- 1School of Chemical Engineering and Technology, Tianjin University, Tianjin, China
- 2Key Laboratory of Systems Bioengineering of the Ministry of Education, Tianjin University, Tianjin, China
- 3SynBio Research Platform, Collaborative Innovation Center of Chemical Science and Engineering, Tianjin, China
Carnosic acid (CA), a phenolic tricyclic diterpene, has many biological effects, including anti-inflammatory, anticancer, antiobesity, and antidiabetic activities. In this study, an efficient biosynthetic pathway was constructed to produce CA in Saccharomyces cerevisiae. First, the CA precursor miltiradiene was synthesized, after which the CA production strain was constructed by integrating the genes encoding cytochrome P450 enzymes (P450s) and cytochrome P450 reductase (CPR) SmCPR. The CA titer was further increased by the coexpression of CYP76AH1 and SmCPR ∼t28SpCytb5 fusion proteins and the overexpression of different catalases to detoxify the hydrogen peroxide (H2O2). Finally, engineering of the endoplasmic reticulum and cofactor supply increased the CA titer to 24.65 mg/L in shake flasks and 75.18 mg/L in 5 L fed-batch fermentation. This study demonstrates that the ability of engineered yeast cells to synthesize CA can be improved through metabolic engineering and synthetic biology strategies, providing a theoretical basis for microbial synthesis of other diterpenoids.
Introduction
Diterpenoids have diverse structures and biological activities (Wang and Weller, 2006). Naturally occurring cyclic diterpenoids, such as tanshinones, paclitaxel, or platensimycin, possess antimicrobial and antitumor activities. Among them, paclitaxel has been extensively used as an anticancer drug (Vranová et al., 2013; Sultan et al., 2021). Ginkgo lactone can be used to treat cardiovascular disease as a natural platelet activation antagonist (Liu et al., 2018) and is also employed to treat Alzheimer’s disease (Sarkar et al., 2020). Carnosic acid (CA) is a phenolic tricyclic diterpene (Mena et al., 2016) that has attracted much interest for its pharmacological properties against obesity, neurodegenerative ailment, and cancer (Barni et al., 2012; Dickmann et al., 2012). At the same time, CA is extensively utilized in many other fields due to its safety, nontoxicity, and high-temperature resistance, including pharmaceuticals, cosmetics, food additives, and spices (Jordán et al., 2012; Ou et al., 2018).
At present, phytoextraction is the primary source of commercial CA, but the content of terpenoids in the plant is low (Liu and Khosla, 2010; Weathers et al., 2011), and the phytoextraction approach is restricted by the supply of plant material (Pickens et al., 2011; Birtic et al., 2015). Plant terpenoids synthesis by microorganisms is an effective method to solve these problems. Saccharomyces cerevisiae has a clear genetic background and is among the most thoroughly studied eukaryotes. In addition, S. cerevisiae can be easily cultured, grows rapidly, and possesses a natural mevalonate acid (MVA) pathway, which makes it convenient for extensive industrial usage (Tholl, 2006). S. cerevisiae is widely used in the microbial synthesis of terpenoids (Paramasivan and Mutturi, 2017), such as the monoterpene alcohol citronellol (Jiang et al., 2021), the sesquiterpene artemisinic acid (Paddon et al., 2013), the diterpenoid paclitaxel precursor taxadiene (Nowrouzi et al., 2020), the triterpenoid ginsenoside compound K (Shi et al., 2021), and the tetraterpenoid carotenoids (Cataldo et al., 2020). CA production and metabolic pathway reconstruction were also accomplished in S. cerevisiae. The biosynthetic route of CA is shown in Figure 1. A copalyl diphosphate synthase (CPS) and a kaurene synthase-like (KSL) enzyme catalyze geranylgeranyl diphosphate (GGPP) into miltiradiene (Gao et al., 2009; Brueckner et al., 2014). Several academics have suggested that the conversion of miltiradiene into abietatriene is a spontaneous oxidation process (Ignea et al., 2016; Scheler et al., 2016). CYP76AH24 catalyzes the oxidation of the labdane skeleton at C-12 and C-11 to produce 11-hydroxy-ferruginol, which is then catalyzed by CYP76AK6 to produce CA in the final steps (Ignea et al., 2016). Furthermore, CYP76AH1 can reportedly directly oxidize miltiradiene and produce ferruginol (Guo et al., 2013). As an essential precursor of CA, the biosynthesis of miltiradiene is of great interest. Dai et al. obtained 365 mg/L miltiradiene by the fusion of SmKSL with SmCPS and ERG20 with BTS1, together with the optimization of the MVA pathway (Dai et al., 2012). Through further screening, fusion, and truncation of diterpene synthases and enhancement of the GGPP supply, the titer of miltiradiene reached approximately 3,500 mg/L (Hu et al., 2020). Ignea and colleagues first constructed an S. cerevisiae platform for CA production. They fused the FPP synthase mutant Erg20p(F96C) with CPP synthase and expressed the HEM3 gene, leading to 1 mg/L CA (Ignea et al., 2016). Then, they adjusted the linker length used for the fusion protein and balanced the co-expression of cytochrome P450 reductase (CPR), cytochrome P450 enzymes (P450s), and cytochrome b5 (Cytb5), leading to 18 mg/L CA (Ignea et al., 2017). In addition, Scheler et al. (2016) expressed GGPP synthase, miltiradiene synthase, CPS, ATR1, and the two P450s CYP76AH1 and CYP76AK8, obtaining 2.74 mg/L CA. However, the CA titer was still meager compared to miltiradiene, which indicates that the rate-limiting step is the conversion of miltiradiene by P450s.
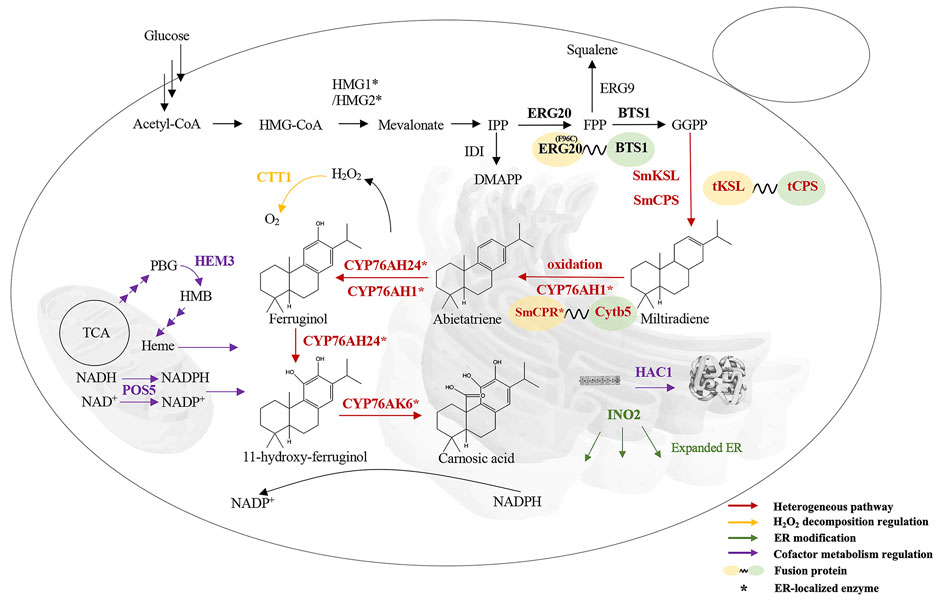
FIGURE 1. Overall strategy of CA production in S. cerevisiae. HMG1/HMG2, HMG-CoA reductase; IDI, isopentenyl pyrophosphate isomerase; ERG20, FPP synthase; ERG9, squalene synthetase; BTS1, GGPP synthase; SmCPS/SmKSL, miltiradiene synthase; CYP76AH24, 11-hydroxy-ferruginol synthase; CYP76AK6, CA synthase; CYP76AH1, ferruginol synthase; HMG-CoA, hydroxymethylglutaryl-CoA; SmCPR, cytochrome P450 reductase; Cytb5, cytochrome B5; CTT1, catalase; ER, endoplasmic reticulum; INO2, transcription factor that promotes phospholipid synthesis; HAC1, transcription factor that promotes protein folding; HEM3, heme synthase; TCA, tricarboxylic acid cycle; POS5, NADH kinase. Three arrows represent multistep reactions.
Researchers have used many engineering strategies targeting P450s to improve terpenoid production. Zhu et al. (2018) boosted the yield of total triterpenoids 5.61-fold by screening CPRs from different plant sources. Our lab fused P450s with CPR and increased the enzyme activity 4.5-fold (Zhao et al., 2016). Our lab overexpressed the endoplasmic reticulum (ER) size regulatory factor INO2 to boost P450s expression in ER. As a result, forskolin titer increased by 2.61 times in S. cerevisiae (Ju et al., 2022). These efforts are significant and helpful for CA pathway optimization. However, when exogenous pathways are introduced into S. cerevisiae, regulation of the rate-limiting step alone is not sufficient to maximize the synthesis of the target product.
In this study, CA was produced in S. cerevisiae by integrating a heterologous biosynthesis pathway, as shown in Figure 1. Further metabolic optimization mainly focused on P450s modules and the endogenous metabolic network boosted the CA titer to 24.65 mg/L in shake flasks and 75.18 mg/L in 5 L fed-batch fermentation.
Materials and Methods
Strains, Reagents, and Culture Media
For the development of all engineered strains, S. cerevisiae 3HP-F was utilized as the initial strain (Zhang et al., 2019). Jinkairui Biotechnology Co., Ltd. (Wuhan, China) codon optimized and synthesized the DNA fragments encoding SmCPS (copalyl diphosphate synthase) (GenBank: EU003997.1), SmKSL (kaurene synthase-like enzyme) (GenBank: EF635966.2), CYP76AH24 (11-hydroxy-ferruginol synthase) (GenBank: KT157044.1), CYP76AK6 (CA synthase) (GenBank: KT157045.1), GuCPR (cytochrome P450 reductase) (GenBank: QCZ35624.1) and AtCPR (GenBank: BT008426.1), and cloned them into the plasmid pUC57. GENEWIZ (Beijing, China) codon optimized and synthesized the DNA fragment encoding SmCPR (GenBank: CBX24555). Yeast transformants were screened on synthetic medium lacking specific amino acids. Standard culture was conducted in yeast extract peptone dextrose medium (YPD), which comprised 2% peptone, 1% yeast extract, and 2% glucose. GENEWIZ (Beijing, China) synthesized the primers. TIANGEN (Beijing, China) provided the mini plasmid extraction and DNA gel mini purification kits. S. cerevisiae W303-1a genomic DNA was used to amplify the promoter, terminator, optional marker, and other native sequences.
Construction and Integration of Yeast Expression Cassettes
We used the lithium acetate method to transform S. cerevisiae as described previously (Gietz and Schiestl, 2007). The expression cassette containing the coding DNA sequence, the promoter and terminator was constructed using fusion polymerase chain reaction (PCR). The expression cassettes and the expression fragments are shown in Supplementary Table S1 and Supplementary Figure S1. The primers, coding DNA sequences, promoter and terminator sequences used in the construction of strains are shown in Supplementary Tables S2–S4.
Cultivation and Fermentation of Yeast
A single colony was inoculated into a tube containing 3 ml of YPD medium and cultivated for 16–18 h at 220 rpm at 30°C. The culture was then inoculated into a shake flask with 30 ml of YPD medium to an initial OD600 of 0.05, then cultivated for 96 h under the same condition. All the experiments in conical flasks were done in triplicate. A conventional spectrophotometer (Oppler, 752 N, China) was used to determine the OD600.
Extraction and Analysis of Metabolites
Miltiradiene, ferruginol, and CA were extracted using n-hexane from lysed cells and the supernatant of the fermentation broth. The fermentation broth was centrifuged at 10000 g for 10 min. The cell precipitate and supernatant were separated into two centrifuge tubes, after which n-hexane was added to both, and additional glass beads were added to the cell precipitate. Both tubes were then shaken vigorously in a vortex shaker for 40 min and centrifuged. The upper organic phases were aspirated with a syringe and combined. Ferruginol and CA standards were purchased from Solarbio (Beijing, China).
Miltiradiene was identified by gas chromatography-mass spectrometry. A Shimadzu GC-MS-TQ8030 apparatus with a GC column HP-5ms (Agilent Technologies, 30 m × 0.250 mm × 0.25 μm) was used to analyze the samples (1 μL). The temperature gradient was as follows: injection temperature, 250°C; 5 min at 150°C, ramp at 5°C/min to 250°C, then hold for 5 min. The spectra were scanned between 30 and 550 m/z with an ion source temperature of 260°C.
Ferruginol and CA were identified by liquid chromatography-mass spectrometry (LC-MS). High-performance liquid chromatography (HPLC) with an Elite P230II high-pressure pump system was used to quantify ferruginol and CA. A Grace Apollo C18 column (4.6 × 250 mm, 5 mm) was used for chromatographic separation. The detection ultraviolet is 230 nm. The LC-MS analysis was carried out using an Agilent Zorbax SB Aq column (100 mm × 2.1 mm × 3 μm) with Surveyor LC System (Thermo Finnigan, San Jose, CA, United States). Negative ionization was used to evaluate the samples. The elution conditions were as follows: the injection volume was 30 μL; the eluent was 40:60 water: acetonitrile; the column temperature was 30°C; the flow rate was 1 ml/min; scanning mode: first-level full scan. For compound identification, we compared the mass spectra and retention times with authentic standards.
A bioanalyzer (SBA-40C, Shandong Academy of Sciences, China) and an Aminex HPX-87H column (Bio-Rad, United States) were used to measure glucose and ethanol concentrations. The flow rate was 0.6 ml/min; the eluent was 5 mM H2SO4; the column temperature was 65°C.
Compound Quantification and Statistical Analysis
The internal standard for miltiradiene quantitation was 1-eicosene. A calibration curve with an R2 coefficient of more than 0.99 (Supplementary Figure S6) was used to quantify the ferruginol and CA in LC analysis. The highest and lowest deviations from three different cultivations were represented by error bars. Univariate analysis (t-test) was used to assess the statistical significance (p-value).
Fluorescence Measurement
A single colony was inoculated into a tube containing 3 ml of YPD medium and cultivated for 16–18 h at 220 rpm at 30°C. The culture was used to inoculate another tube with 3 ml of YPD medium to 0.2 initial OD600, then cultivated at 220 rpm and 30°C for 48 h for fluorescence measurements using Infinite 200 PRO Multimode Microplate Reader (TECAN, Switzerland). All the experiments were done in triplicate.
Quantitative PCR for Gene Copy Number Detection
The number of integrated heterologous expression cassettes was determined using absolute qPCR with Arg4 as an internal reference gene (Abad et al., 2010). We used the primers listed in Supplementary Table S2 to quantify the open reading frames of CYP76AH24, CYP76AK6, CYP76AH1, SmCPR, and ARG4 genes to generate standard curves. Genomic DNA was extracted from distinct colonies and three repeated qPCR assays were conducted using the LightCycler 480 System with TaqMan probe qPCR TB Green Premix Ex Tap II (Vazyme, China). Detection based on the TaqMan probe was described by Zhang et al. (2014).
Fed-Batch Cultivation
The preserved engineered S. cerevisiae strains were streaked onto a YPD plate and activated to obtain a single colony. This single colony was cultured in a test tube containing 3 ml of YPD at 220 rpm and 30°C for 16–18 h, and the resulting seed culture was transferred to 200 ml of YPD in a shake flask and cultured for 16–18 h under the same condition. The secondary seed culture was inoculated 2 L of YPD medium in a 5 L fermenter at a volume ratio of 1:10. The automatic addition of 2.5 M sulfuric acid and 5 M ammonia kept the pH constant at 5.5. The airflow was 2 vvm, the fermentation temperature was 30°C, and the rotation speed was varied between 200 and 600 rpm to maintain the dissolved oxygen (DO) above 35% of the atmospheric value.
The conditions for two-stage fed-batch fermentation were the same as the batch fermentation. When the initial glucose was completely spent, feeding was initiated with a solution containing 500 g/L glucose, 5.12 g/L MgSO4·7H2O, 0.28 g/L Na2SO4, 3.5 g/L K2SO4, 9 g/L KH2PO4, 0.6 g/L uracil, 0.5 g/L adenine, 1.2 g/L lysine, 12 ml vitamin solution and 10 ml trace element solution. As previously reported, the vitamin and trace element solution was prepared (Zhou et al., 2010). When glucose consumption was complete and the ethanol produced in the process of metabolism was consumed, feeding with ethanol (95%, V/V) was initiated and the ethanol concentration in the fermenter was kept at 1–6 g/L in addition to 350 ml of feeding solution components other than glucose was added. The highest and lowest deviations from three different cultivations were represented by error bars.
Results
Reconstructing the Miltiradiene Biosynthesis Pathway in S. cerevisiae
The codon-optimized miltiradiene synthesis genes from Salvia miltiorrhiza (SmCPS and SmKSL) were integrated into S. cerevisiae 3HP-F to form the WM1 strain. The strong promoters PPGK1 and PTDH3 were selected to express these two genes, respectively. Based on published mass spectra, the new peak (retention time (RT) = 17.82 min) was identified as miltiradiene (Hu et al., 2020) (Figure 2). We compared the peak areas of the internal standard 1-eicosene and miltiradiene to quantify a titer of 0.18 mg/L for miltiradiene after 4 days of shake flask culture.
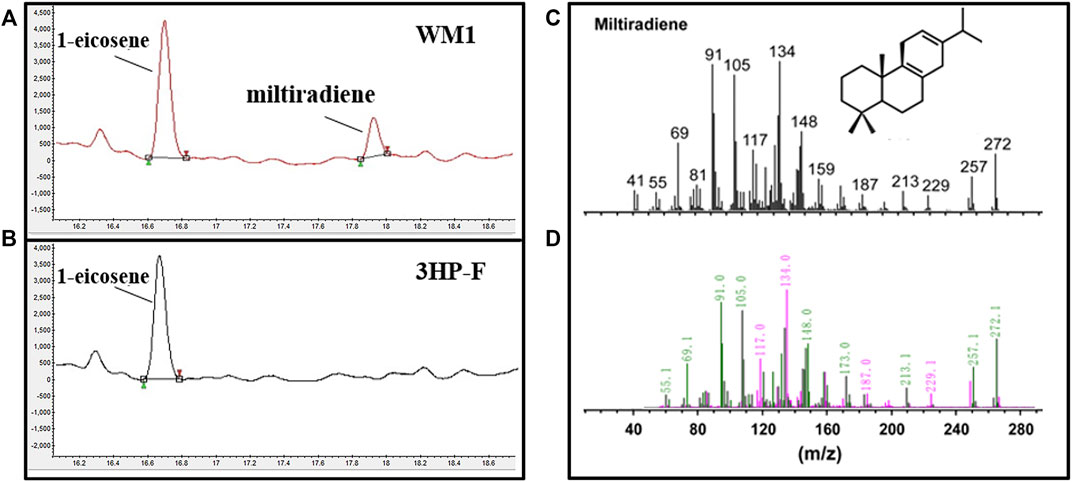
FIGURE 2. Miltiradiene production in S. cerevisiae. (A) Chromatogram of miltiradiene produced by strain WM1; (B) Chromatogram of the original strain 3HP-F; (C) GC–MS spectra of miltiradiene published in the literature (Hu et al., 2020); (D) GC–MS spectra of the chromatographic peak at RT = 17.82 min.
Plant diterpenes are synthesized in plastids, and diterpene synthases usually contain an N-terminal plastidic transit peptide (Bohlmann et al., 1998). However, the transit peptide was reported to hinder the heterologous expression of diterpene synthases (Hu et al., 2020). Here, we identified the transit peptide of miltiradiene synthases using the ChloroP online tool (https://www.cbs.dtu.dk/services/ChloroP/) to construct the truncated variants tSmCPS and tSmKSL. Supplementary Table S3 shows the plastidic transit peptide coding sequences. The strong promoters PPGK1 and PTDH3 were selected to co-express tSmCPS and tSmKSL from the ura3 locus of the strain 3HP-F to form WM2, which produced 1.27 mg/L miltiradiene, representing a 7.06-fold increase over the original strain (Figure 3). Jiang et al. (2017) removed the plastidic transit peptide from the N-terminus of geraniol synthase and fused a red fluorescent protein to its C-terminus to characterize the expression level of the correctly folded protein using fluorescence measurements. To investigate the expression of tSmCPS and tSmKSL, we fused the red fluorescent protein mCherry to the C-terminus of SmCPS, tSmCPS, SmKSL, and tSmKSL and expressed these four fusion proteins in S. cerevisiae. The relative fluorescence (RFU) of tSmCPS and tSmKSL was respectively 2.1 and 2.5 times higher than that of SmCPS and SmKSL, indicating that the expression of both enzymes was enhanced (Supplementary Figure S2).
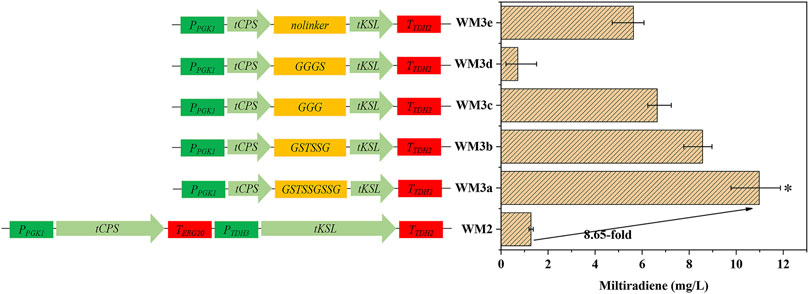
FIGURE 3. Protein fusion and linker selection. WM2: Coexpression of tSmCPS and tSmKSL. WMa–e: Fusion of tSmCPS and tSmKSL using five flexible linkers: GSTSSGSSG, GSTSSG, GGG, GGGS, no linker. *: The product titer of strain WM3a was significantly higher than that of WM3b. *p < 0.05; **p < 0.01; ***p < 0.001. Histograms illustrating the production of corresponding strains. Experiments were performed in triplicate.
Fusion proteins can boost the effective local concentration of substrates and enzymes, improving the product yield. Linker length has a crucial effect on the function of fusion proteins (Haga et al., 2013). For example, extremely long linkers make the fusion proteins prone to degradation and affect the product yield, while extremely short linkers affect the spatial conformation of the protein, which causes it to fail to play its original catalytic role. We fused tSmCPS and tSmKSL using five flexible linkers to obtain strains WM3a-e (Figure 3). The GSTSSGSSG linker had the best effect, and the titer of miltiradiene reached 10.98 mg/L, 8.65 times higher than strain WM2, co-expressing tSmCPS and tSmKSL (Supplementary Figure S3). Overexpression of the BTS1-GGGS-ERG20, a fusion of two critical enzymes of the MVA pathway, can considerably increase miltiradiene production (Zhou et al., 2012). In addition the ERG20(F96C) mutant can considerably enhance GGPP accumulation while having no apparent effect on FPP production (Ignea et al., 2015). Accordingly, BTS1-GGGS-ERG20F96Cp was overexpressed to create the WM4 strain, which reached a miltiradiene titer of 172.77 mg/L (Supplementary Figure S3).
Selection of Cytochrome P450 Reductases for Cytochrome P450 Enzymes (P450s) to Produce Carnosic Acid
P450s are frequently utilized in the synthesis of drugs, vitamins, and spices (Ignea et al., 2015). However, few plant P450s exhibited high activity, and have been optimized using protein engineering, metabolic engineering, redox chaperone engineering, and substrate engineering approaches to increase terpene production (Xiao et al., 2019). Thus, choosing an appropriate functional cytochrome P450 reductase (CPR) is essential for maximizing the redox coupling efficiency.
Three distinct CPR encoding genes were chosen for co-expression with P450s CYP76AH24 and CYP76AK6 (from S. miltiorrhiza) in WM4, resulting in strains WCA1a (AtCPR from Arabidopsis thaliana), WCA1b (GuCPR from Glycyrrhiza uralensis), and WCA1c (SmCPR from S. miltiorrhiza). The processed samples were analyzed using LC–MS after 4 days of culture. As shown in Supplementary Figure S4, CA and ferruginol were identified as new peaks by LC–MS analysis. WCA1c containing SmCPR produced 52.5 μg/L of CA, 70% more than WCA1a containing AtCPR and 30% more than strain WCA1b containing GuCPR (Figure 4A). A high coupling efficiency and electron transfer compatibility were reported in the homologous CYP-CPR reconstituted system, which boosted the monooxygenase activity (Dietrich et al., 2005; Jensen and Møller, 2010), and was consistent with our findings.
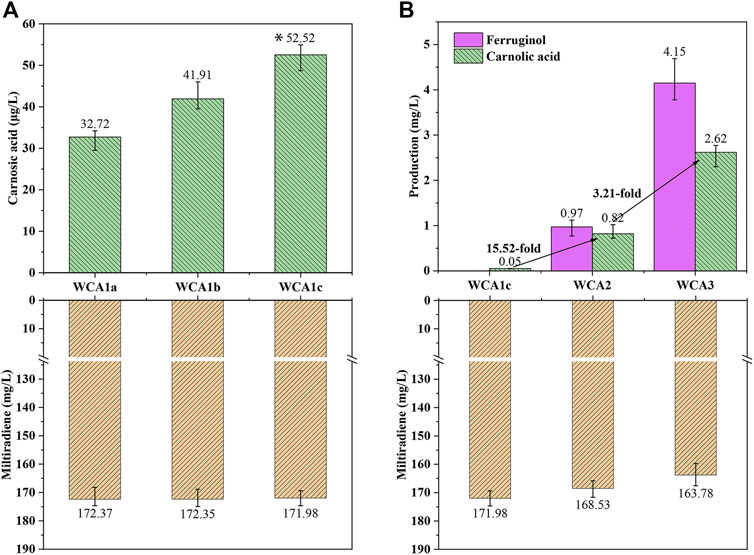
FIGURE 4. CA production in S. cerevisiae increased in a stepwise manner. (A) Production of CA and miltiradiene by strains WCA1a–c. *: The CA titer of strain WCA1c is significantly higher than that of WCA1b. *p < 0.05; **p < 0.01; ***p < 0.001 (B) Production of miltiradiene, ferruginol and CA by strain WCA2-3. The data are the averages of three separate experiments.
The conversion rate of the precursor miltiradiene was low, and may be attributed to the low efficiency of spontaneous oxidation of miltiradiene into abietatriene. By contrast, CYP76AH1 can reportedly directly oxidize miltiradiene to produce ferruginol (Guo et al., 2013). To further improve the CA titer, we overexpressed CYP76AH1 to develop the WCA2 strain. The ferruginol and CA titers reached 0.97 and 0.81 mg/L, indicating that CYP76AH expression could increase the conversion of miltiradiene into ferruginol (Figure 4B).
Cytochrome B5 (Cytb5) acts as an electron transporter in many biological oxidation reactions (Zhang et al., 2007). It appears to act as a specific electron donor when involved in catalysis together with NADPH-cytochrome B5 reductase or NADPH-CPR (Gilep et al., 2001). Therefore, we overexpressed Cytb5 from S. pomifera to develop the WCA3 strain with a CA titer of 2.62 mg/L, which was 3.21 times higher than in WCA2. Furthermore, the ferruginol titer also increased 4.27 times, reaching 4.15 mg/L (Figure 4B).
Optimization of Carnosic Acid Production by the Fusion of Cytochrome P450 Monooxygenase and Cytochrome P450 Reductases
The fusion of CPR with a compatible P450 enzyme could reportedly result in evolutionary advantages in terms of catalytic efficiency (McLean et al., 2007), and a fusion of the P450s CYP3A4, NADPH-CPR, and Cytb5 showed high activity (Inui et al., 2007). Therefore, we fused CYP76AH1, SmCPR, and SpCytb5 to explore the optimal fusion strategy for CA production. In our previous study, protopanaxadiol synthase and a truncated variant of ATR1 without its N-terminal transmembrane region were used to construct a fusion protein with higher activity (Zhao et al., 2016). Here, we used the TMHMM server to predict the transmembrane regions of SmCPR and SpCytb5, as shown in Supplementary Table S3. First, the truncated SmCPR was fused with CYP76AH1 and co-expressed with native SpCytb5 to construct the WCA4a strain. However, the results showed a sharp decline in CA production relative to the WCA3 strain (Figures 5A,E). We then fused the truncated SpCytb5 with CYP76AH1 and SmCPR, respectively, and then co-expressed it with native SmCPR or CYP76AH1, resulting in the strains WCA4b and WCA4c. The results showed that the CA titer of WCA4b and WCA4c was improved compared with WCA3 (Figures 5B,C,E), whereby the product titer of the best strain WCA4c reached 3.17 mg/L (Figure 5C), indicating that the fusion of truncated SpCytb5 and SmCPR improved the electron transport efficiency. Finally, we fused the truncated SmCPR, truncated SpCytb5, and CYP76AH1 to construct the WCA4d strain. However, its CA titer was unsatisfactory (Figure 5D). According to these results, we found that after excising the transmembrane region of SmCPR protein, the CA titer was significantly reduced regardless of the fusion method (Figure 5). In addition, we detected carnosol, a spontaneous oxidation product of CA, in WCA4c, which is in line with earlier research (Scheler et al., 2016). Because the product titer of strain WCA4c showed the largest increase, we chose the coexpression of SmCPR∼t28SpCytb5 fusion protein and CYP76AH1 for further experiments.
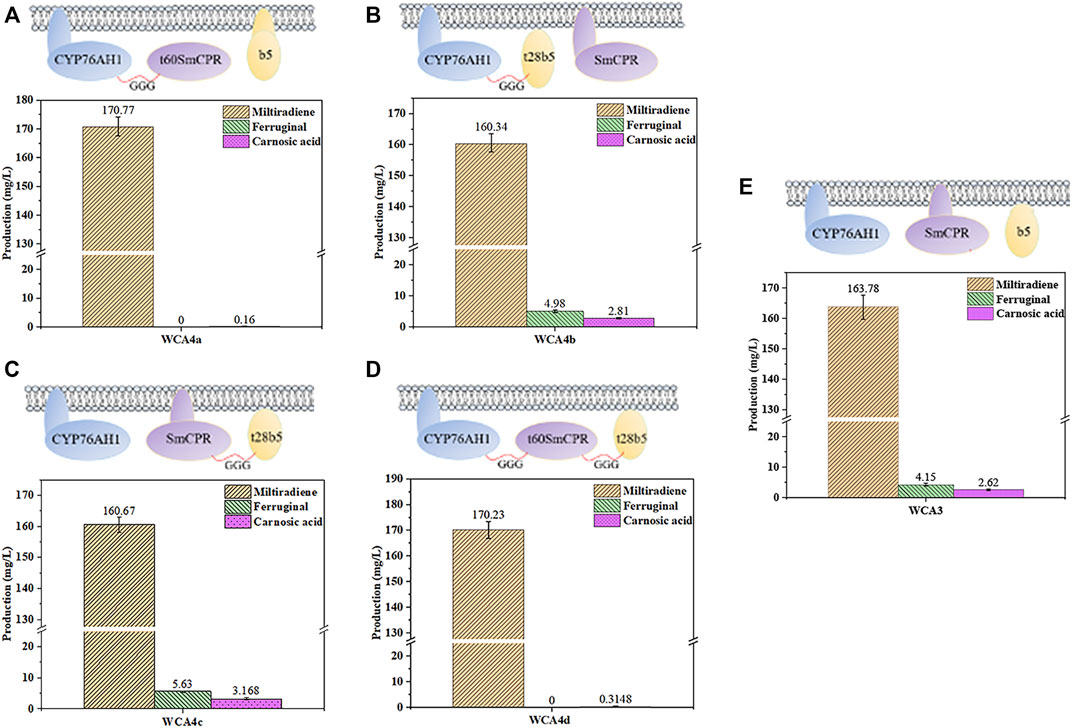
FIGURE 5. Influence of different fusion strategies on miltiradiene, ferruginol and CA production. (A) WCA4a: CYP76AH1 ∼ t60SmCPR, SpCytb5; (B) WCA4b: SmCPR, CYP76AH1 ∼ t28SpCytb5; (C) WCA4c: SmCPR ∼ t28SpCytb5, CYP76AH1; (D) WCA4d: CYP76AH1 ∼ t60SmCPR ∼ t28SpCytb5. (E) WCA3: CYP76AH1, SmCPR and SpCytb5 in the natural state. The data are the averages of three separate experiments.
To further improve CA production, we chose a multicopy site to integrate the expression cassettes encoding the CA synthesis pathway. First, WCA4c was used as the chassis strain, and the SmCPR∼t28SpCytb5 fusion gene and CYP76AH1 were co-expressed from the δ site to obtain the WCA5 strain with CA and ferruginol titers of 4.30 and 12.74 mg/L, respectively. Then, we integrated the CYP76AH24 and CYP76AK6 genes into the ribosomal DNA site, resulting in the WCA6 strain with the highest CA titer of 6.20 mg/L. The gene copy number of strain WCA6 is shown in Supplementary Figure S5.
Expression of Catalase-Related Genes in S. cerevisiae
The normal aerobic metabolism of S. cerevisiae is accompanied by the generation of reactive oxygen species (Temple et al., 2005). In the heterologous synthesis of terpene products in microorganisms, P450s and their reductases are sometimes poorly coupled, resulting in the generation of reactive oxygen species (Paddon et al., 2013). In the CA production strain, we expressed three heterologous P450s, which may have caused excessive accumulation of reactive oxygen species. H2O2 is accumulated due to ATP synthesis and spontaneous or enzymatic hydrolysis of O2 during respiratory metabolism, which can cause damage to the cell (Temple et al., 2005). Catalase is a primary H2O2 scavenging enzyme essential for preserving intracellular homeostasis of reactive oxygen species. In S. cerevisiae, the ScCTA1 catalase is localized to peroxisomes and mitochondria, where it degrades H2O2 produced during aerobic respiration and β-oxidation (Dzanaeva et al., 2020). The second ScCTT1 catalase is localized in the cytoplasm and responds to oxidative stress caused by H2O2 accumulation (Martins et al., 2019).
As shown in Figure 6A, we expressed ScCTA1 from the MET17 locus of WCA6 to construct the WCA7a strain. Overexpression of ScCTA1 increased CA production to 7.57 mg/L and ferruginol production to 11.38 mg/L. Next, we expressed ScCTT1 from the MET17 locus of WCA6 to construct the WCA7b strain, which exhibited increases of CA and ferruginol production to 8.84 mg/L and 14.64 mg/L. However, when the two genes were simultaneously overexpressed, CA production was reduced. Therefore, the WCA7b strain was selected for subsequent experiments.
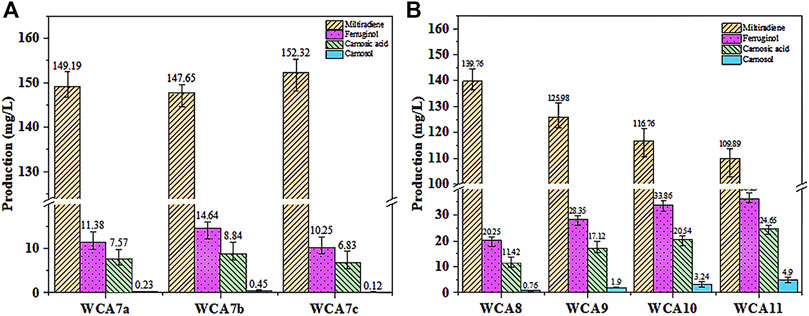
FIGURE 6. CA production in S. cerevisiae increased in a stepwise manner. (A) Effect of the overexpression of ScCTA1 and ScCTT1 catalases on the product titer. (B) Fermentation was conducted in YPD medium. WCA8, INO2 gene overexpressed; WCA9, HEM3 gene overexpressed; WCA10, POS5 gene overexpressed; WCA11, HAC1 gene overexpressed. The data are the averages of three separate experiments.
Effect of Endoplasmic Reticulum Modification and Cofactor Metabolism Regulation on Carnosic Acid Synthesis
The subcellular organelles of eukaryotes have different functions, providing a unique physicochemical environment and critical functions for cell survival. For instance, the Endoplasmic Reticulum (ER) offers a microenvironment designed for rapid and precise protein processing (Malhotra et al., 2008). Accordingly, the ER volume is a crucial determinant of the protein folding capacity of cells (Schuck et al., 2009). The ER membrane is mainly constituted by phospholipids, and the transcription factor INO2 can activate the expression of related genes to promote phospholipid synthesis, thus increasing the area of the ER membrane. In a recent study, overexpression of INO2 in yeast expanded the ER area, leading to a 71-fold increase in squalene production (Kim et al., 2019). As shown in Figure 6B, the INO2 overexpression in strain WCA8 boosted the CA titer by 29%, reaching 11.42 mg/L, and the ferruginol titer was 20.25 mg/L.
Heme is the main cofactor of P450s, and modifying the endogenous heme synthesis pathway was reported as a viable approach to enhance the activity of P450s (Michener et al., 2012). We overexpressed the HEM3 (heme synthase) gene in the WCA9 strain, which increased the CA titer by 50% to 17.12 mg/L. To increase the NADPH supply, we overexpressed the NADH kinase gene (POS5) (Miyagi et al., 2009) in WCA10, which increased the CA titer by 20%–20.54 mg/L, whereby the ferruginol titer also increased to 33.86 mg/L (Figure 6B). The transcription factor HAC1 can activate the transcription of proteins folding-related genes, activating the unfolded protein response (UPR) in the ER (Khan and Schroeder, 2008; Jonikas et al., 2009; Qu et al., 2020). To reduce the pressure caused by the expansion of the ER, we overexpressed the transcription factor HAC1 in WCA11, which increased the CA and ferruginol titers to 24.65 and 36.29 mg/L, corresponding to yields of 1.23 and 1.81 mg/g, respectively (Figure 6B).
Carnosic Acid Production in a 5-L Bioreactor
We performed batch and fed-batch fermentation of the best strain WCA11 in a 5-L bioreactor to confirm the shake-flasks results. The residual glucose concentration, cell growth, ethanol concentration, and CA titer were evaluated during batch fermentation. Glucose was rapidly consumed within 12 h at the beginning of the fermentation (Figure 7A). At the same time, ethanol accumulation reached up to 11.93 g/L, after which ethanol was the carbon source and consumed after 48 h. CA production was detected every 12 h from the beginning of the fermentation, and reached a peak of 31.04 mg/L at 96 h. The titer of ferruginol and miltiradiene reached 54.80 and 212.45 mg/L, respectively. The final OD600 of the strain reached 29.23 (Figure 7A).
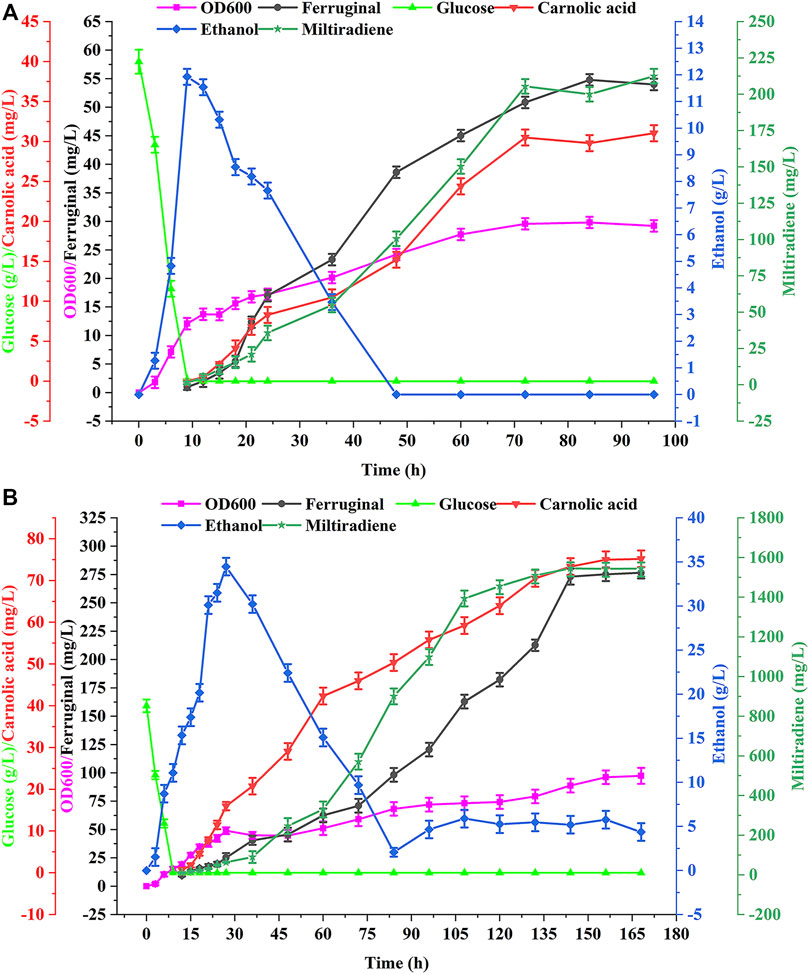
FIGURE 7. Production of CA in batch and fed-batch fermentations in a 5 L bioreactor. (A) Batch fermentation in a 5 L bioreactor using strain WCA11. The fermentation was conducted in YPD medium with 40 g/L glucose. (B) WCA11 fed-batch fermentation in a 5 L bioreactor. The data are the averages of three separate experiments.
As shown in Figure 7B, the OD600 of WCA11 was 3.33-fold higher in fed-batch fermentation than in batch fermentation. The CA titer also increased considerably, reaching 75.18 mg/L, representing a 142.2% increase compared with batch fermentation, which is the highest CA titer reported in yeast to date, to our knowledge, (Table 1). The ferruginol and miltiradiene titers also increased to 276.58 and 1,543.27 mg/L, respectively. The two-stage feeding strategy therefore showed good results (Figure 7B).
Discussion
Diterpenoids have diverse structures and notable biological activities. However, the yield of traditional plant extraction is generally poor since the relevant compounds are present in low quantities as secondary metabolites. The phenolic tricyclic diterpene CA has valuable pharmacological and biological activities. Recently, many studies have been devoted to analyzing the synthesis pathway of CA. However, there are few studies on combinatorial engineering strategies to produce CA, and its high-level production in microorganisms is still challenging.
The spontaneous oxidation of miltiradiene into abietatriene is the subject of controversy. The CYP76AH24 enzyme reportedly catalyzes the oxidation of the labdane skeleton at C-12 and C-11 to produce 11-hydroxy-ferruginol (Ignea et al., 2016). CYP76AH1 can directly convert miltiradiene into abietatriene (Guo et al., 2013). In our study, the co-expression of CYP76AH24 and CYP76AH1 boosted the output of CA by more than 15 times compared with CYP76AH24 alone, indicating that spontaneous oxidation is a rate-limiting step to some extent. It is also possible that CYP76AH24 alone is less efficient than in the presence of CYP76AH1. Indeed, CYP76AH24 would have to introduce two hydroxyl groups, whereas CYP76AH1 introduces only one hydroxyl group, and its product can then serve as a substrate for CYP76AH24. However, because we could not source an authentic reference standard of 11-hydroxy-ferruginol, its production could not be confirmed. Although we attempted to identify 11-hydroxy-ferruginol among the new LC-MS peaks and found that the mass spectrum of a peak was similar to a published mass spectrum of 11-hydroxy-ferruginol, because there was no standard, a definitive confirmation could not be obtained (Supplementary Figure S7). We also detected several peaks, which may have been degradation products of 11-hydroxy-ferruginol or other by-products.
Overexpression of cytochrome B5 (Cytb5) increased the production of artemisinin (Paddon et al., 2013). In our study, the expression of Cytb5 from S. pomifera caused a greater than 3-fold increase in CA production, proving that Cytb5 could effectively improve the catalytic efficiency of P450 enzymes. Multicopy integration is a common strategy in microbial biosynthesis of natural products (Zhang et al., 2018). After applying the multicopy integration strategy, CA production was nearly doubled in this study. Reasonable regulation of the supply of cofactors can increase metabolic fluxes and promote product accumulation (Wang et al., 2017). The cofactor regulation strategy used in this study increased CA production more than 2-fold.
The combined engineering strategy used in this study gradually increased the production of CA (Table 2). During this process, the output of the intermediate product miltiradiene gradually decreased (Supplementary Table S5), but the remaining titer was still 109.89 mg/L. Therefore, engineering CYP76AH1, or mining other enzymes with higher activities may be an effective way to increase the production of CA. Recently, researchers used protein engineering to functionally optimize CYP76AH1, and they designed a mutant that catalyzes the highly efficient production of 11-hydroxy-ferruginol in yeast (Mao et al., 2020). Based on the findings of this study, this strategy may lead to a substantial increase of CA production. Additionally, with the gradual increase of CA production, the production of intermediate ferruginol also gradually increased (Supplementary Table S5). The titer of ferruginol increased to 276.58 mg/L in fed-batch fermentation. In comparison, the yield of CA was 75.18 mg/L, which suggests that the low conversion rate of the intermediate ferruginol is a critical problem that limits the final CA yield. Exploring a more optimal fed-batch fermentation strategy may improve the yield of the product CA. Furthermore, Engineering CYP76AH24 and CYP76AK6 to improve their catalytic activities may be a practical solution. Finally, based on the findings of this study related to fusion protein design and signal peptide truncation, the selection of linkers and truncations can still be further studied to obtain a higher CA titer.
In summary, we described a combined engineering strategy to gradually increase the CA output. As a result, the CA titer reached 24.65 mg/L in shake flasks and 75.18 mg/L in 5 L fed-batch fermentation. This engineering strategy has reference value for improving the production of other diterpenoids in microbial cell factories.
Data Availability Statement
The original contributions presented in the study are included in the article/Supplementary Material, further inquiries can be directed to the corresponding author.
Author Contributions
All the authors provided a significant, direct, and intellectual contribution to the work and have provided permission for it to be published. PW and XB performed all experiments, analyzed the data, and wrote the manuscript. CZ, PW, and XB designed the experiments. WL, CZ, and PW conceived the idea. WL modified the manuscript.
Funding
This work was financially supported by the National Key Research and Development Program of China (2020YFA0907900 and 2019YFA0905100).
Conflict of Interest
The authors declare that the research was conducted in the absence of any commercial or financial relationships that could be construed as a potential conflict of interest.
Publisher’s Note
All claims expressed in this article are solely those of the authors and do not necessarily represent those of their affiliated organizations, or those of the publisher, the editors and the reviewers. Any product that may be evaluated in this article, or claim that may be made by its manufacturer, is not guaranteed or endorsed by the publisher.
Supplementary Material
The Supplementary Material for this article can be found online at: https://www.frontiersin.org/articles/10.3389/fbioe.2022.916605/full#supplementary-material
References
Abad, S., Kitz, K., Hörmann, A., Schreiner, U., Hartner, F. S., and Glieder, A. (2010). Real-time PCR-Based Determination of Gene Copy Numbers inPichia Pastoris. Biotechnol. J. 5, 413–420. doi:10.1002/biot.200900233
Barni, M. V., Carlini, M. J., Cafferata, E. G., Puricelli, L., and Moreno, S. (2012). Carnosic Acid Inhibits the Proliferation and Migration Capacity of Human Colorectal Cancer Cells. Oncol. Rep. 27, 1041–1048. doi:10.3892/or.2012.1630
Birtić, S., Dussort, P., Pierre, F.-X., Bily, A. C., and Roller, M. (2015). Carnosic Acid. Phytochemistry 115, 9–19. doi:10.1016/j.phytochem.2014.12.026
Bohlmann, J., Meyer-Gauen, G., and Croteau, R. (1998). Plant Terpenoid Synthases: Molecular Biology and Phylogenetic Analysis. Proc. Natl. Acad. Sci. U.S.A. 95, 4126–4133. doi:10.1073/pnas.95.8.4126
Brückner, K., Božić, D., Manzano, D., Papaefthimiou, D., Pateraki, I., Scheler, U., et al. (2014). Characterization of Two Genes for the Biosynthesis of Abietane-type Diterpenes in Rosemary (Rosmarinus Officinalis) Glandular Trichomes. Phytochemistry 101, 52–64. doi:10.1016/j.phytochem.2014.01.021
Cataldo, V. F., Arenas, N., Salgado, V., Camilo, C., Ibáñez, F., and Agosin, E. (2020). Heterologous Production of the Epoxycarotenoid Violaxanthin in Saccharomyces cerevisiae. Metab. Eng. 59, 53–63. doi:10.1016/j.ymben.2020.01.006
Dai, Z., Liu, Y., Huang, L., and Zhang, X. (2012). Production of Miltiradiene by Metabolically engineeredSaccharomyces Cerevisiae. Biotechnol. Bioeng. 109, 2845–2853. doi:10.1002/bit.24547
Dickmann, L. J., Vandenbrink, B. M., and Lin, Y. S. (2012). In Vitro hepatotoxicity and Cytochrome P450 Induction and Inhibition Characteristics of Carnosic Acid, a Dietary Supplement with Antiadipogenic Properties. Drug Metab. Dispos. 40, 1263–1267. doi:10.1124/dmd.112.044909
Dietrich, M., Grundmann, L., Kurr, K., Valinotto, L., Saussele, T., Schmid, R. D., et al. (2005). Recombinant Production of Human Microsomal Cytochrome P450 2D6 in the Methylotrophic Yeast Pichia pastoris. Chembiochem 6, 2014–2022. doi:10.1002/cbic.200500200
Dzanaeva, L., Kruk, B., Ruchala, J., Nielsen, J., Sibirny, A., and Dmytruk, K. (2020). The Role of Peroxisomes in Xylose Alcoholic Fermentation in the Engineered Saccharomyces cerevisiae. Cell. Biol. Int. 44, 1606–1615. doi:10.1002/cbin.11353
Gao, W., Hillwig, M. L., Huang, L., Cui, G., Wang, X., Kong, J., et al. (2009). A Functional Genomics Approach to Tanshinone Biosynthesis Provides Stereochemical Insights. Org. Lett. 11, 5170–5173. doi:10.1021/ol902051v
Gietz, R. D., and Schiestl, R. H. (2007). High-efficiency Yeast Transformation Using the LiAc/SS Carrier DNA/PEG Method. Nat. Protoc. 2, 31–34. doi:10.1038/nprot.2007.13
Gilep, A. A., Guryev, O. L., Usanov, S. A., and Estabrook, R. W. (2001). Reconstitution of the Enzymatic Activities of Cytochrome P450s Using Recombinant Flavocytochromes Containing Rat Cytochrome B5 Fused to NADPH-Cytochrome P450 Reductase with Various Membrane-Binding Segments. Archives Biochem. Biophysics 390, 215–221. doi:10.1006/abbi.2001.2372
Guo, J., Zhou, Y. J., Hillwig, M. L., Shen, Y., Yang, L., Wang, Y., et al. (2013). CYP76AH1 Catalyzes Turnover of Miltiradiene in Tanshinones Biosynthesis and Enables Heterologous Production of Ferruginol in Yeasts. Proc. Natl. Acad. Sci. U.S.A. 110, 12108–12113. doi:10.1073/pnas.1218061110
Haga, T., Hirakawa, H., and Nagamune, T. (2013). Fine Tuning of Spatial Arrangement of Enzymes in a PCNA-Mediated Multienzyme Complex Using a Rigid Poly-L-Proline Linker. Plos One 8, e75114. doi:10.1371/journal.pone.0075114
Hu, T., Zhou, J., Tong, Y., Su, P., Li, X., Liu, Y., et al. (2020). Engineering Chimeric Diterpene Synthases and Isoprenoid Biosynthetic Pathways Enables High-Level Production of Miltiradiene in Yeast. Metab. Eng. 60, 87–96. doi:10.1016/j.ymben.2020.03.011
Ignea, C., Athanasakoglou, A., Andreadelli, A., Apostolaki, M., Iakovides, M., Stephanou, E. G., et al. (2017). Overcoming the Plasticity of Plant Specialized Metabolism for Selective Diterpene Production in Yeast. Sci. Rep. 7, 8855. doi:10.1038/s41598-017-09592-5
Ignea, C., Athanasakoglou, A., Ioannou, E., Georgantea, P., Trikka, F. A., Loupassaki, S., et al. (2016). Carnosic Acid Biosynthesis Elucidated by a Synthetic Biology Platform. Proc. Natl. Acad. Sci. U.S.A. 113, 3681–3686. doi:10.1073/pnas.1523787113
Ignea, C., Trikka, F. A., Nikolaidis, A. K., Georgantea, P., Ioannou, E., Loupassaki, S., et al. (2015). Efficient Diterpene Production in Yeast by Engineering Erg20p into a Geranylgeranyl Diphosphate Synthase. Metab. Eng. 27, 65–75. doi:10.1016/j.ymben.2014.10.008
Inui, H., Maeda, A., and Ohkawa, H. (2007). Molecular Characterization of Specifically Active Recombinant Fused Enzymes Consisting of CYP3A4, NADPH-Cytochrome P450 Oxidoreductase, and Cytochrome B5. Biochemistry 46, 10213–10221. doi:10.1021/bi700164q
Jensen, K., and Møller, B. L. (2010). Plant NADPH-Cytochrome P450 Oxidoreductases. Phytochemistry 71, 132–141. doi:10.1016/j.phytochem.2009.10.017
Jiang, G.-Z., Yao, M.-D., Wang, Y., Zhou, L., Song, T.-Q., Liu, H., et al. (2017). Manipulation of GES and ERG20 for Geraniol Overproduction in Saccharomyces cerevisiae. Metab. Eng. 41, 57–66. doi:10.1016/j.ymben.2017.03.005
Jiang, G., Yao, M., Wang, Y., Xiao, W., and Yuan, Y. (2021). A "Push-Pull-Restrain" Strategy to Improve Citronellol Production in Saccharomyces cerevisiae. Metab. Eng. 66, 51–59. doi:10.1016/j.ymben.2021.03.019
Jonikas, M. C., Collins, S. R., Denic, V., Oh, E., Quan, E. M., Schmid, V., et al. (2009). Comprehensive Characterization of Genes Required for Protein Folding in the Endoplasmic Reticulum. Science 323, 1693–1697. doi:10.1126/science.1167983
Jordán, M. J., Lax, V., Rota, M. C., Lorán, S., and Sotomayor, J. A. (2012). Relevance of Carnosic Acid, Carnosol, and Rosmarinic Acid Concentrations in the In Vitro Antioxidant and Antimicrobial Activities of Rosmarinus Officinalis (L.) Methanolic Extracts. J. Agric. Food Chem. 60, 9603–9608. doi:10.1021/jf302881t
Ju, H., Zhang, C., He, S., Nan, W., and Lu, W. (2022). Construction and Optimization of Saccharomyces cerevisiae for Synthesizing Forskolin. Appl. Microbiol. Biotechnol. 106, 1933–1944. doi:10.1007/s00253-022-11819-z
Khan, S. U., and Schröder, M. (2008). Engineering of Chaperone Systems and of the Unfolded Protein Response. Cytotechnology 57, 207–231. doi:10.1007/s10616-008-9157-9
Kim, J.-E., Jang, I.-S., Son, S.-H., Ko, Y.-J., Cho, B.-K., Kim, S. C., et al. (2019). Tailoring the Saccharomyces cerevisiae Endoplasmic Reticulum for Functional Assembly of Terpene Synthesis Pathway. Metab. Eng. 56, 50–59. doi:10.1016/j.ymben.2019.08.013
Liu, T., and Khosla, C. (2010). A Balancing Act for Taxol Precursor Pathways in E. coli. Science 330, 44–45. doi:10.1126/science.1195014
Liu, X.-w., Yang, J.-l., Niu, W., Jia, W.-w., Olaleye, O. E., Wen, Q., et al. (2018). Human Pharmacokinetics of Ginkgo Terpene Lactones and Impact of Carboxylation in Blood on Their Platelet-Activating Factor Antagonistic Activity. Acta Pharmacol. Sin. 39, 1935–1946. doi:10.1038/s41401-018-0086-7
Malhotra, J. D., Miao, H., Zhang, K., Wolfson, A., Pennathur, S., Pipe, S. W., et al. (2008). Antioxidants Reduce Endoplasmic Reticulum Stress and Improve Protein Secretion. Proc. Natl. Acad. Sci. U.S.A. 105, 18525–18530. doi:10.1073/pnas.0809677105
Mao, Y., Ma, Y., Chen, T., Ma, X., Xu, Y., Bu, J., et al. (2020). Functional Integration of Two CYP450 Genes Involved in Biosynthesis of Tanshinones for Improved Diterpenoid Production by Synthetic Biology. ACS Synth. Biol. 9, 1763–1770. doi:10.1021/acssynbio.0c00136
Martins, D., Nguyen, D., and English, A. M. (2019). Ctt1 Catalase Activity Potentiates Antifungal Azoles in the Emerging Opportunistic Pathogen Saccharomyces cerevisiae. Sci. Rep. 9, 9185. doi:10.1038/s41598-019-45070-w
McLean, K. J., Girvan, H. M., and Munro, A. W. (2007). Cytochrome P450/redox Partner Fusion Enzymes: Biotechnological and Toxicological Prospects. Expert Opin. Drug Metabolism Toxicol. 3, 847–863. doi:10.1517/17425255.3.6.847
Mena, P., Cirlini, M., Tassotti, M., Herrlinger, K., Dall’Asta, C., and Del Rio, D. (2016). Phytochemical Profiling of Flavonoids, Phenolic Acids, Terpenoids, and Volatile Fraction of a Rosemary (Rosmarinus Officinalis L.) Extract. Molecules 21, 1576. doi:10.3390/molecules21111576
Michener, J. K., Nielsen, J., and Smolke, C. D. (2012). Identification and Treatment of Heme Depletion Attributed to Overexpression of a Lineage of Evolved P450 Monooxygenases. Proc. Natl. Acad. Sci. U.S.A. 109, 19504–19509. doi:10.1073/pnas.1212287109
Miyagi, H., Kawai, S., and Murata, K. (2009). Two Sources of Mitochondrial NADPH in the Yeast Saccharomyces cerevisiae. J. Biol. Chem. 284, 7553–7560. doi:10.1074/jbc.M804100200
Nowrouzi, B., Li, R. A., Walls, L. E., d’Espaux, L., Malcı, K., Liang, L., et al. (2020). Enhanced Production of Taxadiene in Saccharomyces cerevisiae. Microb. Cell. Fact. 19, 200. doi:10.1186/s12934-020-01458-2
Ou, J., Huang, J., Zhao, D., Du, B., and Wang, M. (2018). Protective Effect of Rosmarinic Acid and Carnosic Acid against Streptozotocin-Induced Oxidation, Glycation, Inflammation and Microbiota Imbalance in Diabetic Rats. Food Funct. 9, 851–860. doi:10.1039/c7fo01508a
Paddon, C. J., Westfall, P. J., Pitera, D. J., Benjamin, K., Fisher, K., McPhee, D., et al. (2013). High-level Semi-synthetic Production of the Potent Antimalarial Artemisinin. Nature 496, 528–532. doi:10.1038/nature12051
Paramasivan, K., and Mutturi, S. (2017). Progress in Terpene Synthesis Strategies through Engineering of Saccharomyces cerevisiae. Crit. Rev. Biotechnol. 37, 974–989. doi:10.1080/07388551.2017.1299679
Pickens, L. B., Tang, Y., and Chooi, Y.-H. (2011). Metabolic Engineering for the Production of Natural Products. Annu. Rev. Chem. Biomol. Eng. 2, 211–236. doi:10.1146/annurev-chembioeng-061010-114209
Qu, Z., Zhang, L., Zhu, S., Yuan, W., Hang, J., Yin, D., et al. (2020). Overexpression of the Transcription Factor HAC1 Improves Nerolidol Production in Engineered Yeast. Enzyme Microb. Technol. 134, 109485. doi:10.1016/j.enzmictec.2019.109485
Sarkar, C., Quispe, C., Jamaddar, S., Hossain, R., Ray, P., Mondal, M., et al. (2020). Therapeutic Promises of Ginkgolide A: A Literature-Based Review. Biomed. Pharmacother. 132, 110908. doi:10.1016/j.biopha.2020.110908
Scheler, U., Brandt, W., Porzel, A., Rothe, K., Manzano, D., Božić, D., et al. (2016). Elucidation of the Biosynthesis of Carnosic Acid and its Reconstitution in Yeast. Nat. Commun. 7, 12942. doi:10.1038/ncomms12942
Schuck, S., Prinz, W. A., Thorn, K. S., Voss, C., and Walter, P. (2009). Membrane Expansion Alleviates Endoplasmic Reticulum Stress Independently of the Unfolded Protein Response. J. Cell. Biol. 187, 525–536. doi:10.1083/jcb.200907074
Shi, Y., Wang, D., Li, R., Huang, L., Dai, Z., and Zhang, X. (2021). Engineering Yeast Subcellular Compartments for Increased Production of the Lipophilic Natural Products Ginsenosides. Metab. Eng. 67, 104–111. doi:10.1016/j.ymben.2021.06.002
Sultan, M., Nearing, J. T., Brown, J. M., Huynh, T. T., Cruickshank, B. M., Lamoureaux, E., et al. (2021). An In Vivo Genome‐wide shRNA Screen Identifies BCL6 as a Targetable Biomarker of Paclitaxel Resistance in Breast Cancer. Mol. Oncol. 15, 2046–2064. doi:10.1002/1878-0261.12964
Temple, M. D., Perrone, G. G., and Dawes, I. W. (2005). Complex Cellular Responses to Reactive Oxygen Species. Trends Cell. Biol. 15, 319–326. doi:10.1016/j.tcb.2005.04.003
Tholl, D. (2006). Terpene Synthases and the Regulation, Diversity and Biological Roles of Terpene Metabolism. Curr. Opin. Plant Biol. 9, 297–304. doi:10.1016/j.pbi.2006.03.014
Vranová, E., Coman, D., and Gruissem, W. (2013). Network Analysis of the MVA and MEP Pathways for Isoprenoid Synthesis. Annu. Rev. Plant Biol. 64, 665–700. doi:10.1146/annurev-arplant-050312-120116
Wang, L., and Weller, C. L. (2006). Recent Advances in Extraction of Nutraceuticals from Plants. Trends Food Sci. Technol. 17, 300–312. doi:10.1016/j.tifs.2005.12.004
Wang, M., Chen, B., Fang, Y., and Tan, T. (2017). Cofactor Engineering for More Efficient Production of Chemicals and Biofuels. Biotechnol. Adv. 35, 1032–1039. doi:10.1016/j.biotechadv.2017.09.008
Weathers, P. J., Arsenault, P. R., Covello, P. S., McMickle, A., Teoh, K. H., and Reed, D. W. (2011). Artemisinin Production in Artemisia Annua: Studies in Planta and Results of a Novel Delivery Method for Treating Malaria and Other Neglected Diseases. Phytochem. Rev. 10, 173–183. doi:10.1007/s11101-010-9166-0
Xiao, H., Zhang, Y., and Wang, M. (2019). Discovery and Engineering of Cytochrome P450s for Terpenoid Biosynthesis. Trends Biotechnol. 37, 618–631. doi:10.1016/j.tibtech.2018.11.008
Zhang, C., Ju, H., Lu, C.-Z., Zhao, F., Liu, J., Guo, X., et al. (2019). High-titer Production of 13R-Manoyl Oxide in Metabolically Engineered Saccharomyces cerevisiae. Microb. Cell. Fact. 18, 73. doi:10.1186/s12934-019-1123-z
Zhang, C., Liu, J., Zhao, F., Lu, C., Zhao, G.-R., and Lu, W. (2018). Production of Sesquiterpenoid Zerumbone from Metabolic Engineered Saccharomyces cerevisiae. Metab. Eng. 49, 28–35. doi:10.1016/j.ymben.2018.07.010
Zhang, H., Im, S.-C., and Waskell, L. (2007). Cytochrome B5 Increases the Rate of Product Formation by Cytochrome P450 2B4 and Competes with Cytochrome P450 Reductase for a Binding Site on Cytochrome P450 2B4. J. Biol. Chem. 282, 29766–29776. doi:10.1074/jbc.M703845200
Zhang, X., Xue, C., Zhao, F., Li, D., Yin, J., Zhang, C., et al. (2014). Suitable Extracellular Oxidoreduction Potential Inhibit Rex Regulation and Effect Central Carbon and Energy Metabolism in Saccharopolyspora Spinosa. Microb. Cell. Fact. 13, 98. doi:10.1186/s12934-014-0098-z
Zhao, F., Bai, P., Liu, T., Li, D., Zhang, X., Lu, W., et al. (2016). Optimization of a Cytochrome P450 Oxidation System for Enhancing Protopanaxadiol Production inSaccharomyces Cerevisiae. Biotechnol. Bioeng. 113, 1787–1795. doi:10.1002/bit.25934
Zhou, X., Zhou, J., Tian, H., and Yuan, Y. (2010). Dynamic Lipidomic Insights into the Adaptive Responses ofSaccharomyces Cerevisiaeto the Repeated Vacuum Fermentation. OMICS A J. Integr. Biol. 14, 563–574. doi:10.1089/omi.2010.0016
Zhou, Y. J., Gao, W., Rong, Q., Jin, G., Chu, H., Liu, W., et al. (2012). Modular Pathway Engineering of Diterpenoid Synthases and the Mevalonic Acid Pathway for Miltiradiene Production. J. Am. Chem. Soc. 134, 3234–3241. doi:10.1021/ja2114486
Keywords: carnosic acid, Saccharomyces cerevisiae, terpenoid, miltiradiene, synthetic biology
Citation: Wei P, Zhang C, Bian X and Lu W (2022) Metabolic Engineering of Saccharomyces cerevisiae for Heterologous Carnosic Acid Production. Front. Bioeng. Biotechnol. 10:916605. doi: 10.3389/fbioe.2022.916605
Received: 09 April 2022; Accepted: 16 May 2022;
Published: 02 June 2022.
Edited by:
Anderson Garbuglio Oliveira, University of São Paulo, BrazilReviewed by:
Jifeng Yuan, Xiamen University, ChinaXiaowei Li, Chalmers University of Technology, Sweden
Copyright © 2022 Wei, Zhang, Bian and Lu. This is an open-access article distributed under the terms of the Creative Commons Attribution License (CC BY). The use, distribution or reproduction in other forums is permitted, provided the original author(s) and the copyright owner(s) are credited and that the original publication in this journal is cited, in accordance with accepted academic practice. No use, distribution or reproduction is permitted which does not comply with these terms.
*Correspondence: Wenyu Lu, d2VueXVsdUB0anUuZWR1LmNu
†These authors have contributed equally to this work