- 1Key Laboratory of Shaanxi Province for Craniofacial Precision Medicine Research, College of Stomatology, Xi’an Jiaotong University, Xi’an, China
- 2School of Chemistry, Xi’an Jiaotong University, Xi’an, China
Calcium phosphate (CaP)-based bioceramics are the most widely used synthetic biomaterials for reconstructing damaged bone. Accompanied by bone healing process, implanted materials are gradually degraded while bone ultimately returns to its original geometry and function. In this progress report, we reviewed the complex and tight relationship between the bone healing response and CaP-based biomaterials, with the emphasis on the in vivo degradation mechanisms of such material and their osteoinductive properties mediated by immune responses, osteoclastogenesis and osteoblasts. A deep understanding of the interaction between biological healing process and biomaterials will optimize the design of CaP-based biomaterials, and further translate into effective strategies for biomaterials customization.
1 Introduction
The clinical intervention of bone healing is necessary for bone defects beyond the critical size, such as large segmental bone defects caused by severe trauma, tumor resection, cancer, congenital diseases, or small-scale alveolar bone defects (Davies et al., 2017; Majidinia et al., 2018). Given inevitable drawbacks of autografts (including donor site pain, increased operative time, risk of wound infection, and insufficient availability), many synthetic biomaterials have been specifically designed as an alternative for bone repair and regeneration (Pina et al., 2015; Xu et al., 2017; Stastny et al., 2019). Since the 1970s, calcium phosphate (CaP)-based bioceramics have received the most attention, and several of them [e.g., β-tricalcium phosphate (β-TCP) and hydroxyapatite (HA or HAP)] have already been clinically applied for orthopedic and maxillofacial surgery (Schaefer et al., 2011; Danoux et al., 2015).
For bone-filling purposes, CaPs have been utilized as bioactive components of solid ceramic, coatings, self-setting CaP cements (CPC), as well as advanced polymers, intending to function as a scaffold for bone formation (Parent et al., 2017). The family of CaP biomaterials comprises varying phase compositions, including HA [Ca10(PO4)6(OH)2], tricalcium phosphate [TCP, Ca3(PO4)2], octacalcium phosphate [OCP, Ca8(HPO4)2(PO4)4·5H2O], dicalcium phosphate dihydrate [DCPD, CaHPO4.2H2O], and amorphous calcium phosphate [ACP, CaxHy(PO4)z·nH2O, n = 3–4.5; 15–20% H2O], can be used in a variety of applications due to differences in solubility, stability, and mechanical strength (Jeong et al., 2019; Dorozhkin, 2021). CaP-based biomaterials provide a strong biomaterial/bone interface and demonstrate promising biological properties such as biodegradability, osteoconductivity, and in some cases even osteoinductivity (i.e., the ability of the material to induce de novo bone formation without the presence of osteogenic factors) (LeGeros, 2008; Chai et al., 2012; Stastny et al., 2019). In addition, CaPs can be manufactured in large quantities at relatively low cost, meeting the requirements of socially responsible tissue engineering and regenerative medicine (Habraken et al., 2016).
Much effort has been spent on optimizing biomechanical and physico-chemical properties to “engineer” materials for better “osteoinductivity” (Bigi and Boanini, 2017; Ruffini et al., 2021). In this context, the stiffness, porosity and pore size, surface microstructure, phase composition, and crystallinity have all been shown to affect the bone regeneration capacity of CaP-based materials. These bone-matching “static” physico-chemical parameters and the underlying osteogenesis mechanisms have been summarized in recent reviews for material design optimization (Habraken et al., 2016; Galvan-Chacon and Habibovic, 2017; Przekora, 2019; Dee et al., 2020). Nevertheless, the laboratory-prepared bone graft materials are often not successful in clinical application (Chen Z. et al., 2016). The regeneration capacity of biomaterials is usually evaluated by in vitro osteoblastic lineage (Diez-Escudero et al., 2017b), and in vivo bone histomorphology measurements (Yuan et al., 2000; Chiba et al., 2016). However, the biological process of bone healing to implanted materials has always been neglected. The mechanisms underlying bone healing are not simply led by osteoblasts, but a synergy of multiple systems involving a series of biological events including early inflammation response and long-term reconstruction process (Newman et al., 2021; Zhu et al., 2021). Therefore, the optimization and design of CaP-based biomaterials should keep pace with the rapid progress in bone healing biology, which eventually determines the in vivo fate of CaP-based biomaterials and bone healing effects.
In this review, we first summarized the biological process of bone healing and then focused on major developments in the bone healing response to CaP-based biomaterials. Understanding the interaction between biomaterials and host response during the bone healing process is important for improving the design of CaP-based biomaterials. Simultaneously, we also provide an outlook toward customizable CaP-polymer composite biomaterial strategies.
2 Overview of Bone Healing Process
There are three overlapping stages of the bone healing process: inflammation, bone formation and bone remodeling (Maruyama et al., 2020; Newman et al., 2021; Zhu et al., 2021).
Inflammation begins immediately after the bone is broken and lasts for several days. Driven by cytokines, macrophages and other immune cells (granulocytes, lymphocytes, monocytes, etc.) infiltrate bone defects, which trigger inflammatory reactions, clean up bone-tissue debris, as well as form vascular tissue and granulation tissue (Maruyama et al., 2020). Macrophages demonstrate broad roles here in regulating bone tissue homeostasis. Under the stimulation of the locally ischemic and hypoxic environment, and cytokines [e.g., macrophage colony-stimulating factor (M-CSF) and receptor activator of NF-κB ligand (RANKL)], macrophages polarize towards “M1” type. They secrete a series of pro-inflammatory cytokines [e.g., tumor necrosis factor-α (TNF-α), interleukin-1 (IL-1), IL-6], which in turn recruit mesenchymal stem cells (MSCs), osteoprogenitor cells, and fibroblasts for tissue repair (Miron et al., 2016; Zhu et al., 2021). Macrophages transform towards M2 wound-healing type at the end of the inflammatory stage and secrete anti-inflammatory cytokines [e.g., IL-4, IL-10, and transforming growth factors beta (TGF-β)] in favor of establishing osteogenic environments (Oliveira et al., 2021).
During the bone formation process, there are two repair mechanisms depending on the location of the bone defect. The cancellous and inter-cortical bone regions are repaired by endochondral ossification, while the subperiosteal and adjacent soft tissue regions are repaired by intramembranous ossification (Zhu et al., 2021). In cancellous and inter-cortical bone regions, the recruited MSCs aggregate, proliferate and differentiate into chondrocytes. They secrete an avascular cartilage matrix, and the granulation tissue is gradually replaced by the fibrocartilage- and hyaline cartilage-rich soft callus (Nossin et al., 2021). Along with new blood vessel formation, endothelial cells, osteoblasts, and chondrocytes secrete matrix metalloproteinases to degrade the cartilage matrix. Mature hypertrophic chondrocytes undergo apoptosis or transition into osteoblast-like cells, which together with osteoblasts, contribute to the secretion of type I collagen and the extracellular matrix (ECM) mineralization. Finally, the soft callus is transformed into the disordered woven bone (Tsang et al., 2015; Fu et al., 2021). Once the periosteum and adjacent soft tissue regions are injured, the MSCs recruited from the periosteum, bone marrow, adjacent soft tissues, and peripheral circulation and osteoprogenitor cells within the periosteum initiate the intramembranous bone formation process. MSCs and osteoprogenitor cells differentiate into osteoblasts. These cells secrete and mineralize ECM, forming hard callus directly under the periosteum (Wang et al., 2020).
Bone remodeling is the final stage of bone healing and typically lasts for several months (ElHawary et al., 2021). As a dynamic process, the irregular woven bone is reconstructed by bone resorption and osteogenesis process under mechanical stimulation and further developed as mature lamellar bone (Frost, 2004; Loi et al., 2016; ElHawary et al., 2021). The crosstalk between osteoblasts and osteoclasts plays an important role in this phase. Bone ultimately returns to its original shape and restores the geometry and function of the damaged part (Zhu et al., 2021).
3 Calcium Phosphate-Based Biomaterials Participate in Bone Healing Process
With similar composition to bone minerals, CaP-based biomaterials induce biological responses like those occur during bone healing (Diez-Escudero et al., 2017a). On the one hand, multiple cells involved in bone healing mediate the degradation and resorption of implanted materials. This is critical for CaP-based biomaterials to provide the space into which the bone and vascular tissues can grow (Rustom et al., 2019). The concomitant release of inorganic ions from the biodegradation of CaPs is also necessary for modifying the behavior of cells/tissues and enhances the osteoinductivity of the biomaterials (Tang et al., 2018). On the other hand, the osteoinductivity of CaP-based biomaterials is complicated in vivo as inflammation and osteoclastogenesis accompany the entire process of bone healing. Recent studies have pointed out that the physico-chemical properties of CaP-based biomaterials could affect the activity and function of immune cells and osteoclasts, and indirectly or directly affect osteogenesis, respectively (Figure 1). In this section, we will detail these tight and complex interactions between CaP-based biomaterials and bone healing responses.
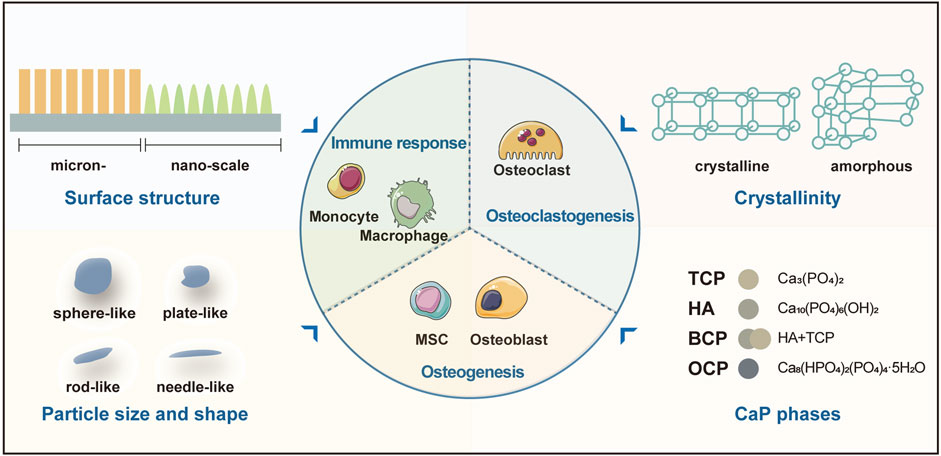
FIGURE 1. The physico-chemical properties of CaP-based biomaterials affect the activity and function of immune cells, osteoclasts, and osteoblasts. Abbreviation: BCP, biphasic calcium phosphate; TCP, tricalcium phosphate; HA, hydroxyapatite; MSC, mesenchymal stem cell; OCP, octacalcium phosphate.
3.1 Mechanisms of in vivo Biodegradation of Calcium Phosphate-Based Biomaterials
After implantation, the host body will first undergo a universal immune response to the materials. Proteins (e.g., fibrinogen, vitronectin, complement, and fibronectin) from blood and interstitial fluid will immediately adsorb on the material surface and then trigger an acute inflammation. Chemoattractants and cytokines such as platelet factor 4 (PF-4), platelet-derived growth factor (PDGF), and TGF-β lead to monocyte colonization and differentiate into macrophages and osteoclasts (Chen Z. et al., 2016; Galvan-Chacon and Habibovic, 2017). Meanwhile, CaP-based biomaterials will undergo degradation and resorption processes in vivo (Sheikh et al., 2015). Degradation is a physical process utilizing chemical dissolution or mechanical degradation. The disintegration and fragmentation of implants are often accompanied by the release of material particles (Sheikh et al., 2015; Galvan-Chacon and Habibovic, 2017). Biomaterial degradation paths the way for cell-mediated resorption processes that involve macrophages, osteoclasts, and multinucleated giant cells (Sheikh et al., 2015; Miron and Bosshardt, 2016; Xiao et al., 2016).
CaP particles can be directly engulfed via phagocytosis and intracellular digestion by macrophages and osteoclasts (size <10 μm), as well as giant cells (size between 10 and 100 μm) (Heymann et al., 2001; Wenisch et al., 2003; Nicolin et al., 2016). The particles, if larger than 100 μm, will undergo extracellular degradation within the acidic microenvironment created by osteoclasts (Galvan-Chacon and Habibovic, 2017). Solubility of the biomaterial (Yamada et al., 1997; Detsch et al., 2010; Wu and Uskokovic, 2016) and topographic characteristics such as specific surface area and the porosity content (Zimmer et al., 2013; Davison et al., 2014; Diez-Escudero et al., 2017b) are relevant physicochemical factors that influence the osteoclasts resorption activity.
In addition, cytokines released by monocytes/macrophages are responsible for the recruitment of osteoprogenitor cells and MSCs (Przekora, 2019). Cytokines such as M-CSF, RANKL and osteoprotegerin are responsible for stimulating osteoclastogenesis (Chen Z. et al., 2016). Contrary to previous beliefs that all inflammatory responses should be suppressed after biomaterial implantation, healing is promoted by suppression of extended chronic inflammatory responses (Miron and Bosshardt, 2016; Batool et al., 2021).
3.2 The Interaction Between Bone Healing Response and Calcium Phosphate-Based Biomaterials
3.2.1 Calcium Phosphate-Based Materials Regulate Osteoimmune
The concept of “osteoimmunomodulation” was proposed by Chen Z. et al. (2016) to emphasize the important roles of bone immune response in determining the in vivo fate of bone substitute materials. Macrophages are major effector cells in determining the duration and intensity of material-induced immune responses and are indispensable for osteogenesis (Chen et al., 2014a; Xiao et al., 2021). Chen and his team (Chen et al., 2014b) demonstrated that macrophages switch to the M2 phenotype through the calcium-sensing receptor (CaSR) pathway in response to β-TCP powder extracts. At the same time, the secreted bone morphogenetic protein 2 (BMP-2), as well as anti-inflammatory genes (IL-10 and IL-1rα) were up-regulated, and inflammatory genes (IL-1β and IL-6) were significantly down-regulated in macrophages. When bone marrow mesenchymal stem cells (BMSCs) were cultured in macrophage/β-TCP extracts conditioned medium, the osteogenic differentiation of BMSCs was significantly enhanced. More recent studies also reported similar findings that under the stimulation of biphasic calcium phosphate (BCP) ceramics, the expression of anti-inflammatory cytokine (IL-10) and growth factors, including vascular endothelial growth factor (VEGF), PDGF, epidermal growth factor (EGF), BMP-2 and TGF-β1 was increased, which might synergistically create a pro-osteogenic micro-environment, resulting in MSCs recruitment and differentiation into osteoblasts (Chen X. et al., 2016; Wang et al., 2017). Compared with macrophage/BCP conditioned media, the degradation particles of BCP might play a more substantial role in this process (Wang et al., 2017). However, the increased expression of inflammatory factors [IL-6, monocyte chemoattractant protein-1 (MCP-1), and TNF-α] in macrophages was found by Wang et al. (2017), and the increment of these pro-inflammatory cytokines did not seem to inhibit the osteogenic differentiation of MSCs. The reason may be that the effects of inflammatory cytokines are dose-dependent, e.g., only IL-6 of high concentration (above 10 ng ml−1) was proven to exhibit an inhibitory effect on BCP-induced osteogenesis (Chen X. et al., 2016). Further, more in vivo evaluations are needed for validating the immune-stimulated osteogenesis after CaP-based biomaterials implantation.
3.2.2 Calcium Phosphate-Based Materials Regulate Osteoclast-Mediated Osteogenesis
Bone resorption mediated by osteoclasts is tightly coupled with osteogenesis of osteoblasts (Chen et al., 2018; Kim et al., 2020). Substantial efforts have been made in modifying the properties of CaP-based materials to regulate the activities of osteoclasts. And the phases, surface structure, and crystallinity of CaP materials have been shown to control osteoclast activities (Takami et al., 2009; Davison et al., 2014; Uskokovic et al., 2019; Wang et al., 2021). Wang et al. (2021) prepared a series of CPC scaffolds with different calcium and phosphate ratios (Ca/P) and found that slight release of phosphate ions from CPC with higher Ca/P ratio (1.67) improved osteoclastogenesis. The released phosphate ions promoted osteoclast differentiation via increased affinity between RANKL and RANK, as well as robust NF-κB signaling transduction. Rat calvarial defect model further supported that CPC with high Ca/P ratio could ameliorate osteoclast-mediated bone healing. In addition to ions effects, the topographical structure and crystallinity of CaP materials could also be tuned to control osteoclast activities. For instance, Davison et al. (2014) indicated that β-TCP with submicron structure feature facilitated human peripheral blood monocytes differentiation into osteoclasts compared to microstructure. Similarly, another study pointed out that the surface structure of BCP affected osteoclastogenesis and bone ectopic formation after material implantation into the dorsal muscle of dogs (Davison et al., 2015). A recent study also showed that osteoclasts could sense the crystallinity of materials and higher crystallinity enhances metabolic and resorption activities of osteoclasts (Uskokovic et al., 2019).
In addition, the phases of CaPs in implanted materials could affect the osteoblasts-osteoclasts crosstalk in vitro. Shiwaku et al. (2015) found that osteoclasts cultured on BCPs with low HA content (5%) increased the expression of positive coupling factors, including sphingosine-kinase 1 (SPHK1) and collagen triple helix repeat containing 1 (Cthrc1). In a subsequent study, they cultured osteoclasts on HA/OCP or HA/TCP disks to investigate how the distinct chemical composition and crystal structure affect osteoclast formation and the osteoclasts-osteoblasts crosstalk. Both OCP and β-TCP had a similar ability to create multinucleated osteoclasts. OCP promoted the expression of complement component 3a (C3a) and β-TCP enhanced that of EphrinB2 (EfnB2) and Cthrc1 in osteoclasts, respectively. In turn, these secreted positive coupling factors promoted osteoblast differentiation and function (Shiwaku et al., 2019). Chemical properties, such as dissolution and precipitation around OCP and β-TCP crystals, may be factors that contribute to similar and different cellular responses in these CaP phases.
3.2.3 Biological Cell Responses to Internalized Calcium Phosphate Particles
Nanoscale CaP particles are produced from degradation or mechanical abrasion of macroscopic implants or direct implantation as nanoscale bone substitute materials (Epple, 2018). In all these cases, the surrounding tissue is subjected to calcium phosphate nanoparticles (CaP-NPs) at varying concentrations. Apart from small CaP-NPs (a few nm in diameter) that can directly penetrate the cell membrane on their own, almost all CaP nanoparticles are internalized by endocytosis and/or phagocytosis (Mahmoudi et al., 2011). Primary endosomes or phagosomes then fuse with cytoplasmic lysosomes to dissolve the CaP-NPs into calcium and phosphate. The cargo is eventually delivered into the cytoplasm (Epple, 2018). The CaP-NP-induced apoptotic pathway is mediated via a mitochondrial-dependent pathway (Xu et al., 2012; Xue et al., 2017). The sizes (Shi et al., 2009), surface topography and morphology (Zhao et al., 2007; Okada et al., 2010) and concentrations (Xu et al., 2012) of CaP-NPs have significant effects on the apoptotic level. The CaP-NPs are not cytotoxic per se, but the release of a large number of calcium ions after dissolution of the CaP-NPs is harmful. And apoptosis is induced by increased intracellular calcium ions concentration (Masouleh et al., 2017).
It is worthy that particles released from CaP materials degradation should not disturb the ability of bone-forming cells to form new bone. Therefore, in addition to studying the effect of materials’ macroscopic properties on the osteogenic ability of MSCs/osteoblasts, more studies on the effects of CaP-NPs on these cells and the underlying mechanisms have been investigated (Albulescu et al., 2019; Khotib et al., 2021). Particle size, shape, and concentration will have different effects on the function, viability, proliferation, and extracellular matrix of osteoblasts (Sheikh et al., 2015; Li et al., 2022). CaP-NPs have dose-dependent effects on osteoblasts and the maximum number of nanoparticles a single osteoblast can tolerate is 50 (Pioletti et al., 2000). Small CaP particles (1–10 μm) are less biocompatible than large particles (>10 μm) on the viability, proliferation and gene expression of osteoblasts (Pioletti et al., 2000). The CaP-NPs with higher specific surface area could increase the adhesion of osteoblasts. However, Xu et al. (2012) found that larger specific surface areas of CaP-NPs might induce higher apoptosis rates in the concentration range of 20–100 μg/ml. Jin et al. (2017) have studied the effect of unfunctionalized calcium phosphate nanorods (HA; 20 nm in diameter and 80 nm in length) on MC3T3 cells. They observed an uptake by micropinocytosis and a significant cytotoxicity above 40 mg L−1, which was caused by oxidative stress and lysosomal rupture. In a study using SAOS-2 osteoblasts, nano-HA were actively internalized and are retained within intracellular membrane-bound compartments. Dissolution of the nano-HA was observed within phagolysosomes. After 24 h of internalization, re-precipitation of needle-like minerals appears in the intracellular membrane-bound compartments (Rossi et al., 2018). These re-precipitated nanoparticles may provide a cellular protective mechanism against the cytotoxicity of rapidly elevated cytoplasmic calcium ions (Rossi et al., 2018).
In addition, particle size also significantly affects the activity of stimulating osteogenic differentiation. Yang et al. (2018) cultured MSCs with three different-sized nano-HA (∼50, ∼100, and ∼150 nm, resp.) and found that smaller-sized nano-HA (∼50 and ∼100 nm) accelerated the expression of osteoblast-like cell osteogenic genes (Yang et al., 2018). This is in line with that smaller-sized nano-HA (40–1000 nm) appeared to be more effective in stimulating osteogenic differentiation of hMSCs (Weissenboeck et al., 2006). An in vitro enzymatic reaction route has been employed for generating biomimetic amorphous calcium phosphate (EACP) nanominerals (Jiang et al., 2018). The amorphous nanomineral is composed of organic-inorganic hybrid nanoparticles. Adenosine triphosphate (ATP) provides the pool of phosphate ions for EACP formation. After internalization of EACP into human bone marrow-derived mesenchymal stem cells (hBMSCs), the release of ADP/AMP biomolecules and calcium ions activate adenosine 5′-monophosphate (AMP)-activated protein kinase (AMPK) and induces autophagy and osteogenic differentiation of hBMSCs (Jiang et al., 2018).
4 Customizable Calcium Phosphate-Polymer Composite Biomaterials
Although humans share a set of bone healing mechanisms, the effects of bone healing vary in individuals, depending on the conditions of bone defects. Such diversities account for the different rates and effects of bone regeneration. Because these biomaterials are likely to be transplanted into an ‘unfriendly’ host, the disease state, and other specifics of the host, such as age, ongoing chronic inflammation and infection should be well understood and taken into consideration. Accordingly, the fabrication of a customizable bone graft to match the condition of the bone defect is important for efficient bone regeneration.
Customizable bone graft substitutes may be designed by following two principles: 1) Tailored external construction and internal structural mimicking of the microenvironment of the defect site. The specific technologies and methods relevant to the design and manufacture of such materials are summarized in another review (Forrestal et al., 2017). 2) Customized biodegradation rate that matches the new bone formation rate, which needs to be complemented by an appropriate in vivo bone healing response. In this context, introducing CaPs into smart hydrogel systems may be a promising solution.
Hydrogels possess good biocompatibility and tissue integration properties and serve as the carriers in drug systems and bone tissue engineering biomaterials (Forrestal et al., 2017; Mantha et al., 2019). In response to stimulus [including exogenous (thermoresponsively-, magnetically-, electroresponsively- or light-triggered) and internal physical/biochemical (pH-, redox- or enzyme-sensitive)], hydrogel systems can change their structures, compositions or conformations to support the release of encapsulated active species (Claassen et al., 2019; Wei et al., 2022). The specific microenvironment of bone healing process, such as specific immune environment or enzyme release, can be used to design corresponding intelligent stimulus-responsive biomaterials for rapid reactive bone therapy and bone regeneration. Enzyme-responsive systems provide enlightenments for the selection of biomolecules. The design of enzyme-responsive systems for drug delivery relies on esterase- or protease-mediated cleavage of esters or short peptide sequences, resulting in effective drug release (Ramasamy et al., 2017). By choosing an appropriate peptide sequence, enzyme-responsive materials may be designed to respond exclusively to a target enzyme (Ramasamy et al., 2017). To be specific, tissue-nonspecific alkaline phosphatase (TNAP) may be considered a candidate. The TNAP hydrolyzes inorganic pyrophosphate (PPi, a potent mineralization inhibitor) and ATP into monophosphate (Pi, inorganic phosphate) to control ECM mineralization (Bottini et al., 2018). Ideally, a matching peptide sequence can make polymers respond exclusively to TNAP. New bone formation that coordinates with the biodegradation of CaP-polymer composites may be achieved by harnessing the TNAP-response to achieve a customized biodegradation rate.
5 Conclusion
Synthetic biodegradable bone grafts are gradually being replaced by the patient’s own bone. After implantation, tight and complex interactions occur between CaP-based biomaterials and the bone healing process. Multiple cells involved in bone healing mediate the materials’ degradation and resorption, which is essential to provide the space into which the bone and vascular tissues can grow. The physico-chemical properties of materials are also necessary for modifying the behavior of cells/tissues and enhancing the osteoinductivity of the biomaterials. In light with previous studies, we summarized the osteoinductive mechanism of CaP-based biomaterials accompanying bone healing (Figure 2). Under the stimulation with CaP-based biomaterials, the expression of inflammatory factors (IL-1β) is decreased, and the production of anti-inflammatory cytokine (IL-10, IL-1rα), as well as growth factors (VEGF, PDGF, EGF, BMP-2 and TGF-β1) is increased in macrophages, which synergistically create a pro-osteogenic micro-environment. The surface topography and crystallinity of CaP-based biomaterials can be sensed by osteoclasts and regulate their metabolic activity and resorption capacity, then influencing bone formation. These material particles are eventually internalized into cells and are involved in the regulation of cell homeostasis and cell differentiation. Based on the in-depth understanding of the involvement of CaP-based biomaterials in the bone healing process, we propose the future design of these materials is to develop biomaterials with customizability, such as tailored geometry and biodegradation rate.
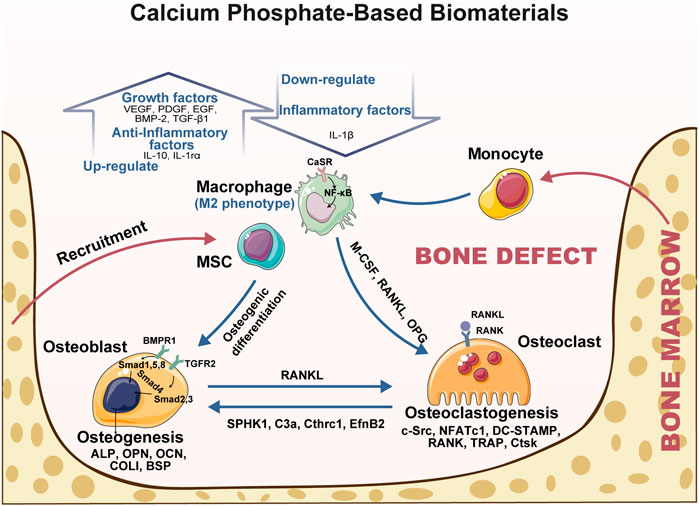
FIGURE 2. The interactions between CaP-based biomaterials and bone healing response. The osteoinductivity of CaP-based biomaterials can be “programmed” by their physico-chemical properties and are necessary for modifying the behavior of immune cells, osteoclasts, and MSCs/osteoblasts. Under the simulation with CaP-based biomaterials, the expression of inflammatory factors (IL-1β) is decreased, and the production of anti-inflammatory cytokine (IL-10, IL-1rα), as well as growth factors (VEGF, PDGF, EGF, BMP-2, and TGF-β1) is increased in macrophages. The surface structure and crystallinity of CaP-based biomaterials can be sensed by osteoclasts and regulate their metabolically active and resorption capacity. All above synergistically create a pro-osteogenic micro-environment leading to effects on bone formation. Abbreviation: ALP, alkaline phosphatase; BMP-2, bone morphogenetic protein two; BSP, bone sialoprotein; CaSR, calcium-sensing receptor; C3a, complement component 3a; Cthrc1, collagen triple helix repeat containing one; COL-I, collagen I; Ctsk, cathepsin k. DC-STAMP, dendritic cell-specific transmembrane protein; EfnB2, EphrinB2; EGF, epidermal growth factor; IL, interleukin; SPHK1, sphingosine-kinase one; MCP-1, monocyte chemoattractant protein-1; M-CSF, macrophage colony-stimulating factor; NFATc1, nuclear factor of activated T cells one; OCN, osteocalcin; OPN, osteopontin; OPG, osteoprotegerin; PDGF, platelet derived growth factor; RANKL, receptor activator of NF-κB ligand; TGF-β, transforming growth factor beta; TNF-α, tumor necrosis factor-α; TRAP, tartrate-resistant acid phosphatase; VEGF, vascular endothelial growth factor.
Author Contributions
YZ and TS drafted the manuscript and contributed equally to this work. YZ, TS, SW, ZL, and YC investigated related publications. AL and DP reviewed and edited the work. All authors contributed to the article and approved the submitted version.
Funding
This work was financially supported by the National Natural Science Foundation of China (81870798, 82170927, and 82071078), the Natural Science Basic Research Program of Shaanxi Province (2022JC-57) and the Fundamental Research Funds for the Central Universities (xtr012019007 and sxtr042021004).
Conflict of Interest
The authors declare that the research was conducted in the absence of any commercial or financial relationships that could be construed as a potential conflict of interest.
Publisher’s Note
All claims expressed in this article are solely those of the authors and do not necessarily represent those of their affiliated organizations, or those of the publisher, the editors and the reviewers. Any product that may be evaluated in this article, or claim that may be made by its manufacturer, is not guaranteed or endorsed by the publisher.
References
Albulescu, R., Popa, A.-C., Enciu, A.-M., Albulescu, L., Dudau, M., Popescu, I. D., et al. (2019). Comprehensive In Vitro Testing of Calcium Phosphate-Based Bioceramics with Orthopedic and Dentistry Applications. Materials 12, 3704. doi:10.3390/ma12223704
Batool, F., Özçelik, H., Stutz, C., Gegout, P.-Y., Benkirane-Jessel, N., Petit, C., et al. (2021). Modulation of Immune-Inflammatory Responses through Surface Modifications of Biomaterials to Promote Bone Healing and Regeneration. J. Tissue Eng. 12, 204173142110414. doi:10.1177/20417314211041428
Bigi, A., and Boanini, E. (2017). Functionalized Biomimetic Calcium Phosphates for Bone Tissue Repair. J. Appl. Biomaterials Funct. Mater. 15, e313–e325. doi:10.5301/jabfm.5000367
Bottini, M., Mebarek, S., Anderson, K. L., Strzelecka-Kiliszek, A., Bozycki, L., Simão, A. M. S., et al. (2018). Matrix Vesicles from Chondrocytes and Osteoblasts: Their Biogenesis, Properties, Functions and Biomimetic Models. Biochimica Biophysica Acta (BBA) - General Subj. 1862, 532–546. doi:10.1016/j.bbagen.2017.11.005
Chai, Y. C., Carlier, A., Bolander, J., Roberts, S. J., Geris, L., Schrooten, J., et al. (2012). Current Views on Calcium Phosphate Osteogenicity and the Translation into Effective Bone Regeneration Strategies. Acta Biomater. 8, 3876–3887. doi:10.1016/j.actbio.2012.07.002
Chen, X., Wang, J., Wang, J., Zhu, X., Yang, X., Fan, Y., et al. (2016a). The Positive Role of Macrophage Secretion Stimulated by BCP Ceramic in the Ceramic-Induced Osteogenic Differentiation of Pre-osteoblasts via Smad-Related Signaling Pathways. RSC Adv. 6, 102134–102141. doi:10.1039/C6RA23362G
Chen, X., Wang, Z., Duan, N., Zhu, G., Schwarz, E. M., and Xie, C. (2018). Osteoblast-osteoclast Interactions. Connect. Tissue Res. 59, 99–107. doi:10.1080/03008207.2017.1290085
Chen, Z., Klein, T., Murray, R. Z., Crawford, R., Chang, J., Wu, C., et al. (2016b). Osteoimmunomodulation for the Development of Advanced Bone Biomaterials. Mater. Today 19, 304–321. doi:10.1016/j.mattod.2015.11.004
Chen, Z., Mao, X., Tan, L., Friis, T., Wu, C., Crawford, R., et al. (2014a). Osteoimmunomodulatory Properties of Magnesium Scaffolds Coated with β-tricalcium Phosphate. Biomaterials 35, 8553–8565. doi:10.1016/j.biomaterials.2014.06.038
Chen, Z., Wu, C., Gu, W., Klein, T., Crawford, R., and Xiao, Y. (2014b). Osteogenic Differentiation of Bone Marrow MSCs by β-tricalcium Phosphate Stimulating Macrophages via BMP2 Signalling Pathway. Biomaterials 35, 1507–1518. doi:10.1016/j.biomaterials.2013.11.014
Chiba, S., Anada, T., Suzuki, K., Saito, K., Shiwaku, Y., Miyatake, N., et al. (2016). Effect of Resorption Rate and Osteoconductivity of Biodegradable Calcium Phosphate Materials on the Acquisition of Natural Bone Strength in the Repaired Bone. J. Biomed. Mat. Res. 104, 2833–2842. doi:10.1002/jbm.a.35828
Claassen, C., Gerlach, T., and Rother, D. (2019). Stimulus‐Responsive Regulation of Enzyme Activity for One‐Step and Multi‐Step Syntheses. Adv. Synth. Catal. 361, 2387–2401. doi:10.1002/adsc.201900169
Danoux, C. B. S. S., Bassett, D. C., Othman, Z., Rodrigues, A. I., Reis, R. L., Barralet, J. E., et al. (2015). Elucidating the Individual Effects of Calcium and Phosphate Ions on hMSCs by Using Composite Materials. Acta Biomater. 17, 1–15. doi:10.1016/j.actbio.2015.02.003
Davies, O. G., Cox, S. C., Williams, R. L., Tsaroucha, D., Dorrepaal, R. M., Lewis, M. P., et al. (2017). Annexin-enriched Osteoblast-Derived Vesicles Act as an Extracellular Site of Mineral Nucleation within Developing Stem Cell Cultures. Sci. Rep. 7, 12639. doi:10.1038/s41598-017-13027-6
Davison, N. L., ten Harkel, B., Schoenmaker, T., Luo, X., Yuan, H., Everts, V., et al. (2014). Osteoclast Resorption of Beta-Tricalcium Phosphate Controlled by Surface Architecture. Biomaterials 35, 7441–7451. doi:10.1016/j.biomaterials.2014.05.048
Davison, N., Su, J., Su, J., Yuan, H., van den Beucken, J., de Bruijn, J., et al. (2015). Influence of Surface Microstructure and Chemistry on Osteoinduction and Osteoclastogenesis by Biphasic Calcium Phosphate Discs. eCM 29, 314–329. doi:10.22203/ecm.v029a24
Dee, P., You, H. Y., Teoh, S.-H., and Le Ferrand, H. (2020). Bioinspired Approaches to Toughen Calcium Phosphate-Based Ceramics for Bone Repair. J. Mech. Behav. Biomed. Mater. 112, 104078. doi:10.1016/j.jmbbm.2020.104078
Detsch, R., Hagmeyer, D., Neumann, M., Schaefer, S., Vortkamp, A., Wuelling, M., et al. (2010). The Resorption of Nanocrystalline Calcium Phosphates by Osteoclast-like Cells. Acta Biomater. 6, 3223–3233. doi:10.1016/j.actbio.2010.03.003
Diez-Escudero, A., Espanol, M., Beats, S., and Ginebra, M.-P. (2017a). In Vitro degradation of Calcium Phosphates: Effect of Multiscale Porosity, Textural Properties and Composition. Acta Biomater. 60, 81–92. doi:10.1016/j.actbio.2017.07.033
Diez-Escudero, A., Espanol, M., Montufar, E. B., Di Pompo, G., Ciapetti, G., Baldini, N., et al. (2017b). Focus Ion Beam/Scanning Electron Microscopy Characterization of Osteoclastic Resorption of Calcium Phosphate Substrates. Tissue Eng. Part C. Methods 23 (2), 118–124. doi:10.1089/ten.TEC.2016.0361
Dorozhkin, S. V. (2021). Synthetic Amorphous Calcium Phosphates (ACPs): Preparation, Structure, Properties, and Biomedical Applications. Biomater. Sci. 9, 7748–7798. doi:10.1039/d1bm01239h
ElHawary, H., Baradaran, A., Abi-Rafeh, J., Vorstenbosch, J., Xu, L., and Efanov, J. I. (2021). Bone Healing and Inflammation: Principles of Fracture and Repair. Semin. Plast. Surg. 35, 198–203. doi:10.1055/s-0041-1732334
Epple, M. (2018). Review of Potential Health Risks Associated with Nanoscopic Calcium Phosphate. Acta Biomater. 77, 1–14. doi:10.1016/j.actbio.2018.07.036
Forrestal, D. P., Klein, T. J., and Woodruff, M. A. (2017). Challenges in Engineering Large Customized Bone Constructs. Biotechnol. Bioeng. 114, 1129–1139. doi:10.1002/bit.26222
Frost, H. M. (2004). A 2003 Update of Bone Physiology and Wolff's Law for Clinicians. Angle Orthod. 74, 3–15. doi:10.1043/0003-3219(2004)074<0003:AUOBPA>2.0.CO;2
Fu, R., Liu, C., Yan, Y., Li, Q., and Huang, R.-L. (2021). Bone Defect Reconstruction via Endochondral Ossification: A Developmental Engineering Strategy. J. Tissue Eng. 12, 204173142110042. doi:10.1177/20417314211004211
Galván-Chacón, V. P., and Habibovic, P. (2017). Deconvoluting the Bioactivity of Calcium Phosphate-Based Bone Graft Substitutes: Strategies to Understand the Role of Individual Material Properties. Adv. Healthc. Mat. 6, 1601478. doi:10.1002/adhm.201601478
Habraken, W., Habibovic, P., Epple, M., and Bohner, M. (2016). Calcium Phosphates in Biomedical Applications: Materials for the Future? Mater. Today 19, 69–87. doi:10.1016/j.mattod.2015.10.008
Heymann, D., Guicheux, J., and Rousselle, A. V. (2001). Ultrastructural Evidence In Vitro of Osteoclast-Induced Degradation of Calcium Phosphate Ceramic by Simultaneous Resorption and Phagocytosis Mechanisms. Histol. Histopathol. 16, 37–44. doi:10.14670/HH-16.37
Jeong, J., Kim, J. H., Shim, J. H., Hwang, N. S., and Heo, C. Y. (2019). Bioactive Calcium Phosphate Materials and Applications in Bone Regeneration. Biomater. Res. 23, 4. doi:10.1186/s40824-018-0149-3
Jiang, Y.-Y., Zhou, Z.-F., Zhu, Y.-J., Chen, F.-F., Lu, B.-Q., Cao, W.-T., et al. (2018). Enzymatic Reaction Generates Biomimic Nanominerals with Superior Bioactivity. Small 14, 1804321. doi:10.1002/smll.201804321
Jin, Y., Liu, X., Liu, H., Chen, S., Gao, C., Ge, K., et al. (2017). Oxidative Stress-Induced Apoptosis of Osteoblastic MC3T3-E1 Cells by Hydroxyapatite Nanoparticles through Lysosomal and Mitochondrial Pathways. RSC Adv. 7, 13010–13018. doi:10.1039/c7ra01008g
Khotib, J., Gani, M. A., Budiatin, A. S., Lestari, M. L. A. D., Rahadiansyah, E., and Ardianto, C. (2021). Signaling Pathway and Transcriptional Regulation in Osteoblasts during Bone Healing: Direct Involvement of Hydroxyapatite as a Biomaterial. Pharmaceuticals 14, 615. doi:10.3390/ph14070615
Kim, J.-M., Lin, C., Stavre, Z., Greenblatt, M. B., and Shim, J.-H. (2020). Osteoblast-osteoclast Communication and Bone Homeostasis. Cells 9, 2073. doi:10.3390/cells9092073
LeGeros, R. Z. (2008). Calcium Phosphate-Based Osteoinductive Materials. Chem. Rev. 108, 4742–4753. doi:10.1021/cr800427g
Li, X., Zhou, Q., Wu, Y., Feng, C., Yang, X., Wang, L., et al. (2022). Enhanced Bone Regenerative Properties of Calcium Phosphate Ceramic Granules in Rabbit Posterolateral Spinal Fusion through a Reduction of Grain Size. Bioact. Mater. 11, 90–106. doi:10.1016/j.bioactmat.2021.10.006
Loi, F., Córdova, L. A., Pajarinen, J., Lin, T.-h., Yao, Z., and Goodman, S. B. (2016). Inflammation, Fracture and Bone Repair. Bone 86, 119–130. doi:10.1016/j.bone.2016.02.020
Mahmoudi, M., Azadmanesh, K., Shokrgozar, M. A., Journeay, W. S., and Laurent, S. (2011). Effect of Nanoparticles on the Cell Life Cycle. Chem. Rev. 111, 3407–3432. doi:10.1021/cr1003166
Majidinia, M., Sadeghpour, A., and Yousefi, B. (2018). The Roles of Signaling Pathways in Bone Repair and Regeneration. J. Cell Physiol. 233, 2937–2948. doi:10.1002/jcp.26042
Mantha, S., Pillai, S., Khayambashi, P., Upadhyay, A., Zhang, Y., Tao, O., et al. (2019). Smart Hydrogels in Tissue Engineering and Regenerative Medicine. Materials 12, 3323. doi:10.3390/ma12203323
Maruyama, M., Rhee, C., Utsunomiya, T., Zhang, N., Ueno, M., Yao, Z., et al. (2020). Modulation of the Inflammatory Response and Bone Healing. Front. Endocrinol. 11, 386. doi:10.3389/fendo.2020.00386
Masouleh, M. P., Hosseini, V., Pourhaghgouy, M., and Bakht, M. K. (2017). Calcium Phosphate Nanoparticles Cytocompatibility versus Cytotoxicity: A Serendipitous Paradox. Cpd 23, 2930–2951. doi:10.2174/1570163814666170321115007
Miron, R. J., and Bosshardt, D. D. (2016). OsteoMacs: Key Players Around Bone Biomaterials. Biomaterials 82, 1–19. doi:10.1016/j.biomaterials.2015.12.017
Miron, R. J., Zohdi, H., Fujioka-Kobayashi, M., and Bosshardt, D. D. (2016). Giant Cells Around Bone Biomaterials: Osteoclasts or Multi-Nucleated Giant Cells? Acta Biomater. 46, 15–28. doi:10.1016/j.actbio.2016.09.029
Newman, H., Shih, Y. V., and Varghese, S. (2021). Resolution of Inflammation in Bone Regeneration: From Understandings to Therapeutic Applications. Biomaterials 277, 121114. doi:10.1016/j.biomaterials.2021.121114
Nicolin, V., Baldini, G., De Iaco, D., Bortul, R., Turco, G., and Nori, S. L. (2016). Looking for Calcium Phosphate Composite Suitable to Study Osteoclast Endocytosis: Preliminary Observations. Transl. Med. UniSa 14, 15–20.
Nossin, Y., Farrell, E., Koevoet, W. J. L. M., Datema, F., Somoza, R. A., Caplan, A. I., et al. (2021). The Releasate of Avascular Cartilage Demonstrates Inherent Pro-angiogenic Properties In Vitro and In Vivo. Cartilage 13 (2_Suppl. l), 559S–570S. doi:10.1177/19476035211047628
Okada, S., Ito, H., Nagai, A., Komotori, J., and Imai, H. (2010). Adhesion of Osteoblast-like Cells on Nanostructured Hydroxyapatite. Acta Biomater. 6, 591–597. doi:10.1016/j.actbio.2009.07.037
Oliveira, C. S., Leeuwenburgh, S., and Mano, J. F. (2021). New Insights into the Biomimetic Design and Biomedical Applications of Bioengineered Bone Microenvironments. Apl. Bioeng. 5, 041507. doi:10.1063/5.0065152
Parent, M., Baradari, H., Champion, E., Damia, C., and Viana-Trecant, M. (2017). Design of Calcium Phosphate Ceramics for Drug Delivery Applications in Bone Diseases: A Review of the Parameters Affecting the Loading and Release of the Therapeutic Substance. J. Control. Release 252, 1–17. doi:10.1016/j.jconrel.2017.02.012
Pina, S., Oliveira, J. M., and Reis, R. L. (2015). Natural-based Nanocomposites for Bone Tissue Engineering and Regenerative Medicine: A Review. Adv. Mat. 27, 1143–1169. doi:10.1002/adma.201403354
Pioletti, D. P., Takei, H., Lin, T., Van Landuyt, P., Jun Ma, Q., Yong Kwon, S., et al. (2000). The Effects of Calcium Phosphate Cement Particles on Osteoblast Functions. Biomaterials 21, 1103–1114. doi:10.1016/s0142-9612(99)00250-1
Przekora, A. (2019). The Summary of the Most Important Cell-Biomaterial Interactions that Need to Be Considered during In Vitro Biocompatibility Testing of Bone Scaffolds for Tissue Engineering Applications. Mater. Sci. Eng. C 97, 1036–1051. doi:10.1016/j.msec.2019.01.061
Ramasamy, T., Ruttala, H. B., Gupta, B., Poudel, B. K., Choi, H.-G., Yong, C. S., et al. (2017). Smart Chemistry-Based Nanosized Drug Delivery Systems for Systemic Applications: A Comprehensive Review. J. Control. Release 258, 226–253. doi:10.1016/j.jconrel.2017.04.043
Rossi, A. L., Longuinho, M. M., Tanaka, M. N., Farina, M., Borojevic, R., and Rossi, A. M. (2018). Intracellular Pathway and Subsequent Transformation of Hydroxyapatite Nanoparticles in the SAOS-2 Osteoblast Cell Line. J. Biomed. Mat. Res. 106, 428–439. doi:10.1002/jbm.a.36256
Ruffini, A., Sandri, M., Dapporto, M., Campodoni, E., Tampieri, A., and Sprio, S. (2021). Nature-inspired Unconventional Approaches to Develop 3D Bioceramic Scaffolds with Enhanced Regenerative Ability. Biomedicines 9, 916. doi:10.3390/biomedicines9080916
Rustom, L. E., Poellmann, M. J., and Wagoner Johnson, A. J. (2019). Mineralization in Micropores of Calcium Phosphate Scaffolds. Acta Biomater. 83, 435–455. doi:10.1016/j.actbio.2018.11.003
Schaefer, S., Detsch, R., Uhl, F., Deisinger, U., and Ziegler, G. (2011). How Degradation of Calcium Phosphate Bone Substitute Materials Is Influenced by Phase Composition and Porosity. Adv. Eng. Mat. 13, 342–350. doi:10.1002/adem.201000267
Sheikh, Z., Abdallah, M.-N., Hanafi, A., Misbahuddin, S., Rashid, H., and Glogauer, M. (2015). Mechanisms of In Vivo Degradation and Resorption of Calcium Phosphate Based Biomaterials. Materials 8 (11), 7913–7925. doi:10.3390/ma8115430
Shi, Z., Huang, X., Cai, Y., Tang, R., and Yang, D. (2009). Size Effect of Hydroxyapatite Nanoparticles on Proliferation and Apoptosis of Osteoblast-like Cells. Acta Biomater. 5, 338–345. doi:10.1016/j.actbio.2008.07.023
Shiwaku, Y., Neff, L., Nagano, K., Takeyama, K.-I., de Bruijn, J., Dard, M., et al. (2015). The Crosstalk between Osteoclasts and Osteoblasts Is Dependent upon the Composition and Structure of Biphasic Calcium Phosphates. PLoS One 10, e0132903. doi:10.1371/journal.pone.0132903
Shiwaku, Y., Tsuchiya, K., Xiao, L., and Suzuki, O. (2019). Effect of Calcium Phosphate Phases Affecting the Crosstalk between Osteoblasts and Osteoclasts In Vitro. J. Biomed. Mat. Res. 107, 1001–1013. doi:10.1002/jbm.a.36626
Stastny, P., Sedlacek, R., Suchy, T., Lukasova, V., Rampichova, M., and Trunec, M. (2019). Structure Degradation and Strength Changes of Sintered Calcium Phosphate Bone Scaffolds with Different Phase Structures during Simulated Biodegradation In Vitro. Mater. Sci. Eng. C 100, 544–553. doi:10.1016/j.msec.2019.03.027
Takami, M., Mochizuki, A., Yamada, A., Tachi, K., Zhao, B., Miyamoto, Y., et al. (2009). Osteoclast Differentiation Induced by Synthetic Octacalcium Phosphate through Receptor Activator of NF-Κb Ligand Expression in Osteoblasts. Tissue Eng. Part A 15 (12), 3991–4000. doi:10.1089/ten.TEA.2009.0065
Tang, Z., Li, X., Tan, Y., Fan, H., and Zhang, X. (2018). The Material and Biological Characteristics of Osteoinductive Calcium Phosphate Ceramics. Regen. Biomater. 5, 43–59. doi:10.1093/rb/rbx024
Tsang, K. Y., Chan, D., and Cheah, K. S. E. (2015). Fate of Growth Plate Hypertrophic Chondrocytes: Death or Lineage Extension? Dev. Growth Differ. 57, 179–192. doi:10.1111/dgd.12203
Uskoković, V., Janković-Častvan, I., and Wu, V. M. (2019). Bone Mineral Crystallinity Governs the Orchestration of Ossification and Resorption during Bone Remodeling. ACS Biomater. Sci. Eng. 5, 3483–3498. doi:10.1021/acsbiomaterials.9b00255
Wang, D., Gilbert, J. R., Zhang, X., Zhao, B., Ker, D. F. E., and Cooper, G. M. (2020). Calvarial versus Long Bone: Implications for Tailoring Skeletal Tissue Engineering. Tissue Eng. Part B Rev. 26, 46–63. doi:10.1089/ten.TEB.2018.0353
Wang, J., Liu, D., Guo, B., Yang, X., Chen, X., Zhu, X., et al. (2017). Role of Biphasic Calcium Phosphate Ceramic-Mediated Secretion of Signaling Molecules by Macrophages in Migration and Osteoblastic Differentiation of MSCs. Acta Biomater. 51, 447–460. doi:10.1016/j.actbio.2017.01.059
Wang, X., Yu, Y., Ji, L., Geng, Z., Wang, J., and Liu, C. (2021). Calcium Phosphate-Based Materials Regulate Osteoclast-Mediated Osseointegration. Bioact. Mater. 6, 4517–4530. doi:10.1016/j.bioactmat.2021.05.003
Wei, H., Cui, J., Lin, K., Xie, J., and Wang, X. (2022). Recent Advances in Smart Stimuli-Responsive Biomaterials for Bone Therapeutics and Regeneration. Bone. Res. 10, 17. doi:10.1038/s41413-021-00180-y
Weissenböck, M., Stein, E., Undt, G., Ewers, R., Lauer, G., and Turhani, D. (2006). Particle Size of Hydroxyapatite Granules Calcified from Red Algae Affects the Osteogenic Potential of Human Mesenchymal Stem Cells In Vitro. Cells Tissues Organs 182, 79–88. doi:10.1159/000093062
Wenisch, S., Stahl, J.-P., Horas, U., Heiss, C., Kilian, O., Trinkaus, K., et al. (2003). In Vivo mechanisms of Hydroxyapatite Ceramic Degradation by Osteoclasts: Fine Structural Microscopy. J. Biomed. Mat. Res. 67A, 713–718. doi:10.1002/jbm.a.10091
Wu, V. M., and Uskoković, V. (2016). Is There a Relationship between Solubility and Resorbability of Different Calcium Phosphate Phases In Vitro ? Biochimica Biophysica Acta (BBA) - General Subj. 1860, 2157–2168. doi:10.1016/j.bbagen.2016.05.022
Xiao, L., Shiwaku, Y., Hamai, R., Tsuchiya, K., Sasaki, K., and Suzuki, O. (2021). Macrophage Polarization Related to Crystal Phases of Calcium Phosphate Biomaterials. Ijms 22, 11252. doi:10.3390/ijms222011252
Xiao, W., Wang, Y., Pacios, S., Li, S., and Graves, D. T. (2016). Cellular and Molecular Aspects of Bone Remodeling. Front. Oral Biol. 18, 9–16. doi:10.1159/000351895
Xu, H. H., Wang, P., Wang, L., Bao, C., Chen, Q., Weir, M. D., et al. (2017). Calcium Phosphate Cements for Bone Engineering and Their Biological Properties. Bone Res. 5, 17056. doi:10.1038/boneres.2017.56
Xu, Z., Liu, C., Wei, J., and Sun, J. (2012). Effects of Four Types of Hydroxyapatite Nanoparticles with Different Nanocrystal Morphologies and Sizes on Apoptosis in Rat Osteoblasts. J. Appl. Toxicol. 32 (6), 429–435. doi:10.1002/jat.1745
Xue, Y., Chen, Q., and Sun, J. (2017). Hydroxyapatite Nanoparticle-Induced Mitochondrial Energy Metabolism Impairment in Liver Cells: In Vitro and In Vivo Studies. J. Appl. Toxicol. 37, 1004–1016. doi:10.1002/jat.3450
Yamada, S., Heymann, D., Bouler, J. M., and Daculsi, G. (1997). Osteoclastic Resorption of Calcium Phosphate Ceramics with Different Hydroxyapatite/β-Tricalcium Phosphate Ratios. Biomaterials 18, 1037–1041. doi:10.1016/s0142-9612(97)00036-7
Yang, X., Li, Y., Liu, X., Zhang, R., and Feng, Q. (2018). In Vitro Uptake of Hydroxyapatite Nanoparticles and Their Effect on Osteogenic Differentiation of Human Mesenchymal Stem Cells. Stem Cells Int. 2018, 1–10. doi:10.1155/2018/2036176
Yuan, H., Li, Y., de Bruijn, J., de Groot, K., and Zhang, X. (2000). Tissue Responses of Calcium Phosphate Cement: A Study in Dogs. Biomaterials 21, 1283–1290. doi:10.1016/S0142-9612(00)00016-8
Zhao, Y., Zhang, Y., Ning, F., Guo, D., and Xu, Z. (2007). Synthesis and Cellular Biocompatibility of Two Kinds of HAP with Different Nanocrystal Morphology. J. Biomed. Mat. Res. 83B, 121–126. doi:10.1002/jbm.b.30774
Zhu, G., Zhang, T., Chen, M., Yao, K., Huang, X., Zhang, B., et al. (2021). Bone Physiological Microenvironment and Healing Mechanism: Basis for Future Bone-Tissue Engineering Scaffolds. Bioact. Mater. 6, 4110–4140. doi:10.1016/j.bioactmat.2021.03.043
Keywords: calcium phosphate, biomaterials, osteoinductivity, biodegradation, bone healing
Citation: Zhang Y, Shu T, Wang S, Liu Z, Cheng Y, Li A and Pei D (2022) The Osteoinductivity of Calcium Phosphate-Based Biomaterials: A Tight Interaction With Bone Healing. Front. Bioeng. Biotechnol. 10:911180. doi: 10.3389/fbioe.2022.911180
Received: 02 April 2022; Accepted: 21 April 2022;
Published: 16 May 2022.
Edited by:
Kaili Lin, Shanghai Jiao Tong University, ChinaReviewed by:
Fucong Tian, Augusta University, United StatesXingge Yu, Shanghai Ninth People’s Hospital, China
Copyright © 2022 Zhang, Shu, Wang, Liu, Cheng, Li and Pei. This is an open-access article distributed under the terms of the Creative Commons Attribution License (CC BY). The use, distribution or reproduction in other forums is permitted, provided the original author(s) and the copyright owner(s) are credited and that the original publication in this journal is cited, in accordance with accepted academic practice. No use, distribution or reproduction is permitted which does not comply with these terms.
*Correspondence: Ang Li, ZHJsaWFuZ0B4anR1LmVkdS5jbg==; Dandan Pei, cGVpZGFuZGFuQHhqdHUuZWR1LmNu
†These authors have contributed equally to this work