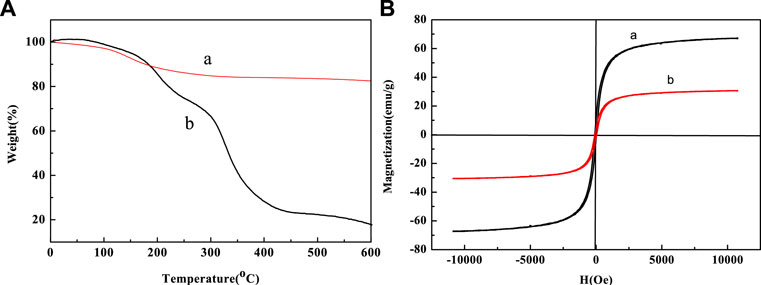
FIGURE 7. (A) TG curves of (a) Fe3O4 and (b) Fe3O4@PNIPAM-b-PAzoMA and (B) hysteresis loops of (a)bare Fe3O4 nanoparticles and (b)Fe3O4@PNIPAM-b-PAzoMA nanoparticles at room temperature.
Figure 7B shows the hysteresis graph of Fe3O4 and Fe3O4@PNIPAM-b-PAzoMA nanoparticles. Due to the small particle size, the saturation magnetization (Ms) of Fe3O4 was about 68.02 emu/g. The Ms of Fe3O4@PNIPAM-b-PAzoMA nanoparticles decreased significantly, which was 30.01 emu/g. This was because polymer-coated Fe3O4 changed the magnetic anisotropy and increased the directional obstruction on the surface. Fe3O4@PNIPAM-b-PAzoMA nanoparticles showed no coercivity, so we could judge that the nanoparticles had a certain degree of super-paramagnetism.
3.4 Assessment of the Cell Viability
The cytotoxicity study on normal cells (L-929, with high activity) and tumor cells (MCF-7, with insensitive to apoptosis induced by various chemotherapeutic drugs) was carried out to investigate the preliminary biocompatibility of the resulting Fe3O4@PNIPAM-b-PAzoMA nanoparticles in Figure 8. MTT studies showed that macro-CTA, PNIPAM-b-PAzoMA, and Fe3O4@PNIPAM-b-PAzoMA nanoparticles had very low toxicity to L-929 cells and MCF-7 cells, and the cell survival rate remained above 80% even at sample concentrations up to 60 μmol/ml.
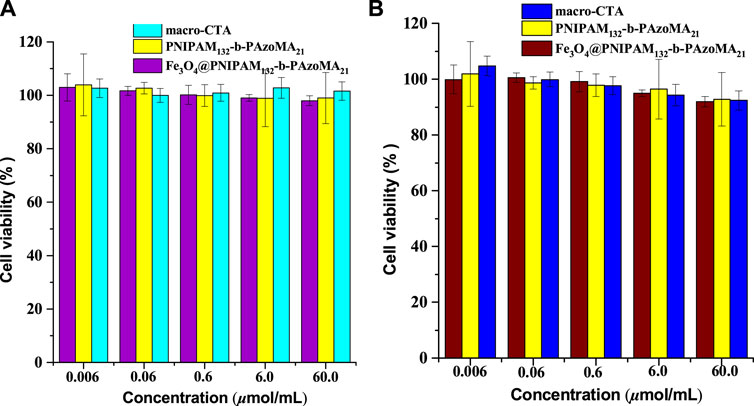
FIGURE 8. Cell viability of (A) the L-929 cells and (B) the MCF-7 cells incubated with the samples (macro-CTA, PNIPAM-b-PAzoMA, and Fe3O4@PNIPAM-b-PAzoMA nanoparticles), over a range of sample concentrations from 0.006 to 60 μmol/ml by MTT assay for 48 h.
4 Conclusion
In this study, the thermo- and light-responsive polymer-coated magnetic nanoparticles were synthesized. The size of the Fe3O4@PNIPAM-b-PAzoMA nanoparticles was about 40–50 nm. Fe3O4@PNIPAM-b-PAzoMA nanoparticles had excellent temperature sensitivity, photosensitivity, thermostability, and super-paramagnetism and no significant cytotoxicity. Fe3O4@PNIPAM-b-PAzoMA may be used for good drug delivery in the tumor microenvironment and for potential antitumor therapy.
Data Availability Statement
The original contributions presented in the study are included in the article/Supplementary Material; further inquiries can be directed to the corresponding authors.
Author Contributions
All authors listed have made a substantial, direct, and intellectual contribution to the work and approved it for publication.
Funding
We are grateful to the Jilin science and technology department, science and technology development project No. 20200201098JC, the Jilin Province Education Department the Science and Technology development project (Nos. JJKH20170415KJ, JJKH20191059KJ, JJKJ20200461KJ, and JJKH20220469KJ), the National College Student Innovation Training Program (202013706063), the Jilin University Student Innovation Program (S202113706036), and Jilin province science and technology project of traditional Chinese medicine (2022088), for financial support.
Conflict of Interest
The authors declare that the research was conducted in the absence of any commercial or financial relationships that could be construed as a potential conflict of interest.
Publisher’s Note
All claims expressed in this article are solely those of the authors and do not necessarily represent those of their affiliated organizations, or those of the publisher, the editors, and the reviewers. Any product that may be evaluated in this article, or claim that may be made by its manufacturer, is not guaranteed or endorsed by the publisher.
Acknowledgments
The authors would like to thank all reviewers of this article for their comments and suggestions.
Supplementary Material
The Supplementary Material for this article can be found online at: https://www.frontiersin.org/articles/10.3389/fbioe.2022.931830/full#supplementary-material
References
Alimoradi, H., Matikonda, S. S., Gamble, A. B., Giles, G. I., and Greish, K. (2016). Hypoxia Responsive Drug Delivery Systems in Tumor Therapy. Curr. Pharm. Des. 22 (19), 2808–2820. doi:10.2174/1381612822666160217130049
Baggiolini, A., Callahan, S. J., Montal, E., Weiss, J. M., Trieu, T., Tagore, M. M., et al. (2021). Developmental Chromatin Programs Determine Oncogenic Competence in Melanoma. Science 373, 1–10. doi:10.1126/science.abc1048
Bathfield, M., Reboul, J., Cacciaguerra, T., Lacroix-Desmazes, P., and Gérardin, C. (2016). Thermosensitive and Drug-Loaded Ordered Mesoporous Silica: A Direct and Effective Synthesis Using PEO-B-PNIPAM Block Copolymers. Chem. Mat. 28 (10), 3374–3384. doi:10.1021/acs.chemmater.6b00595
Brazel, C. S. (2009). Magnetothermally-responsive Nanomaterials: Combining Magnetic Nanostructures and Thermally-Sensitive Polymers for Triggered Drug Release. Pharm. Res. 26 (3), 644–656. doi:10.1007/s11095-008-9773-2
Chen, J., Wang, X., Yuan, Y., Chen, H. T., Zhang, L. P., Xiao, H. H., et al. (2021). Exploiting the Acquired Vulnerability of Cisplatin-Resistant Tumors with a Hypoxia-Amplifying DNA Repair-Inhibiting (HYDRI) Nanomedicine. Sci. Adv. 7, 13–25. doi:10.1126/sciadv.abc5267
Couzin-Frankel, J. (2013). Breakthrough of the Year 2013. Cancer Immunotherapy. Science 342 (6165), 1432–1433. doi:10.1126/science.342.6165.1432
Cui, G. H., Dong, S. G., Sui, C. H., Duan, Q., and Feng, B. (2020). Fabrication of Composite Fe3O4 Nanoparticles Coupled by Thermo-Responsive and Fluorescent Eu Complex on Surface Int. J. Polym. Mater. Polym. Biomaterials 8, 1–7.
Cui, G., Zhao, K., You, K., Gao, Z., Kakuchi, T., Feng, B., et al. (2020). Synthesis and Characterization of Phenylboronic Acid-Containing Polymer for Glucose-Triggered Drug Delivery+. Sci. Technol. Adv. Mater. 21, 1–10. doi:10.1080/14686996.2019.1700394
Cullity, B. D. (1978). Elements of X-Ray Diffraction. Phillippines: Addison-Wesley Publishing Company Inc.
Du, J., Shi, C., Wu, W., Bian, X., Chen, P., Cui, Q., et al. (2017). Synthesis of Core-Shell Structured FAU/SBA-15 Composite Molecular Sieves and Their Performance in Catalytic Cracking of Polystyrene. Sci. Technol. Adv. Mater. 18 (1), 939–949. doi:10.1080/14686996.2017.1396561
Eberhardt, C. S., Kissick, H. T., Patel, M. R., Cardenas, M. A., Prokhnevska, N., Obeng, R. C., et al. (2021). Functional HPV-specific PD-1+ Stem-like CD8 T Cells in Head and Neck Cancer. Nature 597, 279–284. doi:10.1038/s41586-021-03862-z
Farokhzad, O., and Langer, R. (2006). Nanomedicine: Developing Smarter Therapeutic and Diagnostic Modalities☆. Adv. Drug Deliv. Rev. 58, 1456–1459. doi:10.1016/j.addr.2006.09.011
Ge, J., Neofytou, E., Cahill, T. J., Beygui, R. E., and Zare, R. N. (2012). Drug Release from Electric-Field-Responsive Nanoparticles. ACS Nano 6 (1), 227–233. doi:10.1021/nn203430m
Ge, R., Liu, C., Zhang, X., Wang, W., Li, B., Liu, J., et al. (2018). Photothermal-Activatable Fe3O4 Superparticle Nanodrug Carriers with PD-L1 Immune Checkpoint Blockade for Anti-metastatic Cancer Immunotherapy. ACS Appl. Mat. Interfaces 10 (24), 20342–20355. doi:10.1021/acsami.8b05876
Girase, M. L., Patil, P. G., and Ige, P. P. (2019). Polymer-drug Conjugates as Nanomedicine: a Review. Int. J. Polym. Mater. Polym. Biomaterials 69 (15), 990–1014. doi:10.1080/00914037.2019.1655745
Gotwals, P., Cameron, S., Cipolletta, D., Cremasco, V., Crystal, A., Hewes, B., et al. (2017). Prospects for Combining Targeted and Conventional Cancer Therapy with Immunotherapy. Nat. Rev. Cancer 17, 286–301. doi:10.1038/nrc.2017.17
Hawkins, M. J., Soon-Shiong, P., and Desai, N. (2008). Protein Nanoparticles as Drug Carriers in Clinical Medicine. Adv. Drug Deliv. Rev. 60, 876–885. doi:10.1016/j.addr.2007.08.044
He, G., Ma, Y., Zhou, H., Sun, S., Wang, X., Qian, H., et al. (2019). Mesoporous NiS2 Nanospheres as a Hydrophobic Anticancer Drug Delivery Vehicle for Synergistic Photothermal-Chemotherapy. J. Mat. Chem. B 7, 143–149. doi:10.1039/c8tb02473a
Huo, M., Yuan, J., Tao, L., and Wei, Y. (2014). Redox-responsive Polymers for Drug Delivery: from Molecular Design to Applications. Polym. Chem. 5 (5), 1519–1528. doi:10.1039/c3py01192e
IARC (2020). Latest Global Burden of Cancer Data for 2020. (Lyon, France: International Agency for Research on Cancer).
Jia, S., Fong, W.-K., Graham, B., and Boyd, B. J. (2018). Photoswitchable Molecules in Long-Wavelength Light-Responsive Drug Delivery: From Molecular Design to Applications. Chem. Mat. 30, 2873–2887. doi:10.1021/acs.chemmater.8b00357
Karimi, M., Ghasemi, A., Zangabad, P. S., Rahighi, R., Moosavi Basri, S. M., Mirshekari, H., et al. (2016). Smart Micro/nanoparticles in Stimulus-Responsive Drug/gene Delivery Systems. Chem. Soc. Rev. 45, 1457–1501. doi:10.1039/c5cs00798d
Lai, J. T., Filla, D., and Shea, R. (2002). Functional Polymers from Novel Carboxyl-Terminated Trithiocarbonates as Highly Efficient RAFT Agents. Macromolecules 35, 6754–6756. doi:10.1021/ma020362m
Li, M., Luo, Z., and Zhao, Y. (2018). Self-Assembled Hybrid Nanostructures: Versatile Multifunctional Nanoplatforms for Cancer Diagnosis and Therapy. Chem. Mat. 30, 25–53. doi:10.1021/acs.chemmater.7b03924
Lin, L.-S., Cong, Z.-X., Cao, J.-B., Ke, K.-M., Peng, Q.-L., Gao, J., et al. (2014). Multifunctional Fe3O4@Polydopamine Core-Shell Nanocomposites for Intracellular mRNA Detection and Imaging-Guided Photothermal Therapy. ACS Nano 8, 3876–3883. doi:10.1021/nn500722y
Liu, F., Kozlovskaya, V., Medipelli, S., Xue, B., Ahmad, F., Saeed, M., et al. (2015). Temperature-Sensitive Polymersomes for Controlled Delivery of Anticancer Drugs. Chem. Mat. 27 (23), 7945–7956. doi:10.1021/acs.chemmater.5b03048
Liu, J., Bao, X., Kolesnik, I., Jia, B., Yu, Z., Xing, C., et al. (2022). Enhancing the In Vivo Stability of Polycation Gene Carriers by Using PEGylated Hyaluronic Acid as a Shielding System. BIO Integr 2 (2), 50–56. doi:10.15212/bioi-2021-0033
Liu, J., Huang, Y., Kumar, A., Tan, A., Jin, S., Mozhi, A., et al. (2014). pH-Sensitive Nano-Systems for Drug Delivery in Cancer Therapy. Biotechnol. Adv. 32 (4), 693–710. doi:10.1016/j.biotechadv.2013.11.009
Lu, S., Wang, Q., Zhang, G., Dong, X., Yang, C. T., Song, Y., et al. (2020). American Association for Cancer Research (AACR) Annual Meeting 2020. (New Orleans: LA: American Association for Cancer Research).
Ndebele, R. T., Yao, Q., Shi, Y.-N., Zhai, Y.-Y., Xu, H.-L., Lu, C.-T., et al. (2021). Progress in the Application of Nano- and Micro-based Drug Delivery Systems in Pulmonary Drug Delivery. BIO Integr. doi:10.15212/bioi-2021-0028
Nguyen, M., Felidj, N., and Mangeney, C. (2016). Looking for Synergies in Molecular Plasmonics through Hybrid Thermoresponsive Nanostructures. Chem. Mat. 28 (11), 3564–3577. doi:10.1021/acs.chemmater.6b00230
Olejniczak, J., Carling, C.-J., and Almutairi, A. (2015). Photocontrolled Release Using One-Photon Absorption of Visible or NIR Light. J. Control. Release 219, 18–30. doi:10.1016/j.jconrel.2015.09.030
Rwei, A. Y., Wang, W., and Kohane, D. S. (2015). Photoresponsive Nanoparticles for Drug Delivery. Nano Today 10 (4), 451–467. doi:10.1016/j.nantod.2015.06.004
Satarkar, N., and Hilt, J. (2008). Magnetic Hydrogel Nanocomposites for Remote Controlled Pulsatile Drug Release. J. Control. Release 130 (3), 246–251. doi:10.1016/j.jconrel.2008.06.008
Schroeder, A., Kost, J., and Barenholz, Y. (2009). Ultrasound, Liposomes, and Drug Delivery: Principles for Using Ultrasound to Control the Release of Drugs from Liposomes. Chem. Phys. Lipids 162 (1−2), 1–16. doi:10.1016/j.chemphyslip.2009.08.003
Shanmugam, V., Selvakumar, S., and Yeh, C.-S. (2014). Near-infrared Light-Responsive Nanomaterials in Cancer Therapeutics. Chem. Soc. Rev. 43 (17), 6254–6287. doi:10.1039/c4cs00011k
Shi, C., Wu, Z., Yang, F., and Tang, Y. (2021a). Janus Particles with pH Switchable Properties for High-Efficiency Adsorption of PPCPs in Water. Solid State Sci. 119, 106702–106709. doi:10.1016/j.solidstatesciences.2021.106702
Shi, C., Zhang, X., Zhang, X., Chen, P., and Xu, L. (2021b). Ultrasonic Desulfurization of Amphiphilic Magnetic-Janus Nanosheets in Oil-Water Mixture System. Ultrason. Sonochemistry 76, 105662–125668. doi:10.1016/j.ultsonch.2021.105662
Shibaev, V., Bobrovsky, A., and Boiko, N. (2003). Photoactive Liquid Crystalline Polymer Systems with Light-Controllable Structure and Optical Properties. Prog. Polym. Sci. 28 (5), 729–836. doi:10.1016/s0079-6700(02)00086-2
Sortino, S. (2012). Photoactivated Nanomaterials for Biomedical Release Applications. J. Mat. Chem. 22 (2), 301–318. doi:10.1039/c1jm13288a
Swain, A. K., Pradhan, L., and Bahadur, D. (2015). Polymer Stabilized Fe3O4-Graphene as an Amphiphilic Drug Carrier for Thermo-Chemotherapy of Cancer. ACS Appl. Mat. Interfaces 7 (15), 8013–8022. doi:10.1021/acsami.5b02536
Tong, R., Tang, L., and Cheng, J. (2012). Development and Application of Anticancer Nanomedicine. Cheng J. 1, 31–46. doi:10.1007/978-1-4614-2305-8_3
Wang, X., Yang, Y., Gao, P., Yang, F., Shen, H., Guo, H., et al. (2015). Synthesis, Self-Assembly, and Photoresponsive Behavior of Tadpole-Shaped Azobenzene Polymers. ACS Macro Lett. 4, 1321–1326. doi:10.1021/acsmacrolett.5b00698
Wu, F., Li, Q., Zhang, X., Liu, L., Wu, S., Sun, D., et al. (2012). Fabrication and Characterization of Thermo-Sensitive Magnetic Polymer Composite Nanoparticles. J. Magnetism Magnetic Mater. 324, 1326–1330. doi:10.1016/j.jmmm.2011.11.032
Yao, Y., Yu, Y., Wan, X., Yan, D., Chen, Y., Luo, J., et al. (2021). Azobenzene-Based Cross-Linked Small-Molecule Vesicles for Precise Oxidative Damage Treatments Featuring Controlled and Prompt Molecular Release. Chem. Mat. 33, 7357–7366. doi:10.1021/acs.chemmater.1c01860
Keywords: Poly(N-isopropylacrylamide) (PNIPAM), magnetic nanoparticles, thermo-sensitive, light-sensitive, Poly(6-[4-(4-methoxy phenyl azo)-phenoxyl- hexyl methacrylate]) (PAzoMA)
Citation: Cui G, Wang H, Long S, Zhang T, Guo X, Chen S, Kakuchi T, Duan Q and Zhao D (2022) Thermo- and Light-Responsive Polymer-Coated Magnetic Nanoparticles as Potential Drug Carriers. Front. Bioeng. Biotechnol. 10:931830. doi: 10.3389/fbioe.2022.931830
Received: 29 April 2022; Accepted: 13 June 2022;
Published: 12 July 2022.
Edited by:
Junqing Wang, Sun Yat-sen University, ChinaReviewed by:
Shuangxi Xing, Northeast Normal University, ChinaSanjun Shi, Chengdu University of Traditional Chinese Medicine, China
Copyright © 2022 Cui, Wang, Long, Zhang, Guo, Chen, Kakuchi, Duan and Zhao. This is an open-access article distributed under the terms of the Creative Commons Attribution License (CC BY). The use, distribution or reproduction in other forums is permitted, provided the original author(s) and the copyright owner(s) are credited and that the original publication in this journal is cited, in accordance with accepted academic practice. No use, distribution or reproduction is permitted which does not comply with these terms.
*Correspondence: Qian Duan, ZHVhbnFpYW44OEBob3RtYWlsLmNvbQ==; Donghai Zhao, Y3VpeXVoYW4xOTgxXzBAc29odS5jb20=
†These authors have contributed equally to this work