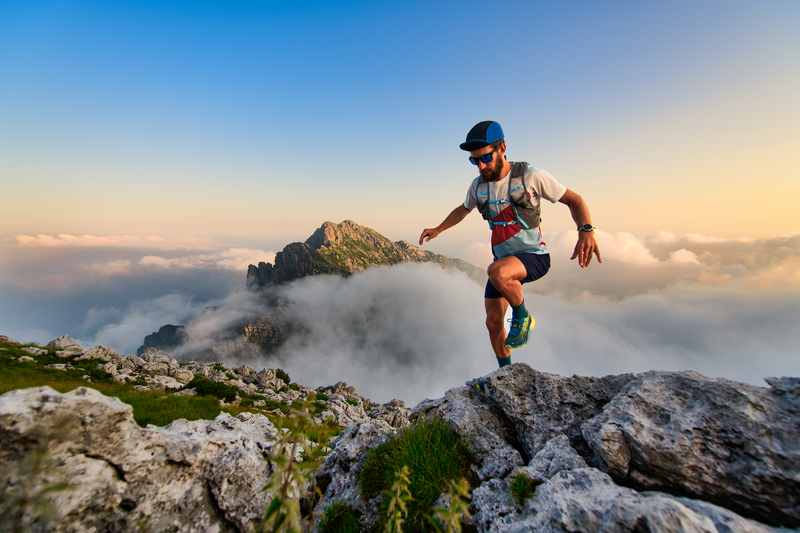
95% of researchers rate our articles as excellent or good
Learn more about the work of our research integrity team to safeguard the quality of each article we publish.
Find out more
REVIEW article
Front. Bioeng. Biotechnol. , 06 January 2023
Sec. Bioprocess Engineering
Volume 10 - 2022 | https://doi.org/10.3389/fbioe.2022.907500
This article is part of the Research Topic Current Challenges in Biodegradable Polyhydroxyalkanoates: From The Cradle To The Grave View all 7 articles
Growing concerns over the use of limited fossil fuels and their negative impacts on the ecological niches have facilitated the exploration of alternative routes. The use of conventional plastic material also negatively impacts the environment. One such green alternative is polyhydroxyalkanoates, which are biodegradable, biocompatible, and environmentally friendly. Recently, researchers have focused on the utilization of waste gases particularly those belonging to C1 sources derived directly from industries and anthropogenic activities, such as carbon dioxide, methane, and methanol as the substrate for polyhydroxyalkanoates production. Consequently, several microorganisms have been exploited to utilize waste gases for their growth and biopolymer accumulation. Methylotrophs such as Methylobacterium organophilum produced highest amount of PHA up to 88% using CH4 as the sole carbon source and 52–56% with CH3OH. On the other hand Cupriavidus necator, produced 71–81% of PHA by utilizing CO and CO2 as a substrate. The present review shows the potential of waste gas valorization as a promising solution for the sustainable production of polyhydroxyalkanoates. Key bottlenecks towards the usage of gaseous substrates obstructing their realization on a large scale and the possible technological solutions were also highlighted. Several strategies for PHA production using C1 gases through fermentation and metabolic engineering approaches are discussed. Microbes such as autotrophs, acetogens, and methanotrophs can produce PHA from CO2, CO, and CH4. Therefore, this article presents a vision of C1 gas into bioplastics are prospective strategies with promising potential application, and aspects related to the sustainability of the system.
Industries and societies are facing problems with the sustainable production of value-added chemicals while seeking reductions in greenhouse gas emissions (Ai-Yaeeshi et al., 2020). Currently, fossil fuels fulfill the need for energy and chemicals and play a major role in the global economy (Abe et al., 2019). Such resources are present in limited quantities, and their excessive use lead to an environmental pollution (Ai-Yaeeshi et al., 2020). In addition to anthropogenic activities, industries are also emitting waste gases, including carbon dioxide (CO2), methane (CH4), and carbon monoxide (CO) into the atmosphere every year (Choi et al., 2020a). Several approaches, such as physical, chemical methods, are employed to reduce pollution however; physio-chemical methods require intensive energy. To overcome this problem, these waste gases (C1) can be valorized to produce value-added chemicals by biological approaches (Dürre and Eikmanns, 2015). Some microbes can grow on these C1 compounds, including methanol (CH3OH). Therefore, they would help to mitigate waste gases from the environment while simultaneously exploiting them to produce value-added bioproducts such as polyhydroxyalkanoates (PHAs) a biodegradable biopolymer (Dürre and Eikmanns, 2015) (Figure 1). Once realized on a large scale, this could be a paradigm approach towards the valorization of waste gases to biopolymers. Such a waste biorefinery strategy offers a great potential towards a sustainable economy (Kumar et al., 2016). PHA production from C1 carbon sources have more advances such as i) direct bioconversion process ii) fermentation approach without sterilization.
FIGURE 1. Schematic diagram showing a brief represenation of overall C1 substrates assimilation pathway for PHA production. CBS: Calvin-Benson-Bassham cycle; RUMP: Ribulose Monophosphate Pathway.
Synthetic polymers are irreplaceable materials in our daily life and are commonly used in packaging, electronic devices, and the transportation of household materials. Globally, annual plastic production has reached 311 million tons and by 2050, it is anticipated to reach up to 500 million metric tons (Liu et al., 2020). The overuse of synthetic plastics leads to a prodigious amount of waste generated in the environment. The primary disadvantage is its non-biodegradable nature and accumulation in the environment in enormous quantities. When exposed to solar radiation, synthetic plastics generate greenhouse gases such as CH4 and ethylene. This scenario has encouraged many researchers to search for alternative polymers. Green alternatives by replacing synthetic plastics with biodegradable polymers such as PHAs are considered sustainable approaches (Raza et al., 2018). Microorganisms accumulate PHAs as carbon reservoirs under nutrient limiting conditions (Ray and Kalia, 2017a,b; Kumar et al., 2015). They have attractive physio-chemical properties such as elastomeric, piezoelectric, biodegradability, non-toxicity, and biocompatibility (Steinbüchel and Lutke-Eversloh, 2003; Chen and Wu, 2005; Bugnicourt et al., 2014; Ray and Kalia, 2017c). The physical properties of PHA depend on its monomeric composition, which is classified according to the number of carbon chain lengths such as short-chain length (scl, C2-C5), medium-chain length (mcl, C6-C14), and long-chain length in the monomer (lcl, C15-C20) (Ray and Kalia, 2017c). To date, one hundred sixty monomers of PHAs have been identified. Depending on the monomeric composition, PHAs have various applications (Singh et al., 2015; Choi et al., 2020b). Several homopolymers and co-polymers of PHAs are used in industrial and biomedical applications such as packaging, food additives, films, fibers, drug delivery, medical implants, scaffold preparation, tissue engineering, memory enhancers, and biofuels (Ray et al., 2018). However, higher substrate cost is the limiting factor for large-scale production.
Biowastes have been a key player in the production of value added bio-products, but the availability, difficulty in pretreatment has become an issue (Kumar et al., 2013). On the other hand, anthropogenic activities including industries lead to the emission of waste gasses, which cause environmental pollution. To reduce pollution, some gas-fermenting microbes can grow on C1 compounds to produce PHAs and, lead to the elimination of the consumption of biowastes (Dürre and Eikmanns, 2015). Several Gram-negative bacteria are capable of producing PHAs such as Azotobacter, Comamonas, Pseudomonas, Ralstonia, Cupriavidus, Haloferax, and Klebsiella, while some Gram-positive bacteria accumulate PHAs such as Streptomyces, Rhodococcus, Clostridium, Staphylococcus, Nocardia, Microlunatus, and Bacillus from diverse substrates (Kumar et al., 2016; Ray and Kalia, 2017a).
C1 gases play a major role in global warming. Commonly, C1 gases can be generated from household wastes, industrial wastes and agricultural wastes. Thus, they can be exploited by microbes for the production of valuable bio-products such as PHAs. This approach could help to alleviate the environmental crises of global warming and plastic waste. In this present review, recent advances in PHA production approaches from carbon dioxide (CO2), methane (CH4), methanol (CH3OH), carbon monoxide (CO), and formate are discussed. Utilization of C1 gases by autotrophs, acetogens and methanotrophs are discussed briefly. Several approaches to enhance PHA production such as metabolic engineering, process engineering etc. are discussed. These approaches help to establish a complete closed-loop PHA production from C1 gases and its effective degradation. This review articles encompasses the utility of PHA produced by C1 sources in various biotechnological applications.
Since the industrial revolution, CO2, a strong greenhouse gas emitter which has reached up to 43%, and by the year 2100, it is expected to increase by 60% (Kumar et al., 2016). The microbial approach towards CO2 sequestration and fixation are sustainable strategies for CO2 mitigation and are more energy-efficient methods as compared to catalytic conversion (Claassens, 2017). Ribulose-1,5-bisphosphate and carbonic anhydrase help microbes to capture and store CO2 thereby reducing greenhouse gases in the atmosphere (Kumar et al., 2016). Hydrogen-oxidizing bacteria, cyanobacteria, and algae are capable of consuming CO2 from industrial flue gas as the sole energy source or in the form of sodium bicarbonate (NaHCO3) or sodium carbonate (NaCO3). They produce several valuable bioproducts including PHA and also treat wastewater by removing organic matter, and heavy metals (Oswald et al., 1957; Rosgaard et al., 2012; Nozzi et al., 2013; Lee et al., 2015).
A technological approach based on the cyanobacterial treatment of wastewater was successfully employed for treating municipal and industrial wastewaters (Arias et al., 2020). Chlorella vulgaris, Chlorella pyrenoidosa, Rhodovulum viride, Scenedesmus obliquus, and Thermosynechococcus elongatus can tolerate high CO2 concentrations. Cyanobacterial CO2 fixation is mainly influenced by physical parameters, such as type of cultivation, type of bioreactors, pH, nutrients, temperature, and light (Salehizadeh et al., 2020). However, under nutrient-limiting conditions, cyanobacteria produce different types of storage compounds, such as glycogen, polyhydroxybutyrate (PHB) (carbon-rich), cyanophycin (nitrogen-rich), and polyphosphate (Price et al., 2020) (Figure 2 and Figure 3).
FIGURE 2. Schematic diagram showing PHA production pathway in cyanobacteria utilizing CO2 as carbon source. RuBP: Ribulose I, 5 his phosphate; 3PGA: 3-phosphoglyceric acid; 2PGA: 2-phosphoglyceric acid; PEP: Phosphoenolpyruvate.
FIGURE 3. Storage materials present in cyanobacteria. The figure is a representation of various storage materials produced by cyanobacteria under nutrient limiting conditions.
Through the oxidative pentose pathway, glycogen is synthesized in the presence of sunlight and CO2 (Shinde et al., 2020). When compared to glycogen, PHAs could serve as long-term storage material within the cell. Chlorogloea fritschii was the first reported cyanobacteria to produce PHA by utilizing acetate in a photoautotrophic environment (Carr, 1966; Singh et al., 2017). Fatty acid biosynthesis and nitrogen assimilation are proposed biosynthetic pathways for the production of PHAs (Mozejko-Ciesielska et al., 2018; Price et al., 2020). Cyanobacteria are capable of performing oxygenic photosynthesis via Calvin-Benson-Bassham (CBB) cycle (Figure 2). They produce ATP and NADPH by capturing the thylakoid membrane from sunlight. These two intermediates are utilized for essential nutrient assimilation. The CBB pathway contains 3 phases: carboxylation, reduction, and regeneration. In carboxylation, 6 molecules of 3-phosphoglycerate (PGA) were produced by combining by 3 molecules of CO2 and 6 molecules of ribulose 1, 4-bisphosphate (RuBP). Subsequently, the production of NADPH and ATP occurs to reduce PGA to triose phosphate and dihydroxyacetone phosphate (DHAP). 5 molecules of PGA are utilized to reproduce 3 molecules of RuBP. Cyanobacteria uptakes Ci, CO2, and HCO3− inside the cell by CO2 concentrating mechanism. There are five types of mechanisms; 3 for the uptake of HCO3− (BicA, SbtA, and BCT1) and 2 for the uptake of CO2 (NDH-I3 and NDH-I4) in cyanobacteria. CO2 transport occurs through Ci transporters at the plasma membrane. Bicarbonate transporters help to transport intracellular HCO3− ions across the plasma membrane, chloroplast, and periplasmic carbonic anhydrase convert HCO3− to CO2 (Durall and Lindblad, 2015).
Furthermore, the remaining 3-PGA is utilized for the synthesis of cellular material depending upon nutrient availability. RuBisCO, phosphoribulokinase, and sedoheptulose bisphosphatase are the major enzymes of the Calvin-Benson-Bassham cycle (Kumar et al., 2018). Under nutrient-depleted conditions, 3-PGA diverts the pathway towards PHA biosynthesis (Figure 2). Several cyanobacterial species, such as Spirulina maxima, Aphanocapsa sp, Synechocystis sp. UNIWG, Synechocystis sp. PCC 6803, Nostoc muscorum, and S. platensis accumulate PHAs (Singh and Mallick, 2017).
The synthesis of PHA governed by the following enzymes:
a) ß-ketothiolase (encoded by phaA) catalyzes the conversion of acetyl CoA to acetoacetyl-CoA, b) acetoacetyl-CoA reductase (encoded by phaB) help to reduces acetoacetyl-CoA to 3-hydroxybutyryl-CoA, and c) polymerization of 3-hydroxybutyryl-CoA to PHB carried out by PHA synthase (encoded by phaEC) (Taroncher-Oldenburg et al., 2000; Carpine et al., 2017). PHA synthase can also incorporate other hydroxy acid monomers within the PHA. PHA synthase is categorized into four groups: 1) Class I PhaC subunit contains 60–73 kDa in Ralstonia eutropha, 2) Class II PhaC subunit contains 60–65 kDa in Pseudomonas oleovorans, 3) Class III PhaC and PhaE subunit contains 40 kDa each in Allochromaticum vinosum, and Thiocapsa pfennigii, and 4) Bacillus megaterium contains 40 kDa and 22 kDa of Class IV (PhaC and PhaR) (Ray and Kalia, 2017b). Only Class III PHA synthase has been observed in cyanobacterial species (Singh and Mallick, 2017). Cyanobacterial gene locations are slightly different from other bacteria. In bacteria, all four genes are present in one single operon, but in cyanobacteria, two separate operons are present. PhaA and PhaB are putatively co-expressed and present in the first loci while PhaEC, present in the second loci (Price et al., 2020).
PHB production from CO2 is gaining interest because of its low cost of production (Carpine et al., 2017). Under nitrogen-limiting conditions, Synechocystis PCC6803 produced 4.1% PHB of the total cell dry weight (Wu et al., 2001). Synechococcus MA19, a thermophilic organism, was reported to produce 55% PHB (Nishioka et al., 2001). However, non-thermophilic cyanobacterial species produced PHB up to 20–25% (Troschl et al., 2017a; Troschl et al., 2017b) (Table 1). However, some bacterial species such as Cupriavidus eutrophus B-10646, Bacillus cereus SS105 produced 55–85% of PHA in a batch and continuous stirred tank reactor (Table 1). Supplementation of CO2 to R. eutropha B5786 led to the production of 51% of PHA in a batch fermentation system (Table 1). Some cyanobacteria produce PHB through nitrogen fixation. Calothrix species produced PHB from 17 to 25% of total cell dry mass through nitrogen fixation (Price et al., 2020). Arthrospira subsalsa is a species of filamentous cyanobacteria that produces up to 14.7% PHB under increased salinity (Shrivastav et al., 2010). Acetate addition to the system led to the enhancement, in PHB content up to 40% by another group of cyanobacteria Nostoc. Further addition of propionate and valerate to the CO2-containing medium resulted in an enhanced P (3HB-co-3HV) content (Bhati Malick, 2015). By contrast, under phosphorous limiting conditions, co-utilization of acetate and fructose enhanced the PHB production up to 38% (w/w) of cell dry mass of Synechocyctis PCC6803 (Panda and Malick, 2007). Other cyanobacteria, such as Calothrix scytonemicola TISTR 8095 produced PHB up to 356.6 mg/L in 44 days under nitrogen limitation (Kaewbai-ngam et al., 2016). Microbial mutualism have the potential role in the bioproduction of value-added byproducts. In one study, Azotobacter vinelandii, a diazotroph and S. elongates PCC7942 co-cultured for the production of PHB by fixing CO2 from the atmosphere. Here, 13C bicarbonate was added to increase the cell growth and produced 13C labeled PHB. This study proved the model for cross feeding between two organisms (Smith Francis, 2016). Co-cultures containing S. elongatus cscB and P. putida cscAB utilized CO2 for the production of PHA in two-phase systems. In the first phase, S. elongates cscB fixes and converts CO2 to sucrose via the Calvin-Benson-Bassham cycle pathway. Sucrose will then release into the culture supernatant through heterologous sucrose permease cscB activity. P. putida cscAB used sucrose as a carbon source to produced PHA up to 23.8 mg/L/h with a yield of 156 mg/L after 16 days. Here, 3-hydroxydecanoic acid was found to be the major monomer (Löwe et al., 2017). The first report of mcl-PHA production was found in co-cultures containing S. elongates and P. putida. Here P. putida was utilized as chassis for the generation of PHA by operating metabolic pathways (Löwe et al., 2017). Some photosynthetic bacteria utilize CO2 to produce PHA such as purple sulfur bacteria and purple non-sulfur bacteria. PHB homopolymer was found in purple sulfur bacteria while co-polymers such as 3HB and 3HV were found in purple non-sulfur bacteria (Higuchi-Takeuchi et al., 2016). However, from GPC analysis it was observed that photosynthetic purple bacteria produced PHAs with high molecular weight (3,000–994,000 g mol−1) which will be favorable for industrial application. The polydispersity index range was found between 1.5 and 6.7 Mn (Higuchi-Takeuchi et al., 2016). A Horizontal tubular photobioreactor was installed in an Energie-Versorgung Niederosterreich AG power company present in Austria for PHB production. Here, one ton of CO2 was used as feed for cyanobacteria and produced 115 kg PHB and biogas up to 320 m3 (Salehizadeh et al., 2020). Thus, this strategy will enhance CO2 assimilation capacity and could be a promising solution towards large-scale PHB production. Cyanobacteria can efficiently adapt to any environmental condition, which suggests that this group of microorganisms does not require any energy-rich source for their growth, and therefore, they can be exploited for the generation of valuable byproducts such as PHAs and carbohydrates (Parmar et al., 2011; Arias et al., 2020).
Synechocystis species are the model organisms studied for various value-added chemicals and biomaterial production (Price et al., 2020). They have been engineered to produce both PHAs and hydrogen. Overexpression of PHB genes in Synechocystis sp. PCC6803 increased PHB content. Here, the promoter of the RuBisCO gene was successfully employed for the expression systems in Synechocystis sp. PCC6803. Up regulation of RuBisCO activity in the presence of CO2 helps to enhance PHB production (Yu et al., 2013). C. necator PHA synthase gene was expressed in Synechococcus sp. PCC7942 and produced 25% PHB as compared to the non-recombinant strain under phototrophic and heterotrophic conditions (Price et al., 1998). PHA genes were expressed from C. necator in Synechocystis sp. PCC7002 and produced up to 52% PHA under heterotrophic conditions (Akiyama et al., 2011). Synechocystis sp. PCC 6803 was optimized to produce both the (S) and (R) configurations of 3-hydroxybutyrate by inserting two additional pathways that were introduced and produced 533.4 mg/L of PHB (Wang et al., 2013) (Table 1). Recently, Synechocystis sp. PCC6803 was engineered to enhance PHA production from CO2. Here, the focus was trying to engineer central carbon metabolism, two different target regions, phosphotransacetylase, and acetyl-CoA hydrolase were deleted in Synechocystis sp. PCC6803 to see the effect on PHB production. In addition, Bifidobacterium breve when expressed with heterologous phosphoketolase produced 12% of PHB content and 7.3 mg/L of productivity (Carpine et al., 2017) (Table 1). Microbial mutualism is beneficial for enhanced PHB production. 2.5-fold of PHB was produced by Synechocystis sp. PCC 6714 with a yield of 37% when compared with the wild-type bacteria (Kamravamanesh et al., 2018). Genetically engineered cyanobacteria Synechocystis sp. generated 1.84 g/L of PHB in 10 days by utilizing CO2 with a productivity rate of 263 mg/L/day. Here, acetoacetyl-CoA was found to be the main bottleneck for the enhanced production of PHB (Wang et al., 2018). Cyanobacterial expression vectors containing pha genes were constructed in Synechocystis sp. PCC 6803 and PHB productivity reached 12-fold higher as compared to the wild-type bacteria (Hondo et al., 2015). Synechococcus sp. PCC7942 harboring the gene from Alcaligenes eutrophus was able to produce 25% of PHB (Nikel et al., 2006). Supplementation of 10% CO2 and poultry litter with an aeration rate of 0.1 vvm to Nostoc muscorum Agardh produced 774 mg/L of PHAs (Bhatti and Mallick, 2015). Arthrospira (Spirulina) platensis MUR126 co-produced phycocyanin and polyhydroxybutyrate (PHB) under photoautotrophic and mixotrophic condition. Additional supplementation of CO2 enhanced the PHA content up to 33% with 23% biomass and 30% phycocyanin.
The material properties of cyanobacterial PHAs are gaining interest because of their biodegradation properties. Upon degradation, microorganisms break the ester bond and degrade the polymer into oligomers and monomers, whereas petroleum-based synthetic plastics are very difficult to degrade and pollute in the environment. PHB has material properties that are similar to synthetic plastics such as crystallinity, tensile strength, and melting temperature. However, PHB is more brittle than synthetic plastics. The incorporation of 3HV into the PHB chain reduces its brittleness and increases its elasticity, and it becomes tougher and more flexible. This feature makes biopolymers suitable candidates for industrial and biomedical applications. Aulosira fertilissima produced PHB and P (3HB-co-3HV), with 155–174°C melting temperature and 0.6–5.5°C of the glass transition temperature. The extracted PHB and P (3HB-co-3HV) also have Young’s modulus ranging from 1.2-3.4 to GPa, while elongation at break property varies from 4.9 to 87.2% (Singh et al., 2017).
Hydrogen-oxidizing bacteria are the defined group of bacteria that utilize H2 and O2 as an electron donor and acceptor with the help of ribulose biphosphate for fixing CO2 within the bacterial cell (Salehizadeh et al., 2020). A. eutropha is capable of converting reduce form of CO2 to PHA under different H2/CO2 stochiometric ratios. In addition, R. eutropha can produce PHB up to 75% (Khosravi-Darani et al., 2013) (Table 1). The autotrophic culture of C. necator was also checked for PHB production. The utilization of CO2 led to the production of up to 72% PHB with 60 g/L of dry cell mass (Garcia-Gonzalez and De Wever, 2018). Fed-batch cultivation of the PHA copolymer resulted in 65 g/L cell dry weight and PHBV of up to 27 mol% (Garcia-Gonzalez and De Wever, 2018). The co-utilization of CO2 and valeric acid produced 1.14 g/L of P(3HB-co-3HV) by C. necator DSM 545 under mixotrophic conditions. Here, CO2 was continuously sparged to prevent the accumulation of substrate in the medium (Ghysels et al., 2018). Acetic acid can indeed act as an indirect reservoir of CO2 for the production of both PHA homo and co-polymer. The consumption of acetic acid was found to be favorable for C. necator growth and PHA production.
Worldwide, CH4 stands as the second most greenhouse gas agent which contributes 20% towards climate change and traps atmospheric heat about 25 times higher than CO2 (Mounasamy et al., 2020). Sixty-three percent of CH4 is generated from anthropogenic activities and the remainder from coal mining, land filling, anaerobic wastewater treatment (Strong et al., 2016). In natural gas, methane counts for 90%. Due to the current world wide demand it is estimated to increase up to 44.0% by 2040 (Liu et al., 2020). Currently, CH4 accounts for 18% of the total greenhouse gas emissions (EU-28) (Cantera et al., 2018a). This situation raises concerns about global warming and has compelled researchers to look for novel approaches that can help to reduce greenhouse gases and environmental pollution. The production of PHB from CH4 may act as a sequestration process, and the biotechnological approach could be the best solution for the abatement of methane because it is cost-effective and has a low impact on the environment. Such approaches are mainly based on the biocatalytic action of microorganisms known as methanotrophs that convert CH4 into CO2 and H2O using O2 as an electron acceptor (Cantera et al., 2018a; Cantera et al., 2018b). Methanotrophs can grow on C1 carbon compounds, and some can utilize multicarbon sources as an energy source in specific environments (Semrau, 2011). The well-studied methanotrophs are aerobic methane-oxidizing bacteria that are categorized as γ-proteobacteria (Type I) and α-proteobacteria (Type II). For carbon assimilation, they follow the ribulose monophosphate pathway (RUMP) and the serine cycle (Pieja et al., 2017). The key enzyme for CH4 oxidation is methane monooxygenase which can be found in soluble or particulate forms. Methanogenesis requires two reducing equivalents for the oxidation of CH4 into CH3OH, where anaerobic methanotrophic archaea and non-methanotrophic bacterial consortia conduct the anaerobic methane oxidation.
Bioconversion of CH4 reduces nitrate, sulfur, and metals with the help of methyl-CoA reductase through reverse methanogenesis (Haynes Gonzalez, 2014; Lawton and Rosenzweig, 2016). Efficient PHB production was found in Type II methanotrophs that follow the serine pathway. Methylosinus trichosporium OB3b, Methylocystis paravus, Methylosinus trichosporium IMV3011, Methylosinus sporium, Methylocystis hirsute, Methylocystis spp. GB25 MTS, and Methylocella tundae are the most efficient PHB producers (Liu et al., 2020) (Table 2).
In a sequential step, methanotrophic bacteria oxidize CH4 to CO2 with intermediates such as methanol, formaldehyde, and formate. CH4 is oxidized to methanol by particulate and soluble methane monooxygenase. Formaldehyde is produced in the second step of oxidation. However, a high concentration of reducing equivalents is, therefore, required to convert cytosolic formaldehyde to formate during the 2nd step of oxidation. NADH+ specific and cytochrome-linked enzymes are involved in this process. In the case of type-I methanotrophs, formaldehyde fixation is catalyzed by 3-hexulosephosphate synthase and formed (D-arabino)-3-hexulose-6-phosphate, which in turn converted to different intermediates and CO2. Here, for enzyme activation Mg2+ and Mn2+ co-factors are used. In contrast, type-II methanotrophs activate formaldehyde oxidation by pterin cofactor, which is catalyzed by tetrahydrofolate (H4F) enzymes. Tetrahydrofolate enzymes supply the formaldehyde acceptor known as N5, N10 methylene-H4F, which supports the chemical reaction between formaldehyde and glycine to form serine. Subsequently, depending on nutrient availability and CO2 production type-II methanotrophs move towards TCA cycle or PHB production pathway (Figure 1).
The equation for the PHB cycle is
where FP and FPH2 is oxidized and reduced flavoprotein, respectively.
Several methanotrophs utilize CH4 and produce PHB under nutrient-limiting conditions (Laycock et al., 2013). Co-substrate addition might be helpful for higher PHA copolymer production. Furthermore, there could be a combination of biological and chemical approaches that can help to produce PHAs with improved properties. The quality of methanotrophic PHA remains compromised in large-scale industrial production and its use over synthetic plastics. The addition of C1 substrates such as formate to CH4, was capable of slowing the PHB consumption of Methylocystis parvus OBBP (Pieja et al., 2011). Under some conditions, CH3OH maintains methylotrophic activity (Khosravi-Darani et al., 2013). Methylotrophic trichosporium produces PHB by utilizing CH4 and CH3OH under short non-axenic conditions. Here, the addition of 0.1% (v/v) CH3OH maintained the oxidation level of CH4 (Zhang et al., 2008). The experiments were operated in a two-stage cultivation step to maintain the growth and produce up to 0.6 g/L of PHB. Other studies have proposed that the addition of methanol and other C1 substrates to CH4 enhances methane bioconversion, cell biomass, and PHA content (by 10–35% (w/w)) (Zhang et al., 2008) (Table 2). The addition of citric acid also resulted in enhanced PHA production because it acts as an inhibitor for the TCA cycle and accumulates twice amount of PHA as the control, even under sufficient nutrient conditions. PHB production increased from 12 to 40% (w/w) with Mw up to 1.5 × 106 Da (Zhang et al., 2008).
Furthermore, the supplementation of volatile fatty acids such as acetic acid, propionic acid, butyric acid, and valeric acid to CH4 enhanced PHB production in Methylocystis hirsuta with a yield of 10–30% (López et al., 2019) (Table 2). The co-utilization of valerate and methane led to 3HV production up to 20–40 mol% by Methylocystis hirsuta, Methylocystis parvus OBBP, and Methylosinus trichosporium (Cal et al., 2016; Myung et al., 2016). However, the co-utilization of propionate and n-pentanol did not significantly affect the 3HV content in Methylocyctis species (Cal et al., 2016; Myung et al., 2016) (Table 2). Interestingly, when co-fed under copper limitation, Valerate and CH4 enhanced the PHA and its co-polymer content up to 78% and 50 mol% (Cal et al., 2016). The addition of ω-hydroxy alkanoates to CH4 resulted in new tailor-made co-polymers by Methylocystis parvus, which has a molecular weight ranging from 4.5 × 105 to 1.5 × 106 Da (Myung et al., 2017). Here, the composition of PHA co-polymers consists of P (3HB-co-4HB), P (3HB-co-5-HV-co-3HV), and P (3HB-co-5HV-co-3HV) (Myung et al., 2016) (Table 2).
Most methanotrophic PHA production has occurred using obligate type II methanotrophs via batch fermentation. Its main function is to use pure CH4. Thus, many studies have attempted to design a bioreactor for simultaneous CH4 mitigation and PHB production. Methylocysitis was cultivated in a sequencing batch reactor and a bubble column bed reactor for the accumulation of PHB up to 20–25% (w/w) and 40–50% (w/w), respectively (Pieja et al., 2011; Garcia-Gonzalez and De wever, 2018) (Table 2). PHB production was higher in the case of the bubble column bed reactor. This might have occurred because of the internal gas-recycling system, which allows CH4 mass transfer compared to the sequencing batch reactor. PHB production by Methylocystis sp. GB25 occurred in a two-step phase in the STR with PHB production in batch fermentation (López et al., 2018) (Table 2). Microalgae Scenedesmus sp. and Methylocystis parvus OBBP converted CH4 and CO2 from synthetic biogas into PHB in a two-stage process (Van der Ha et al., 2012) and PHB accumulation was also studied in Methylocystis hirsuta by exploiting CH4 from biogas as a source (López-Neila, 2017). Another study revealed that Methylocycstis-enriched cultures utilize trace amounts of ethane, propane, and butane in natural gas and produced 42% (w/w) of PHB (Myung et al., 2016) (Table 2). Thus, utilization of co-substrates and up-gradation of reactors is desirable to raise PHA production/yield.
Methanol can be generated not only from industrial waste gas but also from biomass and CO2 hydrogenation process (Borisut and Nuchitprasittichai, 2019). CH3OH can be used as feedstock in both biological and chemical industries by methylotrophs for the sustainable production of value-added chemicals (Liao et al., 2016). Under environmental conditions, CH3OH is present in liquid form, thus, easy to handle and bio convert to PHA production. The application of methylotrophic bacteria facilitates PHA production from CH3OH.
Among others, Methylobacterium spp. is the most widely studied organism for PHB synthesis through the serine pathway. NH4+, Mg2+, PO43-, and K+ are the major deficiencies studied during the accumulation of PHB (Khosravi-Darani et al., 2013). Methylobacterium extorquens were studied for PHB and its co-polymer production from CH3OH (Bourque et al., 1992). The optimization of fed-batch fermentation led to a cell dry weight up to 115 g/L and a polymer content of up to 46% (w/w) (Bourque et al., 1992) (Table 2). The maximum PHB production using CH3OH as carbon feedstock was found to be 0.18 g/g. A recent study suggested that the addition of a co-substrate such as valeric acid, n-amyl, or propionic acid to CH3OH could be helpful in the higher production of PHB and its co-polymer (Strong et al., 2016). Enhanced PHB production of up to 136 g/L was achieved by using computer-controlled fed-batch fermentation of CH3OH with 66% cell dry weight using was obtained. It has been proposed that genetic engineering may enhance PHB productivity and yield. Methylobacterium extorquens DSMZ 1340 utilized methanol for the production of PHB. The deficiency of MgSO4 and (NH4)2SO4 in the medium stimulates PHB production, which increased up to 62.3% of total dry cell mass by Methylobacterium extorquens DSMZ 1340. After 35 h of fed-batch fermentation, the yield of PHB was 2.8 g/L/h and 0.98 g/L/h (Mokhtari-Hosseini et al., 2009a). In contrast, the growth of Methylobacterium trichosporium IMV3011 on CH3OH was tested at a feeding rate of 0.1% five times during fermentation. Hence, the concentration of PHB increased to 47.6% with 2.91 g/L cell dry weight. The addition of malic acid at the rate of 0.2 g/L enhanced to 58.5% of PHB production, with 3.32 g/L dry cell mass. Various reports have suggested that PHB production can be enhanced after the addition of acetyl-CoA, malic acid, and citric acid (Xin et al., 2011; Khosravi-Darani et al., 2013). Similarly, the addition of 0.12 g/L of NH4+ produced 7.04 g/L of PHB by Methyloligella halotolerans from methanol with a molecular weight of 8,000–10,000 kDa (Ezhov et al., 2017). Under the nutrient limitations of nitrogen and magnesium, Methylobacterium extroquens DSMZ 1340 produced 9.5 g/L of PHB with a cell dry weight of 65.3% and a yield of 0.16 g/g by utilizing CH3OH (Mokhtari-Hosseini et al., 2009b) (Table 2).
Moreover, Methylobacterium extorquens DSMZ produced 44% (w/w) of PHB by fed-batch cultivation. Recombinant technology helped to produce PHA copolymers such as 3HB, 3HV, and 3HHx within the polymer in Methylobacterium extorquens AM1 (Orita et al., 2014) (Table 2). The present study reported that CO2+ concentration in the medium influences cell growth and PHA productivity. A low concentration of CO2+ helped to achieve 3HV content up to 6 mol%. Furthermore, the pccA gene deletion increased the 3HV fraction in terpolymers (Orita et al., 2014) (Table 2).
Methylobacterium extorquens AM1 utilized CH3OH as a sole source of carbon and produced PHB up to 149 g/L (Suzuki et al., 1986). Methylobacterium extorquens ATCC55366 synthesized up to 46% (w/w) PHB by utilizing CH3OH. Moreover, under oxygen-limiting conditions and addition of 0.01 g/L of CH3OH led to the production of PHB with high molecular weight (900–1,800 kDa). The composition of media was found to be a significant approach for higher cell biomass and PHB production. The cheaper cost of methanol could, therefore, reduce the cost of PHA production when compared to pure sugar, but the yield is low for other substrates (Höfer et al., 2010) (Table 2). Moreover, the tolerance of organisms should be improved for industrial applications (Zhag et al., 2018).
Several thermal power plant and steel plant industries generate large quantities of carbon monoxide. For example, a South Korean steel company (POSCO) generates 60 million Nm3 of CO/day (Hwang et al., 2020). CO can be utilized to produce value added products. However, the processes have several limitations. Being an inert gas, the efficiency of this process is low, and the risk of the explosion has limited the use of CO (Choi et al., 2020a). It is toxic to several microorganisms but some microbes can utilize CO.
Acetobacterium woodii produced PHB using CO as a substrate in a two-phase whole-cell bio-catalytic operating system. A. woodii utilized CO and produced 53.1–60.7 mM of formate in the first step. In the second step, engineered Methylobacterium extorquens subsequently utilized formate, and the PHB content improved by up to 2.24%. The two-stage concept for the bioconversion of CO to PHB offers an effective solution for CO utilization (Hwang et al., 2020). Although, the interest in bioconversion of CO to PHA has increased recently, however, it is still in a nascent stage. Thus, much insight is required about the underlying mechanism. Once deciphered, it would be an asset for the biotechnological industries (Figure 3).
Bioconversion of C1 feed substrates to value-added compounds have attracted researcher owing to the environmental pollution and the shale gas revolution (Durre and Eikmanns, 2015; Humphreys and Minton, 2018; Sun et al., 2019). Electrochemical reduction of carbon dioxide or syngas hydration lead to the generation of formate (HCOO−) which is regarded as environmentally friendly and sustainable microbial feed substrate (Agarwal et al., 2011; Schuchmann and Muller, 2013; Hwang et al., 2015; Shinagawa et al., 2018; Hwang et al., 2020). CO2 is reduced to formate, methane, carbon monoxide, and ethylene (Whipple et al., 2010). Formate can be used as fuel in fuel cells (Joo, 2008; Enthaler et al., 2010). CO2 reduction by the electrochemical reaction can produce formic acid with high faradaic efficiency. Also, one oxygen-stable whole-cell biocatalyst is used to produce formate (Kortlever et al., 2015). It can act as a good electron mediator that has stability, non-toxicity, good permeability. Methylotrophs, non-pigmented Pseudomonas, facultative autotrophs, heterotrophs, and hypomicrobia are capable of growing on formate through serine pathway or ribulose monophosphate (RuMP) pathway. (Hwang et al., 2015) (Figure 3).
In bacteria, formate is oxidized to carbon dioxide by generating NADH (Berrios-Rivera et al., 2002; Yishai et al., 2016; Barin et al., 2018). Several reports are found on the utilization of formate by Escherichia coli using formate assimilating pathways. However, due to slow bacterial growth, the industrial application has been limited (Kim et al., 2019; Bang et al., 2020; Kim et al., 2020). On the other hand, Methylorubrum extorquens can able to utilize formate through the tetrahydrofolate pathway and serine cycle (Yishai et al., 2016; Hwang et al., 2020). Metabolic engineering of Methylorubrum extorquens has enhanced the product yield of various bioproducts from C1 feed such as PHAs, proteins, dicarboxylic acids (Bélanger et al., 2004; Choi et al., 2008; Hofer et al., 2010; Orita et al., 2014; Sonntag et al., 2014, 2015). One study reported that Methylobacterium extorquens utilized formate and produced P(3HB-co-3HV) with 8.9% HV content. Here, bktB, phaJ1, and phaC2 were heterologously expressed and effectively converted propionyl-CoA into 3-HVCoA (Yoon et al., 2021). Methylobacterium extorquens utilizes formate through the Foc A transporter (Lü et al., 2011; Hwang et al., 2020).
The effect of PHA-producing organisms on a commercial scale is indicated by the PHA yield, organism growth rate, and rate of PHB accumulation. The productivity of PHA is directly proportional to the price of the polymer. The conversion of CO2 to PHA depends mainly on the bacterial cell growth rates and cell densities of the organisms. The unavailability of a mass cultivation system is hindering the growth of commercial production of PHA from CO2. Cyanobacterial mediated PHA production is generally influenced by abiotic factors, and operational parameters (Singh et al., 2017; Singh et al., 2019). The cyanobacterial production of PHA varies significantly, and there are high chances of the simultaneous production of other value added byproducts for example, proteins and pigments. The process parameters should be constant to achieve a high PHA yield. The commercial cultivation of PHA can be achieved in two ways: 1) Photobioreactor (closed system) and 2) pond culture systems (open) (Koller and Marsalek, 2015). Some challenges remain, for example, 1) improved photoautotrophic PHA production, 2) enhanced bacterial cell biomass in the photobioreactor, 3) photobioreactors modification for scale-up purpose, 4) Optimized downstream processing, and 5) CO2 uptake capacity (Drosg et al., 2015). An ideal photobioreactor system can maintain a phototrophic culture system for the production of valuable bio-products. An advanced photon system was operated for cultivation system with an average temperature range of 15–60°C (Singh et al., 2017; Singh and Mallick, 2017). Maintaining a monoculture and its productivity is the main problem of the system. Also, compared to open pond systems photobioreactors are more expensive. There are several uncertainties due to the underdevelopment of photobioreactors and open pond systems (Singh et al., 2017). The complexity found in the harvesting and processing of biomass limits the growth of PHA towards commercialization. Harvesting is an approach that creates a bottleneck for commercial scale. Different techniques have been employed for harvesting cyanobacterial biomass, for example, filtration, centrifugation, flocculation, and gravity settling. However, because of the small size, low concentration, and colloidal stability of cyanobacterial biomass, the harvesting process is difficult to recover (Xia et al., 2017). Furthermore, the harvesting cost is 20–30% of the entire process. After the harvesting process, it is necessary to eliminate water from the wet biomass, which helps to further store cyanobacterial feedstock, and therefore, intensive energy is required for the cyanobacteria drying process. A study has reported that drying alone costs 20% of the total production cost. Air-drying is also possible for cyanobacterial biomass production, but it requires a longer time and additional storage place. However, the air-drying process could be replaced by solar and wind energy (Parmar et al., 2011).
The biotechnological approach based on CH4 bioconversion is promising and imminent, but there are some physical and biological limitations. Being a hydrophobic gas pollutant, CH4 has poor solubility in water, which results in a low elimination rate and poor biomass concentration. Uptake of CH4 gas to bacterial communities creates problems, and there is a need to search for innovative strategies that can make the process easier and more successful (Kraakman et al., 2011). Mechanical stress of the bioreactor limits the growth of methanotrophs by hindering the agitation rate (Cantera et al., 2018b). For this reason, conventional bioreactors remain limited (Muñoz et al., 2007; Kraakman et al., 2011; Estrada et al., 2012). Furthermore, an increase in both investment and operating costs is influenced by a low gas-liquid concentration gradient (Estrada et al., 2012). For microbial conversion of waste gas streams (rich in CH4) into valuable bio-products, suspended growth bioreactors are found suitable (Muñoz et al., 2007). Suspended growth membrane diffusion and pressurized bioreactors have been developed to enhance mass transport and require low-moderate energy for operation. Also, through internal gas recirculation, a high mass transfer rate of CH4 can be achieved (Estrada et al., 2014). Conversely, some environmental variables, such as culture conditions, pH, temperature, dissolved oxygen (O2) concentration, CH4 and O2 ratio, cultivation time, and the composition of cultivation media also play a major role in the CH4 conversion process (Semaru et al., 2010; Liao et al., 2016). The addition of a high salt concentration with stable pH values avoids contamination of the whole process. Co-substrates addition to the CH4 conversion process could be applied to tailor the quality of the byproducts. One study demonstrated dioxygenase enzyme for methane activation by oxidizing two methane molecules simultaneously. This approach could enhance process performance (Liao et al., 2016). In this context, “omics” technology will soon contribute to improving methanotroph-based biorefinery. Several companies, such as the Intrexon Corporation and Calysta are conducting more research and investment to find a single methanotroph capable of producing multiple value-added bioproducts (Cantera et al., 2018a; Cantera et al., 2018b). An advanced reactor set-up would certainly be helpful for enhanced bioavailability and conversion of gaseous C1 substrates.
The generation of high value-added bioproducts could be achieved by fed-batch cultivation through a controlled nutrient feed strategy in mechanically stirred fermenters to gain high bacterial cell densities (Harding et al., 2007). Syngas contain CO, that has a high affinity towards metal-containing enzymes which makes the fermentation process toxic. To avoid such toxic substances, enzymatic or chemical extraction methods are used. Although the conversion of C1 substrates to PHA seems lucrative, several limitations need to be overcome to enhance the economic status of PHA (Singh et al., 2017). An eco-friendly, cost-effective PHA recovery is required from biomass.
Using several C1 sources, PHA can be produce in both methylotrophs and autotrophs. However, the overall production cost is comparable to PHA produced from other sugar substrates due to their low utilization efficiency. This strategy can be applied to achieve three objectives: 1) Increasing the PHA content, 2) production of co-polymers, 3) enhancing the mass-transfer rate that affects the final productivity, titer of the PHA production. Several studies have researched the process engineering strategy that aiming to produce various PHAs from C1 substrates. Nutrient limiting conditions favor the production of PHA in microorganisms such as methylotrophic bacteria, a-proteobacteria or Cupriavidus species (Pérez et al., 2019). However, PHA can be degraded within the cells as a reservoir of carbon or redox balance under normal conditions. Methylosinus, Methylocystis, Methylobacterium produce 40% PHA in nutrient limiting conditions while Cupriavidus species produce more than 60% (Joo, 2008). Furthermore, along with nutrient strategy feast and famine feeding process could help to achieve high PHA content. The monomeric compositions of PHA make them stiffer and brittle that limits their application in several fields. Incorporation of co-polymers improves the flexibility, lower melting temperature, glass transition temperature, crystalinity of PHA. Thus, several precursors molecules have been employed for the production of PHA co-polymers with desired material properties and physic-chemical properties in methylotrophic and autotrophic strains. Gas-to- liquid mass transfer plays a major role in microbial PHA production from C1 gases. To improve the low mass transfer rate bubble column and vertical tubular loop reactors were employed for the production of PHA by Methylocystis hirsute using CH4 as a carbon source with a yield of 42.5% and 51.6%. The high cell density of M. hirsuta led to the production of 73.4% PHA in the bubble column reactor (Pieja et al., 2011; López et al., 2019; Mozumder et al., 2015).
Process engineering approaches have led to the production of high yield PHA from C1 resources. But, the host strain development is still facing challenging. Metabolic engineering of microbial strains for the production of high yield PHA depend upon three objectives: 1) engineering of heterotrophs that cannot utilize C1 sources but can produce PHA, 2) engineering of methylotrophs and autotrophs that do not able to produce PHA, and 3) engineering of the metabolic pathways in methylotrophs and autotrophs that produce PHAs and their co-polymers naturally. When CODH from Oligotropha carboxidovorans was introduced in C. necator H16, it produced 49.7% PHA when grew in CO as whole carbon source.
There are several microorganisms can grow efficiently on CO2 as sole carbon source but cannot produce PHA naturally. Due to the absence of Pha operon, Acetogens cannot produce PHA from C1 sources under autotrophic conditions. Although the uptake of CO and CO2 led to the conversion of Acetyl Co-A which is the building block of PHA production (Lembruger. et al., 2019; Flüchter et al., 2019). Insertion of codon optimized Pha operon from C. necator into Clostridium autoethanogenum led to the production of PHA from syngas mixture containing CO and CO2 (Joo, 2008).
The attempts to engineer methylotrophic bacteria for the production of value added bacteria have been increasing slowly. Recombinant M. extorquens AM1 produced P(99 mol%3HB-co-0.7 mol% 3HV) co-polymer and P(99 mol% 3HB-co-0.3 mol%3HHx) terpolymer under CO2+ limited conditions (Lee et al., 2015; Kalyuzhnaya et al., 2015; Pfeifenschneider et al., 2017).
Several microbes such as wild type, mesophilic, extremophilic, and genetically modified organisms have been employed for the production of PHA (Koller and Marsalek, 2015). Next-generation industrial biotechnology (NGIB) approaches help to use genetically tailored strains to enhance the industrial production of PHA. To improve the PHA production efficiency, genetic manipulation was tested from substrate uptake and utilization (Povolo et al., 2010; Chen and Jiang, 2018). CRISPR/cas9 genome has been used to enhance the PHA copolymer production. This approach will help to predetermine the monomeric composition of PHA by knocking out some targeted pathways. Also, synthetic biology approaches help to construct artificial metabolic pathways which lead to the production of high molecular weight of PHA and its co-polymers (Zhou et al., 2012). Also for downstream processing, genome editing strategy was found helpful for process involvement (Gamero et al., 2018). Engineered microbes help to convert renewable substrates to PHA is of great importance towards sustainable production (Koller et al., 2008). PHA production from CO2 using cyanobacteria as photoautotrophic cell factories has gained attention. CO2 from industrial effluent has been used to produce value-added chemicals by microbes at the same time mitigating greenhouse emissions. Optimization of photobioreactor is required to enhance the cyanobacteria cultivation. For optimization, geometric characteristics, mixing behavior, gassing/degassing performance, illumination regime are needed. A recent report revealed that Synechocytis sp. inoculated with tubular photobioreactors was found to enhance PHA production (Troschl et al., 2017a). The photobioreactor was up to 200 L. 6 gas-discharge lamps were used with light-dark cycle of 16 h/8 h. With the help of help PAR sensor, photosynthetically active radiation was measured. A degasser was used in the reactor for removal of oxygen. Probes such as CPS11D and COS51D were used to measure oxygen and pH of the reactor. Pure CO2 was injected to control pH (Troschl et al., 2017b).
Methylocystis sp. utilizes CH4 to produce 0.5 g of PHA that is higher than the heterotrophic PHA production from other carbon sources such as sugars. Gasification of organic waste led to the production of syngas which can be utilized to produce PHA by Rhodospirillum rubrum (Revelles et al., 2016).
The NGIB approach uses artificial intelligence that allows low-cost renewable substrates and wastewater for the production of PHA (Chen and Jiang, 2018). PHA production is mainly influenced by the cost of the substrates. Low-cost substrates such as household wastes, activated sludge, shale gas, or syngas could be used to lower the production cost of PHA (Nikodinovic-Runic et al., 2013). Moreover, bioconversion of renewable high substrates to PHA efficiency is the most significant approach to reduce substrate consumption. Seawater could be used as the widely available and inexhaustible water source could be used as the sustainable source to replace limited water source in next-Generation Industrial biotechnology. Extremophiles are more robust as they can sustain under a long continuous operation controlled by artificial intelligence. Artificial intelligence can be achieved by collecting big data from bioprocessing parameters followed by the machine. Extremophiles and their genetically modified strains such as acidophiles, psychrophiles, thermophiles, methanotrophs are found suitable microorganism for NGIB as they grow rapidly, resistant to contamination, and feasibility for genetic engineering (Chen and Jiang, 2018; Yin et al., 2018; Quillaguaman et al., 2006; Sedlacek et al., 2019).
PHA polymers may possess more than 160 types of monomers that endow a wide range of physical properties (Choi et al., 2020a; Alcântara et al., 2020). Also, the biocompatibility and biodegradability qualities of PHAs have attracted more attention towards the medical field and therapeutic material industries (Figure 4). Therefore, applications of PHAs range from preparing commodity products to agricultural sectors. PHAs are more suitable agents for biomedical applications because the in vivo degradation of PHA is less acidic as compared to polylactic acid and poly (lactic-co-glycolic acid), which cause local necrosis and inflammation (Choi et al., 2020b). The biomedical use of PHAs started when surgical sutures were tested using P (3HB). Sutures made of PHAs were implanted in rats and were found to remain active for a long time without any adverse effects (Shishatskaya et al., 2004). Several co-polymers of PHAs were later exploited in biomedical field in the form of heart valve scaffolds, drug delivery agents, surgical sutures, bone marrow scaffolds, stents, cardiovascular patches, orthopedic pins, adhesion barriers, tendon repair devices, wound dressings, and (Gumel et al., 2013; Ray and Kalia, 2017c; Chen and Zhang, 2018). Owing to their biocompatible nature, PHA-based scaffolds such as P(3HB-co-3HV) used in the preparation of laser-perforated biodegradable scaffold films that repair damaged tissue and enable a higher rate of cell adhesion, growth, and migration (Ellis et al., 2011) (Figure 4). The fabrication of carbon nanotubes with PHBHHx nanocomposite film was reported to support osteogenesis process (Wang et al., 2011; Wu et al., 2013) and similarly, pre-osteoblast cells development was also found by the blending of P (3HB-co-3HHx) and polycaprolactone (Puppi et al., 2017). Other unique approaches have recently been developed, for example, PHB with poly-lactide-caprolactone was used for the preparation of electrospun nanofibrous scaffolds to accelerate progression in cell cycle and prevent necrosis (Daranarong et al., 2014), and PHA microspheres were used for proliferating stem cells and osteoblast regeneration (Wei et al., 2018). Conversely, the application of PHA is focused on the regeneration of new bone by employing bone tissue engineering. A blend of 8% 3HV with 30% hydroxyapatite composition had shown suitable mechanical compressive strength with lower inflammatory response and mineralization (Galego et al., 2000). Terpolyester of PHA scaffolds enhanced osteoblast attachment, propagation, proliferation, and differentiation in nerve cells (Li et al., 2005; Wang et al., 2010). Among various PHA polyesters, P(3HB-co-3HV) was shown to be the best for bone tissue regeneration (Yang et al., 2004). The degradative PHA (3-hydroxybutyrate) has been shown to promote the growth of murine osteoblast MC3T3-E1 cells in vitro. In addition, in vivo studies on ovariectomized rats suggested the role of 3HB in preventing osteoporosis, as revealed by a bone material density and bone biomechanics study (Zhao et al., 2007). A unique PHBHHx-based microgroove surface pattern (10 µm size) influences the interfacial behaviors of bone marrow mesenchymal stem cells and differentially expressed miRNAs in comparison to smooth-surfaced PHBHHx substrates (Wang et al., 2013). PHA also strengthens the skeleton, increases the rate of calcium deposition, and lowers the level of serum osteocalcin (Zhao et al., 2007; Guyot et al., 2020). PHB/chitosan nanofibers have been helpful in cartilage tissue engineering (Sadeghi et al., 2016). These studies demonstrate the beneficial biomaterial properties of PHAs in different medical applications.
FIGURE 4. Production of biopolymers from c, substrates. Applications of polyhydroxyalkanoate (PHA) in different field.
A study reported that PHB film was used for cancer detection (Sabarinathan et al., 2018). Thus, the use of PHB films for cancer detection was time-saving and painless compared to biopsy (Sabarinathan et al., 2018). PHA can also be used as a drug carrier. PHA copolymers were used to release anticancer drug 5-fluorouracil (Choi et al., 2020a). Several periodontal disease bacteria were killed by P(3HB-co-3HV) microspheres and microcapsule containing tetracycline [151]. Electrospun fibrous meshes of PHB-polyethylene oxide were used as drug delivery agents for chlorhexidine against some pathogenic bacteria (Meng et al., 2013). Similarly, a blend of PHB-chitosan was used to deliver ketoprofen, which is an antipyretic (Lins et al., 2014). Also, 3HB derivatives of PHA polymers can be used as effective drugs against mitochondrial damage (Zhang et al., 2013). Methyl ester of the 3HB monomer has shown to be effective against Alzheimer’s and Parkinson’s diseases (Camberos-Luna et al., 2016; Camandola mattson, 2017). Hydroxy acid produced from PHA degradation has also been shown to play a major role in calcium ion stimulation in memory enhancers (Raza et al., 2020). 3HB also a degradative product of PHA has an anti-osteoporosis effect (Cao et al., 2014).
Applications of PHA have been further extended through various chemical functionalization approaches. In addition to medical use, PHAs are also suitable for food packaging, cosmetics, and agricultural applications. Produced from municipal biowastes, P (3HB-co-3HV) were electrospun to prepare bio papers for food packaging (Melendez-Rodriguez et al., 2020). Due to the high radical scavenging activity, high water vapor transmission, antioxidant and antibacterial property, PHB film can act as a biodegradable active packaging material. For example, PHB film is used in the wrapping of chilled salmon (Ma et al., 2018). PHA can be used to make straws and bottles. A degradable straw was prepared using Nodax PHA (Danimer Scientific, U.S.A.) in 2018 (Choi et al., 2020a). Recently, Nestle Co. with Danimer Scientific developed biopolymer-based bottles (Choi et al., 2020b). Moreover, several PHAs blended with different biobased materials are used in the cosmetic industry for the preparation of biobased beauty masks and sanitary napkins (Choi et al., 2020b). PHA microplastics can be used in face cleansers and toothpaste as an alternative to synthetic microplastics, which are harmful to live beings, including aquatic animals (Bouwmeester and Hollmanpeters, 2015). Recently, a sun protection product containing PHA micropowder was introduced (Unilever, U.K.) as containing the first biodegradable cosmetic ingredients derived from renewable biomass (Choi et al., 2020a). Several farmers use plastic materials for greenhouse films and protection nets, which are essential for increasing the quality of crops and protecting them from hazards. High-density polythene is a commonly used synthetic plastic material but owing to its non-biodegradability, PHA films are currently being considered. Large quantities of bags are used in the agricultural sector for fertilizers and seedlings, among others (Adane and Muleta, 2011). In this context, PHA bags have many advantages PHA polymer bags do not release any toxic chemicals into the soil.
PHAs can also be helpful in denitrification. Recently, a modified sequencing batch biofilm reactor was designed to treat landfill leachate. Here, ammonia-oxidizing bacteria and denitrifying bacteria are capable of transforming organic carbon compounds into intracellular PHA (Yin et al., 2018). In this reactor, the process of nitrification (removes NH4+ –N to produce NO2− –N), simultaneous nitrification and denitrification (removes NO2− –N), and endogenous denitrification (removes residual NO2− –N) utilized PHA as a carbon source (Yin et al., 2018). PHA films can be used to prepare biodegradable waste bags, which can contribute to reducing landfills and making the composting process more efficient. PHA films can be used in agriculture and reduce disposal costs (Winnacker, 2019). Recent developments in these areas are promising, and will certainly increase the value of PHA polymers for their useful applications in daily life. PHAs are used in 3D printing implants for humans (Rydz et al., 2019). Chemically modified PHAs such as Polyhydroxyalkanoate-g-poly (N-isopropylacrylamide) has shown thermal responsive hydrophilicties and biocompatibilities at different temperature (Ma et al., 2016). Modified PHA with EU3+ and Tb3+ possesses intense photoluminescence properties with enhanced hydrophilicity and biocompatibility (Yu et al., 2019a, Yu et al., 2019b). PHAs can be also used as nano-vaccines in drug delivery (Parlane et al., 2012).
Various companies are known for the production of PHAs. TianAN is the largest PHAs production company. PhaBuilder, Medpha, COFCO, and Bluepha have been set up to explore Next-Generation Industrial Biotechnology for low-cost PHAs production. Metabolix company was sold to Korean company CJ. RWDC and Danimer have employed recombinant R. eutropha, which can utilize fatty acids for P (3HB-co-3HHx) production. New light and Full cycle companies utilize greenhouse gas, organic wastes respectively for the production of PHAs. Recently, a global organization is known as GO! PHA has been established to produce PHAs (Table 3.).
For the microbial production of several value added bio-products, C1 sources are considered as the most promising and Next-generation carbon sources. Significant efforts have been made on the production of PHAs from C1 sources as it is eco-friendly, economical feasible and it has closed carbon cycle. Several strategies such as processes and metabolic engineering have been evolved to enhance the production efficiency of PHAs and their co-polymers in native autotrophs and methylotrophs. These strategies have extended the utilization of C1 sources for the production of PHAs. Companies like Mango materials and Newlight Technologies have already achieved the goal in producing PHAs on commercial scale from C1 carbon substrates (Joo 2008). The real motive of PHA scale up production is to collaborate with waste fermentation facilities or power plant as they emit GHGs at a substantial rate. However, these waste gases might not contain the actual composition of C1 gases, but a potential still available for the development of a bioreactor. Thus, PHA production from C1 sources can be considered as the most effective solution for the generation of plastics (Joo 2008).
Several waste substrates have been exploited for the production of PHA. However, C1 compounds are a promising feedstock for the biotechnological production of value-added chemicals. These compounds would help protect dwindling fossil fuel resources, reduce greenhouse gas emissions, and contribute to a cleaner climate. In the present scenario, it is easy to modify the microorganisms that contain the indigenous substrate pathways, for example, fixation of the CO2, degradation pathway, and CH4 oxidation. However, it is necessary to evaluate the theoretical limitations of the pathway. In this review, PHB production has studied from CO2, but the yield have been relatively low as compared to other carbon sources. To address this issue, several strategies have been developed to enhance the yield with better physicochemical properties. C1- fermenting microbes need to be engineered for the production of PHB with high yield and to tolerate other toxic gases. In addition to this, metabolic engineering approaches should be developed for the production of PHA copolymers. Life cycle assessment of C1- fermentation based biopolymer production needs to access the potential of C 1 sources mitigation and the circular economy. Furthermore, to evaluate the economic feasibility technoeconomic analysis should be done. All the discussed parameters need to be studied to replace synthetic plastics over biopolymers.
To overcome such problems, genetic engineering can be an approach. Optimizing the discovery and design of novel enzymes and pathways, modifying microorganisms, and developing innovative and efficient operating technologies will contribute towards the ultimate success of sustainable biopolymer production by utilizing C1 carbon sources. Synthetic biology and morphology engineering approaches will enhance the cell density and high volumetric productivity for process economics and suitable downstream development. To improve the thermal and mechanical properties of PHAs microorganisms are capable of utilizing various precursors through metabolic engineering approaches. Apart from this artificial intelligence helps to improve the industrial production of PHAs. To make PHAs production more economy, a co-production of PHAs with other chemicals such as ectoine, inositol, amino acids, hydroxy acids can be done. Next-Generation Industrial Biotechnology offers several opportunities for bioproduction of PHAs with fully automatic bioprocessing plants can be expected.
SR: Conceptualization, Validation, Writing-Original Draft; J-OJ: Review and Editing; IC: Review and Editing, Funding acquisition, Project administration; MK: Conceptualization, Resources, Supervision. SR wrote the manuscript. All authors have read and approved the published version of the manuscript.
This research was supported by the Basic Science Research Program through the National Research Foundation of Korea (NRF) funded by the Ministry of Education (2020R1A6A1A03044512).
The author wish to thank National Research Foundation of Korea (NRF) for providing necessary funds and support.
The authors declare that the research was conducted in the absence of any commercial or financial relationships that could be construed as a potential conflict of interest.
All claims expressed in this article are solely those of the authors and do not necessarily represent those of their affiliated organizations, or those of the publisher, the editors and the reviewers. Any product that may be evaluated in this article, or claim that may be made by its manufacturer, is not guaranteed or endorsed by the publisher.
Abe, J. O., Popoola, A. P. I., Ajenifuja, E., and Popoola, O. M. (2019). Hydrogen energy, economy and storage: Review and recommendation. Int. J. Hydrogen Energy 44, 15072–15086. doi:10.1016/j.ijhydene.2019.04.068
Adane, L., and Muleta, D. (2011). Survey on the usage of plastic bags, their disposal and adverse impacts on environment: A case study in jimma city, southwestern Ethiopia. J. Toxicol. Environ. Health. Sci. 3, 234–248. doi:10.5897/JTEHS.9000067
Agarwal, A. S., Zhai, Y., Hill, D., and Sridhar, N. (2011). The electrochemical reduction of carbon dioxide to formate/formic acid: Engineering and economic feasibility. ChemSusChem 4, 1301–1310. doi:10.1002/cssc.201100220
Akiyama, H., Okuhata, H., Onizuka, T., Kanai, S., Hirano, M., Tanaka, S., et al. (2011). Antibiotics-free stable polyhydroxyalkanoate (PHA) production from carbon dioxide by recombinant cyanobacteria. Bioresour. Technol. 102, 11039–11042. doi:10.1016/j.biortech.2011.09.058
Al-Yaeeshi, A. A., Govindan, R., and Al-Ansari, T. (2020). Techno-economic-based dynamic network design for optimum large-scale carbon dioxide utilisation in process industries. J. Clean. Prod. 275, 122974. doi:10.1016/j.jclepro.2020.122974
Alcântara, J. M. G., Distante, F., Storti, G., Moscatelli, D., Morbidelli, M., and Sponchioni, M. (2020). Current trends in the production of biodegradable bioplastics: The case of polyhydroxyalkanoates. Biotechnol. Adv. 42, 107582. doi:10.1016/j.biotechadv.2020.107582
Arias, D. M., García, J., and Uggetti, E. (2020). Production of polymers by cyanobacteria grown in wastewater: Current status, challenges and future perspectives. N. Biotechnol. 55, 46–57. doi:10.1016/j.nbt.2019.09.001
Asenjo, J. A., and Suk, J. S. (1986). Microbial conversion of methane into poly-β-hydroxybutyrate (PHB): Growth and intracellular product accumulation in a type II methanotroph. J. Ferment. Technol. 64, 271–278. doi:10.1016/0385-6380(86)90118-4
Babel, W. (1992). Pecularities of methylotrophs concerning overflow metabolism, especially the synthesis of polyhydroxyalkanoates. FEMS Microbiol. Lett. 103, 141–148. doi:10.1111/j.1574-6968.1992.tb05831.x
Bang, J., Hwang, C. H., Ahn, J. H., Lee, J. A., and Lee, S. Y. (2020). Escherichia coli is engineered to grow on CO2 and formic acid. Nat. Microbiol. 5, 1459–1463. doi:10.1038/s41564-020-00793-9
Barin, R., Biria, D., Rashid-Nadimi, S., and Asadollahi, M. A. (2018). Enzymatic CO2 reduction to formate by formate dehydrogenase from Candida boidinii coupling with direct electrochemical regeneration of NADH. J. CO2 Util. 28, 117–125. doi:10.1016/j.jcou.2018.09.020
Bélanger, L., Figueira, M. M., Bourque, D., Morel, L., Béland, M., Laramée, L., et al. (2004). Production of heterologous protein by Methylobacterium extorquens in high cell density fermentation. FEMS Microbiol. Lett. 231, 197–204. doi:10.1016/S0378-1097(03)00956-X
Berrıos-Rivera, S. J., Bennett, G. N., and San, K. Y. (2002). Metabolic engineering of Escherichia coli: Increase of NADH availability by overexpressing an NAD+-dependent formate dehydrogenase. Metab. Eng. 4, 217–229. doi:10.1006/mben.2002.0227
Bhati, R., and Mallick, N. (2016). Carbon dioxide and poultry waste utilization for production of polyhydroxyalkanoate biopolymers by Nostoc muscorum Agardh: A sustainable approach. J. Appl. Phycol. 28, 161–168. doi:10.1007/s10811-015-0573-x
Bhati, R., and Mallick, N. (2015). Poly(3-hydroxybutyrate-co-3-hydroxyvalerate) copolymer production by the diazotrophic cyanobacterium Nostoc muscorum Agardh: Process optimization and polymer characterization. Algal Res. 7, 78–85. doi:10.1016/j.algal.2014.12.003
Blankenship, R. E. (2010). Early evolution of photosynthesis. Plant Physiol. 154, 434–438. doi:10.1104/pp.110.161687
Bordel, S., Rodríguez, E., and Muñoz, R. (2019). Genome sequence of Methylocystis hirsuta CSC1, a polyhydroxyalkanoate producing methanotroph. MicrobiologyOpen 8, e00771. doi:10.1002/mbo3.771
Borisut, P., and Nuchitprasittichai, A. (2019). Methanol production via CO2 hydrogenation: Sensitivity analysis and simulation-based optimization. Front. Energy Res. 7, 81. doi:10.3389/fenrg.2019.00081
Bourque, D., Ouellette, B., André, G., and Groleau, D. (1992). Production of poly-β-hydroxybutyrate from methanol: Characterization of a new isolate of Methylobacterium extorquens. Appl. Microbiol. Biotechnol. 37, 7–12. doi:10.1007/BF00174194
Bourque, D., Pomerleau, Y., and Groleau, D. (1995). High-cell-density production of poly-β-hydroxybutyrate (PHB) from methanol by Methylobacterium extorquens: Production of high-molecular-mass PHB. Appl. Microbiol. Biotechnol. 44, 367–376. doi:10.1007/BF00169931
Bouwmeester, H., Hollman, P. C., and Peters, R. J. (2015). Potential health impact of environmentally released micro-and nanoplastics in the human food production chain: Experiences from nanotoxicology. Environ. Sci. Technol. 49, 8932–8947. doi:10.1021/acs.est.5b01090
Bugnicourt, E., Cinelli, P., Lazzeri, A., and Alvarez, V. A. (2014). Polyhydroxyalkanoate (PHA): Review of synthesis, characteristics, processing and potential applications in packaging. Express Polym. Lett. 8, 791–808. doi:10.3144/expresspolymlett.2014.82
Cal, A. J., Sikkema, W. D., Ponce, M. I., Franqui-Villanueva, D., Riiff, T. J., Orts, W. J., et al. (2016). Methanotrophic production of polyhydroxybutyrate-co-hydroxyvalerate with high hydroxyvalerate content. Int. J. Biol. Macromol. 87, 302–307. doi:10.1016/j.ijbiomac.2016.02.056
Camandola, S., and Mattson, M. P. (2017). Brain metabolism in health, aging, and neurodegeneration. EMBO J. 3, 1474–1492. doi:10.15252/embj.201695810
Camberos-Luna, L., Gerónimo-Olvera, C., Montiel, T., Rincon-Heredia, R., and Massieu, L. (2016). The ketone body, β-hydroxybutyrate stimulates the autophagic flux and prevents neuronal death induced by glucose deprivation in cortical cultured neurons. Neurochem. Res. 41, 600–609. doi:10.1007/s11064-015-1700-4
Cantera, S., Muñoz, R., Lebrero, R., López, J. C., Rodríguez, Y., and García-Encina, P. A. (2018a). Technologies for the bioconversion of methane into more valuable products. Curr. Opin. Biotechnol. 50, 128–135. doi:10.1016/j.copbio.2017.12.021
Cantera, S., Sánchez-Andrea, I., Lebrero, R., García-Encina, P. A., Stams, A. J. M., and Muñoz, R. (2018b). Multi-production of high added market value metabolites from diluted methane emissions via methanotrophic extremophiles. Bioresour. Technol. 267, 401–407. doi:10.1016/j.biortech.2018.07.057
Cao, Q., Zhang, J., Liu, H., Wu, Q., Chen, J., and Chen, G. Q. (2014). The mechanism of anti-osteoporosis effects of 3-hydroxybutyrate and derivatives under simulated microgravity. Biomaterials 35, 8273–8283. doi:10.1016/j.biomaterials.2014.06.020
Carpine, R., Du, W., Olivieri, G., Pollio, A., Hellingwerf, K. J., Marzocchella, A., et al. (2017). Genetic engineering of Synechocystis sp. PCC6803 for poly-β-hydroxybutyrate overproduction. Algal Res. 25, 117–127. doi:10.1016/j.algal.2017.05.013
Carr, N. G. (1996). The occurrence of poly-β-hydroxybutyrate in the blue-green alga, Chlorogloea fritschii. Biochimica Biophysica Acta - Biophysics Incl. Photosynth. 120, 308–310. doi:10.1016/0926-6585(66)90353-0
Chen, G. Q., and Jiang, X. R. (2018). Engineering microorganisms for improving polyhydroxyalkanoate biosynthesis. Curr. Opin. Biotechnol. 53, 20–25. doi:10.1016/j.copbio.2017.10.008
Chen, G. Q., and Wu, Q. (2005). The application of polyhydroxyalkanoates as tissue engineering materials. Biomaterials 26, 6565–6578. doi:10.1016/j.biomaterials.2005.04.036
Chen, G. Q., and Zhang, J. (2018). Microbial polyhydroxyalkanoates as medical implant biomaterials. Artif. Cells Nanomed. Biotechnol. 46, 1–18. doi:10.1080/21691401.2017.1371185
Chidambarampadmavathy, K., Karthikeyan, O. P., Huerlimann, R., Maes, G. E., and Heimann, K. (2017). Response of mixed methanotrophic consortia to different methane to oxygen ratios. Waste Manag. 61, 220–228. doi:10.1016/j.wasman.2016.11.007
Choi, S. Y., Cho, I. J., Lee, Y., Kim, Y. J., Kim, K. J., and Lee, S. Y. (2020a). Microbial polyhydroxyalkanoates and nonnatural polyesters. Adv. Mat. 32, 1907138. doi:10.1002/adma.201907138
Choi, S. Y., Rhie, M. N., Kim, H. T., Joo, J. C., Cho, I. J., Son, J., et al. (2020b). Metabolic engineering for the synthesis of polyesters: A 100-year journey from polyhydroxyalkanoates to non-natural microbial polyesters. Metab. Eng. 58, 47–81. doi:10.1016/j.ymben.2019.05.009
Choi, Y. J., Gringorten, J. L., Bélanger, L., Morel, L., Bourque, D., Masson, L., et al. (2008). Production of an insecticidal crystal protein from Bacillus thuringiensis by the methylotroph Methylobacterium extorquens. Appl. Environ. Microbiol. 74, 5178–5182. doi:10.1128/AEM.00598-08
Claassens, N. J. (2017). A warm welcome for alternative CO2 fixation pathways in microbial biotechnology. Microb. Biotechnol. 10, 31–34. doi:10.1111/1751-7915.12456
Dalton, H. (1980). “Metabolic and molecular aspects of methylotrophs,” in Microbial growth on C1 compounds: proceedings of the third international symposium held in Sheffield, UK, 12-16 August 1980.
Daranarong, D., Chan, R. T. H., Wanandy, N. S., Molloy, R., Punyodom, W., and Foster, L. J. R. (2014). Electrospun polyhydroxybutyrate and poly(L-lactide-co-ε-caprolactone) composites as nanofibrous scaffolds. Biomed. Res. Int. 2014, 1–12. doi:10.1155/2014/741408
Drosg, B., Fritz, I., Gattermayr, F., and Silvestrini, L. (2015). Photo-autotrophic production of poly (hydroxyalkanoates) in cyanobacteria. Chem. Biochem. Eng. Q. 29, 145–156. doi:10.15255/CABEQ.2014.2254
Durall, C., and Lindblad, P. (2015). Mechanisms of carbon fixation and engineering for increased carbon fixation in cyanobacteria. Algal Res. 11, 263–270. doi:10.1016/j.algal.2015.07.002
Dürre, P., and Eikmanns, B. J. (2015). C1-carbon sources for chemical and fuel production by microbial gas fermentation. Curr. Opin. Biotechnol. 35, 63–72. doi:10.1016/j.copbio.2015.03.008
Ellis, G., Cano, P., Jadraque, M., Martín, M., López, L., Núñez, T., et al. (2011). Laser microperforated biodegradable microbial polyhydroxyalkanoate substrates for tissue repair strategies: An infrared microspectroscopy study. Anal. Bioanal. Chem. 399, 2379–2388. doi:10.1007/s00216-011-4653-8
Enthaler, S., von Langermann, J., and Schmidt, T. (2010). Carbon dioxide and formic acid-the couple for environmental-friendly hydrogen storage? Energy Environ. Sci. 3, 1207–1217. doi:10.1039/B907569K
Estrada, J. M., Lebrero, R., Quijano, G., Pérez, R., Figueroa-González, I., García-Encina, P. A., et al. (2014). Methane abatement in a gas-recycling biotrickling filter: Evaluating innovative operational strategies to overcome mass transfer limitations. Chem. Eng. J. 253, 385–393. doi:10.1016/j.cej.2014.05.053
Estrada, J. M., Rodríguez, E., Quijano, G., and Muñoz, R. (2012). Influence of gaseous VOC concentration on the diversity and biodegradation performance of microbial communities. Bioprocess Biosyst. Eng. 35, 1477–1488. doi:10.1007/s00449-012-0737-x
Ezhov, V. A., Doronina, N. V., Shmareva, M. N., and Trotsenko, Y. A. (2017). Synthesis of high-molecular-mass polyhydroxybutyrate from methanol in Methyloligella halotolerans C2. Appl. Biochem. Microbiol. 53, 47–51. doi:10.1134/S0003683817010112
Fernandes, J. G., Correia, D. M., Botelho, G., Padrão, J., Dourado, F., Ribeiro, C., et al. (2014). PHB-PEO electrospun fiber membranes containing chlorhexidine for drug delivery applications. Polym. Test. 34, 64–71. doi:10.1016/j.polymertesting.2013.12.007
Flüchter, S., Follonier, S., Schiel-Bengelsdorf, B., Bengelsdorf, F. R., Zinn, M., and Dürre, P. (2019). Anaerobic production of poly (3-hydroxybutyrate) and its precursor 3-hydroxybutyrate from synthesis gas by autotrophic clostridia. Biomacromolecules 20 (9), 3271–3282. doi:10.1021/acs.biomac.9b00342
Galego, N., Rozsa, C., Sánchez, R., Fung, J., Analı́a, V., and Santo Tomás, J. (2000). Characterization and application of poly(β-hydroxyalkanoates) family as composite biomaterials. Polym. Test. 19, 485–492. doi:10.1016/S0142-9418(99)00011-2
Gamero, J. E. R., Favaro, L., Pizzocchero, V., Lomolino, G., Basaglia, M., and Casella, S. (2018). Nuclease expression in efficient polyhydroxyalkanoates-producing bacteria could yield cost reduction during downstream processing. Bioresour. Technol. 261, 176–181. doi:10.1016/j.biortech.2018.04.021
Garcia-Gonzalez, L., and De Wever, H. (2018). Acetic acid as an indirect sink of CO2 for the synthesis of polyhydroxyalkanoates (PHA): Comparison with PHA production processes directly using CO2 as feedstock. Appl. Sci. (Basel). 8, 1416. doi:10.3390/app8091416
Ghatnekar, M. S., Pai, J. S., and Ganesh, M. (2002). Production and recovery of poly-3-hydroxybutyrate from Methylobacterium sp. V49. J. Chem. Technol. Biotechnol. 77, 444–448. doi:10.1002/jctb.570
Ghysels, S., Mozumder, M. S. I., De Wever, H., Volcke, E. I. P., and Garcia-Gonzalez, L. (2018). Targeted poly(3-hydroxybutyrate-co-3-hydroxyvalerate) bioplastic production from carbon dioxide. Bioresour. Technol. 249, 858–868. doi:10.1016/j.biortech.2017.10.081
Gopi, K., Balaji, S., and Muthuvelan, B. (2014). Isolation purification and screening of biodegradable polymer PHB producing cyanobacteria from marine and fresh water resources. Iran. J. Energy. Environ. 5, 94–100. doi:10.5829/idosi.ijee.2014.05.01.14
Gumel, A. M., Annuar, M. S. M., and Chisti, Y. (2013). Recent advances in the production, recovery and applications of polyhydroxyalkanoates. J. Polym. Environ. 21, 580–605. doi:10.1007/s10924-012-0527-1
Gupta, A., Bhagwat, S. G., and Sainis, J. K. (2013). Synechococcus elongatus PCC 7942 is more tolerant to chromate as compared to Synechocystis sp. PCC 6803. Biometals 26, 309–319. doi:10.1007/s10534-013-9614-6
Guyot, A. C., Leuxe, C., Disdier, C., Oumata, N., Costa, N., Le Roux, G., et al. (2020). A small compound targeting prohibitin with potential interest for cognitive deficit rescue in aging mice and tau pathology treatment. Sci. Rep. 10, 1143. doi:10.1038/s41598-020-57560-3
Harding, K. G., Dennis, J. S., Von Blottnitz, H., and Harrison, S. T. L. (2007). Environmental analysis of plastic production processes: Comparing petroleum-based polypropylene and polyethylene with biologically-based poly-β-hydroxybutyric acid using life cycle analysis. J. Biotechnol. 130, 57–66. doi:10.1016/j.jbiotec.2007.02.012
Haynes, C. A., and Gonzalez, R. (2014). Rethinking biological activation of methane and conversion to liquid fuels. Nat. Chem. Biol. 10, 331–339. doi:10.1038/nchembio.1509
Helm, J., Wendlandt, K. D., Jechorek, M., and Stottmeister, U. (2008). Potassium deficiency results in accumulation of ultra-high molecular weight poly-β-hydroxybutyrate in a methane-utilizing mixed culture. J. Appl. Microbiol. 105, 1054–1061. doi:10.1111/j.1365-2672.2008.03831.x
Helm, J., Wendlandt, K. D., Rogge, G., and Kappelmeyer, U. (2006). Characterizing a stable methane-utilizing mixed culture used in the synthesis of a high-quality biopolymer in an open system. J. Appl. Microbiol. 101, 387–395. doi:10.1111/j.1365-2672.2006.02960.x
Higuchi-Takeuchi, M., Morisaki, K., Toyooka, K., and Numata, K. (2016). Synthesis of high-molecular-weight polyhydroxyalkanoates by marine photosynthetic purple bacteria. PLoS One 11, e0160981. doi:10.1371/journal.pone.0160981
Höfer, P., Choi, Y. J., Osborne, M. J., Miguez, C. B., Vermette, P., and Groleau, D. (2010). Production of functionalized polyhydroxyalkanoates by genetically modified Methylobacterium extorquens strains. Microb. Cell Fact. 9, 70–13. doi:10.1186/1475-2859-9-70
Hondo, S., Takahashi, M., Osanai, T., Matsuda, M., Hasunuma, T., Tazuke, A., et al. (2015). Genetic engineering and metabolite profiling for overproduction of polyhydroxybutyrate in cyanobacteria. J. Biosci. Bioeng. 120, 510–517. doi:10.1016/j.jbiosc.2015.03.004
Humphreys, C. M., and Minton, N. P. (2018). Advances in metabolic engineering in the microbial production of fuels and chemicals from C1 gas. Curr. Opin. Biotechnol. 50, 174–181. doi:10.1016/j.copbio.2017.12.023
Hwang, H. W., Yoon, J., Min, K., Kim, M. S., Kim, S. J., Cho, D. H., et al. (2020). Two-stage bioconversion of carbon monoxide to biopolymers via formate as an intermediate. Chem. Eng. J. 389, 124394. doi:10.1016/j.cej.2020.124394
Hwang, H., Yeon, Y. J., Lee, S., Choe, H., Jang, M. G., Cho, D. H., et al. (2015). Electro-biocatalytic production of formate from carbon dioxide using an oxygen-stable whole cell biocatalyst. Bioresour. Technol. 185, 35–39. doi:10.1016/j.biortech.2015.02.086
Joó, F. (2008). Breakthroughs in hydrogen storage-formic acid as a sustainable storage material for hydrogen. ChemSusChem 1, 805–808. doi:10.1002/cssc.200800133
Kaewbai-ngam, A., Incharoensakdi, A., and Monshupanee, T. (2016). Increased accumulation of polyhydroxybutyrate in divergent cyanobacteria under nutrient-deprived photoautotrophy: An efficient conversion of solar energy and carbon dioxide to polyhydroxybutyrate by Calothrix scytonemicola TISTR 8095. Bioresour. Technol. 212, 342–347. doi:10.1016/j.biortech.2016.04.035
Kalia, V. C., Patel, S. K. S., Shanmugam, R., and Lee, J. K. (2021). Polyhydroxyalkanoates: Trends and advances toward biotechnological applications. Bioresour. Technol. 326, 124737. doi:10.1016/j.biortech.2021.124737
Kalyuzhnaya, M. G., Puri, A. W., and Lidstrom, M. E. (2015). Metabolic engineering in methanotrophic bacteria. Metab. Eng. 29, 142–152. doi:10.1016/j.ymben.2015.03.010
Kamravamanesh, D., Kovacs, T., Pflügl, S., Druzhinina, I., Kroll, P., Lackner, M., et al. (2018). Increased poly-β-hydroxybutyrate production from carbon dioxide in randomly mutated cells of cyanobacterial strain Synechocystis sp. PCC 6714: Mutant generation and characterization. Bioresour. Technol. 266, 34–44. doi:10.1016/j.biortech.2018.06.057
Kamravamanesh, D., Pflügl, S., Nischkauer, W., Limbeck, A., Lackner, M., and Herwig, C. (2017). Photosynthetic poly-β-hydroxybutyrate accumulation in unicellular cyanobacterium Synechocystis sp. PCC 6714. Amb. Express 7, 143. doi:10.1186/s13568-017-0443-9
Karthikeyan, O. P., Chidambarampadmavathy, K., Cirés, S., and Heimann, K. (2015). Review of sustainable methane mitigation and biopolymer production. Crit. Rev. Environ. Sci. Technol. 45, 1579–1610. doi:10.1080/10643389.2014.966422
Khosravi-Darani, K., Mokhtari, Z. B., Amai, T., and Tanaka, K. (2013). Microbial production of poly(hydroxybutyrate) from C1 carbon sources. Appl. Microbiol. Biotechnol. 97, 1407–1424. doi:10.1007/s00253-012-4649-0
Kim, S. J., Yoon, J., Im, D. K., Kim, Y. H., and Oh, M. K. (2019). Adaptively evolved Escherichia coli for improved ability of formate utilization as a carbon source in sugar-free conditions. Biotechnol. Biofuels 12, 207–212. doi:10.1186/s13068-019-1547-z
Kim, S., Lindner, S. N., Aslan, S., Yishai, O., Wenk, S., Schann, K., et al. (2020). Growth of E. coli on formate and methanol via the reductive glycine pathway. Nat. Chem. Biol. 16, 538–545. doi:10.1038/s41589-020-0473-5
Kim, S. W., Kim, P., Lee, H. S., and Kim, J. H. (1996). High production of poly-β-hydroxybutyrate (PHB) from Methylobacterium organophilum under potassium limitation. Biotechnol. Lett. 18, 25–30. doi:10.1007/BF00137805
Koch, M., Doello, S., Gutekunst, K., and Forchhammer, K. (2019). PHB is produced from glycogen turn-over during nitrogen starvation in Synechocystis sp. PCC 6803. Int. J. Mol. Sci. 20 (8), 1942. doi:10.3390/ijms20081942
Koller, M., Bona, R., Chiellini, E., Fernandes, E. G., Horvat, P., Kutschera, C., et al. (2008). Polyhydroxyalkanoate production from whey by Pseudomonas hydrogenovora. Bioresour. Technol. 99, 4854–4863. doi:10.1016/j.biortech.2007.09.049
Koller, M., and Marsalek, L. (2015). Cyanobacterial polyhydroxyalkanoate production: Status quo and quo vadis? Curr. Biotechnol. 4, 464–480. doi:10.2174/2211550104666150917010849
Kortlever, R., Peters, I., Koper, S., and Koper, M. T. (2015). Electrochemical CO2 reduction to formic acid at low overpotential and with high faradaic efficiency on carbon-supported bimetallic Pd–Pt nanoparticles. ACS Catal. 5, 3916–3923. doi:10.1021/acscatal.5b00602
Kraakman, N. J. R., Rocha-Rios, J., and Van Loosdrecht, M. C. M. (2011). Review of mass transfer aspects for biological gas treatment. Appl. Microbiol. Biotechnol. 91, 873–886. doi:10.1007/s00253-011-3365-5
Kumar, M., Gupta, A., and Thakur, I. S. (2016). Carbon dioxide sequestration by chemolithotrophic oleaginous bacteria for production and optimization of polyhydroxyalkanoate. Bioresour. Technol. 213, 249–256. doi:10.1016/j.biortech.2016.02.038
Kumar, M., Sundaram, S., Gnansounou, E., Larroche, C., and Thakur, I. S. (2018). Carbon dioxide capture, storage and production of biofuel and biomaterials by bacteria: A review. Bioresour. Technol. 247, 1059–1068. doi:10.1016/j.biortech.2017.09.050
Kumar, P., Patel, S. K. S., Lee, J. K., and Kalia, V. C. (2013). Extending the limits of Bacillus for novel biotechnological applications. Biotechnol. Adv. 31, 1543–1561. doi:10.1016/j.biotechadv.2013.08.007
Kumar, P., Ray, S., and Kalia, V. C. (2016). Production of co-polymers of polyhydroxyalkanoates by regulating the hydrolysis of biowastes. Bioresour. Technol. 200, 413–419. doi:10.1016/j.biortech.2015.10.045
Kumar, P., Ray, S., Patel, S. K., Lee, J. K., and Kalia, V. C. (2015). Bioconversion of crude glycerol to polyhydroxyalkanoate by Bacillus thuringiensis under non-limiting nitrogen conditions. Int. J. Biol. Macromol. 78, 9–16. doi:10.1016/j.ijbiomac.2015.03.046
Lawton, T. J., and Rosenzweig, A. C. (2016). Methane-oxidizing enzymes: An upstream problem in biological gas-to-liquids conversion. J. Am. Chem. Soc. 138, 9327–9340. doi:10.1021/jacs.6b04568
Laycock, B., Halley, P., Pratt, S., Werker, A., and Lant, P. (2013). The chemomechanical properties of microbial polyhydroxyalkanoates. Prog. Polym. Sci. 38, 536–583. doi:10.1016/j.progpolymsci.2012.06.003
Lee, C. S., Lee, S. A., Ko, S. R., Oh, H. M., and Ahn, C. Y. (2015). Effects of photoperiod on nutrient removal, biomass production, and algal-bacterial population dynamics in lab-scale photobioreactors treating municipal wastewater. Water Res. 68, 680–691. doi:10.1016/j.watres.2014.10.029
Li, J., Yun, H., Gong, Y., Zhao, N., and Zhang, X. (2005). Effects of surface modification of poly (3-hydroxybutyrate-co-3-hydroxyhexanoate) (PHBHHx) on physicochemical properties and on interactions with MC3T3-E1 cells. J. Biomed. Mat. Res. A 75A, 985–998. doi:10.1002/jbm.a.30504
Liao, J. C., Mi, L., Pontrelli, S., and Luo, S. (2016). Fuelling the future: Microbial engineering for the production of sustainable biofuels. Nat. Rev. Microbiol. 14, 288–304. doi:10.1038/nrmicro.2016.32
Lins, L. C., Bazzo, G. C., Barreto, P. L. M., and Pires, A. T. N. (2014). Composite PHB/chitosan microparticles obtained by spray drying: Effect of chitosan concentration and crosslinking agents on drug relesase. J. Braz. Chem. Soc. 25, 1462–1471. doi:10.5935/0103-5053.20140129
Liu, L. Y., Xie, G. J., Xing, D. F., Liu, B. F., Ding, J., and Ren, N. Q. (2020). Biological conversion of methane to polyhydroxyalkanoates: Current advances, challenges, and perspectives. Environ. Sci. Ecotechnol. 2, 100029. doi:10.1016/j.ese.2020.100029
López, J. C., Merchán, L., Lebrero, R., and Muñoz, R. (2018). Feast-famine biofilter operation for methane mitigation. J. Clean. Prod. 170, 108–118. doi:10.1016/j.jclepro.2017.09.157
López, J. C., Rodríguez, Y., Pérez, V., Lebrero, R., and Muñoz, R. (2019). “CH 4-based polyhydroxyalkanoate production: A step further towards a sustainable bioeconomy,” in Biotechnological Applications of Polyhydroxyalkanoates. (Singapore: Springer), 283–321. doi:10.1007/978-981-13-3759-8_11
López-Neila, J. C. (2017). Methane abatement biotechnologies: Targeting process microbiology, improyement of process perrformance and revalorization. Spain: Bucle. doi:10.35376/10324/28623
Löwe, H., Hobmeier, K., Moos, M., Kremling, A., and Pflüger-Grau, K. (2017). Photoautotrophic production of polyhydroxyalkanoates in a synthetic mixed culture of Synechococcus elongatus cscB and Pseudomonas putida cscAB. Biotechnol. Biofuels 10, 190. doi:10.1186/s13068-017-0875-0
Lü, W., Du, J., Wacker, T., Gerbig-Smentek, E., Andrade, S. L., and Einsle, O. (2011). pH-dependent gating in a FocA formate channel. Science 332, 352–354. doi:10.1126/science.1199098
Ma, Y. M., Wei, D. X., Yao, H., Wu, L. P., and Chen, G. Q. (2016). Synthesis, characterization and application of thermoresponsive polyhydroxyalkanoate-graft-poly (N-isopropylacrylamide). Biomacromolecules 17, 2680–2690. doi:10.1021/acs.biomac.6b00724
Ma, Y., Li, L., and Wang, Y. (2018). Development of PLA-PHB-based biodegradable active packaging and its application to salmon. Packag. Technol. Sci. 31, 739–746. doi:10.1002/pts.2408
Maheshwari, N., Kumar, M., Thakur, I. S., and Srivastava, S. (2018). Production, process optimization and molecular characterization of polyhydroxyalkanoate (PHA) by CO2 sequestering B. cereus SS105. Bioresour. Technol. 254, 75–82. doi:10.1016/j.biortech.2018.01.002
Mata, T. M., Martins, A. A., and Caetano, N. S. (2010). Microalgae for biodiesel production and other applications: A review. Renew. Sustain. Energy Rev. 14, 217–232. doi:10.1016/j.rser.2009.07.020
Melendez-Rodriguez, B., Torres-Giner, S., Lorini, L., Valentino, F., Sammon, C., Cabedo, L., et al. (2020). Valorization of municipal biowaste into electrospun poly (3-hydroxybutyrate-co-3-hydroxyvalerate) biopapers for food packaging applications. ACS Appl. Bio Mat. 3, 6110–6123. doi:10.1021/acsabm.0c00698
Meng, D., Francis, L., Thompson, I. D., Mierke, C., Huebner, H., Amtmann, A., et al. (2013). Tetracycline-encapsulated P (3HB) microsphere-coated 45S5 Bioglass®-based scaffolds for bone tissue engineering. J. Mat. Sci. Mat. Med. 24, 2809–2817. doi:10.1007/s10856-013-5012-4
Mokhtari-Hosseini, Z. B., Vasheghani-Farahani, E., Heidarzadeh-Vazifekhoran, A., Shojaosadati, S. A., Karimzadeh, R., and Darani, K. K. (2009a). Statistical media optimization for growth and PHB production from methanol by a methylotrophic bacterium. Bioresour. Technol. 100, 2436–2443. doi:10.1016/j.biortech.2008.11.024
Mokhtari-Hosseini, Z. B., Vasheghani-Farahani, E., Shojaosadati, S. A., Karimzadeh, R., and Heidarzadeh-Vazifekhoran, A. (2009b). Effect of feed composition on PHB production from methanol by HCDC of Methylobacterium extorquens (DSMZ 1340). J. Chem. Technol. Biotechnol. 84, 1136–1139. doi:10.1002/jctb.2145
Mounasamy, V., Mani, G. K., Ponnusamy, D., Tsuchiya, K., Reshma, P. R., Prasad, A. K., et al. (2020). Investigation on CH4 sensing characteristics of hierarchical V2O5 nanoflowers operated at relatively low temperature using chemiresistive approach. Anal. Chim. Acta X. 1106, 148–160. doi:10.1016/j.aca.2020.01.060
Mozejko-Ciesielska, J., Pokoj, T., and Ciesielski, S. (2018). Transcriptome remodeling of Pseudomonas putida KT2440 during mcl-PHAs synthesis: Effect of different carbon sources and response to nitrogen stress. J. Ind. Microbiol. Biotechnol. 45, 433–446. doi:10.1007/s10295-018-2042-4
Mozumder, M. S. I., Garcia-Gonzalez, L., De Wever, H., and Volcke, E. I. (2015). Poly (3-hydroxybutyrate)(PHB) production from CO2: Model development and process optimization. Biochem. Eng. J. 98, 107–116. doi:10.1016/j.bej.2015.02.031
Muñoz, R., Meier, L., Diaz, I., and Jeison, D. (2015). A review on the state-of-the-art of physical/chemical and biological technologies for biogas upgrading. Rev. Environ. Sci. Biotechnol. 14, 727–759. doi:10.1007/s11157-015-9379-1
Muñoz, R., Villaverde, S., Guieysse, B., and Revah, S. (2007). Two-phase partitioning bioreactors for treatment of volatile organic compounds. Biotechnol. Adv. 25, 410–422. doi:10.1016/j.biotechadv.2007.03.005
Myung, J., Flanagan, J. C. A., Waymouth, R. M., and Criddle, C. S. (2017). Expanding the range of polyhydroxyalkanoates synthesized by methanotrophic bacteria through the utilization of omega-hydroxyalkanoate co-substrates. Amb. Express 7, 118. doi:10.1186/s13568-017-0417-y
Myung, J., Flanagan, J. C. A., Waymouth, R. M., and Criddle, C. S. (2016). Methane or methanol-oxidation dependent synthesis of poly(3-hydroxybutyrate-co-3-hydroxyvalerate) by obligate type II methanotrophs. Process Biochem. 51, 561–567. doi:10.1016/j.procbio.2016.02.005
Myung, J., Galega, W. M., Van Nostrand, J. D., Yuan, T., Zhou, J., and Criddle, C. S. (2015). Long-term cultivation of a stable Methylocystis-dominated methanotrophic enrichment enabling tailored production of poly(3-hydroxybutyrate-co-3-hydroxyvalerate). Bioresour. Technol. 198, 811–818. doi:10.1016/j.biortech.2015.09.094
Nikel, P. I., Pettinari, M. J., Galvagno, M. A., and Méndez, B. S. (2006). Poly(3-hydroxybutyrate) synthesis by recombinant Escherichia coli arcA mutants in microaerobiosis. Appl. Environ. Microbiol. 72, 2614–2620. doi:10.1128/AEM.72.4.2614-2620.2006
Nikodinovic-Runic, J., Guzik, M., Kenny, S. T., Babu, R., Werker, A., and Connor, K. E. (2013). Carbon-rich wastes as feedstocks for biodegradable polymer (polyhydroxyalkanoate) production using bacteria. Adv. Appl. Microbiol. 84, 139–200. doi:10.1016/B978-0-12-407673-0.00004-7
Nishioka, M., Nakai, K., Miyake, M., Asada, Y., and Taya, M. (2001). Production of poly-β-hydroxybutyrate by thermophilic cyanobacterium, Synechococcus sp. MA19, under phosphate-limited conditions. Biotechnol. Lett. 23, 1095–1099. doi:10.1023/A:1010551614648
Nozzi, N., Oliver, J., and Atsumi, S. (2013). Cyanobacteria as a platform for biofuel production. Front. Bioeng. Biotechnol. 1, 7. doi:10.3389/fbioe.2013.00007
Orita, I., Nishikawa, K., Nakamura, S., and Fukui, T. (2014). Biosynthesis of polyhydroxyalkanoate copolymers from methanol by Methylobacterium extorquens AM1 and the engineered strains under cobalt-deficient conditions. Appl. Microbiol. Biotechnol. 98, 3715–3725. doi:10.1007/s00253-013-5490-9
Oswald, W. J., Gotaas, H. B., Golueke, C. G., Kellen, W. R., Gloyna, E. F., and Hermann, E. R. (1957). Algae in waste treatment [with discussion]. Sew. Industrial Wastes 29, 437–457.
Panda, B., and Mallick, N. (2007). Enhanced poly-β-hydroxybutyrate accumulation in a unicellular cyanobacterium, Synechocystis sp. PCC 6803. Lett. Appl. Microbiol. 44, 194–198. doi:10.1111/j.1472-765X.2006.02048.x
Parlane, N. A., Grage, K., Mifune, J., Basaraba, R. J., Wedlock, D. N., Rehm, B. H., et al. (2012). Vaccines displaying mycobacterial proteins on biopolyester beads stimulate cellular immunity and induce protection against tuberculosis. Clin. Vaccine Immunol. 19, 37–44. doi:10.1128/CVI.05505-11
Parmar, A., Singh, N. K., Pandey, A., Gnansounou, E., and Madamwar, D. (2011). Cyanobacteria and microalgae: A positive prospect for biofuels. Bioresour. Technol. 102, 10163–10172. doi:10.1016/j.biortech.2011.08.030
Pfeifenschneider, J., Brautaset, T., and Wendisch, V. F. (2017). Methanol as carbon substrate in the bio-economy: Metabolic engineering of aerobic methylotrophic bacteria for production of value-added chemicals. Biofuels, Bioproducts and Biorefining 11 (4), 719–731. doi:10.1002/bbb.1773
Pérez, R., Cantera, S., Bordel, S., García-Encina, P. A., and Muñoz, R. (2019). The effect of temperature during culture enrichment on methanotrophic polyhydroxyalkanoate production. Int. Biodeterior. Biodegradation 140, 144–151. doi:10.1016/j.ibiod.2019.04.004
Pfluger, A. R., Wu, W. M., Pieja, A. J., Wan, J., Rostkowski, K. H., and Criddle, C. S. (2011). Selection of type I and type II methanotrophic proteobacteria in a fluidized bed reactor under non-sterile conditions. Bioresour. Technol. 102, 9919–9926. doi:10.1016/j.biortech.2011.08.054
Pieja, A. J., Morse, M. C., and Cal, A. J. (2017). Methane to bioproducts: The future of the bioeconomy? Curr. Opin. Chem. Biol. 41, 123–131. doi:10.1016/j.cbpa.2017.10.024
Pieja, A. J., Sundstrom, E. R., and Criddle, C. S. (2011). Poly-3-hydroxybutyrate metabolism in the type II methanotroph Methylocystis parvus OBBP. Appl. Environ. Microbiol. 77, 6012–6019. doi:10.1128/AEM.00509-11
Povolo, S., Toffano, P., Basaglia, M., and Casella, S. (2010). Polyhydroxyalkanoates production by engineered Cupriavidus necator from waste material containing lactose. Bioresour. Technol. 101, 7902–7907. doi:10.1016/j.biortech.2010.05.029
Price, G. D., Sültemeyer, D., Klughammer, B., Ludwig, M., and Badger, M. R. (1998). The functioning of the CO2 concentrating mechanism in several cyanobacterial strains: A review of general physiological characteristics, genes, proteins, and recent advances. Can. J. Bot. 76, 973–1002. doi:10.1139/b98-081
Price, S., Kuzhiumparambil, U., Pernice, M., and Ralph, P. J. (2020). Cyanobacterial polyhydroxybutyrate for sustainable bioplastic production: Critical review and perspectives. J. Environ. Chem. Eng. 8, 104007. doi:10.1016/j.jece.2020.104007
Puppi, D., Morelli, A., and Chiellini, F. (2017). Additive manufacturing of poly(3-hydroxybutyrate-co-3-hydroxyhexanoate)/poly(ε-caprolactone) blend scaffolds for tissue engineering. Bioengineering 4, 49. doi:10.3390/bioengineering4020049
Qin, Q., Ling, C., Zhao, Y., Yang, T., Yin, J., Guo, Y., et al. (2018). CRISPR/Cas9 editing genome of extremophile Halomonas spp. Metab. Eng. 47, 219–229. doi:10.1016/j.ymben.2018.03.018
Quillaguaman, J., Delgado, O., Mattiasson, B., and Hatti-Kaul, R. (2006). Poly (β-hydroxybutyrate) production by a moderate halophile, Halomonas boliviensis LC1. Enzyme Microb. Technol. 38 (1-2), 148–154. doi:10.1016/j.enzmictec.2005.05.013
Ray, S., and Kalia, V. C. (2017b). Biomedical applications of polyhydroxyalkanoates. Indian J. Microbiol. 57, 261–269. doi:10.1007/s12088-017-0651-7
Ray, S., and Kalia, V. C. (2017c). Co-metabolism of substrates by Bacillus thuringiensis regulates polyhydroxyalkanoate co-polymer composition. Bioresour. Technol. 224, 743–747. doi:10.1016/j.biortech.2016.11.089
Ray, S., and Kalia, V. C. (2017a). Microbial cometabolism and polyhydroxyalkanoate co-polymers. Indian J. Microbiol. 57, 39–47. doi:10.1007/s12088-016-0622-4
Ray, S., Sharma, R., and Kalia, V. C. (2018). Co-utilization of crude glycerol and biowastes for producing polyhydroxyalkanoates. Indian J. Microbiol. 58, 33–38. doi:10.1007/s12088-017-0702-0
Raza, Z. A., Abid, S., and Banat, I. M. (2018). Polyhydroxyalkanoates: Characteristics, production, recent developments and applications. Int. Biodeterior. Biodegrad. 126, 45–56. doi:10.1016/j.ibiod.2017.10.001
Raza, Z. A., Khalil, S., and Abid, S. (2020). Recent progress in development and chemical modification of poly(hydroxybutyrate)-based blends for potential medical applications. Int. J. Biol. Macromol. 160, 77–100. doi:10.1016/j.ijbiomac.2020.05.114
Revelles, O., Tarazona, N., García, J. L., and Prieto, M. A. (2016). Carbon roadmap from syngas to polyhydroxyalkanoates in Rhodospirillum rubrum. Environ. Microbiol. 18, 708–720. doi:10.1111/1462-2920.13087
Rosgaard, L., de Porcellinis, A. J., Jacobsen, J. H., Frigaard, N. U., and Sakuragi, Y. (2012). Bioengineering of carbon fixation, biofuels, and biochemicals in cyanobacteria and plants. J. Biotechnol. 162, 134–147. doi:10.1016/j.jbiotec.2012.05.006
Rostkowski, K. H., Pfluger, A. R., and Criddle, C. S. (2013). Stoichiometry and kinetics of the PHB-producing type II methanotrophs Methylosinus trichosporium OB3b and Methylocystis parvus OBBP. Bioresour. Technol. 132, 71–77. doi:10.1016/j.biortech.2012.12.129
Rydz, J., Sikorska, W., Musioł, M., Janeczek, H., Włodarczyk, J., Misiurska-Marczak, M., et al. (2019). 3D-printed polyester-based prototypes for cosmetic applications-future directions at the forensic engineering of advanced polymeric materials. Materials 12, 994. doi:10.3390/ma12060994
Sabarinathan, D., Chandrika, S. P., Venkatraman, P., Easwaran, M., Sureka, C. S., and Preethi, K. (2018). Production of polyhydroxybutyrate (PHB) from Pseudomonas plecoglossicida and its application towards cancer detection. Inf. Med. Unlocked 11, 61–67. doi:10.1016/j.imu.2018.04.009
Sadeghi, D., Karbasi, S., Razavi, S., Mohammadi, S., Shokrgozar, M. A., and Bonakdar, S. (2016). Electrospun poly(hydroxybutyrate)/chitosan blend fibrous scaffolds for cartilage tissue engineering. J. Appl. Polym. Sci. 133, 44171. doi:10.1002/app.44171
Salehizadeh, H., Yan, N., and Farnood, R. (2020). Recent advances in microbial CO2 fixation and conversion to value-added products. Chem. Eng. J. 390, 124584. doi:10.1016/j.cej.2020.124584
Schuchmann, K., and Müller, V. (2013). Direct and reversible hydrogenation of CO2 to formate by a bacterial carbon dioxide reductase. Science 342, 1382–1385. doi:10.1126/science.1244758
Scott, D., Best, D. J., and Higgins, I. J. (1981). Intracytoplasmic membranes in oxygen-limited chemostat cultures of Methylosinus trichosporium OB3b: Biocatalytic implications of physiologically balanced growth. Biotechnol. Lett. 3, 641–644. doi:10.1007/BF00158693
Sedlacek, P., Slaninova, E., Koller, M., Nebesarova, J., Marova, I., and Krzyzanek, V. (2019). PHA granules help bacterial cells to preserve cell integrity when exposed to sudden osmotic imbalances. New Biotechnol. 49, 129–136. doi:10.1016/j.nbt.2018.10.005
Semrau, J. (2011). Bioremediation via methanotrophy: Overview of recent findings and suggestions for future research. Front. Microbiol. 2, 209. doi:10.3389/fmicb.2011.00209
Semrau, J. D., DiSpirito, A. A., and Yoon, S. (2010). Methanotrophs and copper. FEMS Microbiol. Rev. 34, 496–531. doi:10.1111/j.1574-6976.2010.00212.x
Shinagawa, T., Larrazábal, G. O., Martín, A. J., Krumeich, F., and Pérez-Ramírez, J. (2018). Sulfur-modified copper catalysts for the electrochemical reduction of carbon dioxide to formate. ACS Catal. 8, 837–844. doi:10.1021/acscatal.7b03161
Shinde, S., Zhang, X., Singapuri, S. P., Kalra, I., Liu, X., Morgan-Kiss, R. M., et al. (2020). Glycogen metabolism supports photosynthesis start through the oxidative pentose phosphate pathway in cyanobacteria. Plant Physiol. 182, 507–517. doi:10.1104/pp.19.01184
Shishatskaya, E. I., Volova, T. G., Puzyr, A. P., Mogilnaya, O. A., and Efremov, S. N. (2004). Tissue response to the implantation of biodegradable polyhydroxyalkanoate sutures. J. Mater. Sci. Mater. Med. 15, 719–728. doi:10.1023/b:jmsm.0000030215.49991.0d
Shrivastav, A., Mishra, S. K., and Mishra, S. (2010). Polyhydroxyalkanoate (PHA) synthesis by Spirulina subsalsa from Gujarat coast of India. Int. J. Biol. Macromol. 46, 255–260. doi:10.1016/j.ijbiomac.2010.01.001
Singh, A. K., and Mallick, N. (2017). Advances in cyanobacterial polyhydroxyalkanoates production. FEMS Microbiol. Lett. 364, 1–13. doi:10.1093/femsle/fnx189
Singh, A. K., Sharma, L., Mallick, N., and M0000ala, J. (2017). Progress and challenges in producing polyhydroxyalkanoate biopolymers from cyanobacteria. J. Appl. Phycol. 29, 1213–1232. doi:10.1007/s10811-016-1006-1
Singh, A. K., Srivastava, J. K., Chandel, A. K., Sharma, L., Mallick, N., and Singh, S. P. (2019). Biomedical applications of microbially engineered polyhydroxyalkanoates: An insight into recent advances, bottlenecks, and solutions. Appl. Microbiol. Biotechnol. 103 (5), 2007–2032. doi:10.1007/s00253-018-09604-y
Singh, M., Kumar, P., Ray, S., and Kalia, V. C. (2015). Challenges and opportunities for customizing polyhydroxyalkanoates. Indian J. Microbiol. 55, 235–249. doi:10.1007/s12088-015-0528-6
Smith, M. J., and Francis, M. B. (2016). A designed A. vinelandii-S. elongatus coculture for chemical photoproduction from air, water, phosphate, and trace metals. ACS Synth. Biol. 5, 955–961. doi:10.1021/acssynbio.6b00107
Sonnleitner, B., Heinzle, E., Braunegg, G., and Lafferty, R. M. (1979). Formal kinetics of poly-β-hydroxybutyric acid (PHB) production in Alcaligenes eutrophus H16 and Mycoplana rubra R14 with respect to the dissolved oxygen tension in ammonium-limited batch cultures. Eur. J. Appl. Microbiol. Biotechnol. 7, 1–10. doi:10.1007/BF00522473
Sonntag, F., Buchhaupt, M., and Schrader, J. (2014). Thioesterases for ethylmalonyl-CoA pathway derived dicarboxylic acid production in Methylobacterium extorquens AM1. Appl. Microbiol. Biotechnol. 98, 4533–4544. doi:10.1007/s00253-013-5456-y
Sonntag, F., Müller, J. E., Kiefer, P., Vorholt, J. A., Schrader, J., and Buchhaupt, M. (2015). High-level production of ethylmalonyl-CoA pathway-derived dicarboxylic acids by Methylobacterium extorquens under cobalt-deficient conditions and by polyhydroxybutyrate negative strains. Appl. Microbiol. Biotechnol. 99, 3407–3419. doi:10.1007/s00253-015-6418-3
Steinbüchel, A., and Lütke-Eversloh, T. (2003). Metabolic engineering and pathway construction for biotechnological production of relevant polyhydroxyalkanoates in microorganisms. Biochem. Eng. J. 16, 81–96. doi:10.1016/S1369-703X(03)00036-6
Strong, P. J., Laycock, B., Mahamud, S. N. S., Jensen, P. D., Lant, P. A., Tyson, G., et al. (2016). The opportunity for high-performance biomaterials from methane. Microorganisms 4, 11. doi:10.3390/microorganisms4010011
Sun, X., Atiyeh, H. K., Huhnke, R. L., and Tanner, R. S. (2019). Syngas fermentation process development for production of biofuels and chemicals: A review. Bioresour. Technol. Rep. 7, 100279. doi:10.1016/j.biteb.2019.100279
Sundstrom, E. R., and Criddle, C. S. (2015). Optimization of methanotrophic growth and production of poly(3-hydroxybutyrate) in a high-throughput microbioreactor system. Appl. Environ. Microbiol. 81, 4767–4773. doi:10.1128/AEM.00025-15
Suzuki, T., Yamane, T., and Shimizu, S. (1986). Kinetics and effect of nitrogen source feeding on production of poly-β-hydroxybutyric acid by fed-batch culture. Appl. Microbiol. Biotechnol. 24, 366–369. doi:10.1007/BF00294591
Takahashi, H., Miyake, M., Tokiwa, Y., and Asada, Y. (1998). Improved accumulation of poly-3- hydroxybutyrate by a recombinant cyanobacterium. Biotechnol. Lett. 20, 183–186. doi:10.1023/A:1005392827791
Tanaka, K., Miyawaki, K., Yamaguchi, A., Khosravi-Darani, K., and Matsusaki, H. (2011). Cell growth and P (3HB) accumulation from CO2 of a carbon monoxide-tolerant hydrogen-oxidizing bacterium, Ideonella sp. O-1. Appl. Microbiol. Biotechnol. 92, 1161–1169. doi:10.1007/s00253-011-3420-2
Tarawat, S., Incharoensakdi, A., and Monshupanee, T. (2020). Cyanobacterial production of poly(3-hydroxybutyrate-co-3-hydroxyvalerate) from carbon dioxide or a single organic substrate: Improved polymer elongation with an extremely high 3-hydroxyvalerate mole proportion. J. Appl. Phycol. 32, 1095–1102. doi:10.1007/s10811-020-02040-4
Taroncher-Oldenburg, G., Nishina, K., and Stephanopoulos, G. (2000). Identification and analysis of the polyhydroxyalkanoate-specific β-ketothiolase and acetoacetyl coenzyme a reductase genes in the cyanobacterium Synechocystis sp. strain PCC6803. Appl. Environ. Microbiol. 66, 4440–4448. doi:10.1128/AEM.66.10.4440-4448.2000
Toh, P. S., Jau, M. H., Yew, S. P., Abed, R. M., and Sudesh, K. (2008). Comparison of polyhydroxyalkanoates biosynthesis, obilization and the effects on cellular morphology in Spirulina platensis and Synechocystis sp. UNIWG. J. Biosci. 19, 21–38.
Troschl, C., Fritz, I., Sodnikar, K., and Drosg, B. (2017a). Contaminations in mass cultivation of cyanobacteria: Highly resilient Colpoda steinii leads to rapid crash of Synechocystis sp. cultures and is inhibited by partially anoxic conditions. Algal Res. 28, 229–234. doi:10.1016/j.algal.2017.11.002
Troschl, C., Meixner, K., and Drosg, B. (2017b). Cyanobacterial PHA production-Review of recent advances and a summary of three years’ working experience running a pilot plant. Bioengineering 4, 26. doi:10.3390/bioengineering4020026
Ueda, S., Matsumoto, S., Takagi, A., and Yamane, T. (1992). Synthesis of poly(3-hydroxybutyrate-co-3-hydroxyvalerate) from methanol and n-amyl alcohol by the methylotrophic bacteria Paracoccus denitrificans and Methylobacterium extorquens. Appl. Environ. Microbiol. 58, 3574–3579. doi:10.1128/AEM.58.11.3574-3579.1992
Van der Ha, D., Nachtergaele, L., Kerckhof, F. M., Rameiyanti, D., Bossier, P., Verstraete, W., et al. (2012). Conversion of biogas to bioproducts by algae and methane oxidizing bacteria. Environ. Sci. Technol. 46, 13425–13431. doi:10.1021/es303929s
Vermaas, W. F. (2001). Photosynthesis and respiration in cyanobacteria. eLS. doi:10.1038/npg.els.0001670
Volova, T. G., Kalacheva, G. S., and Steinbüchel, A. (2008). Biosynthesis of multi-component polyhydroxyalkanoates by the bacterium Wautersia eutropha. Macromol. Symp. 269, 1–7. doi:10.1002/masy.200850901
Volova, T. G., and Kalacheva, G. S. (2005). The synthesis of hydroxybutyrate and hydroxyvalerate copolymers by the bacterium Ralstonia eutropha. Microbiology 74, 54–59. doi:10.1007/s11021-005-0028-5
Volova, T. G., Kiselev, E. G., Shishatskaya, E. I., Zhila, N. O., Boyandin, A. N., Syrvacheva, D. A., et al. (2013). Cell growth and accumulation of polyhydroxyalkanoates from CO2 and H2 of a hydrogen-oxidizing bacterium, Cupriavidus eutrophus B-10646. Bioresour. Technol. 146, 215–222. doi:10.1016/j.biortech.2013.07.070
Volova, T. G., and Voinov, N. A. (2003). Kinetic parameters of a culture of the hydrogen-oxidizing bacterium Ralstonia eutropha grown under conditions favoring polyhydroxybutyrate biosynthesis. Appl. Biochem. Microbiol. 39, 166–170. doi:10.1023/A:1022585912873
Wang, B., Pugh, S., Nielsen, D. R., Zhang, W., and Meldrum, D. R. (2013). Engineering cyanobacteria for photosynthetic production of 3-hydroxybutyrate directly from CO2. Metab. Eng. 16, 68–77. doi:10.1016/j.ymben.2013.01.001
Wang, B., Xiong, W., Yu, J., Maness, P. C., and Meldrum, D. R. (2018). Unlocking the photobiological conversion of CO2 to (R)-3-hydroxybutyrate in cyanobacteria. Green Chem. 20, 3772–3782. doi:10.1039/C8GC01208C
Wang, L., Wang, Z. H., Shen, C. Y., You, M. L., Xiao, J. F., and Chen, G. Q. (2010). Differentiation of human bone marrow mesenchymal stem cells grown in terpolyesters of 3-hydroxyalkanoates scaffolds into nerve cells. Biomaterials 31, 1691–1698. doi:10.1016/j.biomaterials.2009.11.053
Wang, Y., Jiang, X. L., Yang, S. C., Lin, X., He, Y., Yan, C., et al. (2011). MicroRNAs in the regulation of interfacial behaviors of MSCs cultured on microgrooved surface pattern. Biomaterials 32, 9207–9217. doi:10.1016/j.biomaterials.2011.08.058
Wei, D. X., Dao, J. W., and Chen, G. Q. (2018). A micro-ark for cells: Highly open porous polyhydroxyalkanoate microspheres as injectable scaffolds for tissue regeneration. Adv. Mat. 30, 1802273. doi:10.1002/adma.201802273
Wendlandt, K. D., Geyer, W., Mirschel, G., and Hemidi, F. A. H. (2005). Possibilities for controlling a PHB accumulation process using various analytical methods. J. Biotechnol. 117, 119–129. doi:10.1016/j.jbiotec.2005.01.007
Wendlandt, K. D., Jechorek, M., Helm, J., and Stottmeister, U. (2001). Producing poly-3-hydroxybutyrate with a high molecular mass from methane. J. Biotechnol. 86, 127–133. doi:10.1016/S0168-1656(00)00408-9
Whipple, D. T., and Kenis, P. J. (2010). Prospects of CO2 utilization via direct heterogeneous electrochemical reduction. J. Phys. Chem. Lett. 1, 3451–3458. doi:10.1021/jz1012627
Wijffels, R. H., Kruse, O., and Hellingwerf, K. J. (2013). Potential of industrial biotechnology with cyanobacteria and eukaryotic microalgae. Curr. Opin. Biotechnol. 24, 405–413. doi:10.1016/j.copbio.2013.04.004
Winnacker, M. (2019). Polyhydroxyalkanoates: Recent advances in their synthesis and applications. Eur. J. Lipid Sci. Technol. 121, 1900101. doi:10.1002/ejlt.201900101
Wu, L. P., You, M., Wang, D., Peng, G., Wang, Z., and Chen, G. Q. (2013). Fabrication of carbon nanotube (CNT)/poly(3-hydroxybutyrate-co-3-hydroxyhexanoate) (PHBHHx) nanocomposite films for human mesenchymal stem cell (hMSC) differentiation. Polym. Chem. 4, 4490–4498. doi:10.1039/c3py00668a
Wu, Q., Huang, H., Hu, G., Chen, J., Ho, K. P., and Chen, G. Q. (2001). Production of poly-3-hydroxybutyrate by Bacillus sp. JMa5 cultivated in molasses media. Ant. Van Leeuwenhoek 80, 111–118. doi:10.1023/A:1012222625201
Xia, L., Li, Y., Huang, R., and Song, S. (2017). Effective harvesting of microalgae by coagulation–flotation. R. Soc. open Sci. 4, 170867. doi:10.1098/rsos.170867
Xin, J., Zhang, Y., Dong, J., Song, H., and Xia, C. (2011). An experimental study on molecular weight of poly-3-hydroxybutyrate (PHB) accumulated in Methylosinus trichosporium IMV 3011. Afr. J. Biotechnol. 10, 7078–7087. doi:10.5897/AJB11.085
Yang, M., Zhu, S., Chen, Y., Chang, Z., Chen, G., Gong, Y., et al. (2004). Studies on bone marrow stromal cells affinity of poly (3-hydroxybutyrate-co-3-hydroxyhexanoate). Biomaterials 25, 1365–1373. doi:10.1016/j.biomaterials.2003.08.018
Yezza, A., Fournier, D., Halasz, A., and Hawari, J. (2006). Production of polyhydroxyalkanoates from methanol by a new methylotrophic bacterium Methylobacterium sp. Appl. Microbiol. Biotechnol. 73, 211–218. doi:10.1007/s00253-006-0458-7
Yin, W., Wang, K., Xu, J., Wu, D., and Zhao, C. (2018). The performance and associated mechanisms of carbon transformation (PHAs, polyhydroxyalkanoates) and nitrogen removal for landfill leachate treatment in a sequencing batch biofilm reactor (SBBR). RSC Adv. 8, 42329–42336. doi:10.1039/C8RA07839D
Yishai, O., Lindner, S. N., de la Cruz, J. G., Tenenboim, H., and Bar-Even, A. (2016). The formate bio-economy. Curr. Opin. Chem. Biol. 35, 1–9. doi:10.1016/j.cbpa.2016.07.005
Yoon, J., Chang, W., Oh, S. H., Choi, S. H., Yang, Y. H., and Oh, M. K. (2021). Metabolic engineering of Methylorubrum extorquens AM1 for poly (3-hydroxybutyrate-co-3-hydroxyvalerate) production using formate. Int. J. Biol. Macromol. 177, 284–293. doi:10.1016/j.ijbiomac.2021.02.092
Yu, L. P., Wu, F. Q., and Chen, G. Q. (2019a). Next-Generation Industrial Biotechnology-transforming the current industrial biotechnology into competitive processes. Biotechnol. J. 14, 1800437. doi:10.1002/biot.201800437
Yu, L. P., Zhang, X., Wei, D. X., Wu, Q., Jiang, X. R., and Chen, G. Q. (2019b). Highly efficient fluorescent material based on rare-earth-modified polyhydroxyalkanoates. Biomacromolecules 20, 3233–3241. doi:10.1021/acs.biomac.8b01722
Yu, Y., You, L., Liu, D., Hollinshead, W., Tang, Y. J., and Zhang, F. (2013). Development of Synechocystis sp. PCC 6803 as a phototrophic cell factory. Mar. Drugs 11, 2894–2916. doi:10.3390/md11082894
Zhang, J., Cao, Q., Li, S., Lu, X., Zhao, Y., Guan, J. S., et al. (2013). 3-Hydroxybutyrate methyl ester as a potential drug against Alzheimer's disease via mitochondria protection mechanism. Biomaterials 34, 7552–7562. doi:10.1016/j.biomaterials.2013.06.043
Zhang, S., Qian, X., Chang, S., Dismukes, G. C., and Bryant, D. A. (2016). Natural and synthetic variants of the tricarboxylic acid cycle in cyanobacteria: Introduction of the GABA shunt into Synechococcus sp. PCC 7002. Front. Microbiol. 7, 1972. doi:10.3389/fmicb.2016.01972
Zhang, T., Zhou, J., Wang, X., and Zhang, Y. (2019). Poly-β-hydroxybutyrate production by Methylosinus trichosporium OB3b at different gas-phase conditions. Iran. J. Biotechnol. 17, 10–16. doi:10.21859/ijb.1866
Zhang, J., Shishatskaya, E. I., Volova, T. G., da Silva, L. F., and Chen, G. Q. (2018). Polyhydroxyalkanoates (PHA) for therapeutic applications. Mater. Sci. Eng.: C 86, 144–150. doi:10.1016/j.msec.2017.12.035
Zhang, Y., Xin, J., Chen, L., Song, H., and Xia, C. (2008). Biosynthesis of poly-3-hydroxybutyrate with a high molecular weight by methanotroph from methane and methanol. J. Nat. Gas Chem. 17, 103–109. doi:10.1016/S1003-9953(08)60034-1
Zhao, Y., Zou, B., Shi, Z., Wu, Q., and Chen, G. Q. (2007). The effect of 3-hydroxybutyrate on the in vitro differentiation of murine osteoblast MC3T3-E1 and in vivo bone formation in ovariectomized rats. Biomaterials 28, 3063–3073. doi:10.1016/j.biomaterials.2007.03.003
Zhou, X. Y., Yuan, X. X., Shi, Z. Y., Meng, D. C., Jiang, W. J., Wu, L. P., et al. (2012). Hyperproduction of poly (4-hydroxybutyrate) from glucose by recombinant Escherichia coli. Microb. Cell Fact. 11, 54–58. doi:10.1186/1475-2859-11-54
Keywords: greenhouse gas, PHA (polyhydroxyalkanoates), methanotroph, formate, hydrogen oxidizing bacteria
Citation: Ray S, Jin J-O, Choi I and Kim M (2023) Recent trends of biotechnological production of polyhydroxyalkanoates from C1 carbon sources. Front. Bioeng. Biotechnol. 10:907500. doi: 10.3389/fbioe.2022.907500
Received: 29 March 2022; Accepted: 06 December 2022;
Published: 06 January 2023.
Edited by:
Suchada Chanprateep Napathorn, Chulalongkorn University, ThailandReviewed by:
Akhilesh Kumar Singh, Mahatma Gandhi Central University, Motihari, IndiaCopyright © 2023 Ray, Jin, Choi and Kim. This is an open-access article distributed under the terms of the Creative Commons Attribution License (CC BY). The use, distribution or reproduction in other forums is permitted, provided the original author(s) and the copyright owner(s) are credited and that the original publication in this journal is cited, in accordance with accepted academic practice. No use, distribution or reproduction is permitted which does not comply with these terms.
*Correspondence: Myunghee Kim, Zm9vZHRlY2hAeW51LmFjLmty; Subhasree Ray, c3ViaGFzcmVlLnJheUBzaGFyZGEuYWMuaW4=
Disclaimer: All claims expressed in this article are solely those of the authors and do not necessarily represent those of their affiliated organizations, or those of the publisher, the editors and the reviewers. Any product that may be evaluated in this article or claim that may be made by its manufacturer is not guaranteed or endorsed by the publisher.
Research integrity at Frontiers
Learn more about the work of our research integrity team to safeguard the quality of each article we publish.