- 1Science Center for Future Foods, Jiangnan University, Wuxi, China
- 2Key Laboratory of Industrial Biotechnology, Ministry of Education and School of Biotechnology, Jiangnan University, Wuxi, China
- 3Engineering Research Center of Ministry of Education on Food Synthetic Biotechnology, Jiangnan University, Wuxi, China
- 4Jiangsu Province Engineering Research Center of Food Synthetic Biotechnology, Jiangnan University, Wuxi, China
D-Glucose directly generates 2-keto-L-gulonic acid (2-KLG, precursor of vitamin C) through the 2,5-diketo-D-gluconic acid (2,5-DKG) pathway. 2,5-DKG is the main rate-limiting factor of the reaction, and there are few relevant studies on it. In this study, a more accurate quantitative method of 2,5-DKG was developed and used to screen G. oxydans ATCC9937 as the chassis strain for the production of 2,5-DKG. Combining the metabolite profile analysis and knockout and overexpression of production strain, the non-enzymatic browning of 2,5-DKG was identified as the main factor leading to low yield of the target compound. By optimizing the fermentation process, the fermentation time was reduced to 48 h, and 2,5-DKG production peaked at 50.9 g/L, which was 139.02% higher than in the control group. Effectively eliminating browning and reducing the degradation of 2,5-DKG will help increase the conversion of 2,5-DKG to 2-KLG, and finally, establish a one-step D-glucose to 2-KLG fermentation pathway.
Introduction
L-Ascorbic acid has important roles in food (Cendrowski et al., 2021), medicine (Pardo-Planas et al., 2017), and health (Anitra and Silvia, 2017; Reddy et al., 2020). Recent research has also suggested it has particular functions in electron transfer (Xin et al., 2022) and microbial fuel cells (Islam et al., 2020). Currently, in industry, L-ascorbic acid is mainly synthesized by 2-keto-L-gulonic acid (2-KLG) via a simple chemical reaction, which uses D-sorbitol as a substrate (Cai-Yun Wang et al., 2018; Zhang et al., 2018; Zeng et al., 2020). In addition, 2-KLG can also be directly generated by 2,5-diketo-D-gluconic acid (2,5-DKG) via a simple reduction reaction (Sonoyama et al., 1982). 2,5-DKG is commonly and directly synthesized using D-glucose as a substrate. In addition to 2,5-DKG being the precursor of 2-KLG, recent studies reported its synthesis may also increase rare-earth element recovery in wastewater (Jindra et al., 2016; Schmitz et al., 2021). As 2,5-DKG reactions during synthesis and decomposition processes are unclear, further research in these areas may provide insights on one-step 2-KLG synthesis and environmental protection.
In Erwinia sp. (Sonoyama et al., 1988), G. oxydans (Ji and Gao, 2001), Pseudomonas putida (Nikel and de Lorenzo, 2018), and Tatumella citrea (previously known as Erwinia citrea) (Andreeva et al., 2011; Marin-Cevada and Fuentes-Ramirez, 2016), D-glucose is gradually oxidized to D-gluconic acid (GA), 2-keto-D-gluconic acid (2-KG), and 2,5-DKG via glucose dehydrogenase (GDH), D-gluconic acid dehydrogenase (GADH), and 2-keto-D-gluconic acid dehydrogenase (2-KGDH), respectively, on the cell membrane or in the periplasmic space (Kataoka et al., 2015). Then, 2,5-DKG can be transformed into 2-KLG by 2,5-DKG reductase from Corynebacterium glutamate (Badwar et al., 2020). Based on the reported synthesis and metabolism of 2,5-DKG, a new one-step fermentation route was established for 2-KLG production directly from D-glucose, using recombinant Erwinia herbicola encoding the 2,5-DKG reductase gene from Corynebacterium sp. (Anderson et al., 1985). As one of the cheapest carbon sources, the use of D-glucose for 2-KLG production effectively solves the disadvantages of high energy consumption, high water consumption, long cycle, and difficulty in accurate control in other pathways. However, due to uncharacterized speed-limiting factors, 2,5-DKG pathway research is still in its infancy (Panpan Wang et al., 2018).
The main speed-limiting issue with the 2,5-DKG pathway is the transformation from 2,5-DKG to 2-KLG. This reaction is mainly catalyzed by 2,5-DKG reductase; therefore, generating a highly catalytically efficient 2,5-DKG reductase could facilitate a one-step fermentation system. Previous studies have investigated different strategies to strengthen 2,5-DKG conversion to 2-KLG, and increase 2-KLG production from D-glucose via the 2,5-DKG pathway; however, the effect is not obvious (Scott et al., 2002; Jeudy et al., 2006; Kaswurm et al., 2013).
In addition to generate an efficient 2,5-DKG reductase, it is important to select a good chassis strain (Vivek et al., 2021), optimize 2,5-DKG production processes, and identify possible speed-limiting factors. Previous studies investigated 2,5-DKG production improvements. A mixed culture of two bacteria from soil was developed to convert 50 g/L D-glucose to 2,5-DKG, and generated a 92% conversion ratio (Sulo et al., 2001). In addition, 65.8 g/L 2,5-DKG was harvested from a 10-L bioreactor using a high-performance liquid chromatography (HPLC) method (Qazi et al., 1991). However, the fermentation time in these studies is relatively long, generally more than 120 h. According to our previous studies, due to the lack of standards and the deviation of detection methods, these research data may have large errors. Therefore, the development of a relatively accurate quantitative method monitoring 2,5-DKG changes is warranted.
In this study, we established a quantitative detection method for 2,5-DKG based on pure enzyme catalysis. Using this method, we compared 2,5-DKG production levels in five strains, with G. oxydans ATCC 9937 selected as the optimum strain. After analyzing G. oxydans ATCC 9937-mediated 2,5-DKG production from D-glucose, a putative speed-limiting factor was identified. By knocking out and overexpressing the gene encoding the putative rate-limiting enzyme, we identified browning issues, caused by prolonged fermentation times, as the main factor leading to low 2,5-DKG accumulation. While browning is irreversible, it can be slowed down by shortening fermentation times. To reduce 2,5-DKG production losses caused by browning, we accelerated strain growth and reduced fermentation times by optimizing D-glucose addition at the start of fermentation. Finally, the browning degradation of 2,5-DKG was reduced and production generated a 139.02% increase using the feeding mode. This study provides a basis for further improving the one-step fermentation of D-glucose to produce 2-KLG.
Materials and Methods
Genes, Plasmids, and Strains
Escherichia coli BL21 (DE3) and vector pET28a were obtained from Sangon Biotech Corporation (Shanghai, China), and used to express 2,5-DKG reductase. C. glutamicum ATCC 13032 was purchased from the American Type Culture Collection (ATCC), and used to amplify the gene of 2,5-DKG reductase. The 2,5-DKG reductase gene from C. glutamicum ATCC 13032 was amplified with primer pair dkg-F and dkg-R. The pk18mobsacb vector was used to knock out kgdSLC gene. The plasmid pBBR1MCS-2 is used as a vector to overexpress kgdSLC gene.
G. oxydans ATCC 9937, P. putida ATCC 21813 and C. glutamicum ATCC 13032 were purchased from the American Type Culture Collection (ATCC); Tatumella citrea CICC 10802 and T. citrea CICC 10803 strains were obtained from the China Center of Industrial Culture Collection (Beijing, China); P. putida KT 2440 was preserved in our laboratory. All these strains were cultured in fermentation medium to verify their 2,5-DKG yield.
All primers are list in Table 1.
Media and Culture Condition
D-Gluconic acid, 2-KG, 5-keto-D-gluconic acid (5-KG), and 2-KLG were purchased from Toronto Research Chemicals INC. (Toronto, Canada). An Aminex HPX-87H liquid chromatographic column was purchased from Bio-Rad Laboratories INC. (CA, United States). Yeast extract was purchased from Oxoid (Hampshire, United Kingdom).
D-Sorbitol medium preparation: D-sorbitol (50 g/L), yeast extract (10 g/L), KH2PO4 (1 g/L), MgSO4 (0.25 g/L), and 20 g/L agar were added to solid medium. Fermentation medium: 100 g/L D-glucose, 10 g/L yeast extract, and 0.25 g/L MgSO4.
G. oxydans ATCC 9937 was grown on D-sorbitol solid medium for 20 h at 30°C, after which a single colony was transferred to liquid D-sorbitol medium and cultivated for 24 h at 30°C as seeding, and then was transferred to fermentation medium. T. citrea CICC10802, T. citrea CICC10803, P. putida ATCC21812, P. putida KT2440, and E. coli BL21 were activated in a solid LB medium at 30°C for 16 h and then transferred to a liquid LB medium at 30°C for 16 h, after which was transferred to fermentation medium.
Batch fermentation was performed in a 5-L fermenter (T&J-B type, J Bio-engineering Co., Ltd, Shanghai, China). The stirring speed gradually increased from 300 to 500 rpm and the ventilation ratio was 1 vvm. A pH of 4.9 was automatically maintained by adding 6 mol/L NaOH.
Preparation Method of 2,5-DKG
The fermentation broth was collected and centrifuged to remove bacteria. Used HyperSep Retain-AX solid-phase extraction column (ThermoFisher Scientific, Healthpoint, United States) to remove some protein impurities and pigments in the supernatant, and then lyophilized and stored, and dissolved in water after use.
Overexpression and Purification of 2,5-DKG Reductase
E. coli BL21 (DE3) cells expressing the recombinant plasmid pET28a-Histag-2,5-DKGR were cultured at 220 rpm for 10 h at 37°C. Then, 500 µL of the culture was transferred to TB medium and grown at 220 rpm at 37°C. When the OD600 reached 0.8, the temperature was reduced to 20°C, 0.1 mM isopropyl β-d-1-thiogalactopyranoside was added, and incubation continued for 16 h. Then, cells were harvested by centrifugation at 8,000 g for 5 min. After washing twice in phosphate buffer saline (PBS), cells were disrupted using a high-pressure cell cracker, and the crude enzyme solution was clarified by centrifugation (Lei et al., 2019). The solution was purified using a protein purifier, quick-frozen in liquid nitrogen, and stored at −80°C.
Catalytic Studies on 2,5-DKG Reductase
We prepared different concentrations of 2,5-DKG. Then, added 100 µL 2,5-DKG reductase and excess NADPH, supplemented the volume to 1 ml with Tris-HCl (pH 7.0), and incubated samples at 30°C for 4 h to ensure the substrate was completely transformed to 2-KLG. (Scott et al., 2002). Then, the concentration of 2-KLG in the system was detected by HPLC. In the first reaction, a small amount of 2,5-DKG and sufficient NADPH were added, and reacted for more than 4 h to ensure that 2,5-DKG is completely converted into 2-KLG. Then, the concentration of 2,5-DKG was gradually increased, and the NADPH to be added was estimated according to the results of the first reaction, so as to ensure that the cofactor is always sufficient or excessive to ensure the complete conversion of each time.
To verify the accuracy of the standard curve, we fitted the calculated 2,5-DKG concentration to the HPLC peak area (Figure 1B), and the relationship between the two was determined as follows:
where x = the 2,5-DKG concentration and y = the peak area; therefore, the R2 value of the relationship = 0.9924, indicating relatively high accuracy of the detection method (Figure 1B).
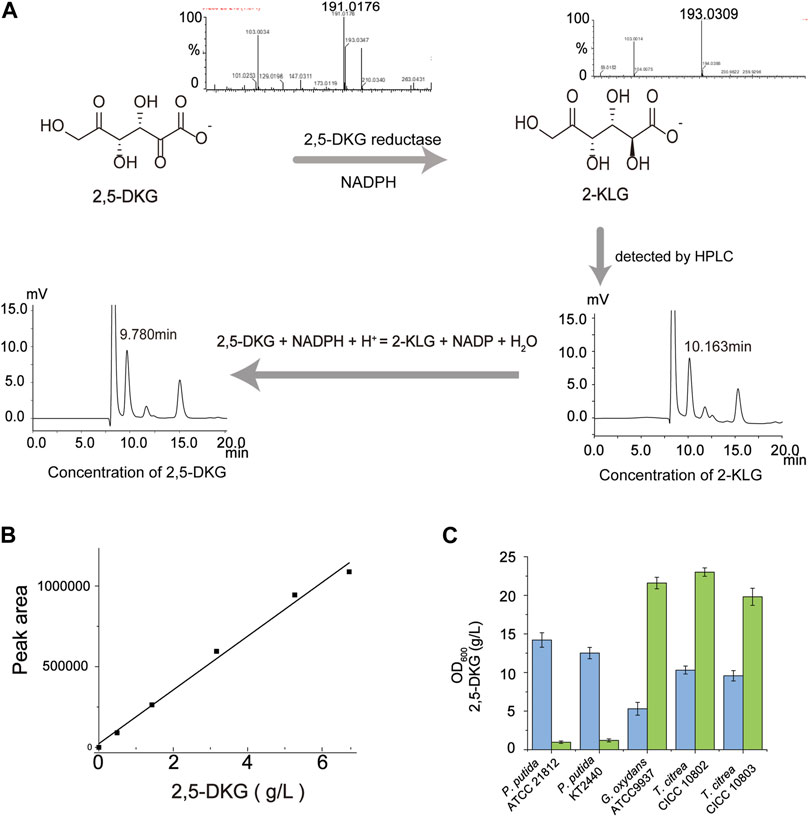
FIGURE 1. Quantification of 2,5-DKG by indirect HPLC analysis. (A) The peak time of 2,5-DKG is different from that of 2-KLG in HPLC detection. Therefore, 2,5-DKG which is difficult to quantify can be transformed into 2-KLG which is easier to quantify, and the real concentration of 2,5-DKG can be calculated from the concentration of 2-KLG. (B) Standard 2,5-DKG curve. (C) Comparing 2,5-DKG production in different strains. P. putida ATCC 21812 (No production); P. putida KT 2440 (No production); G. oxydans ATCC 9937 (21.6 g/L); T. citrea CICC 10802 (23.6 g/L); and T. citrea CICC 10803 (19.8 g/L). Blue = OD600; green = 2,5-DKG concentrations.
Knockout and Overexpression of kgdSLC Gene
The pk18mobsacb vector containing SacB system, previously developed in our laboratory, was used to edit genes in the G. oxydans ATCC 9937 strain (Chen et al., 2021). Using the G. oxydans ATCC 9937 genome as a template, primers were designed to amplify 1000 bp homologous arms upstream and downstream of the kgdS, kgdL and kgdC for PCR knockout (Table 1). Then, upstream and downstream homologous arms of the target gene were recombined using the pK18mobsacb vector to generate a recombinant plasmid. After verification, the plasmid was transformed into G. oxydans ATCC 9937. For more detailed procedures, please refer to our previous study (Qin et al., 2021).
Using the G. oxydans ATCC 9937 genome as a template, primer pair pBBR-kgdSLC-F and pBBR-kgdSLC-R were designed to amplify kgdSLC, primer pair P7-F and P7-R were designed to amplify promoter sequence. Then, kgdSLC and promoter sequence were recombined using the pBBR1MCS-2 vector to generate a recombinant. After verification, the plasmid was transformed into G. oxydans ATCC 9937 to overexpress kgdSLC.
Biomass and High-Performance Liquid Chromatography Analysis
To avoid fermentation broth interference in terms of color generation for spectrophotometric assay, bacteria were centrifuged, resuspended in an equal volume of PBS, and OD600 values were recorded.
D-Gluconic acid, 2-KG, 2,5-DKG, and 2-KLG levels were detected and quantified by HPLC using a Shimadzu LC-20A system equipped with a refractive index detector on an Aminex HPX-87H column at 40°C in 5 mmol/L H2SO4 eluent (0.5 ml/min = flow rate).
2,5-DKG and 2-KLG were measured using LC-MS under the conditions of Table 2.
Browning Measurement by Ultraviolet Spectrophotometer
Samples were taken every 12 h to detect the content of 2,5-DKG and the degree of browning. The browning of fermentation broth was reflected by the absorbance value at 420 nm (Zhao et al., 2017; Tavares et al., 2018).
Result
Identifying an Optimum 2,5-DKG-Producing Strain Using Enzyme-Based Detection
The traditional host strain as a one-step fermentation pathway from D-glucose to 2-KLG has been developing slowly. Therefore, we plan to re-screen the appropriate chassis strain and optimize its ability to produce 2,5-DKG. To obtain such a high producer of 2,5-DKG, an effective detection method of 2,5-DKG is required to be established. 2,5-DKG has no standard and lacks quantitative basis, but its oxidation product 2-KLG can be easily quantified. In this study, 2,5-DKG was transformed into 2-KLG by 2,5-DKG reductase, and the actual content of 2,5-DKG was calculated by quantitative analysis of 2-KLG. Firstly, we compared the peak time difference between 2,5-DKG and 2-KLG in HPLC. The peak time of the two were 9.35 and 10.16 min, respectively. Then, the compound 2,5-DKG was completely converted to 2-KLG under the presence of excess cofactor NADPH (Figure 1A); the substrate 2,5-DKG and product 2-KLG were detected by LC-MS. After the concentration of 2-KLG in the solution is detected, the actual concentration of 2,5-DKG in the solution is calculated according to 100% conversion. To verify the accuracy of this method, a standard curve was produced for the 2,5-DKG quantification based on the calculation of molar conversion (Figure B), and the results show that the detection method is reasonable.
Based on this quantitative method, five strains producing 2,5-DKG were compared (Figure 1C), including G. oxydans ATCC 9937, T. citrea CICC 10802, T. citrea CICC 10803, P. putida ATCC 21813, and P. putida KT 2440. After comparing the yield of 2,5-DKG and OD600 of different strains, G. oxydans ATCC 9937 was identified as having the highest 2,5-DKG production (4.08 g/L/OD600) and was chosen as the starting strain. The strain produced 21.6 g/L 2,5-DKG from 100 g/L D-glucose, with a conversion rate of 20.7%. The conversion rate of G. oxydans ATCC9937 from D-glucose to 2,5-DKG is not high. In order to analyze the reasons for the low conversion rate, a detailed understanding of the whole metabolic process and conversion rate of G. oxydans ATCC9937 synthesizing 2,5-DKG with D-glucose as the substrate are necessary.
Metabolites in the Fermentation Process of 2,5-DKG by G. oxydans ATCC 9937
In G. oxydans, D-glucose is oxidized to GA by membrane-bound GDH, then further oxidized to 2-KG or 5-KG by GADH or glycerol dehydrogenase (Qazi et al., 1991) (Figure 2A). As only 2-KG is oxidized to 2,5-DKG by 2-KGDH. To find out the possible rate-limiting factors, this study verified its ability to produce 2,5-DKG. Through the detection of samples in different fermentation cycles, the product of G. oxydans ATCC9937 in the fermentation medium with D-glucose as the carbon source is shown in Figure 2B. This study did not detect 5-KG in the medium, it was speculated that the process of G. oxydans ATCC 9937 did not or only produce a small amount of 5-KG.
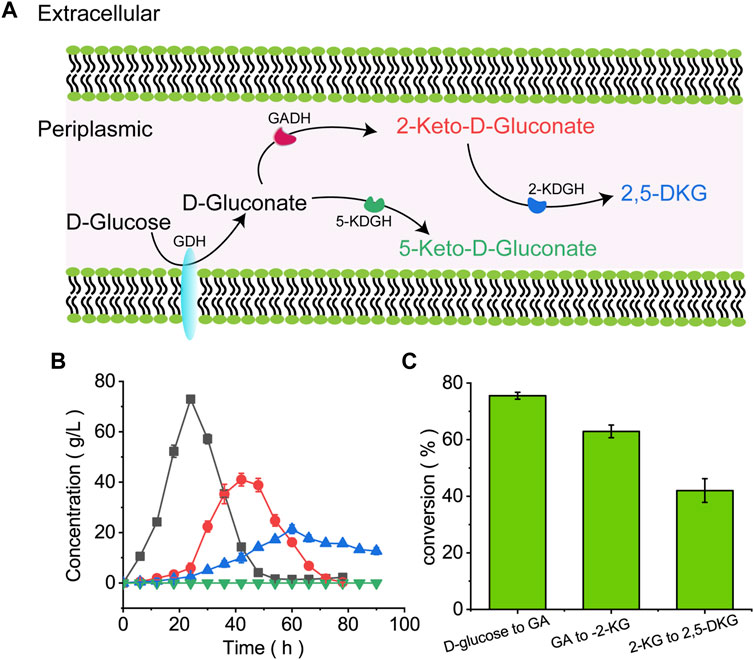
FIGURE 2. The microbial pathway showing D-glucose conversion to 2,5-DKG. (A) D-Glucose conversion to 2,5-DKG in the periplasmic space; (B) Consumption and formation of D-gluconic acid, 2-KG, 2,5-DKG, and 5-KG by G. oxydans ATCC 9937. Black = D-gluconic acid levels; red = 2-KG levels; blue = 2,5-DKG levels; green = 5-KG levels. (C) Illusion rate of various substances.
G. oxydans ATCC 9937 consumed D-glucose in the first 24 h to generate GA and low 2-KG and 2,5-DKG levels. D-gluconic acid accumulation peaked at 24 h, about 72.96 g/L, and the conversion of D-glucose to GA is about 75.5%. GA was consumed after 52 h, 2-KG peaked at 41.03 g/L at approximately 42 h, and the conversion of GA to 2-KG is about 62.8%. 2,5-DKG peaked at 21.3 g/L at approximately 60 h, and then levels of 2,5-DKG gradually decreased to 12.7 g/L at 90 h. Although the results showed that 2-KG was completely consumed in about 68 h, the conversion of 2-KG to 2,5-DKG is only 41.4% (Figure 2C), which is relatively low. The reason for this phenomenon may be an important rate-limiting factor for the accumulation of 2,5-DKG.
Knockout and Overexpression of kgdSLC
To block the synthesis of 2,5-DKG and find out other possible transformation pathways of 2-KG and optimize them, it is necessary to edit the coding gene of 2-KGDH. The gene sequence encoding 2-KGDH in G. oxydans ATCC9937 was found by blast. 2-KGDH contains three subunits, kgdS, kgdL, and kgdC (Shinagawa et al., 2014; Kataoka et al., 2015). In order to further determine that the sequence is correct, we knocked out the three subunits respectively. The result showed that, when compared with original control bacteria, all knockouts failed to convert 2-KG to 2,5-DKG. Until 156 h of fermentation, 2-KG concentrations remained at about 75.1 g/L (Figure 3A); the figure showed that the true conversion rate of GA to 2-KG is about 87.8%, which is much higher than the value we calculated before. No other substances were detected other than 2-KG.
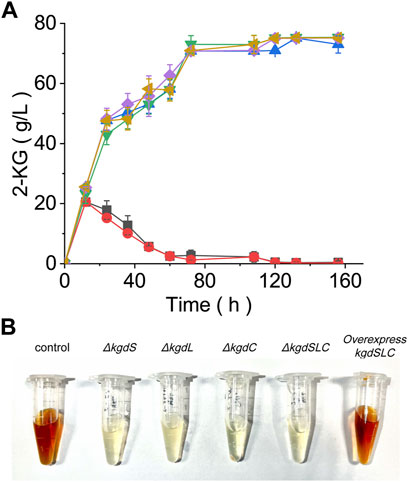
FIGURE 3. Evaluating the fermentation performance of the kgdSLC knockout strain. (A) Changes in 2-KG concentrations in fermentation broth after kgdSLC knockout and overexpression. Green = kgdL knockout. Yellow = kgdS knockout. Blue = kgdC knockout. Purple = combined knockout. Red = Overexpressed kgdSLC. Black = control cells. (B) Broth color changes after kgdSLC knockout. Regardless of single or combined knockout, the broth no longer turned brown; when kgdSLC was overexpressed, the color of the fermentation broth turned brown.
In the absence of side reaction, the low efficiency of 2-KG to 2,5-DKG may also be caused by the low activity or expression of 2-KDGH. So, we used pBBR1MCS-2 plasmid to complement kgdSLC gene, and replaced the p7 promoter with higher strength to improve the copy number of kgdSLC. Then 2-KG can be transformed into 2,5-DKG again, but 2,5-DKG production did not change significantly when compared with the original control bacteria. Thus, the catalytic efficiency of 2-KGDH did not change significantly whether kgdSLC was overexpressed or not. The conversion rate of D-glucose to 2-KG was as high as 76.3%, while the final conversion rate to 2,5-DKG during fermentation was only 23.1%. However, the knockout and overexpression results of kgdSLC showed that the main rate-limiting factor of the reaction maybe is not the conversion of 2-KG. The knockout and overexpression of kgdSLC also brought another phenomenon; when compared with the yellowish color of the fermentation broth of control cells, the experimental group, which no longer produced 2,5-DKG, was always clear (Figure 3B). In control cells, with increased fermentation time, broth color gradually deepened and finally changed to dark brown, similar to soy sauce. In the earliest relevant studies, it is always mentioned that the production of 2,5-DKG is always accompanied by the color change in fermentation broth. But this color change is more like browning in Maillard reaction. It is necessary to study whether the color change is caused by the browning of 2,5-DKG or other factors.
Non-Enzymatic Browning is the Main Factor Leading to the Degradation of 2,5-DKG
The OD420 value of samples is usually an important index to detect the degree of browning in Maillard reaction (Paravisini and Peterson, 2019). To investigate whether the color change in fermentation broth is related to 2,5-DKG, the OD420 values and 2,5-DKG concentrations in fermentation broth at different periods were measured. With increased fermentation time, broth color gradually deepened, and OD420 values maintained an increasing trend. Also, 2,5-DKG concentrations maintained an increasing trend in the early stages, but then declined after peaking at 60 h; the concentration was only 74.52% of the maximum value after 72 h (Figure 4A). Then, 2,5-DKG solution was prepared with a concentration of about 15 g/L, and the same quantity of glutamine as 2,5-DKG was added. After this addition, OD420 absorbance values increased significantly when compared with control group (2,5-DKG solution without glutamine). After 150 h, 2,5-DKG concentrations in control group decreased by 53.9%, and in the experimental plus glutamine group, it decreased by 90.1% (Figure 4B). By fitting OD420 absorbance values of both groups with 2,5-DKG concentrations, we identified a negative correlation between OD420 and 2,5-DKG concentrations, but the relationship was not linear.
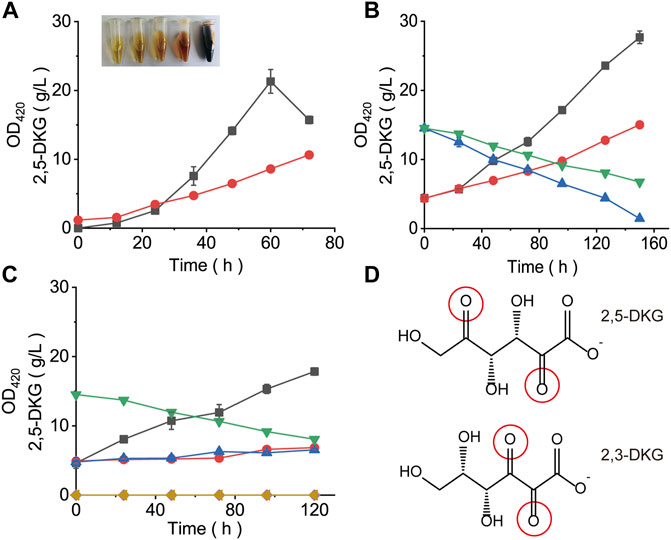
FIGURE 4. Browning formation (absorbance at 420 nm) and the concentration of 2,5-DKG under different conditions. (A) Concentration of 2,5-DKG and absorbance at 420 nm in fermentation broth. Black = 2,5-DKG concentration. Red = Absorbance at 420 nm. With increased fermentation time, 2,5-DKG levels gradually decreased, and the degree of browning gradually increased. (B) 2,5-DKG concentration and absorbance at 420 nm in broth plus glutamine. Red = OD420 in control cells without glutamine. Black = OD420 in the experimental group plus glutamine. Green = 2,5-DKG concentration in control cells. Blue = 2,5-DKG concentration in the experiment group. (C) 2,5-DKG concentration and absorbance at 420 nm in broth plus browning inhibitor. Black = OD420 in control cells without a browning inhibitor. Blue = OD420 in the experimental group plus the browning inhibitor sodium sulfite. Red = OD420 in the experimental group plus the browning inhibitor potassium sulfite. Green = 2,5-DKG concentration in control cells. Yellow = 2,5-DKG concentration in the experiment group. (D) 2,5-DKG and 2,3-DKG structures.
To further verify that browning is caused by 2,5-DKG, we added a Maillard inhibitor to destroy 2,5-DKG, and then browning will not occur again whether glutamine was added or not (Figure 4C). The current research results showed that the degradation of 2,5-DKG leads to the continuous deepening of the color of fermentation broth, and the main mode of degradation is non-enzymatic browning. This study tried to eliminate or inhibit the browning phenomenon of 2,5-DKG, but it did not work. 2,5-DKG continued browning and degraded with the increase in storage time unless refrigerated. Therefore, in order to reduce the browning of 2,5-DKG and improve the yield of 2,5-DKG, minimizing the fermentation time through optimization is the most feasible and efficient way.
Enhanced 2,5-DKG Production by Reducing Fermentation Period
Inappropriate fermentation conditions will inhibit the growth and metabolism of bacteria, so as to prolong the fermentation time and increase the browning degradation rate of 2,5-DKG. In order to reduce the browning rate and increase the yield of 2,5-DKG, we optimized the fermentation process. In this study, the optimization was carried out by changing the initial D-glucose concentration to reduce the growth inhibition of the strain. To find the best initial D-glucose concentration, we compared the growth curves of strains under different D-glucose concentrations, result showed that the strain grew fastest at an initial D-glucose concentration of 20 g/L (Figure 5A), therefore 20 g/L initial D-glucose concentration was used to optimize the subsequent fermentation process. When the initial D-glucose concentration was reduced to 20 g/L, the ability of the strain to produce 2,5-DKG was significantly accelerated, 12.3 g/L of 2,5-DKG was obtained in 16 h, and the growth of the strain was also significantly accelerated. The biomass of 16 h increased by about 56% compared with the control group (100 g/L D-glucose).
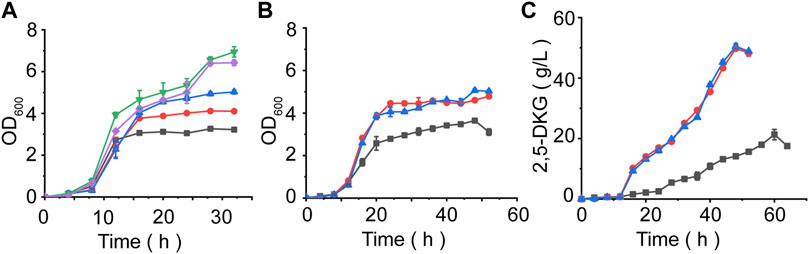
FIGURE 5. Evaluating fermentation performances under different conditions. (A) G. oxydans ATCC9937 growth curve at different D-glucose concentrations. Black = 5 g/L D-glucose. Red = 10 g/L D-glucose. Blue = 15 g/L D-glucose. Green = 20 g/L D-glucose. Purple = 25 g/L D-glucose. (B) The effect of different feeding methods on OD600 values. (C) Time course of 2,5-DKG production using different feeding methods. Black = control, initial D-glucose concentration = 100 g/L. Blue: After initial glucose levels were consumed, further glucose was added in a constant-rate feeding mode. Red: Further glucose was added in a single-dose feed-batch mode after initial glucose quantities were consumed.
In order to further increase the yield of 2,5-DKG, after the initial D-glucose consumption of 20 g/L is completed, we continued to add D-glucose by feeding. The feeding is carried out in two ways; 1) by adding all the remaining D-glucose at one time, and 2) concentrations were maintained at 20 g/L by feeding to finally reach a concentration of 100 g/L. The results showed that the strains grew rapidly in the first 20 h of fermentation, and began to stabilize after 24 h, whether supplemented or not (feeding began at approximately 16 h). However, after reducing the initial D-glucose concentration, the growth of the strain was significantly better than that of the control group. The OD600 of the experimental group was approximately 1.4 times that of the control group, and the final OD600 of different feeding methods has little difference (Figure 5B). When compared with control group, 2,5-DKG production peaked after 48 h, and fermentation time had been reduced by approximately 10 h. 2,5-DKG production increased by 139.02% and 133.63%, peaking at 50.9 g/L and 49.7 g/L, in constant-rate feeding mode and single-dose feed-batch mode, respectively (Figure 5C). Additionally, productivity [1.06 g/(L/h)] was significantly enhanced when compared with results from a previous study (Table 3).
Discussion
Fermentation broth containing 2,5-DKG is usually darker in color, and therefore, the detection method, which was based on NH4OH-HCl reactions (Sulo et al., 2001), caused a large deviation due to culture broth interference. This inevitably affected the absorbance and generated higher 2,5-DKG yields. HPLC approach was relatively accurate; however, a good standard was required to generate a standard curve as 2,5-DKG standards were commercially unavailable, and product purity from self-purification is low. So, there is still a large deviation in the HPLC detection method, resulting in that the detection value of 2,5-DKG in previous studies is much greater than its real value (Qazi et al., 1991). Based on this problem, the method of pure enzyme catalysis can effectively avoid the shortcomings of no standard or insufficient purity of standard, and more accurately quantify 2,5-DKG in the reaction system.
The chassis strain G. oxydans ATCC9937 screened by the new quantitative method has a strong membrane dehydrogenase system (Bringer and Bott, 2016; Zou et al., 2017; Jin et al., 2019; Zhou et al., 2019; Stefanie et al., 2021), only about 10% D-glucose is phosphorylated into the pentose phosphate pathway (PPP pathway) after entering the cell and is used for oxidative function and cell growth (Krajewski et al., 2010). Most D-glucose is oxidized in its periplasmic space (Dai et al., 2022) and the final product of oxidation is 2,5-DKG. Some strains cannot completely consume D-gluconic acid, resulting in the conversion of D-gluconic acid to 2-KG becoming the rate-limiting factor, but this situation did not exist in G. oxydans ATCC9937, which can completely consume the D-gluconic acid and 2-KG produced in the fermentation broth, but the final 2,5-DKG is less, and the conversion from 2-KG to 2,5-DKG is low.
The conventional idea of metabolic engineering is to directly overexpress the rate-limiting enzyme to improve the yield, but this operation is not conducive to the discovery of side effects. 2-KG can be completely consumed, but the yield of its oxidized product 2,5-DKG is very low. When the cause of this situation is unclear, it is very important to first verify whether there are side reactions and convert 2-KG into other substances. To find the speed-limiting factor more accurately, this study first knocked out the possible rate-limiting factor kgdSLC, and then verified its overexpression. However, the knockout and overexpression results of kgdSLC showed that the metabolic pathway of by-products of 2-KG did not seem to exist or was very few. 2-KG is a relatively stable compound, which can exist in the fermentation broth for a long time without other degradation reactions. The powerful periplasmic dehydrogenase system of G. oxydans ATCC9937 can ensure the continuous and efficient reaction of D-glucose to 2,5-DKG, overexpression of kgdSLC by genetic engineering could not further increase the yield of 2,5-DKG. 2-KDGH is not the main rate-limiting factor of the reaction, and the main speed-limiting factor should be the degradation of 2-KG. Many studies have mentioned that 2,5-DKG is easy to degrade, but no research has proved how it is degraded. The data provided in this study showed that the main reason for the low yield of 2,5-DKG is its continuous degradation in the fermentation broth, and the main mode of degradation is browning.
Many substances will be browning during storage (Tan et al., 2020; Fatouros et al., 2021). Browning mainly includes enzymatic and non-enzymatic two pathways (Paravisini and Peterson, 2019; Pham et al., 2020). The browning of 2,5-DKG is mainly through non-enzymatic reaction. This color change was similar to L-ascorbic acid browning, which is caused by a series of complex reactions during its degradation (Paravisini and Peterson, 2019), but is primarily caused by 2,3-DKG (2,3-diketo-L-gulonic acid) production (Forouhar et al., 2004). The hydration of 2,3-DKG occurs during degradation and generates colored substances (Penney and Zilva, 1945). As 2,5-DKG contained two carbonyls (Figure 4D), it may have had similar hydration reactions to 2,3-DKG, thereby producing colored substances. Previous studies suggested that 2,3-DKG may have similar antioxidant properties to L-ascorbic acid (Li et al., 2001). Whether 2,5-DKG is also prone to browning, due to its strong reductive capabilities, warrants further research. Therefore, using color deepening as a standard for 2,5-DKG generation is inaccurate. Thus, 2,5-DKG had no color, whereas a yellowish-brown color was caused by browning. Importantly, this browning required no specific temperature; even at 30°C, 2,5-DKG continued browning with increased content and fermentation time. Because the substances produced by browning are complex, we have not analyzed the detailed browning products for the time being, which may be targeted in subsequent studies. Moreover, the browning phenomenon was irreversible; the only way to slow down the process was to shorten fermentation times and accelerate substrate conversion to 2,5-DKG.
In previous studies, fermentation 2,5-DKG usually takes more than 120 h. However, continuous aeration and stirring during fermentation will further accelerate the browning of 2,5-DKG. Too long fermentation time leads to a large amount of browning of 2,5-DKG in the fermentation broth, resulting in very low actual purity of 2,5-DKG. Although the powerful dehydrogenase system enables to oxidize sugar alcohols in the reaction system without excessive biomass (Zhou et al., 2019; Han et al., 2021), too high initial sugar concentration will still inhibit its growth rate and corresponding enzyme activity, resulting in the increase in reaction time. By adjusting the initial D-glucose concentration in the broth, cell growth and substrate transformation efficiency were both accelerated. The time of bacterial transformation of substrate was also greatly shortened, the significant shortening of fermentation time reduced the browning rate of 2,5-DKG in the medium and greatly increased the final yield of 2,5-DKG. Browning will not only affect the accumulation of 2,5-DKG but also the dark substances produced by browning will stain the cell membrane, which will seriously affect the construction of the subsequent 2,5-DKG to 2-KLG pathway. Further research is required to analyze the main mechanisms underpinning 2,5-DKG browning, verify whether there are metabolites easy to combine with it to cause browning in the fermentation process, and further transform the metabolic pathway.
Data Availability Statement
The original contributions presented in the study are included in the article/Supplementary Material; further inquiries can be directed to the corresponding author.
Author Contributions
GL: methodology, investigation, formal analysis, writing—original draft. XS: investigation, writing—original draft, validation. WZ, SY, and GZ: formal analysis, writing—review and editing. JC: funding acquisition. JZ: supervision, funding acquisition, writing—review and editing.
Funding
This work was supported by the National Key Research and Development Program of China (2019YFA0904900) and National Natural Science Foundation of China (Key Program, 31830068).
Conflict of Interest
The authors declare that the research was conducted in the absence of any commercial or financial relationships that could be construed as a potential conflict of interest.
Publisher’s Note
All claims expressed in this article are solely those of the authors and do not necessarily represent those of their affiliated organizations, or those of the publisher, the editors, and the reviewers. Any product that may be evaluated in this article, or claim that may be made by its manufacturer, is not guaranteed or endorsed by the publisher.
Supplementary Material
The Supplementary Material for this article can be found online at: https://www.frontiersin.org/articles/10.3389/fbioe.2022.918277/full#supplementary-material
References
Anderson, S., Marks, C. B., Lazarus, R., Miller, J., Stafford, K., Seymour, J., et al. (1985). Production of 2-Keto-L-Gulonate, an Intermediate in L-Ascorbate Synthesis, by a Genetically Modified Erwinia Herbicola. Science 230 (4722), 144–149. doi:10.1126/science.230.4722.144
Andreeva, I. G., Golubeva, L. I., Kuvaeva, T. M., Gak, E. R., Katashkina, J. I., and Mashko, S. V. (2011). Identification of Pantoea Ananatis Gene Encoding Membrane Pyrroloquinoline Quinone (PQQ)-dependent Glucose Dehydrogenase and pqqABCDEF Operon Essential for PQQ Biosynthesis. FEMS. Microbiol. Lett. 318 (1), 55–60. doi:10.1111/j.1574-6968.2011.02240.x
Anitra, C., and Silvia, M. (2017). Vitamin C and Immune Function. Nutrients 9 (11), 1211. doi:10.3390/nu91112111
Badwar, M. R., Bakliwal, A. A., Talele, S. G., and Jadhav, A. G. (2020). “Generation of Natural Pharmaceuticals Based on Microbial Transformation of Herbal Constituents,” in Applied Pharmaceutical Science and Microbiology: Novel Green Chemistry Methods and Natural Products, 61–82. doi:10.1201/9781003019565-4
Bringer, S., and Bott, M. (2016). “Central Carbon Metabolism and Respiration in Gluconobacter Oxydans,” in Acetic Acid Bacteria, 235–253. doi:10.1007/978-4-431-55933-7_11
Cendrowski, A., Królak, M., and Kalisz, S. (2021). Polyphenols, L-Ascorbic Acid, and Antioxidant Activity in Wines from Rose Fruits (Rosa Rugosa). Molecules 26 (9), 2561. doi:10.3390/molecules26092561
Chen, Y., Liu, L., Yu, S., Li, J., Zhou, J., and Chen, J. (2021). Identification of Gradient Promoters of Gluconobacter Oxydans and Their Applications in the Biosynthesis of 2-Keto-L-Gulonic Acid. Front. Bioeng. Biotechnol. 9, 673844. doi:10.3389/fbioe.2021.673844
Dai, L., Jiang, W., Jia, R., Zhou, X., and Xu, Y. (2022). Directional Enhancement of 2-Keto-Gluconic Acid Production from Enzymatic Hydrolysate by Acetic Acid-Mediated Bio-Oxidation with Gluconobacter Oxydans. Bioresour. Technol. 348, 126811. doi:10.1016/j.biortech.2022.126811
Fatouros, A., Einhorn-Stoll, U., Kastner, H., Drusch, S., and Kroh, L. W. (2021). Influence of the Carboxylic Function on the Degradation of D-Galacturonic Acid and its Polymers. J. Agric. Food Chem. 69 (32), 9376–9382. doi:10.1021/acs.jafc.1c02388
Forouhar, F., Lee, I., Benach, J., Kulkarni, K., Xiao, R., Acton, T. B., et al. (2004). A Novel NAD-Binding Protein Revealed by the Crystal Structure of 2,3-Diketo-L-Gulonate Reductase (YiaK). J. Biol. Chem. 279 (13), 13148–13155. doi:10.1074/jbc.M313580200
Han, J., Hua, X., Zhou, X., Xu, B., Wang, H., Huang, G., et al. (2021). A Cost-Practical Cell-Recycling Process for Xylonic Acid Bioproduction from Acidic Lignocellulosic Hydrolysate with Whole-Cell Catalysis of Gluconobacter Oxydans. Bioresour. Technol. 333, 125157. doi:10.1016/j.biortech.2021.125157
Islam, J., Chilkoor, G., Jawaharraj, K., Dhiman, S. S., Sani, R., and Gadhamshetty, V. (2020). Vitamin-C-enabled Reduced Graphene Oxide Chemistry for Tuning Biofilm Phenotypes of Methylotrophs on Nickel Electrodes in Microbial Fuel Cells. Bioresour. Technol. 300, 122642. doi:10.1016/j.biortech.2019.122642
Jeudy, S., Monchois, V., Maza, C., Claverie, J.-M., and Abergel, C. (2006). Crystal Structure of Escherichia coli DkgA, a Broad-Specificity Aldo-Keto Reductase. Proteins 62 (1), 302–307. doi:10.1002/prot.20710
Ji, A., and Gao, P. (2001). Substrate Selectivity of Gluconobacter Oxydans for Production of 2,5-Diketo-D-Gluconic Acid and Synthesis of 2-Keto-L-Gulonic Acid in a Multienzyme System. Appl. Biochem. Biotechnol. 94 (3), 213–224. doi:10.1385/abab:94:3:213
Jin, C., Hou, W., Yao, R., Zhou, P., Zhang, H., and Bao, J. (2019). Adaptive Evolution of Gluconobacter Oxydans Accelerates the Conversion Rate of Non-glucose Sugars Derived from Lignocellulose Biomass. Bioresour. Technol. 289, 121623. doi:10.1016/j.biortech.2019.121623
Jindra, M. A., Reed, D. W., Thompson, V. S., and Daubaras, D. L. (2016). Developing a Scalable System for Biorecovery of Critical Materials from Industrial Waste with Gluconobacter Oxydans. Idaho Falls, ID (United States): Idaho National Lab.INL.
Kaswurm, V., Van Hecke, W., Kulbe, K. D., and Ludwig, R. (2013). Engineering of a Bi-enzymatic Reaction for Efficient Production of the Ascorbic Acid Precursor 2-Keto-L-Gulonic Acid. Biochem. Eng. J. 79, 104–111. doi:10.1016/j.bej.2013.07.010
Kataoka, N., Matsutani, M., Yakushi, T., and Matsushita, K. (2015). Efficient Production of 2,5-Diketo-D-Gluconate via Heterologous Expression of 2-ketogluconate Dehydrogenase in Gluconobacter Japonicus. Appl. Environ. Microbiol. 81 (10), 3552–3560. doi:10.1128/AEM.04176-14
Krajewski, V., Simić, P., Mouncey, N. J., Bringer, S., Sahm, H., and Bott, M. (2010). Metabolic Engineering of Gluconobacter Oxydans for Improved Growth Rate and Growth Yield on Glucose by Elimination of Gluconate Formation. Appl. Environ. Microbiol. 76 (13), 4369–4376. doi:10.1128/AEM.03022-09
Lei, Q., Zeng, W., Zhou, J., and Du, G. (2019). Efficient Separation of Alpha-Ketoglutarate from Yarrowia Lipolytica WSH-Z06 Culture Broth by Converting Pyruvate to L-Tyrosine. Bioresour. Technol. 292, 121897. doi:10.1016/j.biortech.2019.121897
Li, M., Suzuki, E., and Kurata, T. (2001). Effects of 2,3-Diketo-L-Gulonic Acid on the Oxidation of Yolk Lipoprotein. Biosci. Biotechnol. Biochem. 65 (3), 599–604. doi:10.1271/bbb.65.599
Marin-Cevada, V., and Fuentes-Ramirez, L. E. (2016). Pink Disease, a Review of an Asymptomatic Bacterial Disease in Pineapple. Rev. Bras. Frutic. 38 (3), 8. doi:10.1590/0100-29452016949
Nikel, P. I., and de Lorenzo, V. (2018). Pseudomonas Putida as a Functional Chassis for Industrial Biocatalysis: from Native Biochemistry to Trans-metabolism. Metab. Eng. 50, 142–155. doi:10.1016/j.ymben.2018.05.005
Paravisini, L., and Peterson, D. G. (2019). Mechanisms Non-Enzymatic Browning in Orange Juice during Storage. Food Chem. 289, 320–327. doi:10.1016/j.foodchem.2019.03.049
Pardo-Planas, O., Prade, R. A., Müller, M., Atiyeh, H. K., and Wilkins, M. R. (2017). Prevention of Melanin Formation during Aryl Alcohol Oxidase Production under Growth-Limited Conditions Using an Aspergillus nidulans Cell Factory. Bioresour. Technol. 243, 874–882. doi:10.1016/j.biortech.2017.06.183
Penney, J. R., and Zilva, S. S. (1945). The Isolation of Barium and Calcium Diketo-L-Gulonates and the Biological Significance of 2:3-Diketo-L-Gulonic Acid. Biochem. J. 39 (1), 1–4. doi:10.1042/bj0390001b
Pham, H. T. T., Kityo, P., Buvé, C., Hendrickx, M. E., and Van Loey, A. M. (2020). Influence of pH and Composition on Nonenzymatic Browning of Shelf-Stable Orange Juice during Storage. J. Agric. Food Chem. 68 (19), 5402–5411. doi:10.1021/acs.jafc.9b07630
Qazi, G. N., Parshad, R., Verma, V., Chopra, C. L., Buse, R., Träger, M., et al. (1991). Diketo-Gluconate Fermentation by Gluconobacter Oxydans. Enzyme. Microb. Technol. 13 (6), 504–507. doi:10.1016/0141-0229(91)90010-8
Qin, Z., Yu, S., Liu, L., Wang, L., Chen, J., and Zhou, J. (2021). A SacB-Based System for Diverse and Multiple Genome Editing in Gluconobacter Oxydans. J. Biotechnol. 338, 31–39. doi:10.1016/j.jbiotec.2021.07.004
Reddy, R. C., Devaranavadagi, B., Yendigeri, S. M., Bagali, S., Kulkarni, R. V., and Das, K. K. (2020). Effect of L-Ascorbic Acid on Nickel-Induced Alteration of Cardiovascular Pathophysiology in Wistar Rats. Biol. Trace. Elem. Res. 195 (1), 178–186. doi:10.1007/s12011-019-01829-w
Schmitz, A. M., Pian, B., Medin, S., Reid, M. C., Wu, M., Gazel, E., et al. (2021). Generation of a Gluconobacter Oxydans Knockout Collection for Improved Extraction of Rare Earth Elements. Nat. Commun. 12 (1), 6693. doi:10.1038/s41467-021-27047-4
Scott, B., Swanson, B. A., Wu, S., Alisha, J., and Stephen, A. (2002). Alteration of the Specificity of the Cofactor-Binding Pocket of Corynebacterium 2,5-Diketo-D-Gluconic Acid Reductase A. Protein. Eng. 15 (2), 131–140. doi:10.1093/protein/15.2.131
Shinagawa, E., Matsushita, K., Adachi, O., and Ameyama, M. (2014). Purification and Characterization of 2-Keto-D-Gluconate Dehydrogenase from Gluconobacter Melanogenus. Agric. Biol. Chem. 45 (5), 1079–1085. doi:10.1080/00021369.1981.10864678
Sonoyama, T., Tani, H., Matsuda, K., Kageyama, B., Tanimoto, M., Kobayashi, K., et al. (1982). Production of 2-Keto-L-Gulonic Acid from D-Glucose by Two-Stage Fermentation. Appl. Environ. Microbiol. 43 (5), 1064–1069. doi:10.1128/aem.43.5.1064-1069.1982
Sonoyama, T., Yagi, S., and Kageyama, B. (1988). Facultatively Anaerobic Bacteria Showing High Productivities of 2,5-Diketo-D-Gluconate from D-Glucose. Agric. Biol. Chem. 52 (3), 667–674. doi:10.1271/bbb1961.52.667
Stefanie, S., Angela, K., Toshiharu, Y., Andrei, F., Tino, P., Helga, E., et al. (2021). FNR-type Regulator GoxR of the Obligatorily Aerobic Acetic Acid Bacterium Gluconobacter Oxydans Affects Expression of Genes Involved in Respiration and Redox Metabolism. Appl. Environ. Microbiol. 87 (11), e00195–00121. doi:10.1128/aem.00195-21
Sulo, P., Hudecová, D., Properová, A., Bašnák, I., and Sedláček, I. (2001). 2,5-Diketo- D-Gluconate Production by a Mixed Culture of Two Newly-Isolated Strains: Flavimonas Oryzihabitans and Pseudomonas cepacia. Biotechnol. Lett. 23 (9), 693–696. doi:10.1023/a:1010360603287
Tan, J., de Bruijn, W. J. C., van Zadelhoff, A., Lin, Z., and Vincken, J.-P. (2020). Browning of Epicatechin (EC) and Epigallocatechin (EGC) by Auto-Oxidation. J. Agric. Food Chem. 68 (47), 13879–13887. doi:10.1021/acs.jafc.0c05716
Tavares, W., Dong, S., Jin, W., Yang, Y., Han, K., Zha, F., et al. (2018). Effect of Different Cooking Conditions on the Profiles of Maillard Reaction Products and Nutrient Composition of Hairtail (Thichiurus Lepturus) Fillets. Food Res. Int. 103, 390–397. doi:10.1016/j.foodres.2017.10.063
Wang, C. Y., Li, Y., Gao, Z. W., Liu, L. C., Zhang, M. Y., Zhang, T. Y., et al. (2018). Establishing an Innovative Carbohydrate Metabolic Pathway for Efficient Production of 2-Keto-L-Gulonic Acid in Ketogulonicigenium Robustum Initiated by Intronic Promoters. Microb. Cell. Fact. 17 (81), 81. doi:10.1186/s12934-018-0932-9
Wang, P., Zeng, W., Xu, S., Du, G., Zhou, J., and Chen, J. (2018). Current Challenges Facing One-step Production of L-Ascorbic Acid. Biotechnol. Adv. 36 (7), 1882–1899. doi:10.1016/j.biotechadv.2018.07.006
Vivek, N., Hazeena, S. H., Alphy, M. P., Kumar, V., Magdouli, S., Sindhu, R., et al. (2021). Recent Advances in Microbial Biosynthesis of C3 – C5 Diols: Genetics and Process Engineering Approaches. Bioresour. Technol. 322, 124527. doi:10.1016/j.biortech.2020.124527
Xin, D., Blossom, B. M., Lu, X., and Felby, C. (2022). Improving Cellulases Hydrolytic Action: An Expanded Role for Electron Donors of Lytic Polysaccharide Monooxygenases in Cellulose Saccharification. Bioresour. Technol. 346, 126662. doi:10.1016/j.biortech.2021.126662
Zeng, W., Wang, P., Li, N., Li, J., Chen, J., and Zhou, J. (2020). Production of 2-Keto-L-Gulonic Acid by Metabolically Engineered Escherichia coli. Bioresour. Technol. 318, 124069. doi:10.1016/j.biortech.2020.124069
Zhang, Y.-h., Lin, J.-y., Bai, L., Huang, M., Chen, H.-q., Yao, S., et al. (2018). Antioxidant Capacities of Bacillus Endophyticus ST-1 and Ketogulonicigenium Vulgare 25B-1 in Vitamin C Fermentation. Biotechnol. Biotechnol. Equip. 32 (3), 628–637. doi:10.1080/13102818.2018.1447854
Zhao, D., Le, T. T., Larsen, L. B., Li, L., Qin, D., Su, G., et al. (2017). Effect of Glycation Derived from α-dicarbonyl Compounds on the In Vitro Digestibility of β-casein and β-lactoglobulin: A Model Study with Glyoxal, Methylglyoxal and Butanedione. Food Res. Int. 102, 313–322. doi:10.1016/j.foodres.2017.10.002
Zhou, P., Yao, R., Zhang, H., and Bao, J. (2019). Unique Glucose Oxidation Catalysis of Gluconobacter Oxydans Constitutes an Efficient Cellulosic Gluconic Acid Fermentation Free of Inhibitory Compounds Disturbance. Biotechnol. Bioeng. 116 (9), 2191–2199. doi:10.1002/bit.27020
Keywords: detection method, 2,5-diketo-D-gluconic acid, browning, Gluconobacter oxydans ATCC 9937, feed-batch
Citation: Li G, Shan X, Zeng W, Yu S, Zhang G, Chen J and Zhou J (2022) Efficient Production of 2,5-Diketo-D-gluconic Acid by Reducing Browning Levels During Gluconobacter oxydans ATCC 9937 Fermentation. Front. Bioeng. Biotechnol. 10:918277. doi: 10.3389/fbioe.2022.918277
Received: 12 April 2022; Accepted: 20 May 2022;
Published: 08 July 2022.
Edited by:
Tian-Qiong Shi, Nanjing Normal University, ChinaReviewed by:
Zhiqiang Wen, Nanjing Normal University, ChinaQuanyu Zhao, Nanjing Tech University, China
Copyright © 2022 Li, Shan, Zeng, Yu, Zhang, Chen and Zhou. This is an open-access article distributed under the terms of the Creative Commons Attribution License (CC BY). The use, distribution or reproduction in other forums is permitted, provided the original author(s) and the copyright owner(s) are credited and that the original publication in this journal is cited, in accordance with accepted academic practice. No use, distribution or reproduction is permitted which does not comply with these terms.
*Correspondence: Jingwen Zhou, emhvdWp3MTk4MkBqaWFuZ25hbi5lZHUuY24=