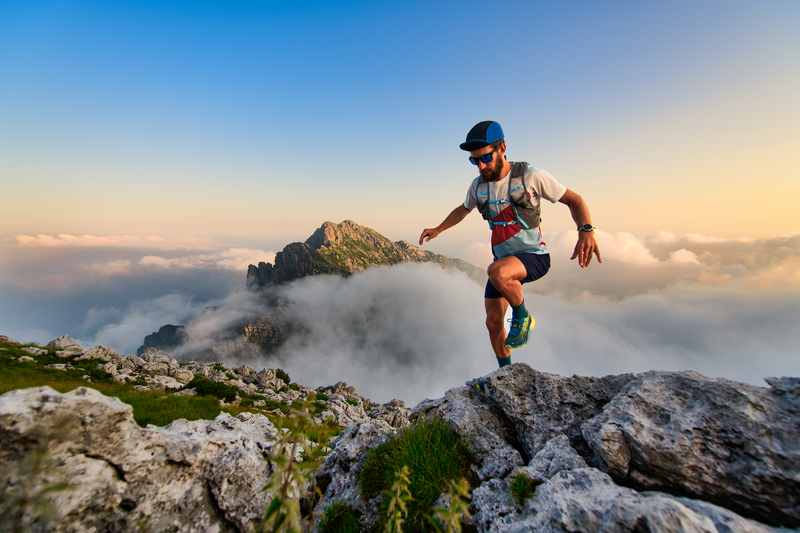
94% of researchers rate our articles as excellent or good
Learn more about the work of our research integrity team to safeguard the quality of each article we publish.
Find out more
MINI REVIEW article
Front. Bioeng. Biotechnol. , 15 July 2022
Sec. Bionics and Biomimetics
Volume 10 - 2022 | https://doi.org/10.3389/fbioe.2022.900389
This article is part of the Research Topic Applications of Biomimetic (Composite) Materials View all 8 articles
Geckos use millions of dry bristles on their toes to adhere to and rapidly run up walls and across ceilings. This has inspired the successful development of dry adhesive materials and their application to climbing robots. The tails of geckos also help realize adaptive and robust climbing behavior. Existing climbing robots with gecko-inspired tails have demonstrated improved locomotion performance. However, few studies have focused on the role of a robot’s gecko-inspired tail when climbing a sloped surface and its effects on the overall locomotion performance. Thus, this paper reviews and analyzes the roles of the tails of geckos and robots in terms of their climbing performances and compares the advantages and disadvantages of robots’ tails made of rigid and soft materials. This review could assist roboticists decide whether a tail is required for their robots and which materials and motion types to use for the tail in order to fulfill their desired functions and even allow the robots to adapt to different environments and tasks.
Bio-inspired robotics uses nature as the inspiration for the design of robotic systems that perform similar to biological systems (Allen (1999); Yang et al. (2018); Pfeifer et al. (2007)). Animal locomotion (e.g., jumping, swimming, flying, crawling, walking, and climbing) has been studied and translated into robotic design principles to create advanced robots capable of navigating in different environments, similar to animals. For example, jumping robots (Armour et al. (2007); Mo et al. (2019, 2020)), inspired by kangaroos, locusts, and frogs, and walking robots (Koh et al. (2010); Manoonpong et al. (2021); Billeschou et al. (2020); Lee et al. (2020)), inspired by centipedes, insects, and dogs, have been studied and developed for traversing uneven or rough terrain. Swimming robots (Ryuh (2009); Ko et al. (2012); Ijspeert et al. (2007)), inspired by fish, jellyfish, and salamanders, have been designed for underwater tasks. Flying robots (Phan et al. (2020); Wenfu et al. (2022); Ramezani et al. (2016)), inspired by insects, birds, and bats, have been built for rescue operations, monitoring, and goods delivery (Huang and Savkin (2018)). Crawling robots (Khan et al. (2022); Yamamoto et al. (2018); Rozen-Levy et al. (2021)), inspired by inchworms and caterpillars, have been developed for branch or pipe crawling. An increasing number of bio-inspired climbing robots have also been proposed over the last few decades. These robots have wide application prospects in narrow space exploration, as well as the inspection and maintenance of pressure vessels, oil tanks, and the glass slabs of high-rise buildings (Dethe and Jaju (2014)).
For bio-inspired climbing robot development, the functional morphology and locomotion behavior of geckos, in particular dry adhesion mechanisms (Autumn and Peattie (2002); Autumn et al. (2006a); Tian et al. (2006); Autumn and Gravish (2008); Puthoff et al. (2010); Stark et al. (2012)) and gaits (Autumn et al. (2005, 2006b); Dai et al. (2011); Schultz JT. et al. (2021)), have been extensively studied. Hence, gecko-inspired climbing robots (Menon et al. (2004); Unver et al. (2006); Kim et al. (2008); Jusufi et al. (2008, 2010, 2011); Estrada et al. (2014); Haomachai et al. (2021); Shao et al. (2022)) and dry adhesive materials (Murphy et al. (2009); Bartlett et al. (2012); Glick et al. (2018); Suthisomboon et al. (2021)) have rapidly been developed. They can adapt to different substrate surfaces (Kim et al. (2008)) and even carry 100x their own weight (e.g., 9g climber (Hawkes et al. (2015))). However, tailless gecko-inspired robots may still be unable to climb on slippery, complex, or steep terrain. Moreover, no synthetic adhesive material can fully capture the desirable properties of a gecko’s foot to enable stable and efficient climbing (Cutkosky (2015)). Adding gecko-inspired tails to robots can significantly improve their climbing because tails play an important role in the locomotion of most mammals and vertebrates (Nabeshima et al. (2019)). These improvements will be discussed in detail later (see Section 2).
Tails usually function as a balancing mechanism, allowing the animal to maintain balance under unstable conditions or to move rapidly and efficiently over rough terrain (Walker et al. (1998); Massaro et al. (2016)). The length of an adult salamander’s tail can reach two-thirds of its body length, indicating the importance of the tail for terrestrial locomotion and balance (Arntzen (1994)). A salamander increases the lateral undulation amplitude of its tail to increase the tail’s contact area and gain thrust while walking on a slippery substrate (Karakasiliotis and Ijspeert (2009)). Similarly, an adult gecko’s tail is almost half the length of its body (Khan (2009); Siddall et al. (2021a); Brown et al. (2010); Jusufi et al. (2010)). Pressing this long tail against a substrate helps maintain balance on slippery and complex terrain, especially on a vertical surface (Figure 1A). Moreover, the morphological features of the tail can help the gecko climb on smooth vertical surfaces, and keeled subcaudal scales can support up to approximately five times body weight (Jusufi et al. (2012)). Geckos can increase their locomotion velocity and maintain their stability on horizontal and vertical surfaces by increasing the lateral undulation amplitude and frequency of their bodies and tails (Schultz J. T. et al. (2021); Wang et al. (2020)). Geckos’ tails also allow them to effectively perform mid-air righting and turning during gliding (Autumn et al. (2005); Jusufi et al. (2008, 2010, 2011); Libby et al. (2012); Figure 1B). For example, Siddall et al. (2021c) recently investigated the role of tails in gliding locomotion using gecko experimental data with mathematical and robotic models and showed that rapid, circular tail rotations can control pitch and yaw motions in a vertical wind tunnel. Moreover, the undulation of the tail also assists a gecko in moving across water (Nirody et al. (2018); Figure 1C).
FIGURE 1. A review of the various studies and models used to assess the role of tail in locomotion. (A) Gecko pressing the tail tip against the wall to avoid slipping during climbing over a slippery gap (Siddall et al. (2021a)). (B) Gecko rotating the tail to achieve air-righting (Jusufi et al. (2008)). (C) The undulation of the tail assists geckos race across the water (Nirody et al. (2018)). (D) RiSE with an active rigid tail as an emergency fifth limb to avoid pitch-back (inspired by panel 1A) (Jusufi et al. (2008)). (E) RiSE with an active soft tail to assist the robot land on a tree (inspired by panel 1A) (Siddall et al. (2021a)). (F) Stickybot with a rotating tail to achieve air-righting (inspired by panel 1B) (Jusufi et al. (2010)). (G) Salamandra robotica II, a salamander inspired amphibious robot with a modular rigid tail that could undulate to help it swim (inspired by panel 1C) (Figure by A. Ijspeert, courtesy Biorobotics Laboratory, EPFL). (H) The slope climbing robot with a passive soft tail to assist in climbing (Siddall et al. (2021b)). (I) Geckobot with an active rigid tail for avoiding pitch-back (Unver et al. (2006)). (J) Compliant fin ray wheg robot with an active soft tail for obstacle crossing (Siddall et al. (2021b)). (K) Tankbot with a passive soft tail for completing plane transition (Unver and Sitti (2009)). (L) Stickybot I with an active tail for avoiding pitch-back (Kim et al. (2007)). (M) Waalbot with a fixed rigid tail to avoid pitch-back (Murphy and Sitti (2007)). Note that the first-row animal figures (A,B,C) correspond to the second-row robot figures (D,E,F,G). All figures are reproduced with permission from respective journals.
Lizards use their tails in several different ways (Libby et al. (2012); Gillis et al. (2009)). For example, the red-headed African Agama lizard can use its tail as an active stabilizing mechanism (Libby et al. (2012)). It raises its tail to adjust the tilt of its body and land successfully on a vertical surface when faced with a lack of footing on a horizontal slippery surface. Based on biological investigations, gecko/lizard-inspired tails were developed and applied to climbing robots to improve their locomotion performance (Menon et al. (2004); Unver et al. (2006); Kim et al. (2008); Lee et al. (2012); Liu et al. (2012)). A tail could provide a preload for a robot to reduce the required adhesive force of its front feet and prevent pitch-back (Unver et al. (2006); Menon et al. (2004); Estrada et al. (2014); Hawkes et al. (2011); Unver and Sitti (2010)). A robot with a larger tail length to body length ratio performs relatively well in terms of, e.g., obstacle traversal (Siddall et al. (2021b)), fall arrest (Menon et al. (2004); Estrada et al. (2014); Hawkes et al. (2011)), and mid-air righting (Jusufi et al. (2008, 2010); Johnson et al. (2012); Libby et al. (2016)). Some climbing robots can use their rigid tails as a support to achieve plane transition (Murphy and Sitti (2007); Murphy et al. (2011); Unver and Sitti (2010)). The aforementioned shows that gecko tails play significant roles in many locomotor scenarios, and many roboticists have applied individual functions of the tail to robots. Therefore, this review compares the advantages and disadvantages of different tail designs, with a focus on different stiffnesses (rigid and soft) and motion types (active, passive, and fixed).
Most studies have focused on developing artificial adhesive feet, which include mechanical (gripping), pneumatic (suction cups), magnetic (permanent magnet), and dry (elastomer adhesive) adhesion (Arzt et al. (2003); Kim et al. (2008); Jiang and Xu (2018); Gu et al. (2018); Schiller et al. (2019); Borijindakul et al. (2021)). Therefore, most early climbing robots were tailless (Kalouche et al. (2014); Haomachai et al. (2021); Srisuchinnawong et al. (2021)). However, animals do not rely solely on stickiness, because even the best adhesives might fail on surfaces that are critically damaged or have a layer of dust, oil, or water (Autumn et al. (2005)). Similarly, a climbing robot will inevitably experience an unexpected slip that results in an undesirable fall. Extensive research on gecko tails has revealed that geckos hold their tails down to maintain balance when their feet slip, preventing them from pitch-back (Autumn et al. (2005, 2006b); Jusufi et al. (2008)). Thus, a gecko-inspired tail has been added to some climbing robots to improve their locomotion performances (Figure 1), e.g., Geckobot (Unver et al. (2006)), Waalbot (Murphy and Sitti (2007)), Waalbot II (Murphy et al. (2011)), Tankbot-I (Unver and Sitti (2009)), Tankbot-IV (Unver and Sitti (2010)), Stickybot I (Hawkes et al. (2011)), Slope climbing robot (Siddall et al. (2021b)), and Mini-Whegs™ 7 (Daltorio et al. (2006)).
Tails can be classified into three classes according to the stiffness of the material: high, medium, and low. A high-stiffness tail cannot be deformed, whereas a medium-stiffness tail can deform under an external force but cannot completely comply or make full contact with the terrain. A low-stiffness tail is soft and complies with the terrain. To compare the roles of soft and rigid tails on robots for locomotion enhancement, we consider medium- and low-stiffness tails to be soft and high-stiffness tails to be rigid. Furthermore, the motion types of tails can be divided into three categories: active, passive, and fixed. An active tail indicates that the robot can control the tail movement in the vertical and/or lateral direction via actuator(s). A passive tail indicates that it can be moved or deformed by an external force. A fixed rigid tail cannot move or deform. As listed in Table 1, all of these tails improve the stability and maximum climbing angle of the robot (Unver et al. (2006); Kim et al. (2008); Hawkes et al. (2011); Lee et al. (2012)). The maximum climbing angle is determined with respect to the horizontal plane. The maximum slope for a slope-climbing robot with a tail length to body length ratio of 0.5 increased to 75° from 45° (tailless prototype) (Siddall et al. (2021b); Figure 1H). The maximum for Mini-Whegs™ 7, which had a tail length to body length ratio of 0.43, increased to 60° from 50° (tailless prototype), and further to 90° when the tail length to body length ratio was increased to 0.74 (Daltorio et al. (2006)). Some of these developed robots could complete plane transition after a tail was added. Tankbot-IV, which had a rigid tail could complete plane transition from the floor to a wall and from a wall to the ceiling (Unver and Sitti (2010)). However, although Tankbot-I had the same maximum climbing angle (180°), it could only complete plane transition from the floor to a wall (Unver and Sitti (2009); Figure 1K). In addition to floor-to-wall and wall-to-ceiling plane transitions, Waalbot II, which had a rigid tail, could also complete plane transition from one wall to another wall (the walls were adjacent to each other at the corner) (Murphy et al. (2011); Figure 1M).
TABLE 1. Characteristics of gecko-inspired robots with a tail. Preload means that the load generated by the tail to prevent pitch-back. Lizard-like gait means that two diagonal pairs of legs switch between stance and swing phases. Wheel gait means that all four “feet/wheels” are attached to the surface all the time (i.e., stance phase).
The offset between a wall and load vector leads to a pitch-back moment (Hawkes et al. (2011)), which causes the front feet of a robot to detach from the wall, resulting in a pitch-back trend. This mainly causes the falling of the climbing robot. However, if a tail is added to a robot, it can generate a preload to transfer some of the load from the rear feet to the front feet, preventing the pitch-back and increasing the maximum slope for the robot (Estrada et al. (2014)). Currently, the most commonly used materials for robot tails are rigid, such as in Geckobot (Unver et al. (2006); Figure 1I), RiSE (Jusufi et al. (2008); Figure 1D), and Waalbot (Murphy and Sitti (2007); Figure 1M). A rigid tail lightly pressed against a wall can create a counter-moment (Autumn et al. (2005); Kim et al. (2008); Hawkes et al. (2011)), which helps create a more even pressure distribution on the robot’s feet and reduces the adhesion required by the front feet (Autumn et al. (2005); Unver et al. (2006); Hawkes et al. (2011); Lee et al. (2012); Estrada et al. (2014)).
RiSE can imitate a gecko’s posture by using its tail (like a bicycle’s kickstand) to regain balance and avoid falling if pitch back was unavoidable (Jusufi et al. (2008); Figure 1D). Tankbot-I (Figure 1K) uses its rigid tail as a support when transiting between vertical and horizontal surfaces (Autumn et al. (2005); Lee et al. (2012); Kalouche et al. (2014)). The length of the tail and angle between the tail and body are also important parameters for a robot with a rigid tail to avoid pitch-back. If the downward forces at the feet are equal and the front and rear feet of the robot have the same friction coefficient, then the feet with a lower normal force slide down or detach from the surface first, and the other feet slide down or detach afterward (Unver et al. (2006)). To prevent this and achieve stable climbing on a constant slope, a certain tail angle should be determined to obtain an additional torque (Lee et al. (2012)) to maintain equal normal forces for the front and rear feet (Unver et al. (2006); Unver and Sitti (2010)). Furthermore, the robot’s tail angle should be adaptively changed to provide appropriate torques for different slopes. Changing the angle of a rigid tail could also adjust the robot’s center of gravity to achieve a stable posture. A longer tail requires less adhesion to prevent the robot from falling (Menon et al. (2004); Unver et al. (2006); Jusufi et al. (2008); Hawkes et al. (2011); Estrada et al. (2014)). However, the weight of the robot increases with the tail length; a longer tail requires more space to turn, limiting the robot’s movement in small spaces (Murphy and Sitti (2007); Unver and Sitti (2010)).
A few climbing robots are gradually adopting soft tails which are usually made of soft materials that can comply with the terrain under a certain load. Typically, the motor-driven soft tail of a slope-climbing robot (Figure 1H) progressively touches the surface from the tip of the tail and generates a preload. Compared to rigid structures, soft structures have higher flexibility, safety, and adaptability, as well as incomparable advantages in narrow space and unstructured environment operations (Majidi (2014); Gu et al. (2018); Schiller et al. (2019)). Furthermore, soft tail has a greater adhesion and a larger effective contact area, facilitating the movement of climbing robots on smooth vertical surfaces. It is also effective in terms of dissipating energy from an impact, reducing damping vibration, and counteracting discontinuous forces and motion (Nguyen et al. (2019); Siddall et al. (2021a)). In contrast, a (simple) active rigid tail can produce large inertial forces and moments with a small rotation when the tail presses against a wall (Saab et al. (2018); Unver et al. (2006)), leading to vibration and even falling. The effect can be reduced by introducing additional damping mechanisms.
Because of the undeformable nature of a rigid tail, the preload process and the surface area contacting the wall is limited by the wall. A soft tail continues to approach the wall until the entire tail is pressed against and makes complete contact with it. Moreover, its adaptability makes it inherently robust to environmental uncertainties (Kim et al. (2013)). To verify the dual fallen tree transition, Siddall et al. (2021b) used two obstacles with heights equal to the height of the robot’s wheels and separation equal to twice the length of the robot. Experiments showed that a fixed rigid tail prevented the robot from pitching when climbing, and a soft tail bent and allowed it to comply with obstacles (Siddall et al. (2021b); Figure 1J). The compliance of a soft tail can also increase the surface area contacting the wall, which is necessary to take advantage of the morphological characteristics (such as keeled subcaudal scales) of a gecko’s tail.
For climbing robots that rely on adhesive feet to move on vertical surfaces (Menon et al. (2004); Unver et al. (2006); Kim et al. (2008); Hawkes et al. (2011); Estrada et al. (2014); Kalouche et al. (2014); Gu et al. (2018); Chen et al. (2019)), the counter-moment generated when the tail contacts the wall can transfer the load from the rear feet to the front feet and reduce the adhesion force required for the front feet to prevent pitch-back (Unver et al. (2006); Jusufi et al. (2008); Kim et al. (2008); Hawkes et al. (2011); Lee et al. (2012); Estrada et al. (2014)). For example, Schultz J. T. et al. (2021) presented a robot that could climb vertical slopes using a rigid tail and claws for adhesion with a gecko-like gait. A rigid tail can provide a large preload, but it can also generate vibrations when the tail is pressed. A soft tail is more stable but it can provide a limited preload. The tail length and Young’s modulus are proportional to the robot’s ability to prevent pitch-back (Menon et al. (2004); Unver et al. (2006); Estrada et al. (2014)). For example, after equipping the robot with a 6.6 cm tail, the maximum slope that Mini-Whegs™ 7 could climb increased from 50° to 60°, and it further improved to 90° when the tail length was 25 cm (Daltorio et al. (2006); Table 1). However, a longer tail also indicates greater weight and greater space required for turning. Thus, it is important to have a suitable tail length to body length ratio (Saab et al. (2018)). According to Table 1, the appropriate tail length to body length ratio ranges for active and passive tails are [0.34, 0.67] and [0.43, 0.74], respectively, whereas the best ratio for a fixed tail is 0.5. This implies that a passive tail requires a longer length than an active one. Analysis using finite element model (FEM) has revealed that the tail length and Young’s modulus are inversely proportional to the adhesion force required by the front feet (Menon et al. (2004)).A soft tail has a greater adhesion and effective contact area and is more adaptable to the environment (Heilmann and Rigney (1981)), making it better at moving over slippery surfaces and complex terrains (Daltorio et al. (2006)). However, a soft tail wears out easily due to frictional adhesion. To avoid pitch-back and achieve plane transition, a rigid tail can be used as a fifth limb and give a point load with a large radius/lever arm, which helps the robot’s front feet stick to the wall (Unver and Sitti (2009)). A soft tail, on the other hand, can convert the ground reaction force (GRF) into elastic deformation and provide an edge load (Siddall et al. (2021b); Santiago et al. (2016)). The addition of a tail can improve the lifting height of the abdomen of a gecko robot during vertical movement, which allows the robot to move over larger obstacles.
The motion type of the tail is another key factor for the environmental adaptability of climbing robots. A fixed rigid tail is suitable for tasks with a constant slope (Figure 1M), whereas a passive tail and a fixed soft tail are more suitable for complex terrain with gradient variations (Figure 1J). Conversely, an active tail is more suitable for special tasks such as obstacle crossing and air-righting by rotating the tail (Figure 1F). Accordingly, considering different combinations of motion type (fixed, active, and passive) and stiffness (soft and rigid) is essential in robot tail design for robustness in various situations. The body motion and gait pattern also influence the tail design. A robot with a bendable body (Haomachai et al. (2021)) may need a soft or lateral undulating tail to compensate for the body swing. A robot with a lizard-like gait pattern (raising two legs at the same time) (Shao et al. (2022)) can benefit from a tail that provides a substantial preload or functions as additional support for stable slope climbing.
The tail lateral undulation has a significant influence on a gecko’s locomotion. The undulating tail could help geckos to run across water by improving their stability and forward velocity (Nirody et al. (2018)). Losing tail motion in geckos results in a more sprawled posture for the loss compensation (Jagnandan and Higham (2017)). However, only a few gecko-inspired robots with undulating tails exist. Undulating tails are commonly used in amphibious robots inspired by lizards and salamanders (Nirody et al. (2018); Karakasiliotis and Ijspeert (2009); Kim et al. (2015); Figure 1G). Typically, each robot implements a specific tail function. Integrating multiple functions for a tail in a robot remains a challenge. In future studies, gecko-tail imitation should focus on the tail actuation system that more closely model muscle mechanics (Xiong et al. (2014); Xiong and Manoonpong (2021)) or hybrid rigid-soft structure/material. For example, soft materials could be used to realize adaptability and 3D dexterity, with rigid links or keels integrated into these soft materials to achieve structural stability (Schwaner et al. (2021)) and an adaptive bio-inspired muscle model can be applied for active tail-joint compliance control with online adaptation (Huerta et al. (2020)).
The tail of a climbing robot is important in terms of improving the maximum climbing angles and avoiding slipping or pitch-back (Jusufi et al. (2008); Kim et al. (2008); Chen et al. (2019)). A longer tail requires less adhesion force for the front feet of the robot to adhere to the substrate (Menon et al. (2004); Unver et al. (2006); Hawkes et al. (2011)); however, the tail length is limited by practical and aesthetic factors. Both rigid and soft tails have advantages and disadvantages. A soft tail is more robust and stable and can more effectively reduce the required adhesion force, while providing a good preload in an unstructured environment. In contrast, a rigid tail can better adjust the robot’s center of gravity, provide a large preload, and aid in plane transition, and it is less prone to wear. The weight of the robot, substrate surface, and environment are the main factors that should be considered when choosing the material and motion type for a robot’s tail. Finally, adding a gecko-inspired tail can help with self-righting and increase locomotion performance in both horizontal and vertical planes.
PM provided the general direction, idea, and supervision of the research. GZ and PM organized and prepared all this manuscript. GZ wrote the manuscript. PM reviewed and edited the manuscript. ZD contributed to the research background. All authors contributed to the article and approved the submitted version.
This work was supported by the National Key Research & Development Program of China (Grant No. 2020YFB1313504) (PM, ZD) and NSFC-DFG (Grant No. 51861135306) (PM).
The authors declare that the research was conducted in the absence of any commercial or financial relationships that could be construed as a potential conflict of interest.
All claims expressed in this article are solely those of the authors and do not necessarily represent those of their affiliated organizations, or those of the publisher, the editors and the reviewers. Any product that may be evaluated in this article, or claim that may be made by its manufacturer, is not guaranteed or endorsed by the publisher.
The author would like to give heartfelt thanks to Pongsiri Borijindakul who gave comments in this paper.
Armour, R., Paskins, K., Bowyer, A., Vincent, J., and Megill, W. (2007). Jumping Robots: a Biomimetic Solution to Locomotion across Rough Terrain. Bioinspir. Biomim. 2, S65–S82. doi:10.1088/1748-3182/2/3/s01
Arntzen, J. W. (1994). Allometry and Autotomy of the Tail in the Golden-Striped Salamander, chioglossa Lusitanica. Amphib. Reptil. 15, 267–274. doi:10.1163/156853894x00047
Arzt, E., Gorb, S., and Spolenak, R. (2003). From Micro to Nano Contacts in Biological Attachment Devices. Proc. Natl. Acad. Sci. U.S.A. 100, 10603–10606. doi:10.1073/pnas.1534701100
Autumn, K., Buehler, M., Cutkosky, M., Fearing, R., Full, R. J., Goldman, D., et al. (2005). “Robotics in Scansorial Environments,” in Unmanned Ground Vehicle Technology VII (Bellingham, WA: International Society for Optics and Photonics), 5804, 291–302. doi:10.1117/12.606157
Autumn, K., Dittmore, A., Santos, D., Spenko, M., and Cutkosky, M. (2006a). Frictional Adhesion: a New Angle on Gecko Attachment. J. Exp. Biol. 209, 3569–3579. doi:10.1242/jeb.02486
Autumn, K., and Gravish, N. (2008). Gecko Adhesion: Evolutionary Nanotechnology. Phil. Trans. R. Soc. A 366, 1575–1590. doi:10.1098/rsta.2007.2173
Autumn, K., Hsieh, S. T., Dudek, D. M., Chen, J., Chitaphan, C., and Full, R. J. (2006b). Dynamics of Geckos Running Vertically. J. Exp. Biol. 209, 260–272. doi:10.1242/jeb.01980
Autumn, K., and Peattie, A. M. (2002). Mechanisms of Adhesion in Geckos. Integr. Comp. Biol. 42, 1081–1090. doi:10.1093/icb/42.6.1081
Bartlett, M. D., Croll, A. B., King, D. R., Paret, B. M., Irschick, D. J., and Crosby, A. J. (2012). Looking beyond Fibrillar Features to Scale Gecko-like Adhesion. Adv. Mat. 24, 1078–1083. doi:10.1002/adma.201104191
Billeschou, P., Bijma, N. N., Larsen, L. B., Gorb, S. N., Larsen, J. C., and Manoonpong, P. (2020). Framework for Developing Bio-Inspired Morphologies for Walking Robots. Appl. Sci. 10, 6986. doi:10.3390/app10196986
Borijindakul, P., Ji, A., Dai, Z., Gorb, S. N., and Manoonpong, P. (2021). Mini Review: Comparison of Bio-Inspired Adhesive Feet of Climbing Robots on Smooth Vertical Surfaces. Front. Bioeng. Biotechnol. 9, 765718. doi:10.3389/fbioe.2021.765718
Brown, R. M., Diesmos, A. C., Duya, M. V., Garcia, H. J. D., and Rico, E. L. B. (2010). New Forest Gecko (Squamata; Gekkonidae; Genus Luperosaurus) from Mt. Mantalingajan, Southern Palawan Island, philippines. J. Herpetology 44, 37–48. doi:10.1670/08-316.1
Chen, R., Fu, L., Qiu, Y., Song, R., and Jin, Y. (2019). A Gecko-Inspired Wall-Climbing Robot Based on Vibration Suction Mechanism. Proc. Institution Mech. Eng. Part C J. Mech. Eng. Sci. 233, 7132–7143. doi:10.1177/0954406219869041
Cutkosky, M. R. (2015). Climbing with Adhesion: from Bioinspiration to Biounderstanding. Interface Focus. 5, 20150015. doi:10.1098/rsfs.2015.0015
Dai, Z., Wang, Z., and Ji, A. (2011). Dynamics of Gecko Locomotion: a Force-Measuring Array to Measure 3d Reaction Forces. J. Exp. Biol. 214, 703–708. doi:10.1242/jeb.051144
Daltorio, K. A., Gorb, S., Peressadko, A., Horchler, A. D., Ritzmann, R. E., and Quinn, R. D. (2006). “A Robot that Climbs Walls Using Micro-structured Polymer Feet,” in Climbing and Walking Robots (Springer), 131–138. doi:10.1007/3-540-26415-9_15
Dethe, R. D., and Jaju, S. (2014). Developments in Wall Climbing Robots: a Review. Int. J. Eng. Res. general Sci. 2, 33–42.
Estrada, M. A., Hawkes, E. W., Christensen, D. L., and Cutkosky, M. R. (2014). “Perching and Vertical Climbing: Design of a Multimodal Robot,” in 2014 IEEE international conference on robotics and automation (ICRA IEEE), 4215–4221. doi:10.1109/icra.2014.6907472
Gillis, G. B., Bonvini, L. A., and Irschick, D. J. (2009). Losing Stability: Tail Loss and Jumping in the Arboreal lizardAnolis Carolinensis. J. Exp. Biol. 212, 604–609. doi:10.1242/jeb.024349
Glick, P., Suresh, S. A., Ruffatto, D., Cutkosky, M., Tolley, M. T., and Parness, A. (2018). A Soft Robotic Gripper with Gecko-Inspired Adhesive. IEEE Robot. Autom. Lett. 3, 903–910. doi:10.1109/lra.2018.2792688
Gu, G., Zou, J., Zhao, R., Zhao, X., and Zhu, X. (2018). Soft Wall-Climbing Robots. Sci. Robot. 3. doi:10.1126/scirobotics.aat2874
Haomachai, W., Shao, D., Wang, W., Ji, A., Dai, Z., and Manoonpong, P. (2021). Lateral Undulation of the Bendable Body of a Gecko-Inspired Robot for Energy-Efficient Inclined Surface Climbing. IEEE Robot. Autom. Lett. 6, 7917–7924. doi:10.1109/lra.2021.3101519
Hawkes, E. W., Christensen, D. L., and Cutkosky, M. R. (2015). “Vertical Dry Adhesive Climbing with a 100× Bodyweight Payload,” in 2015 IEEE International Conference on Robotics and Automation (ICRA) (IEEE), 3762–3769.
Hawkes, E. W., Ulmen, J., Esparza, N., and Cutkosky, M. R. (2011). “Scaling Walls: Applying Dry Adhesives to the Real World,” in 2011 IEEE/RSJ International Conference on Intelligent Robots and Systems (IEEE), 5100–5106. doi:10.1109/iros.2011.6095103
Heilmann, P., and Rigney, D. A. (1981). An Energy-Based Model of Friction and its Application to Coated Systems. Wear 72, 195–217. doi:10.1016/0043-1648(81)90367-7
Huang, H., and Savkin, A. (2018). Towards the Internet of Flying Robots: A Survey. Sensors 18, 4038. doi:10.3390/s18114038
Huerta, C. V., Xiong, X., Billeschou, P., and Manoonpong, P. (2020). “Adaptive Neuromechanical Control for Robust Behaviors of Bio-Inspired Walking Robots,” in International Conference on Neural Information Processing (Springer), 775–786. doi:10.1007/978-3-030-63833-7_65
Ijspeert, A. J., Crespi, A., Ryczko, D., and Cabelguen, J.-M. (2007). From Swimming to Walking with a Salamander Robot Driven by a Spinal Cord Model. science 315, 1416–1420. doi:10.1126/science.1138353
Jagnandan, K., and Higham, T. E. (2017). Lateral Movements of a Massive Tail Influence Gecko Locomotion: an Integrative Study Comparing Tail Restriction and Autotomy. Sci. Rep. 7, 10865–10868. doi:10.1038/s41598-017-11484-7
Jiang, Q., and Xu, F. (2018). Grasping Claws of Bionic Climbing Robot for Rough Wall Surface: Modeling and Analysis. Appl. Sci. 8, 14.
Johnson, A. M., Libby, T., Chang-Siu, E., Tomizuka, M., Full, R. J., and Koditschek, D. E. (2012). “Tail Assisted Dynamic Self Righting,” in Adaptive Mobile Robotics (World Scientific), 611–620. doi:10.1142/9789814415958_0079
Jusufi, A., Goldman, D. I., Revzen, S., and Full, R. J. (2008). Active Tails Enhance Arboreal Acrobatics in Geckos. Proc. Natl. Acad. Sci. U.S.A. 105, 4215–4219. doi:10.1073/pnas.0711944105
Jusufi, A., Kawano, D. T., Libby, T., and Full, R. J. (2010). Righting and Turning in Mid-air Using Appendage Inertia: Reptile Tails, Analytical Models and Bio-Inspired Robots. Bioinspir. Biomim. 5, 045001. doi:10.1088/1748-3182/5/4/045001
Jusufi, A., Libby, T., and Full, R. (2012). “Scales Assist Scaling in Lizards: Keeled, Subcaudal Scale Arrays Engage Substrate during Rapid Vertical Climbing,” in INTEGRATIVE AND COMPARATIVE BIOLOGY (CARY, NC 27513 USA): OXFORD UNIV PRESS INC JOURNALS DEPT, 2001 EVANS RD), 52, E88.
Jusufi, A., Zeng, Y., Full, R. J., and Dudley, R. (2011). Aerial Righting Reflexes in Flightless Animals.
Kalouche, S., Wiltsie, N., Su, H.-J., and Parness, A. (2014). “Inchworm Style Gecko Adhesive Climbing Robot,” in 2014 IEEE/RSJ International Conference on Intelligent Robots and Systems (IEEE), 2319–2324. doi:10.1109/iros.2014.6942876
Karakasiliotis, K., and Ijspeert, A. J. (2009). “Analysis of the Terrestrial Locomotion of a Salamander Robot,” in 2009 IEEE/RSJ International Conference on Intelligent Robots and Systems (IEEE), 5015–5020. doi:10.1109/iros.2009.5354220
Khan, M. B., Chuthong, T., Homchanthanakul, J., and Manoonpong, P. (2022). Electromagnetic Feet with Soft Toes for Adaptive, Versatile, and Stable Locomotion of an Inchworm-Inspired Pipe Crawling Robot. Front. Bioeng. Biotechnol., 242. doi:10.3389/fbioe.2022.842816
Khan, M. S. (2009). Natural History and Biology of Hobbyist Choice Leopard Gecko eublepharis Macularius. Rabwah, Pakistan: Talim ul Islam College.
Kim, H., Lee, D. G., and Seo, T. (2015). Rolling Stability Enhancement via Balancing Tail for a Water-Running Robot. J. Bionic Eng. 12, 395–405. doi:10.1016/s1672-6529(14)60131-1
Kim, S., Laschi, C., and Trimmer, B. (2013). Soft Robotics: a Bioinspired Evolution in Robotics. Trends Biotechnol. 31, 287–294. doi:10.1016/j.tibtech.2013.03.002
Kim, S., Spenko, M., Trujillo, S., Heyneman, B., Mattoli, V., and Cutkosky, M. R. (2007). “Whole Body Adhesion: Hierarchical, Directional and Distributed Control of Adhesive Forces for a Climbing Robot,” in Proceedings 2007 IEEE International Conference on Robotics and Automation (IEEE), 1268–1273. doi:10.1109/robot.2007.363159
Ko, Y., Na, S., Lee, Y., Cha, K., Ko, S. Y., Park, J., et al. (2012). A Jellyfish-like Swimming Mini-Robot Actuated by an Electromagnetic Actuation System. Smart Mat. Struct. 21, 057001. doi:10.1088/0964-1726/21/5/057001
Koh, D., Yang, J., and Kim, S. (2010). “Centipede Robot for Uneven Terrain Exploration: Design and Experiment of the Flexible Biomimetic Robot Mechanism,” in 2010 3rd IEEE RAS & EMBS International Conference on Biomedical Robotics and Biomechatronics (IEEE), 877–881. doi:10.1109/biorob.2010.5627776
Lee, G., Wu, G., Kim, J., and Seo, T. (2012). High-payload Climbing and Transitioning by Compliant Locomotion with Magnetic Adhesion. Robotics Aut. Syst. 60, 1308–1316. doi:10.1016/j.robot.2012.06.003
Lee, J., Hwangbo, J., Wellhausen, L., Koltun, V., and Hutter, M. (2020). Learning Quadrupedal Locomotion over Challenging Terrain. Sci. Robot. 5, eabc5986. doi:10.1126/scirobotics.abc5986
Libby, T., Johnson, A. M., Chang-Siu, E., Full, R. J., and Koditschek, D. E. (2016). Comparative Design, Scaling, and Control of Appendages for Inertial Reorientation. IEEE Trans. Robot. 32, 1380–1398. doi:10.1109/tro.2016.2597316
Libby, T., Moore, T. Y., Chang-Siu, E., Li, D., Cohen, D. J., Jusufi, A., et al. (2012). Tail-assisted Pitch Control in Lizards, Robots and Dinosaurs. Nature 481, 181–184. doi:10.1038/nature10710
Liu, Y., Wu, X., Qian, H., Zheng, D., Sun, J., and Xu, Y. (2012). “System and Design of Clothbot: A Robot for Flexible Clothes Climbing,” in 2012 IEEE International Conference on Robotics and Automation (IEEE), 1200–1205. doi:10.1109/icra.2012.6224620
Majidi, C. (2014). Soft Robotics: a Perspective—Current Trends and Prospects for the Future. Soft Robot. 1, 5–11.
Manoonpong, P., Patanè, L., Xiong, X., Brodoline, I., Dupeyroux, J., Viollet, S., et al. (2021). Insect-inspired Robots: Bridging Biological and Artificial Systems. Sensors (Basel) 21, 7609. doi:10.3390/s21227609
Massaro, L., Massa, F., Simpson, K., Fragaszy, D., and Visalberghi, E. (2016). The Strategic Role of the Tail in Maintaining Balance while Carrying a Load Bipedally in Wild Capuchins (Sapajus Libidinosus): a Pilot Study. Primates 57, 231–239. doi:10.1007/s10329-015-0507-x
Menon, C., Murphy, M., and Sitti, M. (2004). “Gecko Inspired Surface Climbing Robots,” in 2004 IEEE International Conference on Robotics and Biomimetics (IEEE), 431–436.
Mo, X., Ge, W., Miraglia, M., Inglese, F., Zhao, D., Stefanini, C., et al. (2020). Jumping Locomotion Strategies: from Animals to Bioinspired Robots. Appl. Sci. 10, 8607. doi:10.3390/app10238607
Mo, X., Ge, W., Romano, D., Donati, E., Benelli, G., Dario, P., et al. (2019). Modelling Jumping in locusta Migratoria and the Influence of Substrate Roughness. entomologia 38, 317–332. doi:10.1127/entomologia/2019/0607
Murphy, M. P., Kim, S., and Sitti, M. (2009). Enhanced Adhesion by Gecko-Inspired Hierarchical Fibrillar Adhesives. ACS Appl. Mat. Interfaces 1, 849–855. doi:10.1021/am8002439
Murphy, M. P., Kute, C., Mengüç, Y., and Sitti, M. (2011). Waalbot Ii: Adhesion Recovery and Improved Performance of a Climbing Robot Using Fibrillar Adhesives. Int. J. Robotics Res. 30, 118–133. doi:10.1177/0278364910382862
Murphy, M. P., and Sitti, M. (2007). Waalbot: An Agile Small-Scale Wall-Climbing Robot Utilizing Dry Elastomer Adhesives. IEEE/ASME Trans. Mechatron. 12, 330–338. doi:10.1109/tmech.2007.897277
Nabeshima, J., Saraiji, M. Y., and Minamizawa, K. (2019). “Arque: Artificial Biomimicry-Inspired Tail for Extending Innate Body Functions,” in ACM SIGGRAPH 2019 Posters, 1–2.
Nguyen, H.-N., Siddall, R., Stephens, B., Navarro-Rubio, A., and Kovač, M. (2019). “A Passively Adaptive Microspine Grapple for Robust, Controllable Perching,” in 2019 2nd IEEE International Conference on Soft Robotics (RoboSoft) (IEEE), 80–87. doi:10.1109/robosoft.2019.8722779
Nirody, J. A., Jinn, J., Libby, T., Lee, T. J., Jusufi, A., Hu, D. L., et al. (2018). Geckos Race across the Water's Surface Using Multiple Mechanisms. Curr. Biol. 28, 4046–4051. doi:10.1016/j.cub.2018.10.064
Pfeifer, R., Lungarella, M., and Iida, F. (2007). Self-organization, Embodiment, and Biologically Inspired Robotics. science 318, 1088–1093. doi:10.1126/science.1145803
Phan, H. V., Aurecianus, S., Au, T. K. L., Kang, T., and Park, H. C. (2020). Towards the Long-Endurance Flight of an Insect-Inspired, Tailless, Two-Winged, Flapping-Wing Flying Robot. IEEE Robot. Autom. Lett. 5, 5059–5066. doi:10.1109/lra.2020.3005127
Puthoff, J. B., Prowse, M. S., Wilkinson, M., and Autumn, K. (2010). Changes in Materials Properties Explain the Effects of Humidity on Gecko Adhesion. J. Exp. Biol. 213, 3699–3704. doi:10.1242/jeb.047654
Ramezani, A., Shi, X., Chung, S.-J., and Hutchinson, S. (2016). “Bat Bot (B2), a Biologically Inspired Flying Machine,” in 2016 IEEE International Conference on Robotics and Automation (ICRA) (IEEE), 3219–3226. doi:10.1109/icra.2016.7487491
Rozen-Levy, S., Messner, W., and Trimmer, B. A. (2021). The Design and Development of Branch Bot: a Branch-Crawling, Caterpillar-Inspired, Soft Robot. Int. J. Robotics Res. 40, 24–36. doi:10.1177/0278364919846358
Ryuh, Y.-S. (2009). Development of Swimming Mechanism and Algorithm for Fish-type Underwater Robot (1). J. Korea Robotics Soc. 4, 43–48.
Saab, W., Rone, W. S., and Ben-Tzvi, P. (2018). Robotic Tails: a State-Of-The-Art Review. Robotica 36, 1263–1277.
Sangbae Kim, S., Spenko, M., Trujillo, S., Heyneman, B., Santos, D., and Cutkosky, M. R. (2008). Smooth Vertical Surface Climbing with Directional Adhesion. IEEE Trans. Robot. 24, 65–74. doi:10.1109/tro.2007.909786
Santiago, J. L. C., Godage, I. S., Gonthina, P., and Walker, I. D. (2016). Soft Robots and Kangaroo Tails: Modulating Compliance in Continuum Structures through Mechanical Layer Jamming. Soft Robot. 3, 54–63. doi:10.1089/soro.2015.0021
Schiller, L., Seibel, A., and Schlattmann, J. (2019). Toward a Gecko-Inspired, Climbing Soft Robot. Front. Neurorobot. 13, 106. doi:10.3389/fnbot.2019.00106
Schultz, J. T., Beck, H. K., Haagensen, T., Proost, T., and Clemente, C. J. (2021a). Using a Biologically Mimicking Climbing Robot to Explore the Performance Landscape of Climbing in Lizards. Proc. Biol. Sci. 288, 20202576. doi:10.1098/rspb.2020.2576
Schultz, J. T., Cieri, R. L., Proost, T., Pilai, R., Hodgson, M., Plum, F., et al. (2021b). Tail Base Deflection but Not Tail Curvature Varies with Speed in Lizards: Results from an Automated Tracking Analysis Pipeline. Integr. Comp. Biol. 61, 1769–1782. doi:10.1093/icb/icab037
Schwaner, M., Hsieh, S., Braasch, I., Bradley, S., Campos, C., Collins, C., et al. (2021). Future Tail Tales: A Forward-Looking, Integrative Perspective on Tail Research. Integr. Comp. Biol. 17. doi:10.1088/1748-3190/ac5a3c
Shao, D., Wang, Z., Ji, A., Dai, Z., and Manoonpong, P. (2022). A Gecko-Inspired Robot with Cpg-Based Neural Control for Locomotion and Body Height Adaptation. Bioinspiration Biomimetics. doi:10.1088/1748-3190/ac5a3c
Siddall, R., Byrnes, G., Full, R. J., and Jusufi, A. (2021a). Tails Stabilize Landing of Gliding Geckos Crashing Head-First into Tree Trunks. Commun. Biol. 4, 1–12. doi:10.1038/s42003-021-02378-6
Siddall, R., Ibanez, V., Byrnes, G., Full, R. J., and Jusufi, A. (2021c). Mechanisms for Mid-air Reorientation Using Tail Rotation in Gliding Geckos. Integr. Comp. Biol. 61, 478–490. doi:10.1093/icb/icab132
Siddall, R., Fukushima, T., Bardhi, D., Perteshoni, B., Morina, A., Hasimja, E., et al. (2021b). Compliance, Mass Distribution and Contact Forces in Cursorial and Scansorial Locomotion with Biorobotic Physical Models. Adv. Robot. 35, 437–449. doi:10.1080/01691864.2021.1887760
Srisuchinnawong, A., Wang, B., Shao, D., Ngamkajornwiwat, P., Dai, Z., Ji, A., et al. (2021). Modular Neural Control for Gait Adaptation and Obstacle Avoidance of a Tailless Gecko Robot. J. Intelligent Robotic Syst. 101, 1–15. doi:10.1007/s10846-020-01285-y
Stark, A. Y., Sullivan, T. W., and Niewiarowski, P. H. (2012). The Effect of Surface Water and Wetting on Gecko Adhesion. J. Exp. Biol. 215, 3080–3086. doi:10.1242/jeb.070912
Suthisomboon, T., Rukpanich, T., Asawalertsak, N., Borijindakul, P., Ji, A., Dai, Z., et al. (2021). “Venom: Versatile, Adhesive, and Soft Material for Various Surface Adhesion,” in 2021 IEEE 4th International Conference on Soft Robotics (RoboSoftI EEE), 543–546. doi:10.1109/robosoft51838.2021.9479242
Tian, Y., Pesika, N., Zeng, H., Rosenberg, K., Zhao, B., McGuiggan, P., et al. (2006). Adhesion and Friction in Gecko Toe Attachment and Detachment. Proc. Natl. Acad. Sci. U.S.A. 103, 19320–19325. doi:10.1073/pnas.0608841103
Unver, O., and Sitti, M. (2009). “Tankbot: A Miniature, Peeling Based Climber on Rough and Smooth Surfaces,” in 2009 IEEE International Conference on Robotics and Automation (IEEE), 2282–2287. doi:10.1109/robot.2009.5152304
Unver, O., and Sitti, M. (2010). Tankbot: A Palm-Size, Tank-like Climbing Robot Using Soft Elastomer Adhesive Treads. Int. J. Robotics Res. 29, 1761–1777. doi:10.1177/0278364910380759
Unver, O., Uneri, A., Aydemir, A., and Sitti, M. (2006). “Geckobot: A Gecko Inspired Climbing Robot Using Elastomer Adhesives,” in Proceedings 2006 IEEE International Conference on Robotics and Automation, 2006. ICRA 2006 (IEEE), 2329–2335.
Walker, C., Vierck, C. J., and Ritz, L. A. (1998). Balance in the Cat: Role of the Tail and Effects of Sacrocaudal Transection. Behav. Brain Res. 91, 41–47. doi:10.1016/s0166-4328(97)00101-0
Wang, W., Ji, A., Chen, G., Ravi, S., Shen, H., Gorb, S. N., et al. (2020). Kinematics of Gecko Climbing: the Lateral Undulation Pattern. Zoology 140, 125768. doi:10.1016/j.zool.2020.125768
Wenfu, X., Erzhen, P., Juntao, L., Yihong, L., and Han, Y. (2022). Flight Control of a Large-Scale Flapping-Wing Flying Robotic Bird: System Development and Flight Experiment. Chin. J. Aeronautics 35, 235–249.
Xiong, X., and Manoonpong, P. (2021). Online Sensorimotor Learning and Adaptation for Inverse Dynamics Control. Neural Netw. 143, 525–536. doi:10.1016/j.neunet.2021.06.029
Xiong, X., Wörgötter, F., and Manoonpong, P. (2014). Virtual Agonist-Antagonist Mechanisms Produce Biological Muscle-like Functions: an Application for Robot Joint Control. Industrial Robot Int. J. 41, 340–346. doi:10.1108/IR-11-2013-421
Yamamoto, T., Konyo, M., Tadakuma, K., and Tadokoro, S. (2018). High-speed Sliding-Inchworm Motion Mechanism with Expansion-type Pneumatic Hollow-Shaft Actuators for In-Pipe Inspections. Mechatronics 56, 101–114. doi:10.1016/j.mechatronics.2018.10.010
Keywords: gecko locomotion, bio-inspired tails, climbing robots, soft material, biomimetic design
Citation: Zang G, Dai Z and Manoonpong P (2022) The Roles and Comparison of Rigid and Soft Tails in Gecko-Inspired Climbing Robots: A Mini-Review. Front. Bioeng. Biotechnol. 10:900389. doi: 10.3389/fbioe.2022.900389
Received: 20 March 2022; Accepted: 07 June 2022;
Published: 15 July 2022.
Edited by:
Hung-Yin Lin, National University of Kaohsiung, TaiwanReviewed by:
Christofer J Clemente, University of the Sunshine Coast, AustraliaCopyright © 2022 Zang, Dai and Manoonpong. This is an open-access article distributed under the terms of the Creative Commons Attribution License (CC BY). The use, distribution or reproduction in other forums is permitted, provided the original author(s) and the copyright owner(s) are credited and that the original publication in this journal is cited, in accordance with accepted academic practice. No use, distribution or reproduction is permitted which does not comply with these terms.
*Correspondence: Guangyuan Zang, emFuZ2d1YW5neXVhbkBudWFhLmVkdS5jbg==; Poramate Manoonpong, cG9tYUBudWFhLmVkdS5jbg==
Disclaimer: All claims expressed in this article are solely those of the authors and do not necessarily represent those of their affiliated organizations, or those of the publisher, the editors and the reviewers. Any product that may be evaluated in this article or claim that may be made by its manufacturer is not guaranteed or endorsed by the publisher.
Research integrity at Frontiers
Learn more about the work of our research integrity team to safeguard the quality of each article we publish.