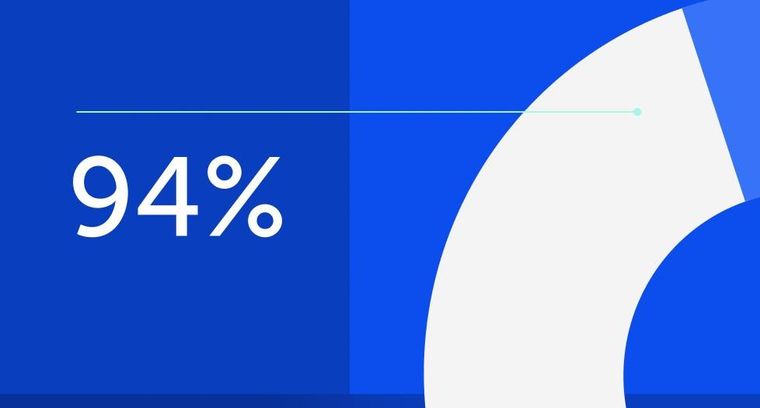
94% of researchers rate our articles as excellent or good
Learn more about the work of our research integrity team to safeguard the quality of each article we publish.
Find out more
ORIGINAL RESEARCH article
Front. Bioeng. Biotechnol., 09 June 2022
Sec. Bioprocess Engineering
Volume 10 - 2022 | https://doi.org/10.3389/fbioe.2022.896261
This article is part of the Research TopicAlgal Biomass and BiofuelsView all 11 articles
The most studied and cultivated microalgae have a temperature optimum between 20 and 35°C. This temperature range hampers sustainable microalgae growth in countries with colder periods. To overcome this problem, psychrotolerant microalgae, such as the snow alga Chloromonas typhlos, can be cultivated during these colder periods. However, most of the research work has been carried out in the laboratory. The step between laboratory-scale and large-scale cultivation is difficult, making pilot-scale tests crucial to gather more information. Here, we presented a successful pilot-scale growth test of C. typhlos. Seven batch mode growth periods were compared during two longer growth tests in a photobioreactor of 350 L. We demonstrated the potential of this alga to be cultivated at colder ambient temperatures. The tests were performed during winter and springtime to compare ambient temperature and sunlight influences. The growth and CO2 usage were continuously monitored to calculate the productivity and CO2 fixation efficiency. A maximum dry weight of 1.082 g L−1 was achieved while a maximum growth rate and maximum daily volumetric and areal productivities of 0.105 d−1, 0.110 g L−1 d−1, and 2.746 g m−2 d−1, respectively, were measured. Future tests to optimize the cultivation of C. typhlos and production of astaxanthin, for example, will be crucial to explore the potential of biomass production of C. typhlos on a commercial scale.
Microalgae are a diverse group of single-celled eukaryotic organisms that are ubiquitously present in a wide range of habitats and conditions. They can use CO2 as a carbon source and sunlight as an energy source to produce organic matter through photosynthesis (Benedetti et al., 2018; Abo et al., 2019; Vale et al., 2020; Vecchi et al., 2020). Their photosynthetic efficiency can be 10 times more efficient than that of terrestrial plants, and when combined with their fast growth rates, they are a promising source of renewable feedstock for different applications (Singh and Ahluwalia, 2013; Benedetti et al., 2018; Khan et al., 2018). It is, thus, no surprise that the cultivation of microalgae has bloomed in recent years due to its vast potential (Richmond, 2000; Chisti, 2007; Singh and Ahluwalia, 2013; Benedetti et al., 2018; Khan et al., 2018). The true potential of microalgae, however, remains untapped on a larger scale. For many microalgae species, several challenges remain to reach sustainable and economically viable cultivation (Richmond, 2000; Chisti, 2007; Mata et al., 2010; Sayre, 2010; Tredici, 2010).
Currently, only a few selective microalgae are commercially cultivated (Garrido-Cardenas et al., 2018; Dolganyuk et al., 2020; Wang et al., 2021). This is often attributed to the legislative burden associated with the cultivation of novel species, for example, the European Novel Foods Regulation (Araújo et al., 2021). Next to the aforementioned, only a select few of the thousands of species are studied (Guiry, 2012; Garrido-Cardenas et al., 2018). Most of this research is carried out on the laboratory scale and often in well-controlled small volumes, making the extrapolation to larger-scale cultivation difficult (Tredici, 2010; Quinn et al., 2011; Moody et al., 2014). Furthermore, most microalgae studied or commercially cultivated are microalgae with optimal growth temperatures between 20 and 35°C (Kvíderová et al., 2017; Cheregi et al., 2019; Suzuki et al., 2019; Dolganyuk et al., 2020). Nevertheless, many countries have a colder climate, making year-round cultivation difficult unless cultures are heated during colder months. Since the production costs of microalgae are still a bottleneck in the expansion of commercialization, a year-round microalgae cultivation, without the need for excessive heating, can support sustainability and economic feasibility (Matsumoto et al., 2017; Garrido-Cardenas et al., 2018; Cheregi et al., 2019; Suzuki et al., 2019).
Cold-adapted microalgae could bridge the colder periods during year-round microalgae cultivation (Kvíderová et al., 2017; Matsumoto et al., 2017; Suzuki et al., 2019). While, cold-adapted microalgae, often referred to as snow algae, are being studied more in recent years (Remias et al., 2016; Kvíderová et al., 2017; Hoham and Remias, 2020); most studies focus on the ecology and taxonomy. A few studies describe the cultivation of snow algae in a laboratory setting, but knowledge of their cultivation on a larger scale is largely absent (Kvíderová, 2010; Kvíderová et al., 2017; Matsumoto et al., 2017). Aside from being able to grow in colder temperatures, snow algae can be a source of polyunsaturated fatty acids (PUFAs) and other valuable compounds such as astaxanthin (Varshney et al., 2015; Kvíderová et al., 2017; Cheregi et al., 2019; Sathasivam et al., 2019; Suzuki et al., 2019). Snow algae, many belonging to the Chloromonas–Chlamydomonas complex, are polyextremophiles adapted to survive in regions with harsh conditions such as low temperatures, high irradiation, and lack of nutrients and liquid water (Remias et al., 2005; Kvíderová, 2010; Kvíderová et al., 2017; Procházková et al., 2018; Hoham and Remias, 2020).
One snow alga with a potential commercial value is the red snow alga Chloromonas typhlos (Chlamydomonas nivalis). It is one of the most studied snow algae and is known to cause the phenomenon of red patches of snow (Mosser et al., 1977; Remias et al., 2005; Cvetkovska et al., 2017). This phenomenon of red snow, often called “watermelon snow”, is caused by the red pigment astaxanthin and its acid ester derivatives (Remias et al., 2005; Cvetkovska et al., 2017). However, to the best of our knowledge, no studies describe the large-scale cultivation of this alga in a photobioreactor. In order to help close the gap between laboratory experiments and large-scale cultivation of the snow alga C. typhlos, we performed a proof-of-concept study in which we grew C. typhlos in a photobioreactor installed in a minimally heated (frost-protection) greenhouse during colder periods.
Chloromonas typhlos (SAG 26.68) was purchased from SAG (Department Experimental Phycology and Culture Collection of Algae, University of Göttingen, Germany). The culture was maintained in the laboratory in a sterilized (autoclaved at 121°C for 20 min) freshwater medium based on the SAG basal medium (version 10.2008). It was kept in a 250-ml Erlenmeyer flask on an orbital shaker at 90 rpm with 70 μmol m−2 s−1 light exposure (cool-white fluorescent) in a climate-controlled room at 22°C (±0.2 SD) under a 16/8-h day/night regime. For upscaling, the cultures were transferred, respectively, to aerated 1, 2, and 40 L recipients. Ambient air was used for aeration, and no extra CO2 was provided during upscaling of the cultures. Cultures in the photobioreactors were grown in the same medium sterilized by filtration (0.1 μm). The freshwater medium had the following composition: 152 mg L−1 HNO3, 22 mg L−1 H3PO4, 148 mg L−1 KOH, 6.3 mg L−1 Fe-DTPA, 42 pg L−1 CuSO4.5H2O, 2.8 μg L−1 ZnSO4, 7.2 μg L−1 MnSO4, 4.3 μg L−1 Na2MoO4, 40 μg L−1 Na2B4O7, 0.2 g L−1 NaHCO3, and 23 mg L−1 MgSO4.7H2O. Furthermore, nitrogen and phosphorus were added to the culture when needed, based on the regular measurement of their concentrations, to maintain non-nutrient conditions. The medium was prepared autonomously by a central computer control unit in the greenhouse and fed to the cultures by a feed supply unit (FertiMiX 600, Hortimax).
A tubular multilayer photobioreactor with a volume of 350 L was used for the cultivation of C. typhlos. The reactor is part of the pilot plant of the EU project “Sunbuilt” and is located in a greenhouse in Geel, Belgium. The photobioreactor consists of transparent unplasticized polyvinylchloride (PVC-u) tubes with an external diameter of 5 cm. To avoid shading of lower located tubes, a triangle-like configuration of the tubes was used (Figure 1). For the inoculation of the photobioreactor, approximately 40 L of a 0.5–1 g L−1 C. typhlos culture was used.
FIGURE 1. Picture of the photobioreactor used to perform the growth experiments with C. typhlos. The red frame shows the C. typhlos cells as seen throughout the experiments. The cells were always green.
C. typhlos was cultivated in a 350-L (Vr) bioreactor during two growth tests ranging from March to May 2019 (growth test 1) and from December 2019 to February 2020 (growth test 2) in non-nutrient limiting conditions. To evaluate the growth of C. typhlos during these two tests, seven (three during the first test and four during the second test) 9-day growth periods were selected and compared. Prior to starting a 9-day growth period, C. typhlos was partially harvested, and the culture was refreshed with a new medium and permitted to regrow before a new 9-day growth period started. The first period at the start of growth test 2 (December 2019), however, started right from inoculation, giving a lower start concentration.
The growth was monitored continuously online with a turbidity sensor (Georg Fischer Signet 4,150 turbidimeter 0–1000 NTU) and offline by dry weight measurements. The medium was circulated by using a centrifugal pump (900 rpm), and filter-sterilized (0.1 μm) ambient air was injected into the lowest tube at a continuous rate for mixing. The working pH was set at 8, continuously measured, and maintained by the injection of CO2 on demand, in the airflow, with a maximum flow of 885.9 ml normal per minute. The total CO2 injected into the photobioreactors was measured during the growth periods (IN-FLOW mass flow meter/controller Bronkhorst, the Netherlands). The ambient temperature inside the greenhouse was monitored (Ektron III-C, Hortiplan) continuously and maintained at night at a minimal temperature of 10°C for frost protection with a gas heater (HHB-100A-230V, Holland Heaters).
When the temperature inside the greenhouse reached 23°C, foggers inside the greenhouse and sprinklers on the roof were turned on automatically to prevent an excessive rise in temperature. Photosynthetically active radiation (PAR) was measured continuously with a PAR sensor (LI-COR LI-190 R Quantum sensor) installed inside the greenhouse, on top of the reactor. A solar irradiance meter (LP02-TR pyranometer) was installed outside the greenhouse to measure total solar radiation. At a measured value of 400 W m−2, a sunscreen inside the greenhouse was automatically partially closed to reduce irradiation by 20–30%. The effect of additional lighting to prolong the length of daytime was studied between 10 December 2019 and 19 February 2020 by providing extra lighting (Philips TL-D 58 W 865; 504 mmol m−2) from 6.30 to 10.30 and 15.30 to 22.00 during 3–4 days to lengthen the day and simulate a 16/8-h day/night cycle. During the other days, the extra lighting was not switched on to see if there was a difference between days with extra lighting versus days without extra lighting. Control, logging, and steering were carried out automatically by computer (MultiMate series III, Hortiplan).
The algal growth in the bioreactor was monitored online every 30 min with a turbidity meter. The measured turbidity was correlated with a dry weight value based on a correlation described previously (Thoré et al., 2021). In addition to the continuous monitoring by turbidity, the dry weight (g L−1) was determined at the start (Cs) and end (Ce) of each batch and at random moments during the growth periods. For this, samples (5 ml/sample) were filtered over pre-dried and pre-weighed Whatman GF/C glass microfiber filter papers (0.45 µm) and washed. After drying for 24 h at 70°C, the samples were cooled in a desiccator before weighing.
The total volumetric biomass productivity Pv (dry g L−1 d−1) for the batch mode was calculated by the formula:
with Cs and Ce, the start and end biomass (g L−1), respectively, and td was the cultivation period (9 days for total and 1 day for (maximum) daily volumetric biomass productivity). The total areal biomass productivity Pa (dry g m−2 d−1) was calculated as follows:
with 350 L being the volume of the reactor, td being 9 days for the total or 1 day for the (maximum) daily biomass areal productivity, and 14 m2 being the total area covered by the reactor.
The specific growth rate was calculated over the 9-day period:
with Ne being the biomass at the end and Ns being the biomass at the start of the 9-day period and td being 9 days.
To know the daily yield on light (PL), the daily areal productivity (Pa) was divided by the total PAR (PART) received for that specific day, resulting in PL:
The total CO2 injected was measured during each 9-day period by using a mass flow meter (IN-FLOW mass flow meter/controller Bronkhorst, the Netherlands). The theoretical total CO2 fixation (CO2th, g) by the algae for each batch was calculated as follows:
with
The CO2 fixation rate CO2fr was calculated as
To have an idea about the CO2 uptake efficiency, the calculated CO2th was divided by the total CO2 (CO2t, g) injected (influent CO2) and multiplied by 100 to know the utilization efficiency percentage (U%):
The aforementioned formula is a simplified version of the formula:
To compare the differences in temperatures, received PAR, and daily biomass production between the seven batches, a one-way analysis of variance (ANOVA) with post hoc Tukey HSD with a significance level of 0.05 was performed. Normality was tested with a Shapiro–Wilk test. Data are given as mean ± SD.
To analyze the 9-day periods, the daily productivities were compared, and a difference was found (p < 0.05): the period 17–26 April was significantly different from periods 17–26 January 2020 and 27 January–5 February 2020, while the period 27 January–5 February 2020 was also significantly different from the period 10–19 December 2019. To further assess the biomass obtained during the cultivation of C. typhlos in the seven 9-day periods, we looked at the specific growth rate and total and maximum volumetric and areal biomass productivities. Table 1 summarizes these results. The highest growth rate was achieved during the 10–19 December period, which was five times higher than the 17–26 April period. Figure 2 shows that the growth halted after 6 days in the 17–26 April period, leading to a lower growth rate. The highest total volumetric biomass productivity (0.067 g L−1 d−1) was obtained during the period 27 January–5 February 2020. While a maximum biomass productivity of 0.110 g L−1 d−1 was obtained during the period 1–10 April 2019, the total volumetric productivity (0.046 g L−1 d−1) was lower than that of three other periods during the winter (Table 1).
TABLE 1. Volumetric and areal biomass productivities in dry weight of C. typhlos grown in batch for 9-day periods in a 350-L horizontal tubular reactor and the specific growth rates are shown. The specific growth rate (µ) is calculated over the 9-day period. The total volumetric (Pv) and areal (Pa) productivities are calculated over the 9-day period by dividing the total dry weight produced by 9. The maximum volumetric (Max Pv) or areal (Max Pa) productivity is the maximum value obtained for the daily productivity during that 9-day period.
FIGURE 2. Growth curves of C. typhlos during seven different 9-day batch growth tests in a 350-L photobioreactor. The end concentration (Ce) of each growth period is depicted next to the respective lines in g L−1.
A potential explanation for the lower total volumetric productivity during the 1–10 April period could be that a maximum ambient temperature of 30.5°C was reached inside the greenhouse on 7 April 2019, causing temperature stress on the cells and leading to a decline in growth. This can be seen in Figure 2 between days 8 and 9. A similar effect could be observed from day 6 during the period 17–26 April 2019. During this period, the lowest total volumetric biomass productivity (0.010 g L−1 d−1) and growth rate (0.020 d−1) were obtained. This growth period had the highest average temperature (22.5°C), the highest maximum temperature (35.2°C), and several (5) consecutive days with temperatures above 30°C (Figure 3). A stress response at a temperature around 30°C is in line with what others have reported (Teoh et al., 2013; Lukeš et al., 2014). Contrary to Teoh et al. (2013) and Lukeš et al. (2014), we measured the ambient temperature in the greenhouse rather than the culture temperature itself. Since multiple parameters can influence the culture temperature and large differences between ambient and culture temperature are possible (Tredici and Materassi, 1992; González-Camejo et al., 2019), future laboratory and pilot-scale tests that measure both the ambient and culture temperatures are required to fully understand the temperature impact on C. typhlos.
FIGURE 3. Box plot of the temperature for each period. Outliers are shown by dots above the maximum. See also Table 2.
Productivities and growth rates for the Chloromonas species were lower than the literature values: over the nine batches average volumetric and areal productivities of 0.043 ± 0.021 g L−1 d−1 and 1.072 ± 0.514 g m−2 d−1, respectively, were obtained, while growth rates varied between 0.020 and 0.105 d−1. However, a comparison is difficult to obtain due to insufficient information or the different cultivation techniques used (Teoh et al., 2013; Idrissi Abdelkhalek et al., 2016; Hulatt et al., 2017; Onuma et al., 2018; Morales-Sánchez et al., 2020; Peng et al., 2021). Compared to commercially cultivated algae, the production and growth rate is lower and needs to be improved to be competitive (de Vree et al., 2015; Barka and Blecker, 2016; Benedetti et al., 2018; Darvehei et al., 2018; Metsoviti et al., 2019).
Aside from temperature stress, algae, in general, react to light stress (Minhas et al., 2016; Shi et al., 2020; Zheng et al., 2020), although C. typhlos has mechanisms; for example, the production of astaxanthin to cope with high light intensities (Gorton et al., 2007; Remias et al., 2010; Zheng et al., 2020). To estimate the influence of total PAR on the growth of C. typhlos, the total averaged PAR was measured. Table 2 shows the average PAR received during the periods, the average daily yield on light (PL), average temperatures, and whether they differ significantly between growth periods.
TABLE 2. Average total PAR, average daily yield in light (PL) for each growth period, and greenhouse temperatures measured during the batch tests. A significance between groups is also shown with p < 0.05. The identifier is used to show the significant differences between periods.
During the 10–19 February period, the total PAR was higher than the spring growth periods. There was a higher total PAR during the February 2020 period due to a few exceptional sunny days throughout this period and the extra artificial lighting. In addition, the sun screens in the greenhouse did not partly close since the minimal closing limit of 400 W m−2 was most often not reached, contrary to the spring periods. Table 2 shows that the growth period 17–26 of January had the lowest temperature and second lowest total PAR light, yet the total volumetric and areal productivities were above the averages of the growth periods (see Table 1). This shows that even at lower temperatures and sunlight, C. typhlos can still be cultivated at comparable productivities to higher temperature and sunlight conditions.
The total PAR received differed significantly between the growth periods. To normalize the growth for PAR received, we looked at the yield per light. Only period 17–26 April 2019 was significantly different from periods 17–26 January 2020 and 27 January–5 February 2020. The time period of 16–26 April 2019 was also significantly different from all other periods in regard to the temperatures reached (Table 2). The period 17–26 April 2019 was also different than most other growth periods for the total light received (only periods 1–10 April 2019 and 10–19 February 2020 were similar). This shows the influence of higher temperatures (>30°C) on the growth of C. typhlos. Table 2 also shows that at lower temperatures, C. typhlos still had a high yield in light.
Like many cold adapted algae, C. typhlos is a potential source of lipids and carotenoids produced at lower temperatures and high light intensities as a mechanism to protect the cells (Gorton et al., 2007; Remias et al., 2010; Liu and Nakamura, 2019; Hoham and Remias, 2020; Shi et al., 2020; Peng et al., 2021). If C. typhlos can produce sufficient biomass and valuable products such as astaxanthin at a low temperature with high light intensities, it can be a sustainable approach to cultivate microalgae during colder periods without excessive heating.
During our growth experiments, C. typhlos cells were green (Figure 1). We did not notice the typical red color indicative of astaxanthin. All experiments were performed in non-nutrient limiting batches, and no attempts were made during these experiments to stress the cells specifically to produce astaxanthin and color red. As no analysis was performed on the astaxanthin content, we cannot rule out that it was produced at levels too low to give the cells their typical red color. For future work, it will be vital to characterize the specific parameters, such as low nitrogen, that produce red C. typhlos cells as astaxanthin is an important and valuable carotenoid (Leya et al., 2009; Han et al., 2013; Sathasivam et al., 2019).
During the winter period, artificial lights were turned on for several days in each period to simulate a 16/8 day-night regime. These days were then compared to days without artificial light. However, no significant difference in biomass productivity was found between the days with artificial light versus the days without (Figure 4). Several reasons might explain why the extra lighting had no effect. One explanation could be that our 9-day test periods were too short to detect a difference. A second reason could be the high variance of received sunlight between the days. The PAR sum from the sunlight ranged from 1,588 to 5,431 mmol m−2. On days with the addition of artificial light, the PAR sum increased by 10–31%; however, due to the high variance, the effect was limited. A third reason could be the use of insufficient or non-specific lighting. The reactors were constructed in 2014 and equipped with fluorescent lamps (6500 K) that only provided minimal extra lighting during the dark period. However, in recent years, more attention has been given to the use of modern LED lamps with a specific light spectrum to optimize the growth of microalgae (Glemser et al., 2016); using better lights might therefore have a significant impact on growth. A last final reason could be the placing of the lights. They were fixed underneath the PBR tubes and only reached a small area of the total PBR tubes. Placing LED lamps closer to each individual tube could increase the effects substantially.
FIGURE 4. Daily productivity of C. typhlos (g L−1 d−1) and PAR sum of each specific day during the two growth periods. Each square represents 1 day.
Figure 5A shows a typical growth pattern based on turbidity measurements (NTU). A general increase in turbidity can be seen over the course of several days (the black arrow in Figure 5A), indicating an increase in cells and biomass. When looking at a 24-h period, without artificial light added on days 3, 4, and 5, a typical pattern can be observed, pointing toward a diurnal rhythm. The cell cycle of photosynthetic microalgae is influenced by a day-night regime in which cell growth (G1) takes place during the light phase (daytime), and the reproductive, cell division phase (S/M) occurs during the dark period (nighttime) (Cross and Umen, 2015; Ivanov et al., 2019; Zou and Bozhkov, 2021). The NTU profile (Figure 5A) can be linked to this diurnal rhythm. The steady increase in NTU a few hours after sunrise to sunset follows the cell growth: the enlargement of the cells and increase in dry weight (Lien and Knutsen, 1979; Spudich and Sager, 1980; Ostgaard and Jensen, 1982; Bišová and Zachleder, 2014; de Winter et al., 2017b). After sunset and during the night, the NTU fluctuated little, which can be linked to the cells no longer growing but dividing within the parental cell (Harper, 1999; Cross and Umen, 2015; Ivanov et al., 2019; Zou and Bozhkov, 2021). Throughout warmer periods, the NTU decreased more overnight, which can be linked to the loss of biomass during the night. During warmer nights, the respiration rates can be higher than on colder nights (De-Luca et al., 2019; Masojídek et al., 2021), leading to more biomass loss and potentially explaining the more pronounced decrease in NTU during warmer nights.
FIGURE 5. (A) shows the typical growth pattern of C. typhlos observed over several days. A representative period during one of the winter periods is shown here with artificial lights being used to extend the day. Artificial lights were kept on between 6.30–10.30 and 15.30–22.00, although slight variances were possible depending on the real sunset and sunrise times of the specific days. (B) shows the difference in the NTU value calculated for every 30 min (NTUx+30min—NTUx) plotted over time. Arrows indicate specific time points or substantial increases/decreases. The symbol ° denotes the artificial light switching off at sunrise, while the symbol * denotes the artificial lights switching on at sunset. See text for more details.
This nightly biomass loss is often attributed to respiration using compounds like carbohydrates during the night (Ogbonna and Tanaka, 1996; Michels et al., 2014; Edmundson and Huesemann, 2015; Åkerström et al., 2016). Next to the aforementioned, general pattern, a rapid and substantial drop or increase in turbidity, respectively, at sunrise and sunset, is seen. To highlight this, Figure 5B shows the difference in NTU between each half-hour registered. The drop in turbidity at sunrise is indicated with red arrows, while the increase at sunset is visualized with green arrows. A rapid decrease or increase points toward a strong and swift reaction of the algae to the presence or absence of light. The NTU is monitored with a nephelometric sensor that uses white light (400–700 nm) that can readably be used by microalgae (Carvalho et al., 2006; de Mooij et al., 2016). Potentially, C. typhlos is able to activate and upregulate its photosynthetic system very fast, leading to a substantial increase in absorption of light while reducing the scattered light and causing a rapid and substantial drop in turbidity (Thorne and Nannestad, 1959; Kourti, 2000; Gregory, 2009). For extremophiles, a fast activation or deactivation of their metabolism can be crucial to cope with harsh conditions (Harel et al., 2004; Peng et al., 2021).
As daughter cells are released from the parental cell at the end of the dark period (Mihara and Hase, 1971; Vítová and Zachleder, 2005; Harris et al., 2009; Bišová and Zachleder, 2014; Zou and Bozhkov, 2021), although it can extend into the light period (Eschbach et al., 2005), many new cells are present and able to influence the turbidity measurements when their photosynthetic apparatus is turned on at sunrise. See also Figure 1 for daughter cells still inside the parental cell after multiple fission. A similar explanation can be given for the substantial rise in NTU at sunset. As the cells switch their metabolism from the light phase to the dark phase, less light is absorbed, thereby increasing the scattering of light and giving a higher NTU value at sunset and during the night.
When artificial light was provided (days 1, 2, and 6), a similar pattern could be observed. A steep decrease in turbidity was observed when the artificial light was turned on prior to sunset (purple dashed arrows), and an increase was seen when turning off the artificial light after sunset (light green dashed arrow; Figure 5B). Figure 5A shows that the effect of the artificial light was similar but not exactly the same. The steady increase of turbidity (NTU) during the growth phase was lower when only artificial light was present as compared to sunlight (plateau between gray and black arrow). However, the substantial increase in turbidity was similar when turning off the artificial light compared to at sunset (Figure 5B, dashed light green arrows). Figure 5B also shows that after turning on the artificial light before sunrise a second, lower drop in turbidity was observable at sunrise (yellow arrows with the symbol “o” on top). Although no significant effect of artificial light was detected on the biomass production of C. typhlos, artificial light did, however, have a noticeable impact on the growth pattern of C. typhlos.
It is possible to use a continuous turbidity measurement in combination with the correlation between turbidity and dry weight to track the dry weight evolution (Thoré et al., 2021). Our results, however, also indicate that caution is needed when interpreting and linking turbidity measurements with the dry weight of the microalga cultivated. However, the growth (turbidity) pattern of C. typhlos was also different compared to other non-snow algae tested. Both P. purpureum and N. gaditana showed a substantial decrease in turbidity during the night (unpublished data) but no substantial decrease or increase respectively at sunrise and sunset. This could potentially indicate a different and faster response of snow algae to a night and day cycle. Like biomass loss overnight, the influence of the day/night cycles of microalgae and their effect on the cells, cell division, and biomass are still understudied and can vary between algae (Edmundson and Huesemann, 2015; de Winter et al., 2017a; León-Saiki et al., 2018). To optimize microalgae cultivation, the nightly biomass loss and circadian rhythm can be important factors to establish the optimal time of harvesting. Future studies should address this.
To investigate the CO2 fixation and utilization efficiency, the total CO2 injected into the photobioreactor was monitored. Using the total biomass produced during each period, the efficiency of CO2 uptake could be calculated (Table 3).
TABLE 3. Overview of the total CO2 injected during each batch growth test, pH was set at 8, and CO2 was injected on demand to maintain a steady pH. The two periods in bold show the highest CO2 utilization efficiency.
During the periods 10–19 December 2019 and 17–26 January 2020, the CO2 utilization efficiency was the highest at 29.97 and 38.42%, respectively. The other periods achieved lower utilization efficiencies with percentages between 2.4 and 9.11%. The exact reasons for this difference are unclear as many factors can influence the CO2 utilization efficiency (Ryu et al., 2009; Daneshvar et al., 2022). Both periods have the lowest total PAR received (2,379 ± 345 and 2,604 ± 791 mmol/m2); however, they do not differ significantly from the periods 19–28 March 2019 and 10–19 February (Table 2).
A second observation is that the maximum greenhouse temperatures reached during these two periods were also the two lowest registered maximum temperatures, but the average temperature of the period 10–19 December was higher than that of three other periods (Table 2). During our tests, the worst efficiencies were seen during the three warmest periods (19–28 March 2019, 17.46 ± 0.9°C; 1–10 April 2019, 18.4 ± 1.4°C; and 17–26 April 2019, 22.4 ± 2.5°C). This is in line with the lower solubility of CO2 at higher temperatures (Singh and Singh, 2014). While we observed a wide range of CO2 fixation rates and utilization efficiency, they are in accord with what others have already found for other algal species (Tang et al., 2011; Ketheesan and Nirmalakhandan, 2012; Lam et al., 2012). In laboratory conditions and smaller working volumes, one can easily maintain specific parameters such as total PAR, pH, temperature, or a day/night period; however, it is less possible in a greenhouse pilot plant or outdoors. In order to truly find a correlation between different parameters and CO2 utilization to improve the utilization efficiency, more pilot plant studies are needed. CO2 utilization efficiency can be very low and is influenced by many parameters such as the temperature, pH, and growth rate, making the design of a reactor with optimal gas exchange expensive and very difficult (Carvalho et al., 2006; Klinthong et al., 2015; Fu et al., 2019), if not impossible. In different (seasonal) weather conditions outdoors or in a greenhouse, it can particularly be arduous. Thus, a more interesting approach for improved sustainability might be to capture the CO2 in the effluent gas and reuse it for the influent, lowering the total CO2 lost into the air.
The psychrotolerant microalga C. typhlos was successfully cultivated in a greenhouse in a photobioreactor with a working volume of 350 L during several batch periods, ranging from winter to spring. A maximum dry weight and growth rate of 1.082 g L−1 and 0.105 d−1, respectively, were achieved while maximum volumetric and areal productivities of 0.110 g L−1 d−1 and 2.746 g m−2 d−1, respectively, were measured. Being one of the first pilot-scale cultivation tests of a snow alga in a photobioreactor, it is difficult to compare with known data from snow algae. One critical factor, negatively influencing the growth, was shown to be ambient temperatures of >30°C. Lower temperatures (11–15°C), however, had no detrimental effect on the growth. Due to the distinct growth pattern influenced by sunset or artificial light, it will be crucial to determine how to use artificial lights to find the optimal light/dark regime. While these experiments were carried out at a set pH of 8, future tests at different pH values will be crucial to determine the optimal pH and CO2 uptake efficiency. Future tests to optimize the cultivation and production of C. typhlos biomass are, thus, crucial to estimate its commercial value. Furthermore, the production of astaxanthin or PUFAs has to be studied to fully explore the potential of C. typhlos.
The raw data supporting the conclusions of this article will be made available by the authors, without undue reservation.
FS: formal analysis and interpretation, writing—original draft, and writing—review and editing; JS: conceptualization, acquisition of data, and writing—review and editing; RA: formal analysis and writing—review and editing; SM: funding acquisition and writing—review and editing.
This research was funded by NORTH-WEST EUROPE INTERREG, grant number NWE 639 as part of the IDEA project (Implementation and development of economically viable algae-based value chains in North-West Europe).
The authors declare that the research was conducted in the absence of any commercial or financial relationships that could be construed as a potential conflict of interest.
All claims expressed in this article are solely those of the authors and do not necessarily represent those of their affiliated organizations, or those of the publisher, the editors, and the reviewers. Any product that may be evaluated in this article, or claim that may be made by its manufacturer, is not guaranteed or endorsed by the publisher.
The authors would like to thank E. Gebruers, I. Noyens, and S. Goossens for helping with sampling for the acquisition of data, A. Wuyts for helping with the cultivation and upscaling of C. typhlos, and A. De Cuyper and E. Van Cantfort for improvement of the manuscript. Furthermore, we would also like to thank the reviewers for aiding in improving the manuscript.
Abo, B. O., Odey, E. A., Bakayoko, M., and Kalakodio, L. (2019). Microalgae to Biofuels Production: a Review on Cultivation, Application and Renewable Energy. Rev. Environ. Health 34, 91–99. doi:10.1515/reveh-2018-0052
Adamczyk, M., Lasek, J., and Skawińska, A. (2016). CO2 Biofixation and Growth Kinetics of Chlorella Vulgaris and Nannochloropsis Gaditana. Appl. Biochem. Biotechnol. 179, 1248–1261. doi:10.1007/s12010-016-2062-3
Åkerström, A. M., Mortensen, L. M., Rusten, B., and Gislerød, H. R. (2016). Biomass Production and Removal of Ammonium and Phosphate by Chlorella Sp. In Sludge Liquor at Natural Light and Different Levels of Temperature Control. Springerplus 5, 676. doi:10.1186/s40064-016-2266-6
Araújo, R., Vázquez Calderón, F., Sánchez López, J., Azevedo, I. C., Bruhn, A., Fluch, S., et al. (2021). Current Status of the Algae Production Industry in Europe: An Emerging Sector of the Blue Bioeconomy. Front. Mar. Sci. 7, 626389. doi:10.3389/fmars.2020.626389
Barka, A., and Blecker, C. (2016). Microalgae as a Potential Source of Single-Cell Proteins. A Review. Biotechnol. Agron. Soc. Environ. 20, 427–436. doi:10.25518/1780-4507.13132
Benedetti, M., Vecchi, V., Barera, S., and Dall’Osto, L. (2018). Biomass from Microalgae: the Potential of Domestication towards Sustainable Biofactories. Microb. Cell Fact. 17, 173. doi:10.1186/s12934-018-1019-3
Bišová, K., and Zachleder, V. (2014). Cell-cycle Regulation in Green Algae Dividing by Multiple Fission. J. Exp. Bot. 65, 2585–2602. doi:10.1093/jxb/ert466
Carvalho, A. P., Meireles, L. A., and Malcata, F. X. (2006). Microalgal Reactors: A Review of Enclosed System Designs and Performances. Biotechnol. Prog. 22, 1490–1506. doi:10.1021/bp060065r
Cheregi, O., Ekendahl, S., Engelbrektsson, J., Strömberg, N., Godhe, A., and Spetea, C. (2019). Microalgae Biotechnology in Nordic Countries - the Potential of Local Strains. Physiol. Plant. 166, 438–450. doi:10.1111/ppl.12951
Chisti, Y. (2007). Biodiesel from Microalgae. Biotechnol. Adv. 25, 294–306. doi:10.1016/j.biotechadv.2007.02.001
Cross, F. R., and Umen, J. G. (2015). TheChlamydomonascell Cycle. Plant J. 82, 370–392. doi:10.1111/tpj.12795
Cvetkovska, M., Hüner, N. P. A., and Smith, D. R. (2017). Chilling Out: the Evolution and Diversification of Psychrophilic Algae with a Focus on Chlamydomonadales. Polar Biol. 40, 1169–1184. doi:10.1007/s00300-016-2045-4
Daneshvar, E., Wicker, R. J., Show, P.-L., and Bhatnagar, A. (2022). Biologically-mediated Carbon Capture and Utilization by Microalgae towards Sustainable CO2 Biofixation and Biomass Valorization - A Review. Chem. Eng. J. 427, 130884. doi:10.1016/j.cej.2021.130884
Darvehei, P., Bahri, P. A., and Moheimani, N. R. (2018). Model Development for the Growth of Microalgae: A Review. Renew. Sustain. Energy Rev. 97, 233–258. doi:10.1016/j.rser.2018.08.027
de Mooij, T., de Vries, G., Latsos, C., Wijffels, R. H., and Janssen, M. (2016). Impact of Light Color on Photobioreactor Productivity. Algal Res. 15, 32–42. doi:10.1016/j.algal.2016.01.015
de Vree, J. H., Bosma, R., Janssen, M., Barbosa, M. J., and Wijffels, R. H. (2015). Comparison of Four Outdoor Pilot-Scale Photobioreactors. Biotechnol. Biofuels 8, 215. doi:10.1186/s13068-015-0400-2
de Winter, L., Cabanelas, I. T. D., Martens, D. E., Wijffels, R. H., and Barbosa, M. J. (2017a). The Influence of Day/night Cycles on Biomass Yield and Composition of Neochloris Oleoabundans. Biotechnol. Biofuels 10, 104. doi:10.1186/s13068-017-0762-8
de Winter, L., Cabanelas, I. T. D., Órfão, A. N., Vaessen, E., Martens, D. E., Wijffels, R. H., et al. (2017b). The Influence of Day Length on Circadian Rhythms of Neochloris Oleoabundans. Algal Res. 22, 31–38. doi:10.1016/j.algal.2016.12.001
De-Luca, R., Bezzo, F., Béchet, Q., and Bernard, O. (2019). Meteorological Data-Based Optimal Control Strategy for Microalgae Cultivation in Open Pond Systems. Complexity 2019, 1–12. doi:10.1155/2019/4363895
Dolganyuk, V., Belova, D., Babich, O., Prosekov, A., Ivanova, S., Katserov, D., et al. (2020). Microalgae: A Promising Source of Valuable Bioproducts. Biomolecules 10, 1153. doi:10.3390/biom10081153
Edmundson, S. J., and Huesemann, M. H. (2015). The Dark Side of Algae Cultivation: Characterizing Night Biomass Loss in Three Photosynthetic Algae, Chlorella Sorokiniana, Nannochloropsis Salina and Picochlorum Sp. Algal Res. 12, 470–476. doi:10.1016/j.algal.2015.10.012
Eschbach, E., John, U., Reckermann, M., Cembella, A., Edvardsen, B., and Medlin, L. (2005). Cell Cycle Dependent Expression of Toxicity by the Ichthyotoxic Prymnesiophyte Chrysochromulina Polylepis. Aquat. Microb. Ecol. 39, 85–95. doi:10.3354/ame039085
Fu, J., Huang, Y., Liao, Q., Xia, A., Fu, Q., and Zhu, X. (2019). Photo-bioreactor Design for Microalgae: A Review from the Aspect of CO2 Transfer and Conversion. Bioresour. Technol. 292, 121947. doi:10.1016/j.biortech.2019.121947
Garrido-Cardenas, J. A., Manzano-Agugliaro, F., Acien-Fernandez, F. G., and Molina-Grima, E. (2018). Microalgae Research Worldwide. Algal Res. 35, 50–60. doi:10.1016/j.algal.2018.08.005
Glemser, M., Heining, M., Schmidt, J., Becker, A., Garbe, D., Buchholz, R., et al. (2016). Application of Light-Emitting Diodes (LEDs) in Cultivation of Phototrophic Microalgae: Current State and Perspectives. Appl. Microbiol. Biotechnol. 100, 1077–1088. doi:10.1007/s00253-015-7144-6
González-Camejo, J., Aparicio, S., Ruano, M. v., Borrás, L., Barat, R., and Ferrer, J. (2019). Effect of Ambient Temperature Variations on an Indigenous Microalgae-Nitrifying Bacteria Culture Dominated by Chlorella. Bioresour. Technol. 290, 121788. doi:10.1016/j.biortech.2019.121788
Gorton, H. L., Williams, W. E., and Vogelmann, T. C. (2007). The Light Environment and Cellular Optics of the Snow Alga Chlamydomonas Nivalis (Bauer) Wille†. Photochem. Photobiol. 73, 611–620. doi:10.1562/0031-8655(2001)0730611tleaco2.0.co2
Gregory, J. (2009). Monitoring Particle Aggregation Processes. Adv. Colloid Interface Sci. 147-148, 109–123. doi:10.1016/j.cis.2008.09.003
Guiry, M. D. (2012). How Many Species of Algae Are There? J. Phycol. 48, 1057–1063. doi:10.1111/j.1529-8817.2012.01222.x
Han, D., Li, Y., and Hu, Q. (2013). Astaxanthin in Microalgae: Pathways, Functions and Biotechnological Implications. Algae 28, 131–147. doi:10.4490/algae.2013.28.2.131
Harel, Y., Ohad, I., and Kaplan, A. (2004). Activation of Photosynthesis and Resistance to Photoinhibition in Cyanobacteria within Biological Desert Crust. Plant Physiol. 136, 3070–3079. doi:10.1104/pp.104.047712
Harper, J. D. I. (1999). “Chlamydomonas Cell Cycle Mutants,” in International Review of Cytology. Editor K. W. Jeon (San Diego: Academic Press), 131–176. doi:10.1016/S0074-7696(08)61387-X
Harris, E. H., Stern, D. B., and Witman, G. B. (2009). “Cell Division,” in The Chlamydomonas Sourcebook. Editors E. H. Harris, D. B. Stern, and G. B. Witman. Second Edition (London: Academic Press), 65–87. doi:10.1016/B978-0-12-370873-1.00003-4
Hoham, R. W., and Remias, D. (2020). Snow and Glacial Algae: A Review1. J. Phycol. 56, 264–282. doi:10.1111/jpy.12952
Hulatt, C. J., Berecz, O., Egeland, E. S., Wijffels, R. H., and Kiron, V. (2017). Polar Snow Algae as a Valuable Source of Lipids? Bioresour. Technol. 235, 338–347. doi:10.1016/j.biortech.2017.03.130
Idrissi Abdelkhalek, E. A., Mohamed, B., Mohammed, A. M., and Lotfi, A. (2016). Growth Performance and Biochemical Composition of Nineteen Microalgae Collected from Different Moroccan Reservoirs. Medit. Mar. Sci. 17, 323–332. doi:10.12681/mms.1320
Ivanov, I. N., Vítová, M., and Bišová, K. (2019). Growth and the Cell Cycle in Green Algae Dividing by Multiple Fission. Folia Microbiol. 64, 663–672. doi:10.1007/s12223-019-00741-z
Ketheesan, B., and Nirmalakhandan, N. (2012). Feasibility of Microalgal Cultivation in a Pilot-Scale Airlift-Driven Raceway Reactor. Bioresour. Technol. 108, 196–202. doi:10.1016/j.biortech.2011.12.146
Khan, M. I., Shin, J. H., and Kim, J. D. (2018). The Promising Future of Microalgae: Current Status, Challenges, and Optimization of a Sustainable and Renewable Industry for Biofuels, Feed, and Other Products. Microb. Cell Fact. 17, 1–36. doi:10.1186/s12934-018-0879-x
Klinthong, W., Yang, Y.-H., Huang, C.-H., and Tan, C.-S. (2015). A Review: Microalgae and Their Applications in CO2 Capture and Renewable Energy. Aerosol Air Qual. Res. 15, 712–742. doi:10.4209/aaqr.2014.11.0299
Kourti, T. (2000). “Turbidimetry in Particle Size Analysis,” in Encyclopedia of Analytical Chemistry (New York: John Wiley & Sons). doi:10.1002/9780470027318.a1517
Kvíderová, J. (2010). Characterization of the Community of Snow Algae and Their Photochemical Performancein Situin the Giant Mountains, Czech Republic. Arct. Antarct. Alp. Res. 42, 210–218. doi:10.1657/1938-4246-42.2.210
Kvíderová, J., Shukla, S. P., Pushparaj, B., and Elster, J. (2017). “Perspectives of Low-Temperature Biomass Production of Polar Microalgae and Biotechnology Expansion into High Latitudes,” in Psychrophiles: From Biodiversity to Biotechnology. Second Edition (New York: Springer International Publishing), 585–600. doi:10.1007/978-3-319-57057-0_25
Lam, M. K., Lee, K. T., and Mohamed, A. R. (2012). Current Status and Challenges on Microalgae-Based Carbon Capture. Int. J. Greenh. Gas Control 10, 456–469. doi:10.1016/j.ijggc.2012.07.010
León-Saiki, G. M., Cabrero Martí, T., van der Veen, D., Wijffels, R. H., and Martens, D. E. (2018). The Impact of Day Length on Cell Division and Efficiency of Light Use in a Starchless Mutant of Tetradesmus Obliquus. Algal Res. 31, 387–394. doi:10.1016/j.algal.2018.02.027
Leya, T., Rahn, A., Lütz, C., and Remias, D. (2009). Response of Arctic Snow and Permafrost Algae to High Light and Nitrogen Stress by Changes in Pigment Composition and Applied Aspects for Biotechnology. FEMS Microbiol. Ecol. 67, 432–443. doi:10.1111/j.1574-6941.2008.00641.x
Lien, T., and Knutsen, G. (1979). Synchronous Growth of Chlamydomonas Reinhardtii (Chlorophyceae): a Review of Optimal Conditions1. J. Phycol. 15, 191–200. doi:10.1111/j.1529-8817.1979.tb02984.x
Liu, Y. c., and Nakamura, Y. (2019). Triacylglycerol Production in the Snow Algae Chlamydomonas Nivalis under Different Nutrient Conditions. Lipids 54, 255–262. doi:10.1002/lipd.12143
Lukeš, M., Procházková, L., Shmidt, V., Nedbalová, L., and Kaftan, D. (2014). Temperature Dependence of Photosynthesis and Thylakoid Lipid Composition in the Red Snow Alga Chlamydomonas Cf. Nivalis (Chlorophyceae). FEMS Microbiol. Ecol. 89, 303–315. doi:10.1111/1574-6941.12299
Mandalam, R. K., and Palsson, B. (1998). Elemental Balancing of Biomass and Medium Composition Enhances Growth Capacity in High-densityChlorella Vulgaris Cultures. Biotechnol. Bioeng. 59, 605–611. doi:10.1002/(sici)1097-0290(19980905)59:5<605::aid-bit11>3.0.co;2-8
Masojídek, J., Ranglová, K., Lakatos, G. E., Silva Benavides, A. M., and Torzillo, G. (2021). Variables Governing Photosynthesis and Growth in Microalgae Mass Cultures. Processes 9, 820. doi:10.3390/pr9050820
Mata, T. M., Martins, A. A., and Caetano, N. S. (2010). Microalgae for Biodiesel Production and Other Applications: A Review. Renew. Sustain. Energy Rev. 14, 217–232. doi:10.1016/j.rser.2009.07.020
Matsumoto, M., Nojima, D., Nonoyama, T., Ikeda, K., Maeda, Y., Yoshino, T., et al. (2017). Outdoor Cultivation of Marine Diatoms for Year-Round Production of Biofuels. Mar. Drugs 15, 94. doi:10.3390/md15040094
Metsoviti, M. N., Papapolymerou, G., Karapanagiotidis, I. T., and Katsoulas, N. (2019). Comparison of Growth Rate and Nutrient Content of Five Microalgae Species Cultivated in Greenhouses. Plants 8, 279. doi:10.3390/plants8080279
Michels, M. H. A., Slegers, P. M., Vermuë, M. H., and Wijffels, R. H. (2014). Effect of Biomass Concentration on the Productivity of Tetraselmis Suecica in a Pilot-Scale Tubular Photobioreactor Using Natural Sunlight. Algal Res. 4, 12–18. doi:10.1016/j.algal.2013.11.011
Mihara, S., and Hase, E. (1971). Studies on the Vegetative Life Cycle of Chlamydomonas Reinhardi Dangeard in Synchronous Culture I. Some Characteristics of the Cell Cycle. Plant Cell Physiology 12 (2), 225–236. doi:10.1093/oxfordjournals.pcp.a074616
Minhas, A. K., Hodgson, P., Barrow, C. J., and Adholeya, A. (2016). A Review on the Assessment of Stress Conditions for Simultaneous Production of Microalgal Lipids and Carotenoids. Front. Microbiol. 7, 546. doi:10.3389/fmicb.2016.00546
Mirón, A. S., Garcı́a, M. C. C., Gómez, A. C., Camacho, F. G., Grima, E. M., and Chisti, Y. (2003). Shear Stress Tolerance and Biochemical Characterization of Phaeodactylum Tricornutum in Quasi Steady-State Continuous Culture in Outdoor Photobioreactors. Biochem. Eng. J. 16, 287–297. doi:10.1016/S1369-703X(03)00072-X
Moody, J. W., McGinty, C. M., and Quinn, J. C. (2014). Global Evaluation of Biofuel Potential from Microalgae. Proc. Natl. Acad. Sci. U.S.A. 111, 8691–8696. doi:10.1073/pnas.1321652111
Morales-Sánchez, D., Schulze, P. S. C., Kiron, V., and Wijffels, R. H. (2020). Production of Carbohydrates, Lipids and Polyunsaturated Fatty Acids (PUFA) by the Polar Marine Microalga Chlamydomonas Malina RCC2488. Algal Res. 50, 102016. doi:10.1016/j.algal.2020.102016
Mosser, J. L., Mosser, A. G., and Brock, T. D. (1977). Photosynthesis in the Snow: the Alga Chlamydomonas Nivalis (Chlorophyceae)1. J. Phycol. 13, 22–27. doi:10.1111/j.1529-8817.1977.tb02881.x
Ogbonna, J. C., and Tanaka, H. (1996). Night Biomass Loss and Changes in Biochemical Composition of Cells during Light/dark Cyclic Culture of Chlorella Pyrenoidosa. J. Ferment. Bioeng. 82, 558–564. doi:10.1016/S0922-338X(97)81252-4
Onuma, Y., Takeuchi, N., Tanaka, S., Nagatsuka, N., Niwano, M., and Aoki, T. (2018). Observations and Modelling of Algal Growth on a Snowpack in North-Western Greenland. Cryosphere 12, 2147–2158. doi:10.5194/tc-12-2147-2018
Ostgaard, K., and Jensen, A. (1982). Diurnal and Circadian Rhythms in the Turbidity of Growing Skeletonema Costatum Cultures. Mar. Biol. 66, 261–268. doi:10.1007/BF00397031
Patil, P. D., Reddy, H., Muppaneni, T., Mannarswamy, A., Schuab, T., Holguin, F. O., et al. (2012). Power Dissipation in Microwave-Enhanced In Situ Transesterification of Algal Biomass to Biodiesel. Green Chem. 14, 809–818. doi:10.1039/c2gc16195h
Peng, Z., Liu, G., and Huang, K. (2021). Cold Adaptation Mechanisms of a Snow Alga Chlamydomonas Nivalis during Temperature Fluctuations. Front. Microbiol. 11, 611080. doi:10.3389/fmicb.2020.611080
Procházková, L., Remias, D., Řezanka, T., and Nedbalová, L. (2018). Chloromonas Nivalis Subsp. Tatrae, Subsp. Nov. (Chlamydomonadales, Chlorophyta): Re-examination of a Snow Alga from the High Tatra Mountains (Slovakia). Fottea 18, 1–18. doi:10.5507/fot.2017.010
Quinn, J., de Winter, L., and Bradley, T. (2011). Microalgae Bulk Growth Model with Application to Industrial Scale Systems. Bioresour. Technol. 102, 5083–5092. doi:10.1016/j.biortech.2011.01.019
Remias, D., Karsten, U., Lütz, C., and Leya, T. (2010). Physiological and Morphological Processes in the Alpine Snow Alga Chloromonas Nivalis (Chlorophyceae) during Cyst Formation. Protoplasma 243, 73–86. doi:10.1007/s00709-010-0123-y
Remias, D., Lütz-Meindl, U., and Lütz, C. (2005). Photosynthesis, Pigments and Ultrastructure of the Alpine Snow algaChlamydomonas Nivalis. Eur. J. Phycol. 40, 259–268. doi:10.1080/09670260500202148
Remias, D., Pichrtová, M., Pangratz, M., Lütz, C., and Holzinger, A. (2016). Ecophysiology, Secondary Pigments and Ultrastructure ofChlainomonassp. (Chlorophyta) from the European Alps Compared withChlamydomonas Nivalisforming Red Snow. FEMS Microbiol. Ecol. 92, fiw030. doi:10.1093/femsec/fiw030
Rendón-Castrillón, L., Ramírez-Carmona, M., Ocampo-López, C., and Giraldo-Aristizabal, R. (2021). Evaluation of the Operational Conditions in the Production and Morphology of Chlorella Sp. Braz. J. Biol. 81, 202–209. doi:10.1590/1519-6984.228874
Richmond, A. (2000). Microalgal Biotechnology at the Turn of the Millennium: A Personal View. J. Appl. Phycol. 12, 441–451. doi:10.1023/a:1008123131307
Ryu, H. J., Oh, K. K., and Kim, Y. S. (2009). Optimization of the Influential Factors for the Improvement of CO2 Utilization Efficiency and CO2 Mass Transfer Rate. J. Industrial Eng. Chem. 15, 471–475. doi:10.1016/j.jiec.2008.12.012
Sathasivam, R., Radhakrishnan, R., Hashem, A., and Abd_Allah, E. F. (2019). Microalgae Metabolites: A Rich Source for Food and Medicine. Saudi J. Biol. Sci. 26, 709–722. doi:10.1016/j.sjbs.2017.11.003
Sayre, R. (2010). Microalgae: The Potential for Carbon Capture. BioScience 60, 722–727. doi:10.1525/bio.2010.60.9.9
Shi, T.-Q., Wang, L.-R., Zhang, Z.-X., Sun, X.-M., and Huang, H. (2020). Stresses as First-Line Tools for Enhancing Lipid and Carotenoid Production in Microalgae. Front. Bioeng. Biotechnol. 8, 610. doi:10.3389/fbioe.2020.00610
Singh, S. P., and Singh, P. (2014). Effect of CO2 Concentration on Algal Growth: A Review. Renew. Sustain. Energy Rev. 38, 172–179. doi:10.1016/j.rser.2014.05.043
Singh, U. B., and Ahluwalia, A. S. (2013). Microalgae: a Promising Tool for Carbon Sequestration. Mitig. Adapt Strateg. Glob. Change 18, 73–95. doi:10.1007/s11027-012-9393-3
Spudich, J. L., and Sager, R. (1980). Regulation of the Chlamydomonas Cell Cycle by Light and Dark. J. Cell Biol. 85, 136–145. doi:10.1083/jcb.85.1.136
Suzuki, H., Hulatt, C. J., Wijffels, R. H., and Kiron, V. (2019). Growth and LC-PUFA Production of the Cold-Adapted Microalga Koliella antarctica in Photobioreactors. J. Appl. Phycol. 31, 981–997. doi:10.1007/s10811-018-1606-z
Tang, D., Han, W., Li, P., Miao, X., and Zhong, J. (2011). CO2 Biofixation and Fatty Acid Composition of Scenedesmus Obliquus and Chlorella Pyrenoidosa in Response to Different CO2 Levels. Bioresour. Technol. 102, 3071–3076. doi:10.1016/j.biortech.2010.10.047
Teoh, M.-L., Phang, S.-M., and Chu, W.-L. (2013). Response of Antarctic, Temperate, and Tropical Microalgae to Temperature Stress. J. Appl. Phycol. 25, 285–297. doi:10.1007/s10811-012-9863-8
Thoré, E. S. J., Schoeters, F., Spit, J., and van Miert, S. (2021). Real-Time Monitoring of Microalgal Biomass in Pilot-Scale Photobioreactors Using Nephelometry. Processes 9, 1530. doi:10.3390/pr9091530
Thorne, R. S. W., and Nannestad, I. (1959). Some Considerations on the Physical Significance of Turbidity Estimates. J. Inst. Brew. 65, 175–188. doi:10.1002/j.2050-0416.1959.tb01443.x
Tredici, M. R., and Materassi, R. (1992). From Open Ponds to Vertical Alveolar Panels: the Italian Experience in the Development of Reactors for the Mass Cultivation of Phototrophic Microorganisms. J. Appl. Phycol. 4, 221–231. doi:10.1007/BF02161208
Tredici, M. R. (2010). Photobiology of Microalgae Mass Cultures: Understanding the Tools for the Next Green Revolution. Biofuels 1, 143–162. doi:10.4155/bfs.09.10
Vale, M. A., Ferreira, A., Pires, J. C. M., and Gonçalves, A. L. (2020). “CO2 Capture Using Microalgae,” in Advances in Carbon Capture (Cambridge: Elsevier), 381–405. doi:10.1016/b978-0-12-819657-1.00017-7
Varshney, P., Mikulic, P., Vonshak, A., Beardall, J., and Wangikar, P. P. (2015). Extremophilic Micro-algae and Their Potential Contribution in Biotechnology. Bioresour. Technol. 184, 363–372. doi:10.1016/j.biortech.2014.11.040
Vecchi, V., Barera, S., Bassi, R., and Dall’Osto, L. (2020). Potential and Challenges of Improving Photosynthesis in Algae. Plants 9, 67. doi:10.3390/plants9010067
Vítová, M., and Zachleder, V. (2005). Points of Commitment to Reproductive Events as a Tool for Analysis of the Cell Cycle in Synchronous Cultures of Algae. Folia Microbiol. 50, 141–149. doi:10.1007/BF02931463
Wang, Y., Tibbetts, S., and McGinn, P. (2021). Microalgae as Sources of High-Quality Protein for Human Food and Protein Supplements. Foods 10, 3002. doi:10.3390/foods10123002
Zheng, Y., Xue, C., Chen, H., He, C., and Wang, Q. (2020). Low-Temperature Adaptation of the Snow Alga Chlamydomonas Nivalis Is Associated with the Photosynthetic System Regulatory Process. Front. Microbiol. 11, 1233. doi:10.3389/fmicb.2020.01233
Keywords: biomass production, greenhouse, microalgae, psychrotolerant, year-round cultivation, CO2 utilization, cold climate
Citation: Schoeters F, Spit J, Azizah RN and Van Miert S (2022) Pilot-Scale Cultivation of the Snow Alga Chloromonas typhlos in a Photobioreactor. Front. Bioeng. Biotechnol. 10:896261. doi: 10.3389/fbioe.2022.896261
Received: 14 March 2022; Accepted: 02 May 2022;
Published: 09 June 2022.
Edited by:
Kanhaiya Kumar, Norwegian University of Science and Technology, NorwayReviewed by:
Dominik Refardt, Zurich University of Applied Sciences, SwitzerlandCopyright © 2022 Schoeters, Spit, Azizah and Van Miert. This is an open-access article distributed under the terms of the Creative Commons Attribution License (CC BY). The use, distribution or reproduction in other forums is permitted, provided the original author(s) and the copyright owner(s) are credited and that the original publication in this journal is cited, in accordance with accepted academic practice. No use, distribution or reproduction is permitted which does not comply with these terms.
*Correspondence: Floris Schoeters, ZmxvcmlzLnNjaG9ldGVyc0B0aG9tYXNtb3JlLmJl
Disclaimer: All claims expressed in this article are solely those of the authors and do not necessarily represent those of their affiliated organizations, or those of the publisher, the editors and the reviewers. Any product that may be evaluated in this article or claim that may be made by its manufacturer is not guaranteed or endorsed by the publisher.
Research integrity at Frontiers
Learn more about the work of our research integrity team to safeguard the quality of each article we publish.