- 1Liaoning Provincial Key Laboratory of Oral Diseases, School and Hospital of Stomatology, China Medical University, Shenyang, China
- 2Department of Plastic Surgery, The First Affiliated Hospital of China Medical University, Shenyang, China
Guided bone regeneration (GBR) is a widely used technique for alveolar bone augmentation. Among all the principal elements, barrier membrane is recognized as the key to the success of GBR. Ideal barrier membrane should have satisfactory biological and mechanical properties. According to their composition, barrier membranes can be divided into polymer membranes and non-polymer membranes. Polymer barrier membranes have become a research hotspot not only because they can control the physical and chemical characteristics of the membranes by regulating the synthesis conditions but also because their prices are relatively low. Still now the bone augment effect of barrier membrane used in clinical practice is more dependent on the body’s own growth potential and the osteogenic effect is difficult to predict. Therefore, scholars have carried out many researches to explore new barrier membranes in order to improve the success rate of bone enhancement. The aim of this study is to collect and compare recent studies on optimizing barrier membranes. The characteristics and research progress of different types of barrier membranes were also discussed in detail.
1 Introduction
Alveolar bone defect is a common oral disease. Insufficient alveolar bone caused by trauma, tumor, periodontitis, and long-time tooth absence has gradually become a huge challenge for the medical application of subsequent implantation, orthodontic, periodontal and functional repair treatments. The absence of natural teeth will cause the loss of functional stimulation of alveolar bone, resulting in progressive, cumulative and irreversible bone resorption, and the alveolar bone cannot maintain the bone contour, nor can it obtain the effect of mucosal support (Cawood and Howell, 1988). There are many ways to improve the alveolar bone, including guided bone regeneration, cleavage of the alveolar crest, bone compression, maxillary sinus lifting, distraction osteogenesis and autologous mass bone grafting (Fu and Wang, 2011; Mohan et al., 2015; Danesh-Sani et al., 2016; Sakkas et al., 2017; Rachmiel et al., 2018). At present, GBR technology is recommended to be used before or during the same period of dental implantation to expand and retain alveolar bone. It has become a widely recognized method for repairing alveolar ridge defects and is the most common bone augmentation technology with the longest clinical application time. After 8 years of follow-up, Kim et al. (2020) found that the success rate of implants followed by GBR treatment was about 77.8%. GBR technology expands the indications of oral implantation, guarantees the biological, aesthetic effects after implantation and restoration as well as reduces the incidence of complications.
It is well-known that the essential substances in the composition of animals and plants, such as proteins and cellulose, are polymer compounds. The ubiquity in the biological community determines their special status in the field of medicine, so they are most commonly used as medical materials. Biomedical polymer materials have the advantages of designable structure, good biological activity, stable physical properties, wide sources and low price. Therefore, as shown in Figure 1, polymers are always hot spots in the development of GBR applications and show an increasing trend year by year. However, it is worth noting that polymers should have high polymer purity, clean production environment and little residue of polymerization additives due to the presence of monomer impurities. Besides, chemical and mechanical properties shall meet the requirements of medical design and function. Such as hardness, elasticity, mechanical strength and fatigue strength. Finally, the material needs to be compatible with other materials like human tissues so that the implanted material has no side effect on body fluids for long-time use. According to their different uses in the medical field, they can be divided into five categories: 1) Materials that are not in direct contact with the tissues of the organism. 2) Materials in contact with skin and mucous membranes (Goodier et al., 2018). 3) Materials in short-term contact with human tissues (Liu et al., 2018). 4) Materials implanted in the body for a long time (Williams, 2008). 5) Pharmaceutical polymers (Ekladious et al., 2019).
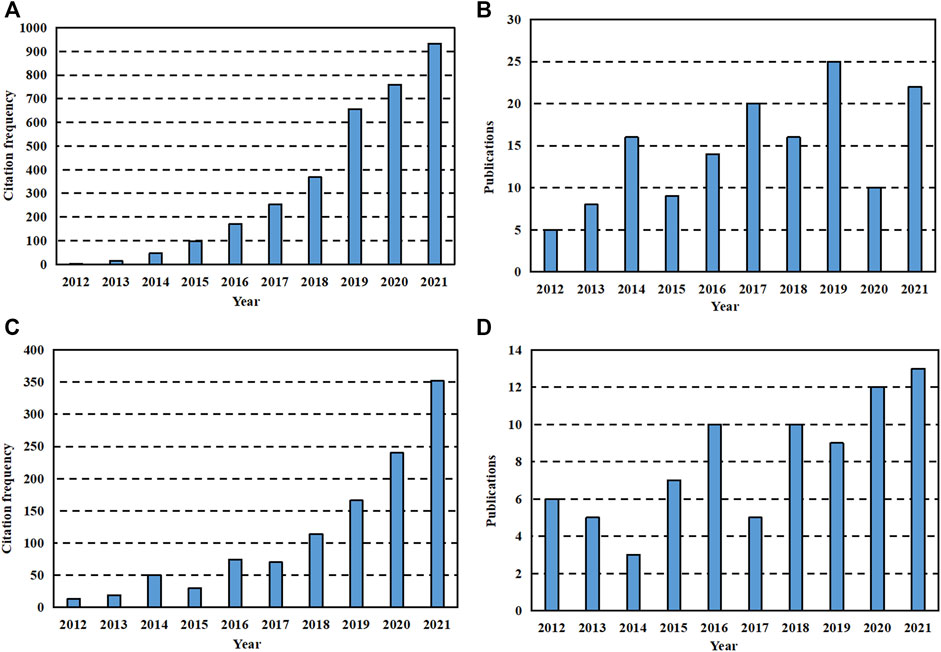
FIGURE 1. Data search results for last 10 years. (A) The citation frequency of literature with “GBR” and “polymer” as keywords retrieved on Web of Science in recent 10 years. (B) The publication of literature with “GBR” and “polymer” as keywords searched on Web of Science in recent 10 years. (C) The sum of citation frequency of literature retrieved on Web of Science with “GBR” and “ceramic” as one group of keywords as well as “GBR “and “metal” as another group of keywords in recent 10 years. (D) The sum of the publication of literature retrieved with “GBR” and “ceramic” as one set of keywords and “GBR “and “metal” as another set of keywords on Web of Science in recent 10 years.
2 Performance Requirements of Barrier Membranes in Guided Bone Regeneration Techniques
The principle of guided bone regeneration comes from guided tissue regeneration (GTR) in periodontium. The periodontal soft tissues such as epithelial cells and connective tissues proliferate and migrate relatively rapidly. GBR aims to insulate the soft tissues from the bone defect with a barrier membrane to provide a fairly closed environment for tissue growth. The cells with regenerative ability in the bone defect area proliferate and differentiate to the maximum extent to ensure the priority of osteogenesis and promote the formation of new bone (Elgali et al., 2017; Nowwarote et al., 2018). Selection of appropriate barrier membrane is the deciding factor in the success of guided bone regeneration. As seen in Table 1, ideal GBR barrier membrane should have good mechanical properties, including favorable space-making properties and clinical operability, favorable biological properties such as satisfactory biocompatibility, bioactive properties, tissue selectivity and antibacterial properties. How to better improve the peri-implant bone volume and further increase the degree of osseointegration is one of the most important properties for GBR.
2.1 Space-Making Properties and Clinical Operability
Spatial maintenance of clinical GBR technology requires attention to maintain osteogenic space, so the barrier membrane materials need to have a series of corresponding characteristics to ensure the space maintenance effect (Won et al., 2016). Firstly, it is required to have appropriate plasticity, which can provide a specific spatial structure to promote the functional reconstruction of alveolar bone according to different defect areas. In addition, the barrier membrane needs to meet a specific strength requirement to withstand external pressure, so adequate rigidity and tear resistance are essential. Meanwhile, the thickness of the barrier membrane is different, which affects the space maintenance characteristics during the implantation process. The practice has shown that placing a thicker collagen membrane can reduce the ingrown soft tissue and promote bone formation (Elgali et al., 2017). In practice, whether the stability of the graft can be maintained determines the success of GBR to a certain extent. Therefore, minimizing the movement of the barrier membrane in the operative area can effectively maintain a stable three-dimensional reconstruction spatial structure. In addition, the appropriately placement of barrier membrane and the bone meal implantation will also affect the effect of bone increment after bone grafting (Benic et al., 2019). The barrier membrane applied to GBR plays a crucial role in forming new bone tissue at the implantation site. Some scholars believe that in GBR technology, the barrier membrane is required to last 4–6 weeks, which is the requirement of regeneration time of total periodontal tissue (Moses et al., 2008). Some scholars also suggest that ideal GBR membrane should maintain its barrier function for 16–24 weeks to meet the requirement of bone tissue regeneration time (Hoornaert et al., 2016).
From the perspective of clinical practicability, GBR barrier membrane needs to meet the specific requirements of convenient for surgical operation. The barrier membrane needs to be easy to cover the bone defect area and conform to the adjacent bone surface. In terms of physical properties, if the barrier membrane is too hard, it may interfere with tissue integration in the bone defect area or lead to soft tissue dehiscence (Won et al., 2016; Aprile et al., 2020).
2.2 Biocompatibility and Bioactive Properties
Barrier membrane for GBR needs to have good biocompatibility to achieve the effect of supporting tissue regeneration. Good biocompatibility requires that it has no negative effects on the prognosis of peripheral cell tissue, bone defect area, and the patients’ overall health (Rakhmatia et al., 2013; Won et al., 2016). The interaction between barrier membrane and tissue has a positive effect on the surrounding tissue, which further leads to the healing of the defect. If the barrier membrane is absorbable, it should have the ability to degrade or integrate into host tissues, reducing the potential incompatibility caused by the barrier membrane.
Bioactivity refers to the ability of biomaterials to produce chemical bonding with living bone which is an important indicator to measure biomaterials. In the application of GBR, it is mainly reflected in the osteogenic capacity of barrier membranes. First, the barrier membrane itself can evoke a local environment in the defect, which is conducive to the regeneration and differentiation of osteoblasts. The environment created by the membrane is also conducive to the formation and reconstruction of the molecular mechanism of coupled bone in the submembrane defect (Omar et al., 2019). In addition, membrane bioactivity can be improved by designing the membrane structure (Liu et al., 2021). In recent years, it has also become an important way to enhance the osteoinductive ability of membranes by adding some inorganic particles, growth factors, etc. Common additives in vitro experiments are Sr-CaP nanoparticles (Ye et al., 2019), octacalcium phosphate (OCP) (Wang Y. et al., 2019), bone morphogenetic protein (BMP) (Yin et al., 2017a) and so on. Common additives in vivo experiments are metformin (Met) (Ebrahimi et al., 2021), epigallocatechin-3-gallate (EGCG) (Chu et al., 2019), etc. Barrier membranes will not only have a passive role but can play an active role at the site of bone defect regeneration (Hoornaert et al., 2016).
2.3 Tissue Selectivity
One of the critical points of the tissue-selective GBR technique is to promote the regeneration of bone tissue in the bone defect area while preventing the peripheral junction of tissue growth. This requires the relevant performance of the barrier membrane to be guaranteed. In terms of selective tissue growth, the barrier membrane should allow the passage of oxygen, tissue fluid and related bioactive substances but prevent the growth of connective tissue cells and epithelial cells into the defect area. In addition, a certain porosity is required to allow cells to adapt to their surroundings, and to provide cells with fully permeate nutrients (Murphy et al., 2000). Appropriate pore size can inhibit the growth of soft tissue and facilitate the diffusion of substances beneficial to the growth of bone tissue (Sheikh et al., 2017a). The selective healing process of the tissue defect can be achieved in two general directions. One is to promote growth which stimulates the growth of the tissue around the bone defect area. The other is to prevent growth which prevents the growth and implantation of epithelial connective tissue cells (Iviglia et al., 2019).
Tissue integration between the barrier membrane and the adjacent bone contour ensures effective adsorption to grow, contributing to the relative seal between the natural bone and the implant material. Tissue integration accelerates the wound healing process in the surgical area and helps prevent the integration of non-osteogenic components such as fibrous connective tissue into the defect site. The ability to create a membrane space of the material is key to achieving tissue integration. Abdelaziz et al. (2020) proposed that the barrier membrane also needed to have good osteoconductivity. That is to say, the compatibility of the old barrier membrane or scaffold with osteoclasts allows osteogenic cells to grow from the edge of the existing bone to form a new bone tissue structure and achieve tissue integration of bone. GBR surgery requires good soft tissue sealing and long-term wound stability to protect the regenerative process (Wang and Boyapati, 2006).
2.4 Antibacterial Properties
Antibacterial property is also a heated topic in the study of barrier membrane modification by scholars nowadays. Bacterial infection has been a common headache in our daily life or clinics. Bacterial adhere on the surface of medical materials, resulting in infections or even failure of materials or surgery operation (Behzadi et al., 2017; Jensen et al., 2017). It has been verified that the adverse effects of barrier membrane exposure on surrounding tissues are mainly due to bacterial invasion. Inflammatory response caused by bacterial invasion can inhibit the growth of osteoblasts, thus affecting the effect of guided bone regeneration and even leading to the failure of surgery. Researchers hoped to ensure or improve bone regeneration by preventing bacterial invasion (Cao et al., 2019). One of the main challenges of GBR restoration is bacterial colonization on the membrane, constitutes to premature membrane degradation (Saarani et al., 2017). Therefore, timely treatment once membrane exposure occurs during the GBR process can minimize the negative effects of exposure (Annibali et al., 2012), which also reflects that tissue integration plays an important role in the success of GBR. Non-resorbable membranes with different pore sizes have been used for exposure experiments. It was found that the osteogenic effect of non-resorbable membranes with pore sizes smaller than the general diameter of bacteria had not been significantly reduced even if they were exposed (Barboza et al., 2010).
3 Specific Types of Barrier Membranes
As shown in Figure 2, different types of barrier membranes can be applied to bone defects during the GBR procedure to play a certain role in osteogenesis. As shown in Figure 3, barrier membranes can be divided into two categories according to the composition, polymer membranes and non-polymer membranes. According to whether it is degradable or not, barrier membrane can be divided into absorbable membrane and non-absorbable membrane. Non-absorbable membranes are still in a minority of the current barrier membrane studies. Judging from the mechanical properties of several common barrier membranes in Table 2, single-component polymers tend to have lower mechanical properties than composite polymers. Both mechanical and biological properties can affect the stability and effectiveness of the barrier membranes. Based on the biological characteristics of several common barrier membranes listed in Table 3, the barrier membranes meet the requirements for the cytotoxicity of surgical implant materials (grade 0–1). Among the rest, PLGA and collagen have class 1 cytotoxicity but are considered biosafe. Their inflammatory responses were within the acceptable range. In addition, it is worth noting that chitosan inflammatory responses are correlated with molecular weight.
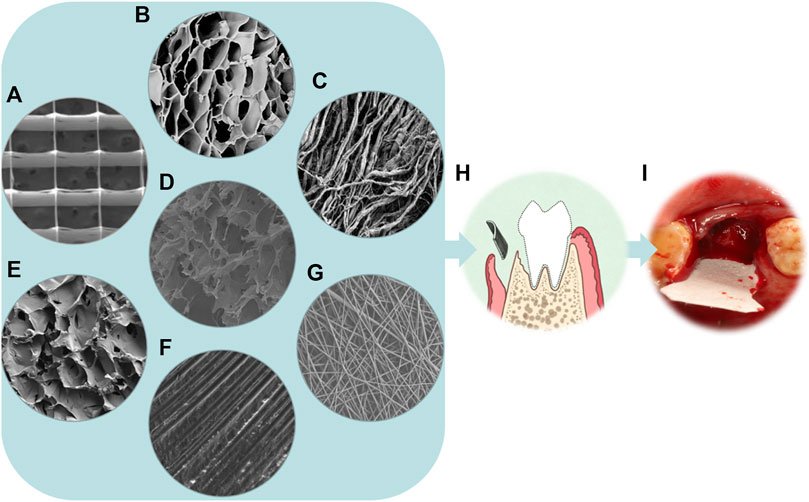
FIGURE 2. From microscopy to reality of barrier membranes. (A) SEM micrograph of the PCL scaffold with the spacing of 500 µm (Dubey et al., 2020). (B) SEM micrograph of the γ-PGA/BC composite hydrogel (Dou et al., 2021). (C) SEM image of a rougher bottom layer with collagen strands of Bio-Gide (magnification×1,000) (Wu et al., 2018). (D) SEM image showing the morphology of the loose layer of an asymmetric porous chitosan membrane (magnification×2,000) (Ma et al., 2016). (E) SEM image showing the morphology of the loose cross-linked collagen layer of the aspirin-loaded chitosan nanoparticles contained in collagen-chitosan membrane (ACS-CCM) (Zhang et al., 2017). (F) SEM image of the dPTFE membrane (magnification×500) (Korzinskas et al., 2018). (G) Field emission scanning electron microscopy(FE-SEM) images of PLGA/PCL electrospinning membranes (magnification×1,000) (Qian et al., 2016). (H) Schematic illustration of the principle of GBR. (I) The implant placement procedure for BioGuide membrane.
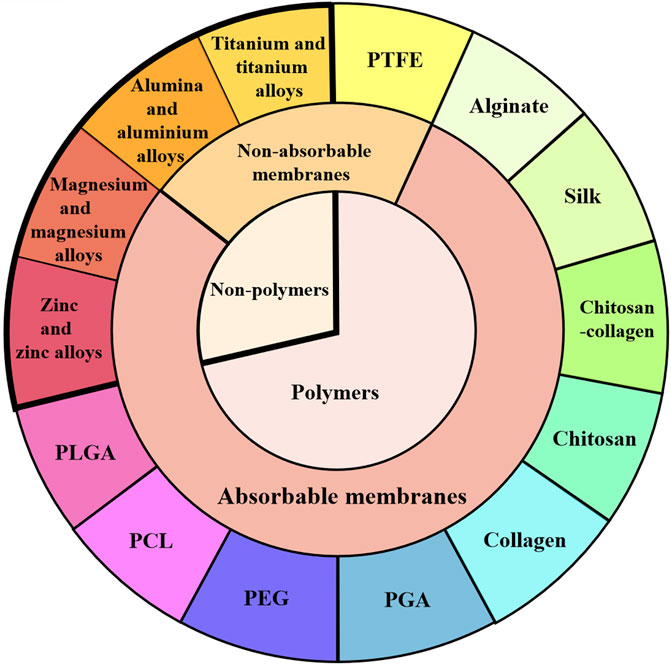
FIGURE 3. Classification of barrier membranes. GBR barrier membranes are classified from polymer and non-polymer, absorbable and non-absorbable barrier membranes. Several typical barrier membranes in each classification are introduced in the text of this paper.
3.1 Polymer Barrier Membranes
3.1.1 Poly(lactic-co-glycolic acid)(PLGA)
Polylactic acid (PLA) is a novel bio-based and renewable biodegradable material made from renewable plant resources such as straws like cereal husks and starchy crops like maize, sweet potato, and cassava. PLA is a good membrane material for treating bone defects. However, PLA membrane degrades rapidly, and the residue of PLA hydrolysis can cause local inflammation and abscess formation (Ovcharenko et al., 2020). Synthetic polymers of PLA have a higher potential to induce osteogenic differentiation and have been developed as an alternative to natural membranes (Jazayeri et al., 2017). PLGA consists of two monomers, lactic acid and hydroxyacetic acid, randomly polymerized. It is a degradable functional polymeric organic compound with good biocompatibility, non-toxicity, good capsule-forming and membrane-forming properties, which is widely used in pharmaceuticals, medical engineering materials and modern industries. The biodegradable synthetic barrier PLGA membrane generally consists of two layers: a dense layer that prevents invasion of soft tissue cells and the other is a thick microfibrous layer in which the blood clot is stabilized, allowing the blood clot to be stabilized osteoblasts to colonize the membrane. It has been suggested that the degradation rate of PLGA depends on the ratio of the two layers. Its general in vivo degradation time is 1–2months (Sun et al., 2017). Hoornaert et al. (2016) demonstrated that the bilayer PLGA membrane maintained structural integrity and barrier function for 16 weeks through in vitro and in vivo experiments. It was degraded by hydrolysis of polymer chains, which was easy to be applied with artificial bone filling particles for bone defect repair. They concluded that the bilayer PLGA membranes might be a safer and more predictable alternative to GBR. With the developing of PLGA barrier membranes, some composite PLGA material have come into use these years. Attapulgite (ATT) is a kind of natural clay material and an aluminum-magnesium silicate, which is widely distributed in China and the United States. ATT polymer composites have better mechanical durability than the corresponding pure polymers. Moreover, the hydrophilicity of the PLGA fiber membrane is improved with the addition of ATT, which can facilitate the penetration of hydrophilic nutrients and regulate cell response to the membrane surface. Xie X. et al. (2020) confirmed that electrospinning ATT doped PLGA fibrous scaffolds could induce the expression of osteogenic factors in bone marrow mesenchymal stem cells (BMSCs). By effectively promoting bone formation of alveolar bone defects, ATT doped PLGA was demonstrated to be an excellent barrier membrane material.
3.1.2 Polycaprolactone
PCL is a synthetic polymer known for its biocompatibility and excellent mechanical properties. Compared to PLGA, PCL has relatively lower cell affinity. However, with excellent mechanical properties, PCL has the advantage of preventing early fracture of the scaffold. It has been shown that adding silica nanoparticles (Si-NPs) into electrospun PCL membranes could greatly improve the mechanical and osteoconductive properties of the membranes (Castro et al., 2018). With good mechanical properties, PCL can be modified for other barrier membranes. For example, the PCL reticulation prepared by over melt electrowriting can not only delay hydrogel degradation and prevent soft tissue invasion, but also provide mechanical support for the regeneration and differentiation of progenitor cells (Dubey et al., 2020). The bioactivity of PCL was enhanced by mixing it with a natural silk fibroin polymer that has low immunogenicity and inherent bioactivity. It could promote bone regeneration not only in periodontal defects but also in other craniomaxillofacial regions. PCL can be modified by mixing with other materials through electrospinning technology. He et al. (2017) found that chitosan/PCL composite membrane significantly improved the ability of bone formation compared with PCL membrane.
3.1.3 Polyethylene Glycol
PEG is a biodegradable polymer with good biocompatibility. With good processability, PEG can be used as an ideal scaffold material and matrix material for other bone substitutes and blended with other polymers to make various new types of biological barrier membranes (Kim J. Y. et al., 2009; Jung et al., 2015). Wechsler et al. (2008) showed that PEG implantation maintained barrier function for up to 4 months in vivo. Some preclinical studies have found that PEG membranes have significant advantages for alveolar ridge bone augmentation in lateral ridge defects (Benic and Hämmerle, 2014). Jung et al. (2009a) used PEG membranes and collagen membranes for regeneration of peri-implant bone defects. They found that the PEG membrane group obtained more bone augmentation and could simplify clinical operation according to the shape of the membrane at the defect site. However, the postoperative exposure rate of PEG membranes (approximately 50%) was much higher than that of collagen membranes (12%) in their study. This may be due to the insufficient antimicrobial properties of the PGE membrane alone. Chitosan-based PEG can be synthesized by photochemical process to improve the thermal stability domain of PGE membrane, so that it has a strong inhibition effect on bacterial growth and reduce the membrane exposure rate (Sautrot-Ba et al., 2019).
3.1.4 Polyglycolic Acid
PGA is an aliphatic polyester polymer material with the least unit carbon count and the fastest degradation rate. Compared with the mainstream like PLA, the current price of PGA is relatively high and its market supply is small. PGA has good biocompatibility, which can be degraded into water and carbon dioxide in the human body, so it is widely used in surgical sutures, fracture internal fixation, tissue engineering repair materials and drug control release system. For its good degradation performance and its degradation product, PGA is rarely applied as a separate barrier membrane but applied by copolymerizating with other materials. The principle is that PGA degrades firstly, leaving pores for the polymer, exposing the active components, increasing the humoral contact area and accelerating the polymer degradation. For instance, biodegradable polymer PGA was blended into the hydroxyapatite/poly-l-lactic acid scaffold fabricated by laser 3D printing to accelerate the degradation (Shuai et al., 2020). A lot of pores produced by the degradation of the scaffold promoted the exposure of HAP from the matrix, which not only activated the deposition of bone like apatite on scaffold but also accelerated apatite growth. Otherwise, the PGA fibers have a significant effect on the physical properties of the collagen scaffolds. In vitro cell culture experiments showed that with the incorporation of PGA fibers, the number of attached cells increased higher than that of the collagen sponge (Toosi et al., 2019).
3.1.5 Collagen
Collagen is the main component of extracellular matrix. With particular cell adhesion sites, collagen has excellent properties on regulating cell morphology, adhesion, growth and differentiation. As one of the most widely used barrier membranes (Sbricoli et al., 2020), collagen has good biocompatibility and comes from a wide range of sources (Wessing et al., 2018). Combined with growth factors, collagen membrane is one of the ideal pre-coating membranes, which can induce new bone formation. Compared with Teflon membrane, the surface of collagen membrane is more attractive to osteoblasts and stimulates their proliferation.
Although collagen membrane has good biocompatibility, it still has problems such as irregular degradation, excessive degradation rate, poor spatial stability leading to tissue regeneration damage (Sheikh et al., 2016; Elgali et al., 2017; Tolstunov et al., 2019), lacking osteogenic potential (Zhou L. et al., 2021) and uncontrollable permeability, which can easily cause surgical site collapse (Sheikh et al., 2017b). There are several available methods for improvement. One is the preparation of nanofiber scaffold materials by electrospinning technology, which can obtain good surface area, mechanical strength and biomimetic effect. The material has better performance than polymer fiber (Cai et al., 2010). Second, multi-layer superposition to obtain good mechanical properties. Li et al. (2019) improved the performance of acellular porcine small intestinal submucosa (SIS) by cladding the lyophilized technique. Multi-layer superposition enables the membrane with mechanical solid support. No significant degradation was observed for 4 weeks, while the degradation was complete 12 weeks after implantation, which met the requirements of GBR technology to provide at least 4–6 weeks barrier effect (Moses et al., 2008). Third, collagen cross-linking. Collagen is cross-linked physically or chemically to improve collagen’s mechanical properties and stability used for GBR (Andrei et al., 2017). Fourth, collagen based composite scaffolds for bone regeneration are fabricated by the addition of different biological materials, such as bioceramic, carbon and polymer materials (Zhang et al., 2017). It has also been suggested that by concentrating collagen membranes, the barrier functions for up to 90 days. But it may lead to altered patterns of inflammatory tissue response (Gueldenpfennig et al., 2020).
Acellular dermal matrix (ADM) membrane is also a kind of collagen membrane. After removing cells in autologous or allogeneic skin tissue, the extracellular matrix with complete structure is retained, which can be used in repairing cartilage defects with type II collagen composite modification (Tiruvannamalai Annamalai et al., 2016). Studies have found that acellular matrix membrane can further promote the production of osteogenic chemokines by forming a local microenvironment conducive to the rapid recruitment of mesenchymal stem cells during bone remodeling. For example, C-X-C chemokine receptor type 4 (CXCR4) and monocyte chemoattractant protein-1 (MCP-1), etc. (Siddiqui et al., 2017; Jin et al., 2018). Momen-Heravi et al. (2018) applied ADM to repair peri-implant bone-cracking and found that new bone was formed around the bone defect area with fine effect of bone reconstruction.
Human amniotic membrane (HAM) is one of the oldest biomaterials used for wound healing in medicine, which consists of collagen and glycosaminoglycan (GAG) as main structural components (Rameshbabu et al., 2016). Similarly to HAM, chorionic membrane is four to five times thicker than HAM (Koob et al., 2015). HAM is a good source of growth factors (Grzywocz et al., 2014), and it is considered as a surgical waste (Fénelon et al., 2018), so it is a highly available and cost-efficient tissue that might be advantageous for bone regeneration. After conservation of extracellular matrix components in fresh and preserved HAM, Fenelon M et al. found that decellularization/lyophilization of HAM increased its mechanical properties and enhanced osteogenic differentiation of human bone marrow stromal cells (hBMSCs), which significantly increased early bone regeneration (Fenelon et al., 2020). Ghanmi et al. found that fresh acellular HAM promoted bone regeneration at the critical size of bone defect (Ghanmi et al., 2018). Besides creating a decellularized amnion/chorion membrane to improve the stiffness of this membrane (Koob et al., 2015), another possibility expected to further enhance the mechanical properties of HAM is to design a multilayered DL-HAM scaffold (Swim et al., 2019).
3.1.6 Chitosan
Chitosan, also known as deacetylchitin, is chemically known as polyglucosamine (1-4)-2-amino-B-D glucose. Chitosan has been widely used in bone tissue engineering in recent years because of its good permeability, antibacterial and immunomodulatory activity, and procoagulant wound healing properties (Ma et al., 2016; Tavelli et al., 2020). The porous scaffolds of chitosan reinforced with calcium phosphate can be used as a bone substitute for bone repair and dental fillings. Besides, chitosan can be degraded by lysozyme in organisms to generate natural metabolites, which are non-toxic and can be absorbed entirely by organisms. Therefore, it is superior to be used as a drug sustained-release agent and applied in controlled release system of drug and growth factors (Morris et al., 2010). Chitosan membrane was implanted subcutaneously in rats and was found to have the ability of maintaining barrier function for up to 6 weeks (Xu et al., 2012). The degradation rate, as well as the mechanical properties of chitosan membranes can be improved by changing their molecular weight and preparation methods. By electrostatic spinning technique, chitosan can be prepared into biofunctional composite with more rigid materials.
3.1.7 Chitosan-Collagen
The addition of chitosan to collagen scaffold material can change the cross-linking of collagen fibers and enhance the structure of the composite material. The scaffold has good bone conductivity, mechanical strength and biological stability (Martino et al., 2005). Collagen electrospun nanofiber is superior to a solid wall in cell proliferation and differentiation. Therefore, chitosan reinforced collagen nanofiber membrane can be used as a natural biological composite polymer to prepare bioabsorbable membrane for GBR purposes. In vivo experiments showed that the fibrous structure of chitosan-collagen nanofibrous scaffold material membrane improved the osteogenic differentiation of bone marrow mesenchymal stem cells (MSCs). Lotfi et al. (2016) developed a chitosan-collagen nanofiber scaffold material. The marginal bone maturation effect was better than that of the bio-guide membrane covered group, with normal inflammatory response, indicating good bone conduction, mechanical strength and biocompatibility. Chitosan has osteogenic properties (Thein et al., 2008) and good tissue separation (Bumgardner et al., 2007). It also has a specific modification effect on collagen biomechanical properties.
3.1.8 Silk
Silk, a macromolecule produced by Cocoon (Bombyx mori) or Spider (Nephila clavipes), can be made into a variety of biomaterials, including membranes, porous scaffolders, gels, suture materials and non-woven webs after purified (Liu et al., 2013; Qi et al., 2017). It is worth mentioning that silk fibroin (SF), a structural protein of silk, has high biocompatibility and less foreign body reaction (Midha et al., 2016; Saleem et al., 2020; Luo et al., 2021). Compared with collagen membrane, SF, as a new biomedical material, has good biocompatibility as well as excellent mechanical properties, controllable degradation and plasticity, which has been widely used in the research of bone tissue engineering. Lee et al. (2014) prepared a thin silk membrane with fiber network structure by a simple separation method. The tensile strength of the silk membrane is similar to that of the collagen membrane in dry state, while higher than that of the collagen membrane in wet state. It was found that SF membranes showed good bone regeneration in the skull defect model with less inflammation and similar volume of bone regeneration was observed compared with collagen membranes (Kim et al., 2014; Song et al., 2014). Yoo et al. (2016) proved that osteoblast-like MG63 cells could attach to, survive on, and proliferated on SF membrane, as a hint of its suitableness for the bone regeneration process. Due to the low cost of SF membranes and zero risk of infection transmission from animal tissues, their clinical use should be encouraged as an alternative to collagen membranes widely used in GBR.
3.1.9 Alginate
Alginate is salt derivatives of alginic acid and constitute long chains of polysaccharides, which provides pliability and gelling adeptness to their structure. It has favorable biocompatibility and can be cross-linked to form hydrogels to form structures similar to the extracellular matrix. It can be coated directly on the surface of the bone defect area. The alginate membrane provides a sound barrier between the connective tissue and the bone defect area. The main defect of alginate barrier membrane is its poor mechanical properties. The membrane often collapses towards the bone defect area, leading to the reduction of osteogenic effect. Therefore, alginate is suitable to be used with other materials with particular strength to prepare composite membranes or improve processing technology to overcome its shortcomings further. Take an effective interaction between the sodium alginate chain and the nanosized hydroxyapatite for an example (D'Elía et al., 2020). Higher nanoscale hydroxyapatite concentrations increase the length and branching of the polymer network, which in turn reduces the water content in the structure. The viscosity and strength of fresh hydrogels are increased while the plasticity of the membranes was reduced.
3.1.10 Poly Tetra Fluoroethylene
PTFE is a macromolecular compound, which is one of the most widely used polymers in polymer non-absorbable barrier membranes. PTFE membrane has good biocompatibility and can effectively protect blood clots, so it is regarded as the gold standard of barrier function materials (Jazayeri et al., 2017). PTFE membrane should ensure good tissue tightness in the treatment process. Expanded PTFE (e-PTFE) is a polytetrafluoroethylene treated by expansion or stretching, which has the sealing property of polytetrafluoroethylene. At the same time, the plastic deformation of modified polytetrafluoroethylene occurs under long-term continuous load. However, bacteria are easy to invade through the pores of the membrane and cause postoperative complications. If it is exposed to the human oral environment before the end of treatment, it will lead to treatment failure. Due to the occurrence of postoperative complications of membrane exposure caused by its easy bacterial invasion, it is gradually replaced by a smaller pore size Dense PTFE (d-PTFE) membrane. But, because the porosity of the d-PTFE membrane is limited, so the blood supply to this area is limited. Commercially available non-resorbable barrier membranes are usually made of Teflon. It is biocompatible, which maintains its integrity during and after implantation. It can not only treat significant, drug-free bone defects and multiwall defects, but also can affect vertical enhancement (Caballé-Serrano et al., 2018). Different from the previous view that PTEE is a bioinert material, Korzinskas et al. (2018) found that the severity of proinflammatory and anti-inflammatory PTEE was equivalent to that of collagen membrane with good biocompatibility. It is not wholly a bioinert material, which can best support bone healing in the context of inducing bone regeneration.
3.1.11 Other Polymers
Some other polymers can also be used in the fabrication of barrier membranes. Hyaluronic acid (HA), a polyanionic natural polymer, is one of the main components of the hydrophilic polymer and extracellular matrix (Sudha and Rose, 2019). Hyaluronic acid membranes were widely used in the field of orthopedics due to the effectiveness in wound healing. Studies have indicated that HA membrane has an effect close to that of the collagen (Yilmaz et al., 2021). Furthermore, membranes based on HA derivatives hold great potential to stimulate mineralization for the periodontal regeneration (Federico et al., 2021), which can be investigated for periodontal barrier applications and used to achieve bone regeneration (Park et al., 2009). Ayanoğlu et al. (2015) conducted a study on the combined use of HA membrane and allograft on bone defects of rabbits. Results indicated that the use of HA membranes alone or in combination with graft can promote bone healing.
Poly(3-hydroxybutyrate) (PHB) is a linear of naturally produced polyesters, unbranched homopolymer consisting of (R)-3-hydroxybutyric acid units, which stored in cells by prokaryotic microorganisms under the condition of nutritional imbalance (Valappil et al., 2007). It also has the characteristics of natural degradable materials and the advantages of synthetic degradable materials (Rodriguez-Contreras, 2019). Karahaliloğlu et al. (2015) fabricated NaOH treated PHB membranes, which showed increased proliferation of human osteoblasts for GBR applications. Partial bone regeneration can be observed in bifurcation defects in dogs by the application of rigid absorbable membranes made of hydroxyapatite and PHB (Reis et al., 2013). In addition, PHB not only degrades into non-toxic oligomers (Chiulan et al., 2017), but also has been found to have positive effects on mesenchymal stem cells (Voinova et al., 2019), which might be a suitable candidate for in vivo use in medical applications.
3.2 Non-Polymer Barrier Membranes
3.2.1 Magnesium and Magnesium Alloys
Compared with other orthopedic degradable materials, the local magnesium-rich environment generated by the degradation of magnesium-based materials can activate different signaling pathways through various signal mediations. The environment can stimulate bone regeneration, improve the adhesion rate of osteoblasts, inhibit the activity of osteoclasts and regulate the signal transduction of osteogenic cells. Magnesium alloy has relatively active chemical properties and is easily degraded and absorbed in the physiological environment. Its degradation can promote calcium deposition. The rate of osteogenesis is fast in the early stage of osteogenesis. Its bone-guiding property is better than titanium as well (Rahman et al., 2020). In addition, magnesium has Young’s modulus similar to that of human bone, which can avoid stress shielding. Thereby the surrounding natural bone can be transferred to the defect and stimulating bone remodeling (Dutta et al., 2020). Different from titanium, magnesium alloy products can self-degrade after bone tissue repair and regeneration. It can promote bone tissue healing because magnesium ion is an essential element for the human body, which is convenient for clinical promotion. In addition, magnesium alloy does not interfere with CT and MRI imaging examination after surgery (Farraro et al., 2014), which facilitates the effect after implantation. Si et al. (2021) have studied a Mg-2.0Zn-1.0Gd alloy (wt%, MZG) membrane for biomedical use. The alloy material has a favorable plastic deformation ability. In order to improve the biological corrosion resistance and cytotoxicity in vitro, a biodegradable magnesium alloy GBR Mesh plate with 0.6 mm Ca-P coating was developed. Experiments indicate that MZG has excellent potential for clinical application as GBR membranes.
The degradation rates of magnesium alloy are too fast and the degradation in vivo produces hydrogen evolution reaction, causing increase in local PH. It affects the growth of the surrounding tissue, hemolysis, dissolved bone happens, even severely limited the application of magnesium alloy in clinic. Modification of magnesium alloys can be achieved by adding alloying elements as well as surface modification. Physical modification, such as modifying microstructural features, can effectively slow down the degradation of magnesium alloy in vitro and in vivo, reduce local hydrogen evolution and pH raise, which ulteriorly improves its biocompatibility (Wu et al., 2019; Saberi et al., 2021). Magnesium alloys can be modified by integrating with other materials such as collagen. Barbeck et al. (2020) embedded HF-treated magnesium-based meshes in a collagen membrane in order to combine the mechanical properties of degradable metal with the biocompatibility of collagen membrane. However, Steigmann et al. (2020) believed that even if the integration behavior of collagen membrane was comparable, pure magnesium membrane could still meet the requirements of biocompatibility.
3.2.2 Zinc and Zinc Alloys
As an essential trace element, Zinc involves in a variety of essential biological functions such as nucleic acid metabolism, signal transduction, apoptosis regulation and gene expression (Dermience et al., 2015; Lin et al., 2017). An vitro study showed that zinc degradation in saline solution did not have a large effect on the surrounding cultures and that the zinc corrosion rate was accelerated after 120 h due to passivation (Meng et al., 2018). Zinc is a clinically ideal material with an intermediate degradation rate between magnesium and iron. Zinc plays a crucial role in the growth and mineralization of bone tissue, which directly activates aminoacyl tRNA synthetase in osteoblasts (Seo et al., 2010) and stimulates cellular protein synthesis (Storrie and Stupp, 2005). In addition, zinc inhibits bone resorption by inhibiting the formation of osteoclast-like cells in bone marrow cells (Frederickson et al., 2005). Zinc also plays an essential role in the preservation of bone mass (Amin et al., 2020). Guo et al. (2020) concluded that microporous pure zinc membranes of 300 μm have superior MC3T3-E1 cytocompatibility in vitro and osteogenic capacity in vivo, which have potential bone regeneration applications in GBR membranes.
3.2.3 Titanium and Titanium Alloys
As a non-resorbable metal barrier membrane, titanium mesh can be used as a barrier membrane alone. It can also be used to strengthen absorbable collagen membrane and enhance polytetrafluoroethylene, etc. Good biomechanical properties of titanium membrane (Alagl and Madi, 2018) can effectively maintain osteogenic space and prevent the migration of epithelial and connective tissue cells. It has been widely used to reconstruct significant jaw defects and alveolar bone defects (Abdel-Hady Gepreel and Niinomi, 2012). Bai et al. (2019) found through 3D printing technology that the thickness and aperture of titanium mesh affected the amount of new bone under the titanium mesh. Appropriate pore size and thickness played a crucial role in promoting the growth of bone tissue. It has been concluded that 0.4 mm thickness titanium mesh not only carries enough strength but also has less stimulation to the mucosa and is more suitable for clinical use. Alagl and Madi (2018) combined a nano-bone graft and alloplastic with Ti-mesh for local Ridge Augmentation. It seems to be a clinically feasible method to repair soft tissue and complex tissue defects in a relatively short time so that suitable implants can be implanted in a short period.
3.2.4 Alumina and Aluminium Alloys
In physiological environment, alumina is an inert material matrix, which is non-absorbable and extremely hard. Al2O3 appeared to have no significant cytotoxic effect (Jamieson et al., 2021), however, aluminium is not considered to exhibit osteogenic properties and even recognized for having adverse effects on osteoblasts (Yang et al., 2018). To overcome these limitations, some composites have been developed from two or more materials to obtain the excellent properties of a single material. For instance, bioglass and hydroxyapatite-coated porous alumina scaffolds showed higher biocompatibility and osteointegration responses compared with pure alumina scaffolds (Camilo et al., 2017). In fact, alumina is more commonly used as a sandblasting coating in dentistry. The use of particles of different sizes to obtain regular roughness values results in changes in osteoblast behavior and binding to bone (Kim et al., 2006). It also stimulates calcium to flow out of the bones (Bushinsky et al., 1995). Studies showed that titanium aluminium vanadium (TiAIV) implants sandblasted with Al2O3 showed higher stability ratio (ISQ) and removal torque values than TiO2 and SiO2 at the end of the first and third months, and had a better effect on bone bonding (Yurttutan and Keskin, 2018).
4 Functional Membranes
With the development of barrier membrane, absorbable functional membrane has received increasing attention these years. There are three main construction methods: 1) Loading antibacterial materials to reduce the repair failure rate caused by inflammation; 2) Loading bioactive factors to increase bone mass through their own osteogenesis and synergistic osteogenesis; 3) The multilayer structure is manufactured to meet different requirements of different contact surfaces such as implant surface, bone surface and epithelial tissue in bone regeneration.
4.1 Antibacterial Barrier Membranes
When loaded with antibiotics, growth factors and adhesion factors, the synthetic membrane can be used as a delivery device for specific preparations. To ensure the effectiveness of guided bone regeneration, it is necessary to reduce inflammatory response caused by bacterial invasion. First, antibiotics were added to the barrier membrane surface. Antibiotics in the barrier membrane can effectively reduce the risk of postoperative infection. Moreover, antibiotics can slow down collagen degradation. There are many antibacterial materials can be loaded onto the barrier membranes. PEG, superhydrophobic structural coating, biomembrane matrix-degrading enzyme coating, silver-releasing ion coating, titanium dioxide photoactive coating, chlorhexidine and other substances are widely used in the fabrication of antibacterial barrier membranes. Second, the application of structural drug-loaded sustained-release systems. At present, electrospinning technology, as a traditional textile industry applying polymer technology, is introduced as a novel production strategy for nanomimetic scaffolds in the field of tissue engineering. It is also a promising controllable drug delivery system, which allows the addition of therapeutic agents to the mesh eye of nonwoven nanofibers during electrospinning. Polymer nanoparticles, nanotubes, micelles, and lipid nanoparticles can be combined with electrospun nanofibers to improve the release profile, drug safety, loading efficiency and better functionality of the fibers. The commonly used therapeutic agents are aspirin (Ghavimi et al., 2020), azithromycin (Wang Z. et al., 2019), metronidazole (He et al., 2018), antibacterial peptide (Wei et al., 2018) and so on. In addition, composite encapsulating antibacterial agents can be prepared by cross-linking technology and may even be used as drug carriers in pH-responsive controlled release drug delivery systems (Mathew et al., 2017; Bi et al., 2019).
4.2 Bioactive Barrier Membranes
The main research hotspot of modern tissue engineered bone construction is how to control a variety of growth factors with different biological activities in time to play a role in different stages of bone healing and mimic the natural osteogenesis process. It is currently proved that the most important factors are osteogenic factors and angiogenic factors. In recent years, the commonly used pro-bone tissue growth factors include bone morphogenetic protein (BMP), vascular endothelial growth factor (VEGF), platelet-derived growth factor (PDGF), insulin-like growth factor (IGF), and transforming growth factor (TGF). BMP is one of the most widely used and studied growth factors for bone tissue engineering. During bone remodeling, signal analysis of the temporal and spatial expression of BMP genes showed that they were very similar to osteogenesis and fracture healing. BMP-2 is one of the relatively known bone formation effects among all growth factors, which has the ability to promote the directional differentiation and proliferation of mesenchymal stem cells into osteoblasts. It can be combined with a variety of carriers with different properties to compose various types of bioactive restorative materials. BMP-loaded carriers can be supramolecular hydrogels (Tan et al., 2019), core-shell nanofibers (da Silva et al., 2019), acellular collagen sponges (Sun T. et al., 2018), etc. The BMP-loaded barrier membrane can obtain site preservation after tooth extraction and obtain bone enhancement at the bone defect site. Among them, the amount of bone enhancement was proportional to the dose of recombinant human BMP-2 (rhBMP-2) (Sun Y.-K. et al., 2018). Thoma et al. (2018) demonstrated that rhBMP-2-loaded xenograft bone blocks showed little difference in results at 4 months compared with autologous bone blocks that were considered as the gold standard, though autologous bone blocks were more mineralized. However, in recent years, rhBMP-9 has shown better osteogenic differentiation ability compared with rhBMP-2 (Fujioka-Kobayashi et al., 2017). Another important growth factor, VEGF, is considered to be the most potent growth factor known to induce angiogenesis (Zhang et al., 2021). It not only affects the differentiation of osteoblasts, but also plays an important role in cartilage resorption and promoting the early and late chondroossification of angiogenesis. The combined use of VEGF and rhBMP-2 can increase angiogenesis and blood supply, promote the formation of new bone and solve the problem of vertical bone regeneration in clinical work (Schorn et al., 2017). There is also TGF (Tan et al., 2021) similar to BMP synergistic osteogenesis. In addition to growth factors, some animal and plant active components such as BMP-2-related peptide P28 (Sun T. et al., 2018), amelogenin (EMD) (Miron et al., 2017) and Icariin (ICA) (Yin et al., 2017b) have also been applied in bone tissue engineering.
4.3 Structurally Layered Barrier Membranes
Multilayer barrier membranes have been designed to meet more complex requirements to endow the barrier membranes with more affluent properties. However, although the membranes are divided into multiple layers, they should still overall meet the primary purpose of GBR, so each layer should have different biological characteristics. For example, the pore size of the inner layer (loose layer) should be large enough to meet the specific requirements of the contact surface between barrier membrane and epithelial tissue, which can stabilize the blood clot and promote the integration of soft tissue in a double-layer membrane. The outer layer (dense layer) has a relatively small pore size to maintain the osteogenic space, block the entry of soft tissue cells in the same time and allow the stem cells and nutrients to pass through. In addition, there is a gap between the bilayer structure to facilitate tissue integration (Rothamel et al., 2012). Kim S.-H. et al. (2009) prepared a double-layer collagen membrane and implanted them in rats. They found that compared with the monolayer collagen membrane, the non-crosslinked collagen membrane bilayer technique could improve the bone resorption and bone strengthening effect of the embedded bone grafting technique. Tai et al. (2014) prepared and found a polyhydroxybutyrate-biphasic calcium phosphate/chitosan membrane that had the role of guiding bone regeneration barrier membranes in periodontal tissue engineering. The membrane developed by Sheikh et al. (2016) had one surface of polyether urethane (PEU), while the other side was hydroxy-terminated polydimethylsiloxane (PDMS) coated with PEU. The non-PDMS coated side adsorbed proteins, which could repair defects in periodontal tissue regeneration and attract growth factors, while the PDMS coated side acted as a barrier for gingival epithelial cells to prevent proliferation and migration of gingival epithelial cells into the defect gap through the soft tissue flap. PCL/PLGA scaffolds obtained biological and mechanical advantages during the mixing process, making up for their respective shortcomings (Kim J. Y. et al., 2009). Abe et al. (2020) made a double phase-out membrane by solid. The PLGA and PCL polymerization method of controlling the membrane degradation can reduce the cost of placing two or more than two membranes in the clinic and reduce the waste of membrane barrier materials.
5 Common Processing Techniques for Barrier Membranes
The barrier membrane processing techniques include electrostatic spinning (Yin Y. et al., 2017), 3D printing (Bai et al., 2019), chemical crosslinking (Oryan et al., 2018), phase inversion (Fu et al., 2017) and other technologies. All kinds of preparation methods have different characteristics. Among them, electrospinning and 3D printing are the most widely used preparation technologies.
Electrospinning technology refers to a method in which polymer solution or melt produce jet stretching under the action of high-pressure electrostatic field force to obtain ultrafine fiber by solvent volatilization or melt curing. This technique can be used to prepare natural polymers such as collagen, SF, alginate; polymers such as PLGA, PCL, PEG, PGA, PTFE; inorganic materials such as alumina, zinc oxide; composite materials such as chitosan-collagen. The nanofibrous film it prepared has a very large specific surface area and a very high porosity (Henry et al., 2017). Compared with traditional materials, electrospun nanofiber scaffolds not only have good diversity and high parameter controllability, but also have unique advantages in personalized applications under different tissues and physiological conditions (Zhang et al., 2012). However, it is still difficult to conduct large-scale nanofiber production because of the slow electrospinning speed, low yield, and nanofiber with too small diameter cannot be obtained. The industrialization research of electrospinning industry should be strengthened in the future.
3D printing is a kind of fast forming technology, based on mathematical model documents (Trenfield et al., 2019). It uses metal powder, plastic and other adhesive materials to make products through heat sources such as laser. 3D printed granular bone graft could better maintain bone defects and support barrier membrane without corresponding clinical tests instead. The design of 3D printed custom titanium mesh avoids nerves and blood vessels, which is important for improving the precise reconstruction of GBR and providing enough space for implantation to reduce exposure rates (Zhou T. et al., 2021). In addition, 3D printing technology is also promising for polymers (Hwang et al., 2017). Park (Park et al., 2018) used 3D printing technology to print a three-dimensional PCL stent adapted to the bone defect area of the animal model and implanted it with β-tricalcium phosphate (β-TCP) powder which successfully maintained the physical space of the bone defect site and promoted the postoperative regeneration of healthy bone without an inflammatory or infectious response. However, while 3D printing solves the needs of personalization and precision in implantation, there are still some problems, such as higher cost, more complicated individual printing process and still deviation, etc.
6 Prospects of the GBR Technique
There are many shortcomings in current GBR technology. First, barrier failure may occur when applying membranes. One scenario is that the membrane is exposed to the oral environment due to poor soft tissue closure. Another circumstance is that the soft tissue is well sealed but the absorbable membrane degrades and loses the barrier protection function. Moreover, it relies mainly on the body’s growth potential to repair the defect. If the activity and number of stem cells are insufficient, it will difficult to predict the osteogenic effect. Therefore, it is difficult to gain satisfactory osteogenic results, if the defect is large or the body is in poor condition.
With the fast development of GBR, further improvement on barrier membrane will become to one of the most important fields in bone regeneration. One is to improve the physical and chemical properties of the membrane to enhance the barrier function, such as the use of new processing methods, preparation of multilayer structure. The other is try to improve the ability of osteogenesis, such as loading drugs and various growth factors, combining osteogenesis and angiogenesis, further reducing the immune response and so on. By enhancing the use of growth factors in the barrier membrane, GBR will improve bone regeneration effects. If the bone mass and bone are stable, it is prospective that GBR could be extended to improve bone augmentation outside the mouth. For example, GBR may become the normally auxiliary means for repairing systemic tissue defect, such as the non-benign bone defect of diabetes and osteoporosis patients, even more extensive defect repair after injury.
Author Contributions
QW and DZ contributed to conception and design of the study. ZY and CW wrote the manuscript. HS edited the manuscript. All authors contributed to manuscript revision, read, and approved the submitted version.
Funding
This work was supported by Liaoning Science and Technology Program (No. 2021-MS-185); The Innovation and Entrepreneurship Training Program for China Medical University (x202110159201); Shenyang Science and Technology Program (No. 19-112-4-029).
Conflict of Interest
The authors declare that the research was conducted in the absence of any commercial or financial relationships that could be construed as a potential conflict of interest.
Publisher’s Note
All claims expressed in this article are solely those of the authors and do not necessarily represent those of their affiliated organizations, or those of the publisher, the editors and the reviewers. Any product that may be evaluated in this article, or claim that may be made by its manufacturer, is not guaranteed or endorsed by the publisher.
References
Abdel-Hady Gepreel, M., and Niinomi, M. (2013). Biocompatibility of Ti-Alloys for Long-Term Implantation. J. Mech. Behav. Biomed. Mater. 20, 407–415. doi:10.1016/j.jmbbm.2012.11.014
Abdelaziz, D., Hefnawy, A., Al-Wakeel, E., El-Fallal, A., and El-Sherbiny, I. M. (2021). New Biodegradable Nanoparticles-In-Nanofibers Based Membranes for Guided Periodontal Tissue and Bone Regeneration with Enhanced Antibacterial Activity. J. Adv. Res. 28, 51–62. doi:10.1016/j.jare.2020.06.014
Abe, G. L., Sasaki, J.-I., Katata, C., Kohno, T., Tsuboi, R., Kitagawa, H., et al. (2020). Fabrication of Novel Poly(lactic Acid/caprolactone) Bilayer Membrane for GBR Application. Dent. Mater. 36 (5), 626–634. doi:10.1016/j.dental.2020.03.013
Açil, Y., Zhang, X., Nitsche, T., Möller, B., Gassling, V., Wiltfang, J., et al. (2014). Effects of Different Scaffolds on Rat Adipose Tissue Derived Stroma Cells. J. Cranio-Maxillofacial Surg. 42 (6), 825–834. doi:10.1016/j.jcms.2013.11.020
Al-Hamed, F. S., Mahri, M., Al-Waeli, H., Torres, J., Badran, Z., and Tamimi, F. (2019). Regenerative Effect of Platelet Concentrates in Oral and Craniofacial Regeneration. Front. Cardiovasc. Med. 6, 126. doi:10.3389/fcvm.2019.00126
Alagl, A. S., and Madi, M. (2018). Localized Ridge Augmentation in the Anterior Maxilla Using Titanium Mesh, an Alloplast, and a Nano-Bone Graft: a Case Report. J. Int. Med. Res. 46 (5), 2001–2007. doi:10.1177/0300060518758226
Amin, N., Clark, C. C. T., Taghizadeh, M., and Djafarnejad, S. (2020). Zinc Supplements and Bone Health: The Role of the RANKL-RANK axis as a Therapeutic Target. J. Trace Elem. Med. Biol. 57, 126417. doi:10.1016/j.jtemb.2019.126417
Andrei, M., Dinischiotu, A., Didilescu, A. C., Ionita, D., and Demetrescu, I. (2018). Periodontal Materials and Cell Biology for Guided Tissue and Bone Regeneration. Ann. Anat. - Anatomischer Anzeiger 216, 164–169. doi:10.1016/j.aanat.2017.11.007
Tiruvannamalai Annamalai, R., Mertz, D. R., Daley, E. L. H., and Stegemann, J. P. (2016). Collagen Type II Enhances Chondrogenic Differentiation in Agarose-Based Modular Microtissues. Cytotherapy 18 (2), 263–277. doi:10.1016/j.jcyt.2015.10.015
Annibali, S., Bignozzi, I., Sammartino, G., La Monaca, G., and Cristalli, M. P. (2012). Horizontal and Vertical Ridge Augmentation in Localized Alveolar Deficient Sites: a Retrospective Case Series. Implant Dent. 21 (3), 175–185. doi:10.1097/ID.0b013e31824ee3e9
Aprile, P., Letourneur, D., and Simon‐Yarza, T. (2020). Membranes for Guided Bone Regeneration: A Road from Bench to Bedside. Adv. Healthc. Mat. 9 (19), 2000707. doi:10.1002/adhm.202000707
Ayanoglu, S., Esenyel, C. Z., Adanır, O., Dedeoğlu, S., İmren, Y., and Esen, T. (2015). Effects of Hyaluronic Acid (Hyalonect) on Callus Formation in Rabbits. Acta Orthop. Traumatol. Turc 49 (3), 319–325. doi:10.3944/AOTT.2015.14.0231
Bai, L., Ji, P., Li, X., Gao, H., Li, L., and Wang, C. (2019). Mechanical Characterization of 3D-Printed Individualized Ti-Mesh (Membrane) for Alveolar Bone Defects. J. Healthc. Eng. 2019, 1–13. doi:10.1155/2019/4231872
Barbeck, M., Kühnel, L., Witte, F., Pissarek, J., Precht, C., Xiong, X., et al. (2020). Degradation, Bone Regeneration and Tissue Response of an Innovative Volume Stable Magnesium-Supported GBR/GTR Barrier Membrane. Ijms 21 (9), 3098. doi:10.3390/ijms21093098
Barboza, E. P., Stutz, B., Ferreira, V. F., and Carvalho, W. (2010). Guided Bone Regeneration Using Nonexpanded Polytetrafluoroethylene Membranes in Preparation for Dental Implant Placements-A Report of 420 Cases. Implant Dent. 19 (1), 2–7. doi:10.1097/ID.0b013e3181cda72c
Behzadi, S., Luther, G. A., Harris, M. B., Farokhzad, O. C., and Mahmoudi, M. (2017). Nanomedicine for Safe Healing of Bone Trauma: Opportunities and Challenges. Biomaterials 146, 168–182. doi:10.1016/j.biomaterials.2017.09.005
Benic, G. I., Eisner, B. M., Jung, R. E., Basler, T., Schneider, D., and Hämmerle, C. H. F. (2019). Hard Tissue Changes after Guided Bone Regeneration of Peri‐implant Defects Comparing Block versus Particulate Bone Substitutes: 6‐month Results of a Randomized Controlled Clinical Trial. Clin. Oral Impl Res. 30 (10), 1016–1026. doi:10.1111/clr.13515
Benic, G. I., and Hämmerle, C. H. F. (20142000). Horizontal Bone Augmentation by Means of Guided Bone Regeneration. Periodontol. 2000 66 (1), 13–40. doi:10.1111/prd.12039
Bi, Y.-g., Lin, Z.-t., and Deng, S.-t. (2019). Fabrication and Characterization of Hydroxyapatite/sodium Alginate/chitosan Composite Microspheres for Drug Delivery and Bone Tissue Engineering. Mater. Sci. Eng. C 100, 576–583. doi:10.1016/j.msec.2019.03.040
Bozkurt, A., Apel, C., Sellhaus, B., van Neerven, S., Wessing, B., Hilgers, R.-D., et al. (2014). Differences in Degradation Behavior of Two Non-cross-linked Collagen Barrier Membranes: an in Vitroandin Vivostudy. Clin. Oral Impl. Res. 25 (12), 1403–1411. doi:10.1111/clr.12284
Bumgardner, J. D., Chesnutt, B. M., Yuan, Y., Yang, Y., Appleford, M., Oh, S., et al. (2007). The Integration of Chitosan-Coated Titanium in Bone: an In Vivo Study in Rabbits. Implant Dent. 16 (1), 66–79. doi:10.1097/ID.0b013e3180312011
Bushinsky, D. A., Sprague, S. M., Hallegot, P., Girod, C., Chabala, J. M., and Levi-Setti, R. (1995). Effects of Aluminum on Bone Surface Ion Composition. J. Bone Min. Res. 10 (12), 1988–1997. doi:10.1002/jbmr.5650101219
Caballé-Serrano, J., Munar-Frau, A., Ortiz-Puigpelat, O., Soto-Penaloza, D., Peñarrocha, M., and Hernández-Alfaro, F. (2018). On the Search of the Ideal Barrier Membrane for Guided Bone Regeneration. J. Clin. Exp. Dent. 10 (5), e477–e483. doi:10.4317/jced.54767
Cai, Y. Z., Wang, L. L., Cai, H. X., Qi, Y. Y., Zou, X. H., and Ouyang, H. W. (2010). Electrospun Nanofibrous Matrix Improves the Regeneration of Dense Cortical Bone. J. Biomed. Mat. Res. 95A (1), 49–57. doi:10.1002/jbm.a.32816
Camilo, C. C., Silveira, C. A. E., Faeda, R. S., de Almeida Rollo, J. M. D., de Moraes Purquerio, B., and Fortulan, C. A. (2017). Bone Response to Porous Alumina Implants Coated with Bioactive Materials, Observed Using Different Characterization Techniques. J. Appl. Biomaterials Funct. Mater. 15 (3), 223–235. doi:10.5301/jabfm.5000347
Cao, Y. B., Liu, C., Pan, W. L., Tu, Y., Li, C. J., and Hua, C. G. (2019). Research Progress on the Modification of Guided Bone Regeneration Membranes. Hua Xi Kou Qiang Yi Xue Za Zhi 37 (3), 325–329. Chinese[Article in Chinese]. doi:10.7518/hxkq.2019.03.019
Cao, Y., and Wang, B. (2009). Biodegradation of Silk Biomaterials. Ijms 10 (4), 1514–1524. doi:10.3390/ijms10041514
Carbonell, J. M., Martín, I. S., Santos, A., Pujol, A., Sanz-Moliner, J. D., and Nart, J. (2014). High-density Polytetrafluoroethylene Membranes in Guided Bone and Tissue Regeneration Procedures: a Literature Review. Int. J. Oral Maxillofac. Surg. 43 (1), 75–84. doi:10.1016/j.ijom.2013.05.017
Carlo Reis, E. C., Borges, A. P. B., del Carlo, R. J., Oliveira, P. M., Sepúlveda, R. V., Fernandes, N. A., et al. (2013). Guided Tissue Regeneration Using Rigid Absorbable Membranes in the Dog Model of Chronic Furcation Defect. Acta Odontol. Scand. 71 (3-4), 372–380. doi:10.3109/00016357.2012.680909
Castro, A. G. B., Diba, M., Kersten, M., Jansen, J. A., van den Beucken, J. J. J. P., and Yang, F. (2018). Development of a PCL-Silica Nanoparticles Composite Membrane for Guided Bone Regeneration. Mater. Sci. Eng. C 85, 154–161. doi:10.1016/j.msec.2017.12.023
Cawood, J. I., and Howell, R. A. (1988). A Classification of the Edentulous Jaws. Int. J. Oral Maxillofac. Surg. 17 (4), 232–236. doi:10.1016/s0901-5027(88)80047-x
Chang, S.-H., Lin, Y.-Y., Wu, G.-J., Huang, C.-H., and Tsai, G. J. (2019). Effect of Chitosan Molecular Weight on Anti-inflammatory Activity in the RAW 264.7 Macrophage Model. Int. J. Biol. Macromol. 131, 167–175. doi:10.1016/j.ijbiomac.2019.02.066
Chen, Z., Ni, S., Han, S., Crawford, R., Lu, S., Wei, F., et al. (2017). Nanoporous Microstructures Mediate Osteogenesis by Modulating the Osteo-Immune Response of Macrophages. Nanoscale 9 (2), 706–718. doi:10.1039/c6nr06421c
Chiulan, I., Frone, A., Brandabur, C., and Panaitescu, D. (2017). Recent Advances in 3D Printing of Aliphatic Polyesters. Bioengineering 5 (1), 2. doi:10.3390/bioengineering5010002
Chu, C., Wang, Y., Wang, Y., Yang, R., Liu, L., Rung, S., et al. (2019). Evaluation of Epigallocatechin-3-Gallate (EGCG) Modified Collagen in Guided Bone Regeneration (GBR) Surgery and Modulation of Macrophage Phenotype. Mater. Sci. Eng. C 99, 73–82. doi:10.1016/j.msec.2019.01.083co;2-f
D'Elía, N. L., Rial Silva, R., Sartuqui, J., Ercoli, D., Ruso, J., Messina, P., et al. (2020). Development and Characterisation of Bilayered Periosteum-Inspired Composite Membranes Based on Sodium Alginate-Hydroxyapatite Nanoparticles. J. Colloid Interface Sci. 572, 408–420. doi:10.1016/j.jcis.2020.03.086
da Silva, T. N., Gonçalves, R. P., Rocha, C. L., Archanjo, B. S., Barboza, C. A. G., Pierre, M. B. R., et al. (2019). Controlling Burst Effect with PLA/PVA Coaxial Electrospun Scaffolds Loaded with BMP-2 for Bone Guided Regeneration. Mater. Sci. Eng. C 97, 602–612. doi:10.1016/j.msec.2018.12.020
Danesh-Sani, S. A., Loomer, P. M., and Wallace, S. S. (2016). A Comprehensive Clinical Review of Maxillary Sinus Floor Elevation: Anatomy, Techniques, Biomaterials and Complications. Br. J. Oral Maxillofac. Surg. 54 (7), 724–730. doi:10.1016/j.bjoms.2016.05.008
Dermience, M., Lognay, G., Mathieu, F., and Goyens, P. (2015). Effects of Thirty Elements on Bone Metabolism. J. Trace Elem. Med. Biol. 32, 86–106. doi:10.1016/j.jtemb.2015.06.005
Di Carlo, S., Ciolfi, A., Grasso, E., Pranno, N., De Angelis, F., Di Gioia, C., et al. (2021). A Retrospective Analysis of Treatment Outcomes Following Guided Bone Regeneration at Sites Exhibiting Severe Alveolar Ridge Atrophy. J. Craniofac Surg. 32 (6), e572–e578. doi:10.1097/SCS.0000000000007735
Di Martino, A., Sittinger, M., and Risbud, M. V. (2005). Chitosan: a Versatile Biopolymer for Orthopaedic Tissue-Engineering. Biomaterials 26 (30), 5983–5990. doi:10.1016/j.biomaterials.2005.03.016
Dubey, N., Ferreira, J. A., Daghrery, A., Aytac, Z., Malda, J., Bhaduri, S. B., et al. (2020). Highly Tunable Bioactive Fiber-Reinforced Hydrogel for Guided Bone Regeneration. Acta Biomater. 113, 164–176. doi:10.1016/j.actbio.2020.06.011
Dutta, S., Gupta, S., and Roy, M. (2020). Recent Developments in Magnesium Metal-Matrix Composites for Biomedical Applications: A Review. ACS Biomater. Sci. Eng. 6 (9), 4748–4773. doi:10.1021/acsbiomaterials.0c00678
Ebrahimi, L., Farzin, A., Ghasemi, Y., Alizadeh, A., Goodarzi, A., Basiri, A., et al. (2021). Metformin-Loaded PCL/PVA Fibrous Scaffold Preseeded with Human Endometrial Stem Cells for Effective Guided Bone Regeneration Membranes. ACS Biomater. Sci. Eng. 7 (1), 222–231. doi:10.1021/acsbiomaterials.0c00958
Ekladious, I., Colson, Y. L., and Grinstaff, M. W. (2019). Polymer-drug Conjugate Therapeutics: Advances, Insights and Prospects. Nat. Rev. Drug Discov. 18 (4), 273–294. doi:10.1038/s41573-018-0005-0
Elgali, I., Omar, O., Dahlin, C., and Thomsen, P. (2017). Guided Bone Regeneration: Materials and Biological Mechanisms Revisited. Eur. J. Oral Sci. 125 (5), 315–337. doi:10.1111/eos.12364
Farraro, K. F., Kim, K. E., Woo, S. L.-Y., Flowers, J. R., and McCullough, M. B. (2014). Revolutionizing Orthopaedic Biomaterials: The Potential of Biodegradable and Bioresorbable Magnesium-Based Materials for Functional Tissue Engineering. J. Biomechanics 47 (9), 1979–1986. doi:10.1016/j.jbiomech.2013.12.003
Federico, S., Pitarresi, G., Palumbo, F. S., Fiorica, C., Yang, F., and Giammona, G. (2021). Hyaluronan Alkyl Derivatives-Based Electrospun Membranes for Potential Guided Bone Regeneration: Fabrication, Characterization and In Vitro Osteoinductive Properties. Colloids Surfaces B Biointerfaces 197, 111438. doi:10.1016/j.colsurfb.2020.111438
Fenbo, M., Xingyu, X., and Bin, T. (2019). Strontium Chondroitin Sulfate/silk Fibroin Blend Membrane Containing Microporous Structure Modulates Macrophage Responses for Guided Bone Regeneration. Carbohydr. Polym. 213, 266–275. doi:10.1016/j.carbpol.2019.02.068
Fénelon, M., Chassande, O., Kalisky, J., Gindraux, F., Brun, S., Bareille, R., et al. (2018). Human Amniotic Membrane for Guided Bone Regeneration of Calvarial Defects in Mice. J. Mater Sci. Mater Med. 29 (6), 78. doi:10.1007/s10856-018-6086-9
Fenelon, M., Etchebarne, M., Siadous, R., Grémare, A., Durand, M., Sentilhes, L., et al. (2020). Assessment of Fresh and Preserved Amniotic Membrane for Guided Bone Regeneration in Mice. J. Biomed. Mater Res. 108 (10), 2044–2056. doi:10.1002/jbm.a.36964
Frederickson, C. J., Koh, J.-Y., and Bush, A. I. (2005). The Neurobiology of Zinc in Health and Disease. Nat. Rev. Neurosci. 6 (6), 449–462. doi:10.1038/nrn1671
Fu, J. H., and Wang, H. L. (2011). Horizontal Bone Augmentation: the Decision Tree. Int. J. Periodontics Restor. Dent. 31 (4), 429–436. doi:10.4012/dmj.2011-028
Fu, L., Wang, Z., Dong, S., Cai, Y., Ni, Y., Zhang, T., et al. (2017). Bilayer Poly(Lactic-Co-Glycolic acid)/Nano-Hydroxyapatite Membrane with Barrier Function and Osteogenesis Promotion for Guided Bone Regeneration. Materials 10 (3), 257. doi:10.3390/ma10030257
Fujioka-Kobayashi, M., Schaler, B., Shirakata, Y., Nakamura, T., Noguchi, K., Zhang, Y., et al. (2017). Comparison of Two Porcine Collagen Membranes Combined with rhBMP-2 and rhBMP-9 on Osteoblast Behavior In Vitro. Int. J. Oral Maxillofac. Implants 32 (4), e221–e230. doi:10.11607/jomi.5652
Ghanmi, S., Trigui, M., Baya, W., Ellouz, Z., Elfeki, A., Charfi, S., et al. (2018). The Periosteum-like Effect of Fresh Human Amniotic Membrane on Bone Regeneration in a Rabbit Critical-Sized Defect Model. Bone 110, 392–404. doi:10.1016/j.bone.2018.03.004
Ghavimi, M. A., Bani Shahabadi, A., Jarolmasjed, S., Memar, M. Y., Maleki Dizaj, S., and Sharifi, S. (2020). Nanofibrous Asymmetric Collagen/curcumin Membrane Containing Aspirin-Loaded PLGA Nanoparticles for Guided Bone Regeneration. Sci. Rep. 10 (1), 18200. doi:10.1038/s41598-020-75454-2
Goodier, M. C., Ronkainen, S. D., and Hylwa, S. A. (2018). Rubber Accelerators in Medical Examination and Surgical Gloves. Dermatitis 29 (2), 66–76. doi:10.1097/DER.0000000000000342
Grzywocz, Z., Pius-Sadowska, E., Klos, P., Gryzik, M., Wasilewska, D., Aleksandrowicz, B., et al. (2014). Growth Factors and Their Receptors Derived from Human Amniotic Cells In Vitro. Folia Histochem Cytobiol. 52 (3), 163–170. doi:10.5603/FHC.2014.0019
Gueldenpfennig, T., Houshmand, A., Najman, S., Stojanovic, S., Korzinskas, T., Smeets, R., et al. (2020). The Condensation of Collagen Leads to an Extended Standing Time and a Decreased Pro-inflammatory Tissue Response to a Newly Developed Pericardium-Based Barrier Membrane for Guided Bone Regeneration. Vivo 34 (3), 985–1000. doi:10.21873/invivo.11867
Guo, H., Xia, D., Zheng, Y., Zhu, Y., Liu, Y., and Zhou, Y. (2020). A Pure Zinc Membrane with Degradability and Osteogenesis Promotion for Guided Bone Regeneration: In Vitro and In Vivo Studies. Acta Biomater. 106, 396–409. doi:10.1016/j.actbio.2020.02.024
Ha, Y.-Y., Park, Y.-W., Kweon, H., Jo, Y.-Y., and Kim, S.-G. (2014). Comparison of the Physical Properties and In Vivo Bioactivities of Silkworm-Cocoon-Derived Silk Membrane, Collagen Membrane, and Polytetrafluoroethylene Membrane for Guided Bone Regeneration. Macromol. Res. 22 (9), 1018–1023. doi:10.1007/s13233-014-2138-2
He, Y., Jin, Y., Wang, X., Yao, S., Li, Y., Wu, Q., et al. (2018). An Antimicrobial Peptide-Loaded Gelatin/Chitosan Nanofibrous Membrane Fabricated by Sequential Layer-By-Layer Electrospinning and Electrospraying Techniques. Nanomaterials 8 (5), 327. doi:10.3390/nano8050327
He, Y., Wang, W., Tang, X., and Liu, X. (2017). Osteogenic Induction of Bone Marrow Mesenchymal Cells on Electrospun Polycaprolactone/chitosan Nanofibrous Membrane. Dent. Mat. J. 36 (3), 325–332. doi:10.4012/dmj.2016-203
Henry, J. J. D., Yu, J., Wang, A., Lee, R., Fang, J., and Li, S. (2017). Engineering the Mechanical and Biological Properties of Nanofibrous Vascular Grafts for In Situ Vascular Tissue Engineering. Biofabrication 9 (3), 035007. doi:10.1088/1758-5090/aa834b
Hoornaert, A., d’Arros, C., Heymann, M.-F., and Layrolle, P. (2016). Biocompatibility, Resorption and Biofunctionality of a New Synthetic Biodegradable Membrane for Guided Bone Regeneration. Biomed. Mat. 11 (4), 045012. doi:10.1088/1748-6041/11/4/045012
Hwang, K.-S., Choi, J.-W., Kim, J.-H., Chung, H., Jin, S., Shim, J.-H., et al. (2017). Comparative Efficacies of Collagen-Based 3D Printed PCL/PLGA/β-TCP Composite Block Bone Grafts and Biphasic Calcium Phosphate Bone Substitute for Bone Regeneration. Materials 10 (4), 421. doi:10.3390/ma10040421
Iviglia, G., Kargozar, S., and Baino, F. (2019). Biomaterials, Current Strategies, and Novel Nano-Technological Approaches for Periodontal Regeneration. Jfb 10 (1), 3. doi:10.3390/jfb10010003
Jamieson, S., Mawdesley, A., Deehan, D., Kirby, J., Holland, J., and Tyson-Capper, A. (2021). Inflammatory Responses to Metal Oxide Ceramic Nanopowders. Sci. Rep. 11 (1), 10531. doi:10.1038/s41598-021-89329-7
Jazayeri, H. E., Tahriri, M., Razavi, M., Khoshroo, K., Fahimipour, F., Dashtimoghadam, E., et al. (2017). A Current Overview of Materials and Strategies for Potential Use in Maxillofacial Tissue Regeneration. Mater. Sci. Eng. C 70 (Pt 1), 913–929. doi:10.1016/j.msec.2016.08.055
Jensen, L. K., Koch, J., Aalbaek, B., Moodley, A., Bjarnsholt, T., Kragh, K. N., et al. (2017). Early Implant-Associated Osteomyelitis Results in a Peri-Implanted Bacterial Reservoir. APMIS 125 (1), 38–45. doi:10.1111/apm.12597
Jin, W., Liang, X., Brooks, A., Futrega, K., Liu, X., Doran, M. R., et al. (2018). Modelling of the SDF-1/CXCR4 Regulated In Vivo Homing of Therapeutic Mesenchymal Stem/stromal Cells in Mice. PeerJ 6, e6072. doi:10.7717/peerj.6072
Jung, R. E., Hälg, G. A., Thoma, D. S., and Hämmerle, C. H. F. (2009a). A Randomized, Controlled Clinical Trial to Evaluate a New Membrane for Guided Bone Regeneration Around Dental Implants. Clin. Oral Implants Res. 20 (2), 162–168. doi:10.1111/j.1600-0501.2008.01634.x
Jung, R. E., Lecloux, G., Rompen, E., Ramel, C. F., Buser, D., and Hammerle, C. H. F. (2009b). A Feasibility Study Evaluating Anin Situformed Synthetic Biodegradable Membrane for Guided Bone Regeneration in Dogs. Clin. Oral Implants Res. 20 (2), 151–161. doi:10.1111/j.1600-0501.2008.01633.x
Jung, U.-W., Lee, I.-K., Park, J.-Y., Thoma, D. S., Hämmerle, C. H. F., and Jung, R. E. (2015). The Efficacy of BMP-2 Preloaded on Bone Substitute or Hydrogel for Bone Regeneration at Peri-Implant Defects in Dogs. Clin. Oral Impl. Res. 26 (12), 1456–1465. doi:10.1111/clr.12491
Karahaliloglu, Z., Ercan, B., Taylor, E. N., Chung, S., Denkbaş, E. B., and Webster, T. J. (2015). Antibacterial Nanostructured Polyhydroxybutyrate Membranes for Guided Bone Regeneration. J. Biomed. Nanotechnol. 11 (12), 2253–2263. doi:10.1166/jbn.2015.2106
Kim, J.-Y., Yang, B.-E., Ahn, J.-H., Park, S. O., and Shim, H.-W. (2014). Comparable Efficacy of Silk Fibroin with the Collagen Membranes for Guided Bone Regeneration in Rat Calvarial Defects. J. Adv. Prosthodont 6 (6), 539–546. doi:10.4047/jap.2014.6.6.539
Kim, J. Y., Yoon, J. J., Park, E. K., Kim, D. S., Kim, S.-Y., and Cho, D.-W. (2009a). Cell Adhesion and Proliferation Evaluation of SFF-Based Biodegradable Scaffolds Fabricated Using a Multi-Head Deposition System. Biofabrication 1 (1), 015002. doi:10.1088/1758-5082/1/1/015002
Kim, M.-J., Kim, C.-W., Lim, Y.-J., and Heo, S.-J. (2006). Microrough Titanium Surface Affects Biologic Response in MG63 Osteoblast-like Cells. J. Biomed. Mat. Res. 79A (4), 1023–1032. doi:10.1002/jbm.a.31040
Kim, O., Choi, J., Kim, B., and Seo, D. (2020). Long‐term Success Rates of Implants with Guided Bone Regeneration or Bone Grafting. Clin. Oral Impl Res. 31, 230. doi:10.1111/clr.171_13644
Kim, S.-H., Kim, D.-Y., Kim, K.-H., Ku, Y., Rhyu, I.-C., and Lee, Y.-M. (2009b). The Efficacy of a Double-Layer Collagen Membrane Technique for Overlaying Block Grafts in a Rabbit Calvarium Model. Clin. Oral Implants Res. 20 (10), 1124–1132. doi:10.1111/j.1600-0501.2009.01744.x
Koob, T. J., Lim, J. J., Zabek, N., and Massee, M. (2015). Cytokines in Single Layer Amnion Allografts Compared to Multilayer Amnion/chorion Allografts for Wound Healing. J. Biomed. Mat. Res. 103 (5), 1133–1140. doi:10.1002/jbm.b.33265
Korzinskas, T., Jung, O., Smeets, R., Stojanovic, S., Najman, S., Glenske, K., et al. (2018). In Vivo Analysis of the Biocompatibility and Macrophage Response of a Non-resorbable PTFE Membrane for Guided Bone Regeneration. Ijms 19 (10), 2952. doi:10.3390/ijms19102952
Lee, S.-W., and Kim, S.-G., (2014). Membranes for the Guided Bone Regeneration. Maxillofac. Plastic Reconstr. Surg. 36 (6), 239–246. doi:10.14402/jkamprs.2014.36.6.239
Lee, S.-Y., Wu, S.-C., Chen, H., Tsai, L.-L., Tzeng, J.-J., Lin, C.-H., et al. (2018). Synthesis and Characterization of Polycaprolactone-Based Polyurethanes for the Fabrication of Elastic Guided Bone Regeneration Membrane. BioMed Res. Int. 2018, 1–13. doi:10.1155/2018/3240571
Li, B. W., Wu, W. Y., Tang, L., Zhang, Y., and Liu, Y. H. (2019). Barrier Effect of Improved Porcine Small Intestinal Submucosa Absorbable Membrane on Early Healing of Mandibular Defects in Rabbits. Beijing Da Xue Xue Bao Yi Xue Ban. 51 (5), 887–892. [Article in Chinese]. doi:10.19723/j.issn.1671-167X.2019.05.016
Li, H., Song, P., Qiao, T., Cui, Q., Song, X., and Zhang, B. (2016). A Quaternary Composite Fiber Membrane for Guided Tissue Regeneration. Polym. Adv. Technol. 27 (2), 178–184. doi:10.1002/pat.3616
Lin, P.-H., Sermersheim, M., Li, H., Lee, P., Steinberg, S., and Ma, J. (2017). Zinc in Wound Healing Modulation. Nutrients 10 (1), 16. doi:10.3390/nu10010016
Liu, D., Xu, G., Jamali, S. S., Zhao, Y., Chen, M., and Jurak, T. (2019). Fabrication of Biodegradable HA/Mg-Zn-Ca Composites and the Impact of Heterogeneous Microstructure on Mechanical Properties, In Vitro Degradation and Cytocompatibility. Bioelectrochemistry 129, 106–115. doi:10.1016/j.bioelechem.2019.05.001
Liu, F., Chen, Q., Liu, C., Ao, Q., Tian, X., Fan, J., et al. (2018). Natural Polymers for Organ 3D Bioprinting. Polymers 10 (11), 1278. doi:10.3390/polym10111278
Liu, S., Dong, C., Lu, G., Lu, Q., Li, Z., Kaplan, D. L., et al. (2013). Bilayered Vascular Grafts Based on Silk Proteins. Acta Biomater. 9 (11), 8991–9003. doi:10.1016/j.actbio.2013.06.045
Liu, W., Dong, X., Qin, H., Sui, L., and Wang, J. (2021). Three-dimensional Porous Reduced Graphene Oxide/hydroxyapatite Membrane for Guided Bone Regeneration. Colloids Surfaces B Biointerfaces 208, 112102. doi:10.1016/j.colsurfb.2021.112102
Lotfi, G., Shokrgozar, M. A., Mofid, R., Abbas, F. M., Ghanavati, F., Baghban, A. A., et al. (2016). Biological Evaluation (In Vitro and In Vivo) of Bilayered Collagenous Coated (Nano Electrospun and Solid Wall) Chitosan Membrane for Periodontal Guided Bone Regeneration. Ann. Biomed. Eng. 44 (7), 2132–2144. doi:10.1007/s10439-015-1516-z
Luo, D., Yao, C., Zhang, R., Zhao, R., Iqbal, M. Z., Mushtaq, A., et al. (2021). Silk Fibroin/Collagen Blended Membrane Fabricated via a Green Papermaking Method for Potential Guided Bone Regeneration Application: In Vitro and In Vivo Evaluation. ACS Biomater. Sci. Eng. 7 (12), 5788–5797. doi:10.1021/acsbiomaterials.1c01060
Ma, S., Adayi, A., Liu, Z., Li, M., Wu, M., Xiao, L., et al. (2016). Asymmetric Collagen/chitosan Membrane Containing Minocycline-Loaded Chitosan Nanoparticles for Guided Bone Regeneration. Sci. Rep. 6, 18–22. doi:10.1038/srep31822
Ma, S., Chen, Z., Qiao, F., Sun, Y., Yang, X., Deng, X., et al. (2014). Guided Bone Regeneration with Tripolyphosphate Cross-Linked Asymmetric Chitosan Membrane. J. Dent. 42 (12), 1603–1612. doi:10.1016/j.jdent.2014.08.015
Mathew, A., Vaquette, C., Hashimi, S., Rathnayake, I., Huygens, F., Hutmacher, D. W., et al. (2017). Antimicrobial and Immunomodulatory Surface-Functionalized Electrospun Membranes for Bone Regeneration. Adv. Healthc. Mater 6. doi:10.1002/adhm.20160134510.1002/adhm.201601345
Meinel, L., Hofmann, S., Karageorgiou, V., Kirker-Head, C., McCool, J., Gronowicz, G., et al. (2005). The Inflammatory Responses to Silk Films In Vitro and In Vivo. Biomaterials 26 (2), 147–155. doi:10.1016/j.biomaterials.2004.02.047
Melke, J., Midha, S., Ghosh, S., Ito, K., and Hofmann, S. (2016). Silk Fibroin as Biomaterial for Bone Tissue Engineering. Acta Biomater. 31, 1–16. doi:10.1016/j.actbio.2015.09.005
Meng, Y., Liu, L., Zhang, D., Dong, C., Yan, Y., Volinsky, A. A., et al. (2019). Initial Formation of Corrosion Products on Pure Zinc in Saline Solution. Bioact. Mater. 4 (1), 87–96. doi:10.1016/j.bioactmat.2018.08.003
Midha, S., Tripathi, R., Geng, H., Lee, P. D., and Ghosh, S. (2016). Elucidation of Differential Mineralisation on Native and Regenerated Silk Matrices. Mater. Sci. Eng. C 68, 663–674. doi:10.1016/j.msec.2016.06.041
Miron, R., Fujioka-Kobayashi, M., Buser, D., Zhang, Y., Bosshardt, D., and Sculean, A. (2017). Combination of Collagen Barrier Membrane with Enamel Matrix Derivative-Liquid Improves Osteoblast Adhesion and Differentiation. Int. J. Oral Maxillofac. Implants 32 (1), 196–203. doi:10.11607/jomi.5011
Mohan, N., Wolf, J., and Dym, H. (2015). Maxillary Sinus Augmentation. Dent. Clin. N. Am. 59 (2), 375–388. doi:10.1016/j.cden.2014.10.001
Momen-Heravi, F., Peters, S. M., Garfinkle, L., and Kang, P. (2018). Acellular Dermal Matrix as a Barrier for Guided Bone Regeneration of Dehiscence Defects Around Dental Implants: A Clinical and Histological Report. Implant Dent. 27 (4), 521–524. doi:10.1097/ID.0000000000000796
Morris, G. A., Kök, S. M., Harding, S. E., and Adams, G. G. (2010). Polysaccharide Drug Delivery Systems Based on Pectin and Chitosan. Biotechnol. Genet. Eng. Rev. 27, 257–284. doi:10.1080/02648725.2010.10648153
Moses, O., Vitrial, D., Aboodi, G., Sculean, A., Tal, H., Kozlovsky, A., et al. (2008). Biodegradation of Three Different Collagen Membranes in the Rat Calvarium: a Comparative Study. J. Periodontology 79 (5), 905–911. doi:10.1902/jop.2008.070361
Murphy, W. L., Kohn, D. H., and Mooney, D. J. (2000). Growth of Continuous Bonelike Mineral within Porous Poly(lactide-Co-Glycolide) Scaffoldsin Vitro. J. Biomed. Mat. Res. 50 (1), 50–58. doi:10.1002/(sici)1097-463610.1002/(sici)1097-4636(200004)50:1<50::aid-jbm8>3.0.co;2-f
Nowwarote, N., Chanjavanakul, P., Kongdecha, P., Clayhan, P., Chumprasert, S., Manokawinchoke, J., et al. (2018). Characterization of a Bioactive Jagged1-Coated Polycaprolactone-Based Membrane for Guided Tissue Regeneration. Archives Oral Biol. 88, 24–33. doi:10.1016/j.archoralbio.2018.01.007
Oktay, E., Demiralp, B., Demiralp, B., Senel, S., Cevdet Akman, A., Eratalay, K., et al. (2010). Effects of Platelet-Rich Plasma and Chitosan Combination on Bone Regeneration in Experimental Rabbit Cranial Defects. J. Oral Implantol. 36 (3), 175–184. doi:10.1563/AAID-JOI-D-09-00023
Omar, O., Elgali, I., Dahlin, C., and Thomsen, P. (2019). Barrier Membranes: More Than the Barrier Effect? J. Clin. Periodontol. 46 (Suppl. 21Suppl Suppl 21), 103–123. doi:10.1111/jcpe.13068
Ortolani, E., Quadrini, F., Bellisario, D., Santo, L., Polimeni, A., and Santarsiero, A. (2015). Mechanical Qualification of Collagen Membranes Used in Dentistry. Ann. Ist. Super. Sanita 51 (3), 229–235. doi:10.4415/ANN_15_03_11
Oryan, A., Kamali, A., Moshiri, A., Baharvand, H., and Daemi, H. (2018). Chemical Crosslinking of Biopolymeric Scaffolds: Current Knowledge and Future Directions of Crosslinked Engineered Bone Scaffolds. Int. J. Biol. Macromol. 107 (Pt A), 678–688. doi:10.1016/j.ijbiomac.2017.08.184
Ovcharenko, N., Greenwell, H., Katwal, D., Patel, A., Hill, M., Shumway, B., et al. (2020). A Comparison of the Effect of Barrier Membranes on Clinical and Histologic Hard and Soft Tissue Healing with Ridge Preservation. Int. J. Periodontics Restor. Dent. 40 (3), 365–371. doi:10.11607/prd.4120
Park, J. K., Yeom, J., Oh, E. J., Reddy, M., Kim, J. Y., Cho, D.-W., et al. (2009). Guided Bone Regeneration by Poly(lactic-Co-Glycolic Acid) Grafted Hyaluronic Acid Bi-layer Films for Periodontal Barrier Applications. Acta Biomater. 5 (9), 3394–3403. doi:10.1016/j.actbio.2009.05.019
Park, S., Lee, H.-J., Kim, K.-S., Lee, S., Lee, J.-T., Kim, S.-Y., et al. (2018). In Vivo Evaluation of 3D-Printed Polycaprolactone Scaffold Implantation Combined with β-TCP Powder for Alveolar Bone Augmentation in a Beagle Defect Model. Materials 11 (2), 238. doi:10.3390/ma11020238
Qi, Y., Wang, H., Wei, K., Yang, Y., Zheng, R.-Y., Kim, I., et al. (2017). A Review of Structure Construction of Silk Fibroin Biomaterials from Single Structures to Multi-Level Structures. Ijms 18 (3), 237. doi:10.3390/ijms18030237
Rachmiel, A., Emodi, O., Aizenbud, D., Rachmiel, D., and Shilo, D. (2018). Two-stage Reconstruction of the Severely Deficient Alveolar Ridge: Bone Graft Followed by Alveolar Distraction Osteogenesis. Int. J. Oral Maxillofac. Surg. 47 (1), 117–124. doi:10.1016/j.ijom.2017.07.007
Rahman, M., Dutta, N. K., and Roy Choudhury, N. (2020). Magnesium Alloys with Tunable Interfaces as Bone Implant Materials. Front. Bioeng. Biotechnol. 8, 564. doi:10.3389/fbioe.2020.00564
Rakhmatia, Y. D., Ayukawa, Y., Furuhashi, A., and Koyano, K. (2013). Current Barrier Membranes: Titanium Mesh and Other Membranes for Guided Bone Regeneration in Dental Applications. J. Prosthodont. Res. 57 (1), 3–14. doi:10.1016/j.jpor.2012.12.001
Rameshbabu, A. P., Ghosh, P., Subramani, E., Bankoti, K., Kapat, K., Datta, S., et al. (2016). Investigating the Potential of Human Placenta-Derived Extracellular Matrix Sponges Coupled with Amniotic Membrane-Derived Stem Cells for Osteochondral Tissue Engineering. J. Mat. Chem. B 4 (44), 613–625. doi:10.1039/c5tb02321a
Rodriguez-Contreras, A. (2019). Recent Advances in the Use of Polyhydroyalkanoates in Biomedicine. Bioengineering 6 (3), 82. doi:10.3390/bioengineering6030082
Rothamel, D., Schwarz, F., Fienitz, T., Smeets, R., Dreiseidler, T., Ritter, L., et al. (2012). Biocompatibility and Biodegradation of a Native Porcine Pericardium Membrane: Results of In Vitro and In Vivo Examinations. Int. J. Oral Maxillofac. Implants 27 (1), 146–154. doi:10.1016/j.ijom.2011.07.902
Saarani, N. N., Jamuna-Thevi, K., Shahab, N., Hermawan, H., and Saidin, S. (2017). Antibacterial Efficacy of Triple-Layered Poly(lactic-Co-Glycolic Acid)/nanoapatite/lauric Acid Guided Bone Regeneration Membrane on Periodontal Bacteria. Dent. Mat. J. 36 (3), 260–265. doi:10.4012/dmj.2016-177
Saberi, A., Bakhsheshi-Rad, H. R., Abazari, S., Ismail, A. F., Sharif, S., Ramakrishna, S., et al. (2021). A Comprehensive Review on Surface Modifications of Biodegradable Magnesium-Based Implant Alloy: Polymer Coatings Opportunities and Challenges. Coatings 11 (7), 747. doi:10.3390/coatings11070747
Sakkas, A., Wilde, F., Heufelder, M., Winter, K., and Schramm, A. (2017). Autogenous Bone Grafts in Oral Implantology-Is it Still a "gold Standard"? A Consecutive Review of 279 Patients with 456 Clinical Procedures. Int. J. Implant Dent. 3 (1), 23. doi:10.1186/s40729-017-0084-4
Saleem, M., Rasheed, S., and Yougen, C. (2020). Silk Fibroin/hydroxyapatite Scaffold: a Highly Compatible Material for Bone Regeneration. Sci. Technol. Adv. Mater. 21 (1), 242–266. doi:10.1080/14686996.2020.1748520
Sautrot-Ba, P., Razza, N., Breloy, L., Andaloussi, S. A., Chiappone, A., Sangermano, M., et al. (2019). Photoinduced Chitosan-PEG Hydrogels with Long-Term Antibacterial Properties. J. Mat. Chem. B 7 (42), 6526–6538. doi:10.1039/c9tb01170f
Sbricoli, L., Guazzo, R., Annunziata, M., Gobbato, L., Bressan, E., and Nastri, L. (2020). Selection of Collagen Membranes for Bone Regeneration: A Literature Review. Materials 13 (3), 786. doi:10.3390/ma13030786
Schorn, L., Sproll, C., Ommerborn, M., Naujoks, C., Kübler, N. R., and Depprich, R. (2017). Vertical Bone Regeneration Using rhBMP-2 and VEGF. Head. Face Med. 13 (1), 11. doi:10.1186/s13005-017-0146-0
Seo, H.-J., Cho, Y.-E., Kim, T., Shin, H.-I., and Kwun, I.-S. (2010). Zinc May Increase Bone Formation through Stimulating Cell Proliferation, Alkaline Phosphatase Activity and Collagen Synthesis in Osteoblastic MC3T3-E1 Cells. Nutr. Res. Pract. 4 (5), 356–361. doi:10.4162/nrp.2010.4.5.356
Sheikh, Z., Hamdan, N., Ikeda, Y., Grynpas, M., Ganss, B., and Glogauer, M. (2017a). Natural Graft Tissues and Synthetic Biomaterials for Periodontal and Alveolar Bone Reconstructive Applications: a Review. Biomater. Res. 21, 9–20. doi:10.1186/s40824-017-0095-5
Sheikh, Z., Khan, A. S., Roohpour, N., Glogauer, M., and Rehman, I. u. (2016). Protein Adsorption Capability on Polyurethane and Modified-Polyurethane Membrane for Periodontal Guided Tissue Regeneration Applications. Mater. Sci. Eng. C 68, 267–275. doi:10.1016/j.msec.2016.05.026
Sheikh, Z., Qureshi, J., Alshahrani, A. M., Nassar, H., Ikeda, Y., Glogauer, M., et al. (2017b). Collagen Based Barrier Membranes for Periodontal Guided Bone Regeneration Applications. Odontology 105 (1), 1–12. doi:10.1007/s10266-016-0267-0
Shi, C. N., Liu, J., Wang, F., and Hong-Yu, X. U. (2017). Preparation of OPG-PLA/CS Nanofibermembranes by Coaxial Electrospinning and its Characterization. Chin. J. Conservative Dent. 27, 141–144. [Article in Chinese]. doi:10.15956/j.cnki.chin.j.conserv.dent.2017.03.004
Shiran, G., Fan, Z., Mengting, L., Ting, H., and Lige, Z. (2016). Preparation of Hydroxyapatite/chitosan-Transforming Growth Factor-β Composite Coatings on Titanium Surfaces and its Effect on the Attachment and Proliferation of Osteoblasts. Hua Xi Kou Qiang Yi Xue Za Zhi 34 (3), 229–233. [Article in Chinese]. doi:10.7518/hxkq.2016.03.003
Shuai, C., Yang, W., Feng, P., Peng, S., and Pan, H. (2021). Accelerated Degradation of HAP/PLLA Bone Scaffold by PGA Blending Facilitates Bioactivity and Osteoconductivity. Bioact. Mater. 6 (2), 490–502. doi:10.1016/j.bioactmat.2020.09.001
Si, J., Shen, H., Miao, H., Tian, Y., Huang, H., Shi, J., et al. (2021). In Vitro and In Vivo Evaluations of Mg-Zn-Gd Alloy Membrane on Guided Bone Regeneration for Rabbit Calvarial Defect. J. Magnesium Alloys 9 (1), 281–291. doi:10.1016/j.jma.2020.09.013
Siddiqui, J. A., Johnson, J., Le Henaff, C., Bitel, C. L., Tamasi, J. A., and Partridge, N. C. (2017). Catabolic Effects of Human PTH (1-34) on Bone: Requirement of Monocyte Chemoattractant Protein-1 in Murine Model of Hyperparathyroidism. Sci. Rep. 7 (1), 15300. doi:10.1038/s41598-017-15563-7
Soesilawati, P., Rizqiawan, A., Roestamadji, R. I., Arrosyad, A. R., Firdauzy, M. A. B., and Abu Kasim, N. H. (2021). In Vitro Cell Proliferation Assay of Demineralized Dentin Material Membrane in Osteoblastic MC3T3-E1 Cells. Ccide 13, 443–449. doi:10.2147/CCIDE.S313184
Song, J. M., Shin, S. H., Kim, Y. D., Lee, J. Y., Baek, Y. J., Yoon, S. Y., et al. (2014). Comparative Study of Chitosan/fibroin-Hydroxyapatite and Collagen Membranes for Guided Bone Regeneration in Rat Calvarial Defects: Micro-computed Tomography Analysis. Int. J. Oral Sci. 6 (2), 87–93. doi:10.1038/ijos.2014.16
Steigmann, L., Jung, O., Kieferle, W., Stojanovic, S., Proehl, A., Görke, O., et al. (2020). Biocompatibility and Immune Response of a Newly Developed Volume-Stable Magnesium-Based Barrier Membrane in Combination with a PVD Coating for Guided Bone Regeneration (GBR). Biomedicines 8 (12), 636. doi:10.3390/biomedicines8120636
Storrie, H., and Stupp, S. (2005). Cellular Response to Zinc-Containing Organoapatite: an In Vitro Study of Proliferation, Alkaline Phosphatase Activity and Biomineralization. Biomaterials 26 (27), 5492–5499. doi:10.1016/j.biomaterials.2005.01.043
Sudha, P. N., and Rose, M. H. (2014). Beneficial Effects of Hyaluronic Acid. Adv. Food Nutr. Res. 72, 137–176. doi:10.1016/B978-0-12-800269-8.00009-9
Sun, T., Liu, M., Yao, S., Ji, Y., Shi, L., Tang, K., et al. (2018a). Guided Osteoporotic Bone Regeneration with Composite Scaffolds of Mineralized ECM/heparin Membrane Loaded with BMP2-Related Peptide. Ijn 13, 791–804. doi:10.2147/IJN.S152698
Sun, X., Xu, C., Wu, G., Ye, Q., and Wang, C. (2017). Poly(Lactic-co-Glycolic Acid): Applications and Future Prospects for Periodontal Tissue Regeneration. Polymers 9 (6), 189. doi:10.3390/polym9060189
Sun, Y.-K., Cha, J.-K., Thoma, D. S., Yoon, S.-R., Lee, J.-S., Choi, S.-H., et al. (2018b). Bone Regeneration of Peri-Implant Defects Using a Collagen Membrane as a Carrier for Recombinant Human Bone Morphogenetic Protein-2. BioMed Res. Int. 2018, 1–9. doi:10.1155/2018/5437361
Swim, M. M., Albertario, A., Iacobazzi, D., Caputo, M., and Ghorbel, M. T. (2019). Amnion-Based Scaffold with Enhanced Strength and Biocompatibility for In Vivo Vascular Repair. Tissue Eng. Part A 25 (7-8), 603–619. doi:10.1089/ten.TEA.2018.0175
Taguchi, Y., Amizuka, N., Nakadate, M., Ohnishi, H., Fujii, N., Oda, K., et al. (2005). A Histological Evaluation for Guided Bone Regeneration Induced by a Collagenous Membrane. Biomaterials 26 (31), 6158–6166. doi:10.1016/j.biomaterials.2005.03.023
Tai, H.-Y., Fu, E., Cheng, L.-P., and Don, T.-M. (2014). Fabrication of Asymmetric Membranes from Polyhydroxybutyrate and Biphasic Calcium Phosphate/chitosan for Guided Bone Regeneration. J. Polym. Res. 21 (5), 1–11. doi:10.1007/s10965-014-0421-8
Tan, J., Zhang, M., Hai, Z., Wu, C., Lin, J., Kuang, W., et al. (2019). Sustained Release of Two Bioactive Factors from Supramolecular Hydrogel Promotes Periodontal Bone Regeneration. ACS Nano 13 (5), 5616–5622. doi:10.1021/acsnano.9b00788
Tan, Y., Zhang, L., Rajoka, M. S. R., Mai, Z., Bahadur, A., Mehwish, H. M., et al. (2021). Jawbones Scaffold Constructed by TGF-Β1 and BMP-2 Loaded Chitosan Microsphere Combining with Alg/HA/ICol for Osteogenic-Induced Differentiation. Polymers 13 (18), 3079. doi:10.3390/polym13183079
Tavelli, L., McGuire, M. K., Zucchelli, G., Rasperini, G., Feinberg, S. E., Wang, H. L., et al. (2020). Extracellular Matrix‐based Scaffolding Technologies for Periodontal and Peri‐implant Soft Tissue Regeneration. J. Periodontol. 91 (1), 17–25. doi:10.1002/JPER.19-0351
Thein-Han, W. W., Kitiyanant, Y., and Misra, R. D. K. (2008). Chitosan as Scaffold Matrix for Tissue Engineering. Mater. Sci. Technol. 24, 1062–1075. doi:10.1179/174328408X341753
Thoma, D. S., Payer, M., Jakse, N., Bienz, S. P., Hüsler, J., Schmidlin, P. R., et al. (2018). Randomized, Controlled Clinical Two-Centre Study Using Xenogeneic Block Grafts Loaded with Recombinant Human Bone Morphogenetic Protein-2 or Autogenous Bone Blocks for Lateral Ridge Augmentation. J. Clin. Periodontol. 45 (2), 265–276. doi:10.1111/jcpe.12841
Tolstunov, L., Hamrick, J. F. E., Broumand, V., Shilo, D., and Rachmiel, A. (2019). Bone Augmentation Techniques for Horizontal and Vertical Alveolar Ridge Deficiency in Oral Implantology. Oral Maxillofac. Surg. Clin. N. Am. 31 (2), 163–191. doi:10.1016/j.coms.2019.01.005
Toosi, S., Naderi-Meshkin, H., Kalalinia, F., HosseinKhani, H., Heirani-Tabasi, A., Havakhah, S., et al. (2019). Bone Defect Healing Is Induced by Collagen Sponge/polyglycolic Acid. J. Mater Sci. Mater Med. 30 (3), 33. doi:10.1007/s10856-019-6235-9
Trenfield, S. J., Awad, A., Madla, C. M., Hatton, G. B., Firth, J., Goyanes, A., et al. (2019). Shaping the Future: Recent Advances of 3D Printing in Drug Delivery and Healthcare. Expert Opin. Drug Deliv. 16 (10), 1081–1094. doi:10.1080/17425247.2019.1660318
Tsai, S.-W., Yu, W.-X., Hwang, P.-A., Hsu, Y.-W., and Hsu, F.-Y. (2019). Fabrication and Characteristics of PCL Membranes Containing Strontium-Substituted Hydroxyapatite Nanofibers for Guided Bone Regeneration. Polymers 11 (11), 1761. doi:10.3390/polym11111761
Unagolla, J. M., and Jayasuriya, A. C. (2019). Enhanced Cell Functions on Graphene Oxide Incorporated 3D Printed Polycaprolactone Scaffolds. Mater. Sci. Eng. C 102, 1–11. doi:10.1016/j.msec.2019.04.026
Valappil, S. P., Peiris, D., Langley, G. J., Herniman, J. M., Boccaccini, A. R., Bucke, C., et al. (2007). Polyhydroxyalkanoate (PHA) Biosynthesis from Structurally Unrelated Carbon Sources by a Newly Characterized Bacillus Spp. J. Biotechnol. 127 (3), 475–487. doi:10.1016/j.jbiotec.2006.07.015
Voinova, V., Bonartseva, G., and Bonartsev, A. (2019). Effect of Poly(3-Hydroxyalkanoates) as Natural Polymers on Mesenchymal Stem Cells. Wjsc 11 (10), 764–786. doi:10.4252/wjsc.v11.i10.764
Wang, H.-L., and Boyapati, L. (2006). "PASS" Principles for Predictable Bone Regeneration. Implant Dent. 15 (1), 8–17. doi:10.1097/01.id.0000204762.39826.0f
Wang, Y., Jiang, Y., Zhang, Y., Wen, S., Wang, Y., and Zhang, H. (2019a). Dual Functional Electrospun Core-Shell Nanofibers for Anti-infective Guided Bone Regeneration Membranes. Mater. Sci. Eng. C 98, 134–139. doi:10.1016/j.msec.2018.12.115
Wang, Z., Liang, R., Jiang, X., Xie, J., Cai, P., Chen, H., et al. (2019b). Electrospun PLGA/PCL/OCP Nanofiber Membranes Promote Osteogenic Differentiation of Mesenchymal Stem Cells (MSCs). Mater. Sci. Eng. C 104, 109796. doi:10.1016/j.msec.2019.109796
Wechsler, S., Fehr, D., Molenberg, A., Raeber, G., Schense, J. C., and Weber, F. E. (2008). A Novel, Tissue Occlusive Poly(ethylene Glycol) Hydrogel Material. J. Biomed. Mat. Res. 85A (2), 285–292. doi:10.1002/jbm.a.31477
Wei, Y., Chang, Y.-H., Liu, C.-J., and Chung, R.-J. (2018). Integrated Oxidized-Hyaluronic Acid/Collagen Hydrogel with β-TCP Using Proanthocyanidins as a Crosslinker for Drug Delivery. Pharmaceutics 10 (2), 37. doi:10.3390/pharmaceutics10020037
Wessing, B., Lettner, S., and Zechner, W. (2018). Guided Bone Regeneration with Collagen Membranes and Particulate Graft Materials: A Systematic Review and Meta-Analysis. Int. J. Oral Maxillofac. Implants 33 (1), 87–100. doi:10.11607/jomi.5461
Williams, D. F. (2008). On the Mechanisms of Biocompatibility. Biomaterials 29 (20), 2941–2953. doi:10.1016/j.biomaterials.2008.04.023
Willis, J., Li, S., Crean, S. J., and Barrak, F. N. (2021). Is Titanium Alloy Ti‐6Al‐4 V Cytotoxic to Gingival Fibroblasts-A Systematic Review. Clin. Exp. Dent. Res. 7 (6), 1037–1044. doi:10.1002/cre2.444
Won, J.-Y., Park, C.-Y., Bae, J.-H., Ahn, G., Kim, C., Lim, D.-H., et al. (2016). Evaluation of 3D Printed PCL/PLGA/β -TCP versus Collagen Membranes for Guided Bone Regeneration in a Beagle Implant Model. Biomed. Mat. 11 (5), 055013. doi:10.1088/1748-6041/11/5/055013
Wu, T.-C., Joshi, S. S., Ho, Y.-H., Pantawane, M. V., Sinha, S., and Dahotre, N. B. (2021). Microstructure and Surface Texture Driven Improvement in In-Vitro Response of Laser Surface Processed AZ31B Magnesium Alloy. J. Magnesium Alloys 9, 1406–1418. doi:10.1016/j.jma.2020.11.002
Wu, W., Li, B., Liu, Y., Wang, X., and Tang, L. (20182018). Effect of Multilaminate Small Intestinal Submucosa as a Barrier Membrane on Bone Formation in a Rabbit Mandible Defect Model. BioMed Res. Int. 2018, 1-11. doi:10.1155/2018/3270293
Xie, X., Shi, X., Wang, S., Cao, L., Yang, C., and Ma, Z. (2020a). Effect of Attapulgite-Doped Electrospun Fibrous PLGA Scaffold on Pro-osteogenesis and Barrier Function in the Application of Guided Bone Regeneration. Ijn 15, 6761–6777. doi:10.2147/IJN.S244533
Xie, Y., Li, S., Zhang, T., Wang, C., and Cai, X. (2020b). Titanium Mesh for Bone Augmentation in Oral Implantology: Current Application and Progress. Int. J. Oral Sci. 12 (1), 37. doi:10.1038/s41368-020-00107-z
Xiong, Y.-Z., Gao, R.-N., Zhang, H., Dong, L.-L., Li, J.-T., and Li, X. (2020). Rationally Designed Functionally Graded Porous Ti6Al4V Scaffolds with High Strength and Toughness Built via Selective Laser Melting for Load-Bearing Orthopedic Applications. J. Mech. Behav. Biomed. Mater. 104, 103673. doi:10.1016/j.jmbbm.2020.103673
Xu, C., Lei, C., Meng, L., Wang, C., and Song, Y. (2012). Chitosan as a Barrier Membrane Material in Periodontal Tissue Regeneration. J. Biomed. Mat. Res. 100B (5), 1435–1443. doi:10.1002/jbm.b.32662
Yang, X., Zhang, J., Ji, Q., Wang, F., Song, M., and Li, Y. (2018). Autophagy Protects MC3T3-E1 Cells upon Aluminum-Induced Apoptosis. Biol. Trace Elem. Res. 185 (2), 433–439. doi:10.1007/s12011-018-1264-7
Ye, H., Zhu, J., Deng, D., Jin, S., Li, J., and Man, Y. (2019). Enhanced Osteogenesis and Angiogenesis by PCL/chitosan/Sr-doped Calcium Phosphate Electrospun Nanocomposite Membrane for Guided Bone Regeneration. J. Biomaterials Sci. Polym. Ed. 30 (16), 1505–1522. doi:10.1080/09205063.2019.1646628
Ye, J., Yao, Q., Anchun, M., Nie, J., Cui Ye, W., Liu, W., et al. (2011). Effects of an Antibacterial Membrane on Osteoblast-like Cells In Vitro. Ijn 6, 1853–1861. doi:10.2147/IJN.S17749
Yilmaz, C., Ersanli, S., Karabagli, M., Olgac, V., and Bolukbasi Balcioglu, N. (2021). May Autogenous Grafts Increase the Effectiveness of Hyalonect Membranes in Intraosseous Defects: An Experimental In Vivo Study. Medicina 57 (5), 430. doi:10.3390/medicina57050430
Yin, L., Wang, K., Lv, X., Sun, R., Yang, S., Yang, Y., et al. (2017a). The Fabrication of an ICA-SF/PLCL Nanofibrous Membrane by Coaxial Electrospinning and its Effect on Bone Regeneration In Vitro and In Vivo. Sci. Rep. 7 (1), 8616. doi:10.1038/s41598-017-07759-8
Yin, L., Yang, S., He, M., Chang, Y., Wang, K., Zhu, Y., et al. (2017b). Physicochemical and Biological Characteristics of BMP-2/igf-1-Loaded Three-Dimensional Coaxial Electrospun Fibrous Membranes for Bone Defect Repair. J. Mater Sci. Mater Med. 28 (6), 94. doi:10.1007/s10856-017-5898-3
Yin, Y., Pu, D., and Xiong, J. (2017c). Analysis of the Comprehensive Tensile Relationship in Electrospun Silk Fibroin/Polycaprolactone Nanofiber Membranes. Membranes 7 (4), 67. doi:10.3390/membranes7040067
Yoo, C.-K., Jeon, J.-Y., Kim, Y.-J., Kim, S.-G., and Hwang, K.-G. (2016). Cell Attachment and Proliferation of Osteoblast-like MG63 Cells on Silk Fibroin Membrane for Guided Bone Regeneration. Maxillofac. Plast. Reconstr. Surg. 38 (1), 17. doi:10.1186/s40902-016-0062-4
Yurttutan, M. E., and Keskin, A. (2018). Evaluation of the Effects of Different Sand Particles that Used in Dental Implant Roughened for Osseointegration. BMC Oral Health 18 (1), 47. doi:10.1186/s12903-018-0509-3
Zhang, C., Zhang, T., Geng, T., Wang, X., Lin, K., and Wang, P. (2021). Dental Implants Loaded with Bioactive Agents Promote Osseointegration in Osteoporosis: A Review. Front. Bioeng. Biotechnol. 9, 591796. doi:10.3389/fbioe.2021.591796
Zhang, D., Tong, A., Zhou, L., Fang, F., and Guo, G. (2012). Osteogenic Differentiation of Human Placenta-Derived Mesenchymal Stem Cells (PMSCs) on Electrospun Nanofiber Meshes. Cytotechnology 64 (6), 701–710. doi:10.1007/s10616-012-9450-5
Qian, Y., Zhang, F., Chen, H., Xu, Y., Yang, J., Zhou, X., et al. (2016). The Preosteoblast Response of Electrospinning PLGA/PCL Nanofibers: Effects of Biomimetic Architecture and Collagen I. Ijn 11, 4157–4171. doi:10.2147/IJN.S110577
Zhang, J., Ma, S., Liu, Z., Geng, H., Lu, X., Zhang, X., et al. (2017). Guided Bone Regeneration with Asymmetric Collagen-Chitosan Membranes Containing Aspirin-Loaded Chitosan Nanoparticles. Ijn 12, 8855–8866. doi:10.2147/IJN.S148179
Zhang, Y. S., Zhang, K., Chen, X. W., Mu, H. Z., Ding, W. W., Qin, M. L., et al. (2020). Mechanical Properties of 3D-Printed Titanium Mesh and its Biocompatibility In Vitro. Shanghai Kou Qiang Yi Xue 29 (3), 250–256. [Article in Chinese]. doi:10.19439/j.sjos.2020.03.005
Zhao, L., He, C., Gao, Y., Cen, L., Cui, L., and Cao, Y. (2008). Preparation and Cytocompatibility of PLGA Scaffolds with Controllable Fiber Morphology and Diameter Using Electrospinning Method. J. Biomed. Mat. Res. 87B (1), 26–34. doi:10.1002/jbm.b.31060
Zhao, M., Sun, Y., Zhang, J., and Zhang, Y. (2018). Novel Translucent and Strong Submicron Alumina Ceramics for Dental Restorations. J. Dent. Res. 97 (3), 289–295. doi:10.1177/0022034517733742
Zhou, L., Su, Y., Wang, J., Wang, J., Wang, X., and Liu, Q. (2021a). Effect of Exposure Rates with Customized versus Conventional Titanium Mesh on Guided Bone Regeneration: A Systematic Review and Meta-Analysis. J. Oral Implantol. doi:10.1563/aaid-joi-D-20-00200
Keywords: guided bone regeneration, barrier membrane, polymer, functional membrane, absorbable material
Citation: Yang Z, Wu C, Shi H, Luo X, Sun H, Wang Q and Zhang D (2022) Advances in Barrier Membranes for Guided Bone Regeneration Techniques. Front. Bioeng. Biotechnol. 10:921576. doi: 10.3389/fbioe.2022.921576
Received: 16 April 2022; Accepted: 30 May 2022;
Published: 22 June 2022.
Edited by:
Jianshe Hu, Northeastern University, ChinaReviewed by:
Babak Akbari, University of Tehran, IranArun Prabhu Rameshbabu, Harvard Medical School, United States
Copyright © 2022 Yang, Wu, Shi, Luo, Sun, Wang and Zhang. This is an open-access article distributed under the terms of the Creative Commons Attribution License (CC BY). The use, distribution or reproduction in other forums is permitted, provided the original author(s) and the copyright owner(s) are credited and that the original publication in this journal is cited, in accordance with accepted academic practice. No use, distribution or reproduction is permitted which does not comply with these terms.
*Correspondence: Qiang Wang, bWZxd2FuZ0BjbXUuZWR1LmNu; Dan Zhang, emRjbXU4ODhAb3V0bG9vay5jb20=
†These authors have contributed equally to this work and share first authorship