- State Key Laboratory of Polymer Materials Engineering, Polymer Research Institute of Sichuan University, Chengdu, China
Advanced polymer processing has received extensive attention due to its unique control of complex force fields and customizability, and has been widely applied in various fields, especially in preparation of functional devices for bioengineering and biotechnology. This review aims to provide an overview of various advanced polymer processing techniques including rotation extrusion, electrospinning, micro injection molding, 3D printing and their recent progresses in the field of cell proliferation, bone repair, and artificial blood vessels. This review dose not only attempts to provide a comprehensive understanding of advanced polymer processing, but also aims to guide for design and fabrication of next-generation device for biomedical engineering.
Introduction
With the development of polymer processing, functional polymer materials and devices for biomedical engineering have ushered in a huge development, and new concepts, new technologies and new methods are still being explored on a global scale (Abdolmaleki et al., 2021; Guo et al., 2021; Zhang et al., 2021; Chen et al., 2022). Among them, the ingenious combination of advanced polymer processing techniques and functional polymer composites demonstrate epoch-making significance of next-generation medical device such as cell proliferation (Motealleh et al., 2021), bone repair, (Xue et al., 2021), drug release, (Kim et al., 2021), artificial blood vessels (El-Ghazali et al., 2021) and thus have attracted extensive attention from academia and industry.
The performance of polymer materials and device is not only derived from the structural characteristics of the molecular chain, but also strongly depends on the morphology and structure formed by the effect of stress and temperature during processing (Ho et al., 2012; Zhang et al., 2019). Advanced polymer processing technology can generate a unique stress and temperature filed with the assistance of special processing equipment and thus enables polymer materials to exhibit excellent properties which are different from conventional processing, exemplified by the helical stress field formed in rotation extrusion (Pi et al., 2019; Sun et al., 2019), the ultra-high shear in micro-injection molding (Jiang et al., 2018; Zhao et al., 2022) and so on. These materials and device provide a broader toolbox for practical clinical application.
In this work, we reviewed the recent advanced processing technology stepping from clarifying the special stress and temperature field during processing and then demonstrated its current and potential applications in cell proliferation, bone repair engineering and artificial blood vessels. In addition, based on the outlined challenges and limitations of advanced processing technology, we summarized the prospects and development directions of advanced processing technology to provide feasible ideas for inspiring future work.
Rotation Extrusion Technology
Principle of Rotation Extrusion Technology
Differing from one-dimensional axial flow in conventional extrusion process (Lu et al., 2020), helical stress filed is generated in rotation extrusion through the controlled rotation of the die and the mandrel, which realizes the multi-dimensional controllable flow of the melt and improves the functionality of the material (Li Y. et al., 2019). Figure 1A shows the schematic illustration of the rotation extrusion rheometer (Li et al., 2021a). The rotation extrusion rheometer can accurately control and exert hoop stress during extrusion, and thus helical motion can be achieved in combination with extrusion and traction. At the same time, the cooling media with various type, pressure and temperature can be adjusted into the polymer tube through the hollow mandrel, so as to regulate the temperature gradient and solidify the off-axis reinforcement phase structure in the polymer tube. During rotation extrusion, fiber network with different helical feature can form (Figures 1B,C) (Li et al., 2021a) Specifically, unidirectional orientation off the extrusion direction can form during syntropic rotation extrusion (ST) and mandrel rotation extrusion (MT), while intersected network with a gradient layer forms in reverse rotation extrusion (RT). The former can induce the reinforcement structure to deviate from the axial orientation and thus to reinforce the hoop strength of the tube, while the latter can form functional network inside the tube and broaden the application. During rotation extrusion process, the additional hoop stress field exhibits great significance in regulating the crystal morphology of the polymer. The increased shear from hoop direction can promote the stretching of molecular chains to enhance crystallinity and simultaneously promote the formation of shish-kebab structure (Yang et al., 2019), in which the anisotropic nature endows materials with higher strength and modulus.
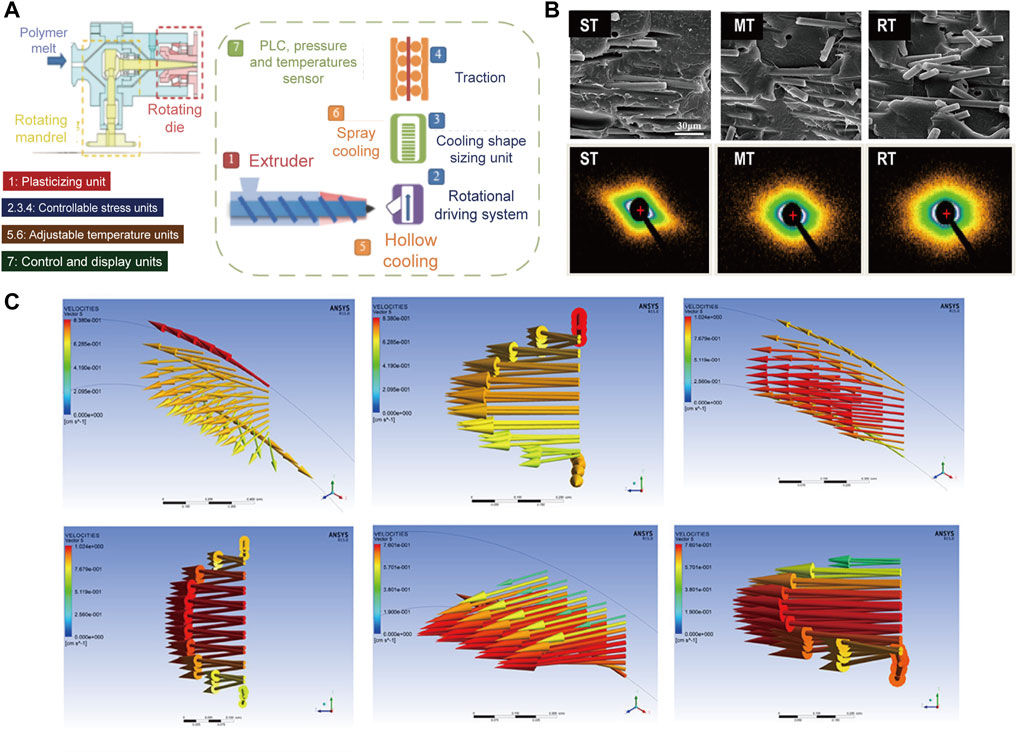
FIGURE 1. (A) Schematic diagram of rotation extrusion rheometer; (B) Scanning electron microscopy (SEM) and small-angle X-ray scattering (SAXS) results of the MT, ST, and RT tubes; (C) Flow characteristics of MT, ST, and RT obtained from Polyflow (Li et al., 2021b). Copyright 2021, American Chemical Society.
Application of Rotation Extrusion Technology in Preparing Medical Tube
Medical catheters are the most important application direction of polymer tubes (Geyer et al., 2019), mainly including surgical catheters (Hu et al., 2018), treatment and nursing catheters (Neoh et al., 2017), and interventional medical catheters (Liu et al., 2021). Interventional therapy is a newly developed medical technology, where catheters and other interventional medical devices can enter the human body to reach the target lesion location and to achieve precise and efficient treatment effects (Jung et al., 2020; Hwang et al., 2022). However, due to the thin wall, the mechanical properties of polymer tubes are generally low. Tubes are prone to kinking and collapse under external loads, causing fatal damage inside the human body (Wang et al., 2020a). Therefore, enhancement of mechanical properties within a limited size is an important development direction of the polymer tube.
The helical force field during rotation extrusion can induce the reinforcing fibers to deviate from the axial alignment, and parallel to the maximum stress in the torque deformation. This unique morphology can endows the microtube with excellent torsional strength to achieve annular strengthening (Nie, 2013; Sun et al., 2019). Liu(Liu et al., 2014) conducted a pioneering work in preparing medical tube with reinforced hoop strength via rotation extrusion, where polystyrene (PS) microfibers with high molecular orientation were helical aligned by the helical convergent flow during polybutene-1 (PB-1) tubing processing (Figure 2A). Moreover, the mechanical strength of the microtubules can be further improved by further controlling the rotation mode of the rotation extrusion. Liu (Liu et al., 2015) demonstrated that a reverse helical configuration of PS microfibers was constructed by forming a reverse helical convergent flow in reverse rotation extrusion, which effectively eliminating the relative slip between fibers. The prepared PS/PB-1 composite tube exhibits significantly enhanced mechanical properties in axial direction (40.3 PMa) and hoop direction (34.9 MPa), which are 34.8 and 48.5% higher than those prepared by conventional extrusion, respectively (Figure 2B).
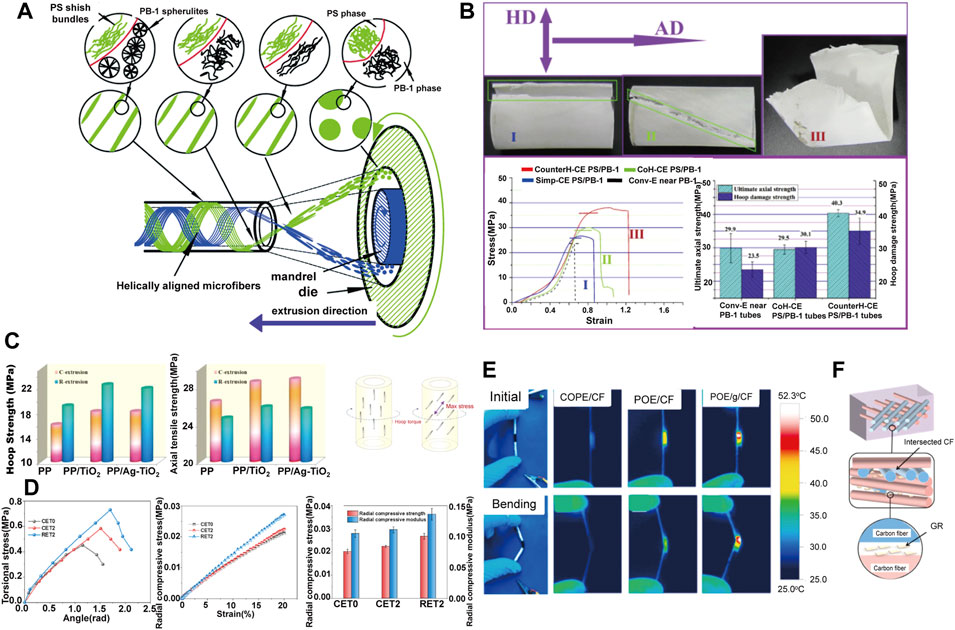
FIGURE 2. (A) Schematic diagram of in situ fibrillation by rotation extrusion technology (Liu et al., 2014) Copyright 2014, Elsevier; (B) Failed composite tubes with different microfiber’s configuration and the corresponding stress–strain data (Liu et al., 2015) Copyright 2015, Elsevier; (C) Hoop strength and axial strength of the tubes prepared at different conditions and stress analysis of hoop torque imposed on polymer tubes (Zhang K. et al., 2018) Copyright 2018, Elsevier; (D) Radial mechanical properties of POE/MMT tubes (Yang C. et al., 2021) Copyright 2021, Elsevier; (E) Infrared thermal images at the maximum temperature under voltage of 5 V with or without bending strain of various tubes. POE/CF referred to convention extruded tubes; (F) The schematic illustration of the confining effect of the GR on CF network (Li et al., 2021b) Copyright 2021, Elsevier.
Similarly, fillers with anisotropic nature are ideal candidate for rotation extrusion. Zhang and Li (Li X. et al., 2015; Zhang et al., 2018b; Pi et al., 2020) enhanced the circumferential strength of polypropylene (PP)/TiO2 tubes by rotation extrusion (Figure 2C). It is notable that these work proved the universality and customizability of the rotation extrusion technology, since by adjusting the type of filler to give the pipeline additional functionality. For instance, TiO2 can improve the antibacterial ability of the pipeline (Alotaibi et al., 2020). Yang (Yang C. et al., 2021) also fabricated reinforced polyolefin elastomer (POE)/montmorillonite (MMT) tubes by rotation extrusion. MMT formed a natural nacre-like structure under helical force field, which improves the hoop strength of the tube, and at the same time shows a strong ability to resist radial compression deformation (Figure 2D). The enhanced anti-deformation ability perfectly fits the needs of bone repair materials, establishing a foundation for the application in the field of bone repair. Moreover, reverse rotation can form a multi-axial ordered structure in polymer melt, which is beneficial to reduce the conductive percolation, form a conductive network, and prepare a new type of polymer functional microtubules. For example, a flexible tubular electrode is prepared by using the principle of Joule heating, which has wide application prospects in hyperthermia under complex conditions such as trachea and intestinal tract (Figures 2E,F) (Li et al., 2021c).
Electrospinning
Principle of Electrospinning
By applying a charged jet, electrospinning processing can stretch the liquid into jet with small diameter under the action of surface tension, where the strong shearing action of the electric field induces more regular orientation arrangement (Li and Xia, 2004a; Xue et al., 2017). In addition, due to the fine diameter of the filaments, multi-axially oriented network structure can be further formed to prepare functional devices. As illustrated in Figures 3A–C), (Xue et al., 2019), liquid is squeezed out of the spinneret due to surface tension, creating pendant droplets. When energized, electrostatic repulsion deforms the droplet into a Taylor cone, from which a charged jet is ejected, and finally deposit on the grounded collector (Sun et al., 2014; Liao et al., 2018). Notably, various secondary structure can be constructed by tuning the electrospinning parameters (Figure 3D) (Yang et al., 2018). Especially, electrospun fibers with core-shell are produced via a coaxial conical jet under a high-voltage electrostatic field (Jiang et al., 2005). Li et al. (Li and Xia, 2004a) prepared fiber tubes by coaxial electrostatic spinning technology; Chen et al. (Chen et al., 2010) prepared core-shell structure microfiber of nanowires in microtubules by multi-fluid coaxial electrostatic spinning technology. Zhao et al. (Zhao et al., 2007) fabricated multi-channel fiber tubes by combining coaxial electrospinning technology with double-nozzle side-by-side electrospinning technology. This multi-cavity structure facilitates controlled release of drug in practical application (Wang et al., 2010; Cheng et al., 2019).
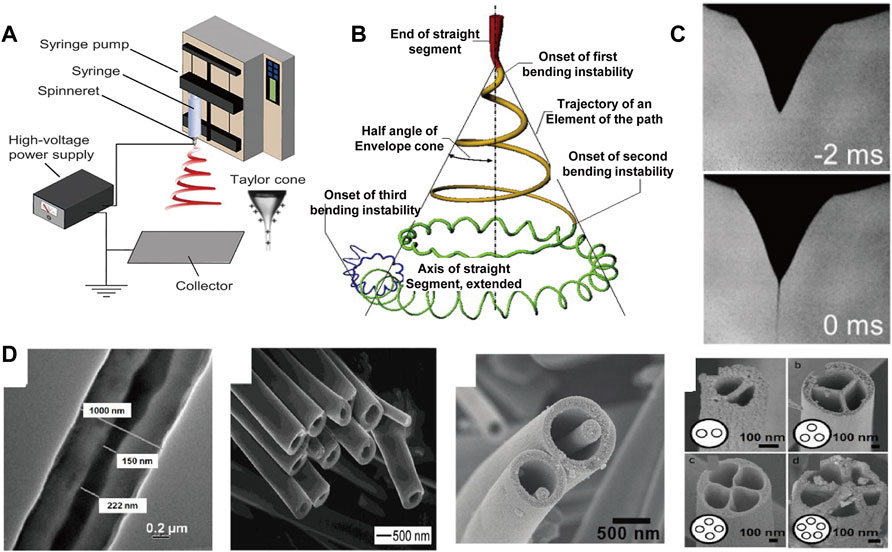
FIGURE 3. (A) Basic setup for electrospinning (Xue et al., 2019) Copyright 2019, American Chemical Society; (B) Schematic diagram of the prototype instantaneous position of the electrospinning jet path (Xue et al., 2019) Copyright 2019, American Chemical Society; (C) Reprinted with permission from ref (Xue et al., 2019) Copyright 2019, American Chemical Society; (D) Structural diversity of the individual electrospinning fiber (Sun et al., 2003; Li and Xia, 2004b; Zhao et al., 2007; Chen et al., 2010) Copyright 2004, American Chemical Society Copyright 2007, American Chemical Society Copyright 2010, American Chemical Society Copyright 2003, Wiley.
Applications of Electrospinning in Bone Repairing and Artificial Blood Vessels
Electrospinning, as a preparation method of nanofibers, has been widely used in drug delivery (Li W. et al., 2015; Yu et al., 2015; Cleeton et al., 2019), and wound dressing (Ghosal et al., 2019; Séon-Lutz et al., 2019; Yue et al., 2021). Additionally, the rapid integration and simple fabrication of shaped fibers also enable electrospinning to be applied in the field of biomedical application such as bone repairing (Cheng et al., 2020; Kandasamy et al., 2020; Ma et al., 2021) and artificial blood vessels (Jang et al., 2020; Zhao et al., 2021).
Although bone grafts can be autografts or allografts, the source of the graft and the strong physical rejection forced researchers to look for more suitable substitutes to mimic the structure and function of bone tissue, while the enriched secondary structure and assembly geometry of electrospinning offer a promising solution (Jones and Hench, 2003). Ibrahim et al. (Yahia et al., 2019) prepared a PCL and chitosan/polyethylene oxide (CS/PEO) composite sandwich nanofiber by electrospinning. The unique sandwich porous three-dimensional structure can effectively promote the deposition of apatite, and the CS on the surface reduces the water contact angle of the material from 116.6 to 57.6, which improves the adhesion of cells. An interesting phenomenon is also observed by Stachewicz (Szewczyk et al., 2019) that by applying negative voltages, the fluorine content on the polyvinylidene fluoride (PVDF) surface is changed and effectively promote the cells on the electrospun fibers to proliferate more, which was favorable for cell adhesion, proliferation and differentiation (Figure 4A). Besides 2D nonwovens, electrospinning can also be used to fabricate 3D devices. Chen (Chen et al., 2016) prepared flexible porous 3D scaffolds by electrospinning, and the scaffolds exhibited excellent bone regeneration and elasticity (Figure 4B). The excellent cytocompatibility deposition ensure its subsequent application of bone repair in rabbits and simultaneously, as a flexible material, it can be applied to various environments and different parts of the body.
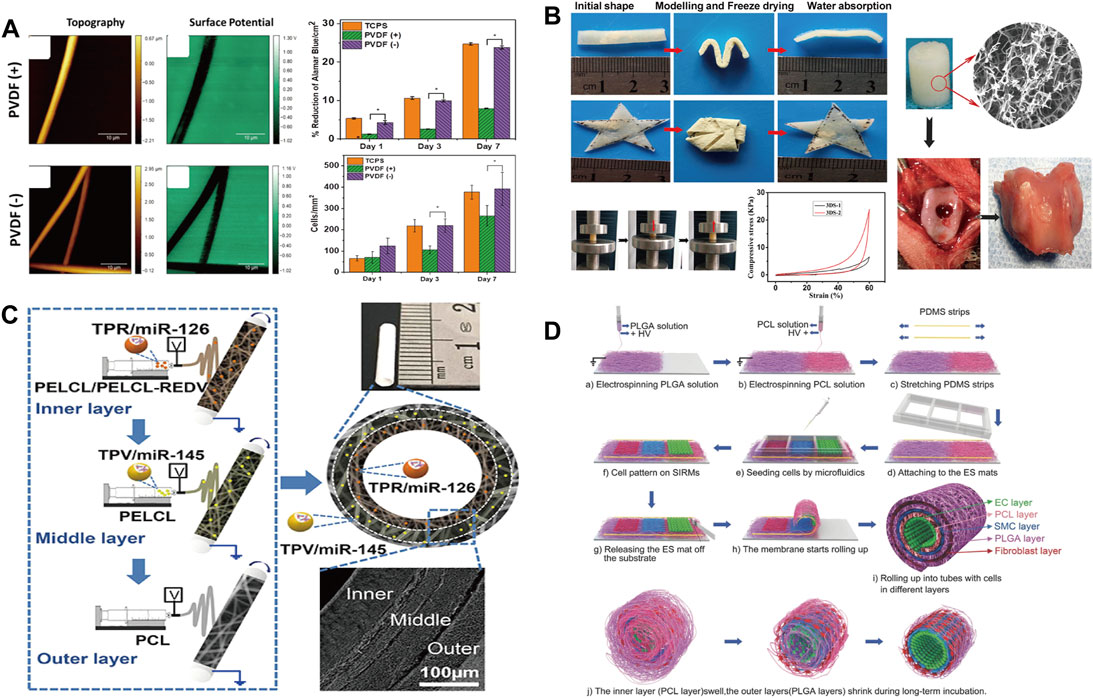
FIGURE 4. (A) Surface morphology and cell proliferation of electrospun PVDF fibers prepared at different voltages (Szewczyk et al., 2018) Copyright 2018, American Chemical Society; (B) Water absorption property. Photographs showing the scaffold shape recovery after water absorption and the mechanical properties and biocompatibility of samples (Chen et al., 2016) Copyright 2016, American Chemical Society; (C) Schematic diagram of multi-layer electrospinning and actual sample diagram (Wen et al., 2020) Copyright 2020, American Chemical Society; (D) Schematic illustration of fabrication of the cell-laden multilayered PCL–PLGA tubes (Cheng et al., 2017) Copyright 2017, Wiley.
Cardiovascular disease, as a global high-mortality disease, has received extensive attention from the academic community (Goins et al., 2019). There is a great demand for artificial blood vessels in clinical operations. The conventional processing technology cannot process small-diameter blood vessels, and due to the existence of thrombosis (Wang et al., 2020b; Wang et al., 2020c) and intimal hyperplasia (Jeong et al., 2020; Park et al., 2020), the artificial blood vessels are difficult to keep unobstructed (Tang et al., 2018). At present, artificial blood vessels are mainly prepared by electrospinning (Murphy and Atala, 2014). Most importantly, the combination of materials and structure derived from electrospinning can endow artificial blood vessel with more tunable properties. Wen et al. (Wen et al., 2020) prepared artificial blood vessels with a three-layer structure by continuously spinning different electrospinning fabrics (Figure 4C). Through the combination of different materials and different cells in the human body, the blood vessels can be unobstructed and exhibits excellent anticoagulation and antiproliferative properties. Cheng et al. (Cheng et al., 2017) employed double nozzles to spin polycaprolactone (PCL) and poly (lactic-co-glycolic acid) (PLGA) fabrics and stack them by winding to form artificial blood vessels (Figure 4D). Due to the expandability of PCL and the shrinkage of PLGA, the rolled composite material perfectly balances the size transformation of PCL and PLGA, and maintains the shape stability inside the artificial blood vessel. Meanwhile, the relatively slow degradation rate of the inner PCL layer were beneficial for the durable mechanical support of the initial structure of the tube, while the faster degradation of the PLGA promoted cell growth and angiogenesis. Although the pore size of electrospun materials often prevents cell infiltration, the control of mass density and geometry as well as fiber orientation opens up new opportunities in the field of artificial blood vessels, leading to better artificial blood vessel grafts in the future (Niklason and Lawson, 2020).
Micro Injection Molding
Principle of Micro Injection Molding
The micro-injection process is currently one of the most important technologies for preparing polymeric micro-device due to low manufacturing cost, high precision, short molding cycle and the ability to prepare complex parts (Figures 5A,B) (Wang, 2019; Piccolo et al., 2021). Although similar with traditional injection molding process, the melt in micro-injection can be described as an ideal case of laminar flow, the shear in the micro confined channel is significantly greater than that of the traditional injection molding process (Jiang et al., 2018). Accordingly, the ultrahigh shearing (107 s−1) can lead to increased degree of orientation and crystallinity by shear-induced crystallization (Cai et al., 2021). For instance, shish-kebab structures are keen to form during micro-injection (Figure 5C) (Han et al., 2021) and thus realize enhancement of physical and chemical properties of the material (Shi et al., 2019). Special crystal modification such as the β crystal of PVDF can also be scalarly fabricated with the assistance of the ultrahigh shear in micro-injection. Nie (Guo et al., 2020) successfully prepared PVDF piezoelectric parts with a relative fraction of β crystals of 52% by micro-injection, and the open-circuit voltage density reached ∼11 V/cm2, with over 4-fold improvement as compared to that fabricated by conventional cast method. Except from crystal, the ultrahigh shear can also promote the mechanism of in-situ fibrillation. It is worth noting that the extremely high shear rate and fast cooling rate of micro-injection can also tailor special interlocking structure which cannot obtained in conventional in-situ fibrillation processing (Cai et al., 2021) (Figure 5D).
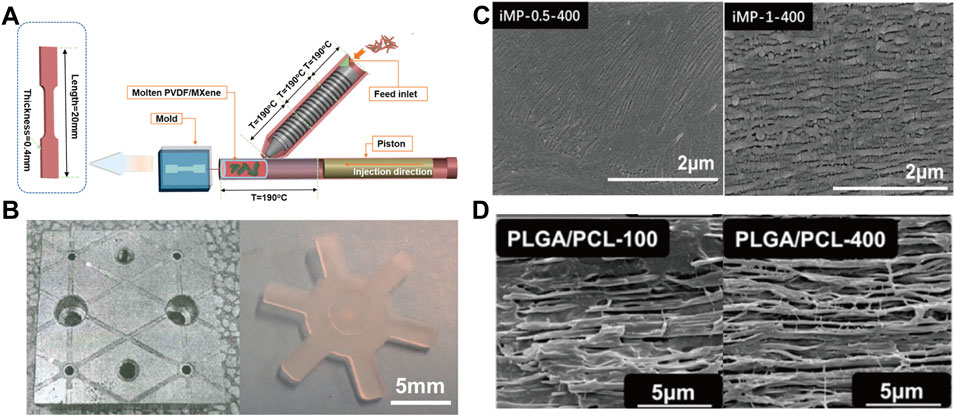
FIGURE 5. (A) Schematic diagram of the micro-injection device (Han et al., 2021) Copyright 2020, American Chemical Society; (B) Micro injection molded parts (Wang et al., 2019) Copyright 2019 Wiley; (C) SEM images of high shear induced crystallization (Han et al., 2021) Copyright 2021, American Chemical Society; (D) SEM images of PLGA/PCL materials prepared by micro-injection at different injection rates (Cai et al., 2021) Copyright 2021, Elsevier.
Applications of Micro Injection Molding in Cell Proliferation and Bone Tissue Engineering
In the field of cell proliferation, the proliferation and differentiation of cells can be effectively regulated by adjusting different processing parameters. The micro structured surfaces will affect the proliferation and differentiation of cells and are increasingly important for biological and clinical applications (Benoit et al., 2008; Yim et al., 2010). The micro-injection process can realize the rapid industrial preparation of microstructures. The super hydrophobic or super hydrophilic design of micro surfaces can be well used in medical devices, especially the local microenvironment (topography and strength) can decisively affect the ability of cells to self-renew and differentiate (Yatsushiro et al., 2016; Shiu et al., 2018). Wang et al. (Wang et al., 2018) fabricated honeycomb-like micro surface arrays with different contact angles by means of micro-injection (Figure 6A). Li et al. (Lee et al., 2019) successfully achieved the preparation of curved parts with sub-micron surface morphology through micro-injection and nano-imprinting (Figure 6B). Zanchetta et al. (Zanchetta et al., 2015) fabricated devices with different microscopic features and studied the proliferation and differentiation of cells under different microscopic features (Figure 6C). Differences in micro surfaces can cause cells to differentiate in different directions while the difference in pore size leads to the difference in proliferation efficiency. The micro injection molding process can prepare large-scale devices for cell proliferation and differentiation (Matschuk et al., 2010). Micro-injection has also shown great potential in the field of bone tissue engineering, to cooperate with new materials and achieve comprehensive biological and mechanical properties (Chen et al., 2017; Yang X. et al., 2021). Cai et al. (Cai et al., 2021) prepared PLGA/PCL fixation plate by in-situ fibrillation in micro-injection process (Figure 6D). The dimensional stability of the material is improved with improved mechanical properties, where the plate maintained its original size after being soaked in phosphate-buffered saline (PBS) for 40 days, laying a foundation for the subsequent preparation of bone repair materials.
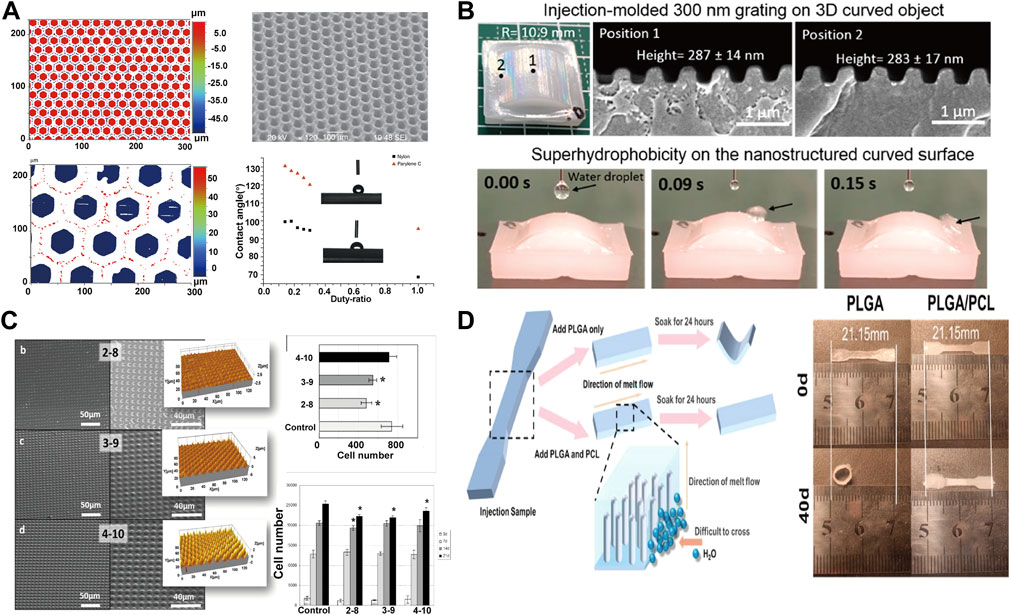
FIGURE 6. (A) Preparation of honeycomb nylon and water contact angle data (Wang et al., 2018) Copyright 2018 Springer Nature; (B) Macroscopic curved submicron superhydrophobic surface structures fabricated using micro injection molding and nanoimprinting (Lee et al., 2019) Copyright 2019, American Chemical Society; (C) SEM images and cell proliferation images of micro-injected samples with different pore sizes (Zanchetta et al., 2015) Copyright 2015, American Chemical Society; (D) Micro injection molding enhances dimensional stability of materials through in-situ fiberization (Cai et al., 2021) Copyright 2021, Elsevier.
Inkjet Printing and Laser Assisted Bioprinting
Principle of Inkjet Printing and Laser Assisted Bioprinting
3D printing allows rapid transformation of computer-aided designs into complex 3D printed parts, enabling rapid on-demand manufacturing (Lee et al., 2017; Wang et al., 2017). Compared with conventional processing, 3D printing technology has better customization and can prepare complex geometry for special purposes, which has great potential in the field of biomedical engineering. Especially, inkjet 3D printing (Murphy and Atala, 2014; Saunders and Derby, 2014; Knowlton et al., 2015; Mandrycky et al., 2016; Lee et al., 2019) and laser assisted bioprinting (Barron et al., 2004; Palla-Papavlu et al., 2011; Dou et al., 2021) exhibits rapid development and inspiring potential, which deserved an opportune review and attention.
Inkjet printing is realized by selectively depositing droplets of printing material to form target entities (Mei et al., 2005; Zhou et al., 2020). The low-viscosity fluid namely the ink is ejected through the nozzle and moves with the platform to accurately deposit on the printing platform to solidify (Figure 7A). Printing inks suitable for biomedical engineering should have biocompatibility, biological function, printability and structural stability, while hydrogels (Boland et al., 2007; Nakamura et al., 2010; Kyle et al., 2017) featuring multifunctionality are similar to extracellular matrix and biological tissues, making it become an ideal candidate for bio ink and realizing the gel 3D printing (Zhang et al., 2020). Although Gel 3D printing is widely used in the fabrication of complex devices, the specific fluid properties and curable nature of droplets also hinder the development of inkjet printing (Zhang B. et al., 2018). The functionality of printed parts depends primarily on the inherent bioactivity of the hydrogel and the retention of contained cells and growth factors (Unal and West, 2020; Advincula et al., 2021). However, the contradiction between printability and structural stability of most hydrogels is not conducive to obtaining high printing accuracy, self-support, and structural fidelity, which is an urgent problem to be solved in the field of bio-3D printing.
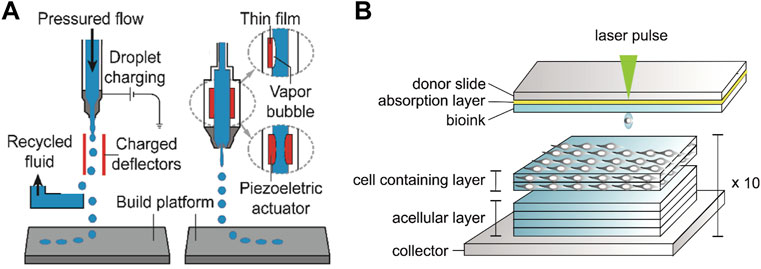
FIGURE 7. (A) Schematic diagram of the inkjet 3D printing device (Zhou et al., 2020) Copyright 2020, Wiley; (B) Schematic diagram of the laser-assisted bioprinting system and printing of the 3D stromal mimicking structures (Sorkio et al., 2018) Copyright 2018, Elsevier.
The laser assisted bioprinting (LAB) printer consisting of a laser generator, a laser path adjustment module and a cell transfer module can focus laser pulse to the laser absorbing layer in the cell transfer module and thus generate air bubbles to push the bioink (usually the sol embedded in cells) downward to print patterns with high cell viability (Figure 7B) (Duocastella et al., 2009; Serra et al., 2009; Duocastella et al., 2010; Guillemot et al., 2010; Unger et al., 2011; Sorkio et al., 2018). Compared with traditional printing methods, LAB achieves higher printing accuracy and resolution through laser deposition (Guillotin et al., 2010; Cheptsov et al., 2019). The emergence of LAB enables 3D printing of structural materials with high cell density (Ringeisen et al., 2010; Kérourédan et al., 2019), and further guarantees the combination with other printing technologies to realize the preparation of various human tissues and organs. It is worth noting that the productivity and efficiency of LAB is not competitive with other printing methods due to the small amount of transferable biological material in each laser pulse, which limits the development of LAB printing technology.
Applications of Inkjet Printing and Laser Assisted Bioprinting in Tissue Engineering
The ability of 3D printing technology to prepare complex structures is widely used in the field of biomedical engineering, (Gao et al., 2018), because 3D printing can effectively modulates the morphological structure of parts (Ma et al., 2018; Wang et al., 2021). For instance, the ability of cells to proliferate and differentiate depends on the morphological structure of the component itself. Ajdary (Ajdary et al., 2019) prepared nanocellulose structural parts with different porosity by direct ink writing (DIW) printing. The porous structure and interconnected network structure increase the permeability of cells in the structure, which facilitates the transport of nutrients and the discharge of metabolic wastes, and leads to an increase in the ability of cells to proliferate. Li et al. (Li T. et al., 2019) also controlled cell proliferation by preparing hollow tubes with different inner diameters by DIW (Figure 8A), where the high specific surface area and surface adhesion of hollow tubes resulted in enhanced cell proliferation and promoted expression of osteogenic genes in cells. Moreover, the 3D printing can endow medical device with shape memory effect which offer new methods in clinical application. In addition, the use of biomolecules for inkjet printing can further modulate cell behavior, with the hope that it can mimic natural tissue and organ growth (Figure 8B) (Li et al., 2020). Nowadays, this method has been widely used in bone and tissue engineering.
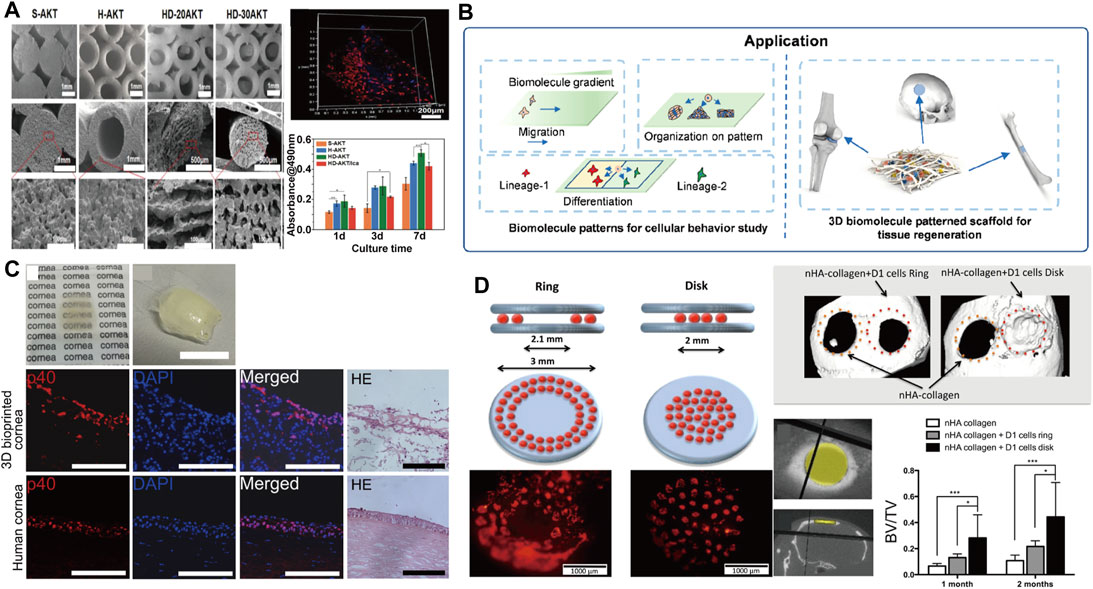
FIGURE 8. (A) SEM topography and cell proliferation data of 3D printed fibers with different pore sizes (Li Y. et al., 2019) Copyright 2019, Wiley; (B) With the printing, biomolecule patterns were applied in cellular behavior study and tissue regeneration (Li et al., 2020) Copyright 2020 American Chemical Society; (C) 3D cornea from hESC-LESCs and Human adipose-derived mesenchymal stem cells (hASCs) fabricated using laser-assisted bioprinting (Sorkio et al., 2018) Copyright 2018, Elsevier; (D) Schematic representation of the in vivo laser assisted bioprinting geometries tested and the experimental diagram of mice calvaria restoration (Keriquel et al., 2017) Copyright 2017 Springer Nature.
Compared with traditional 3D printing technology, LAB technology not only increases the proportion of biological tissues, but also avoids the cell death during processing, setting off a new storm in biomedical engineering. Dias et al. (Dias et al., 2014) studied LAB printing of embryonic stem cells, by which embryoid bodies can be formed. In addition to the development of LAB in the printability of stem cells, LAB has also made achievements in the fields of tissue engineering and bone repair. Sorkio et al. (Sorkio et al., 2018) successfully prepared clinically applicable corneal structures by combining human embryonic stem cell-derived limbal epithelial cells (hESC-LESCs) with collagen I for LAB printing (Figure 8C). Keriquel (Keriquel et al., 2017) induced bone repair by depositing hydroxyapatite particles into critical-sized calvaria defects in mice (Figure 8D). All these works expand the application of LAB technology, and provide more options for clinical surgery to avoid secondary surgery. It is worth noting that the high cost and relatively small geometry figure limit the development of LAB technology and at the same time, the parameters of LAB printing technology are not fully clarified. Consequently LAB is still relatively immature for the fabrication of 3D tissue structures, which also provides a new direction for 3D printing.
Summaries and Perspective
Advanced machining technologies continue to evolve with their unique functionality and manufacturing speeds. Although different processing methods can obtain the same medical device, due to the different force field and temperature field during the processing, its morphological and structural properties also have advantages and disadvantages. This review summarizes the application of advanced processing technology in new medical materials and new devices in a timely manner (Table 1). It is not difficult to imagine that there will be more breakthroughs in advanced processing technology on this basis, but there are still some challenges.
There are also issues to be addressed in the future development of advanced polymer processing. For equipment, iterative updates of processing equipment are required. Advanced processing equipment must be compatible with scale and integration in order to amplify its advantages. This places stringent requirements on manufacturing equipment, which must be highly intelligent and suitable for continuous production. In terms of materials, large-scale preparation technology of materials suitable for advanced processing technology is also highly urgent. The range of materials available for processing technologies such as electrospinning or 3D printing is relatively narrow, because of demanded processing parameters. Therefore, how to expand the material universality of these processing technologies has also become a current research hotspot.
Through advanced processing technology, functional medical materials and devices endow modern medicine with unimaginable convenience. Especially with the development of information technology, it also endows these technologies with the possibility of digital manufacturing and great potential with digital twin technology. These emerging processing technologies will greatly expand the feasibility and diversity of medical technologies, thereby revolutionizing the traditional medical industry.
Author Contributions
TZ: Writing—original draft, Data curation. MN: Investigation, Validation. YL: Writing—review and editing, Supervision, Funding acquisition.
Funding
This work was financially supported by the National Natural Science Foundation of China (No. 52003174 and 51933007), the State Key Laboratory of Polymer Materials Engineering (sklpme 2020-3-05), and the Fundamental Research Funds for the Central Universities (2020SCU12006). Reproduced under the terms of the CC-BY Creative Commons Attribution 4.0 International license (https://creativecommons.org/licenses/by/4.0) (Liu et al., 2014) Copyright 2014, W. Liu, published by Royal Society of Chemistry.
Conflict of Interest
The authors declare that the research was conducted in the absence of any commercial or financial relationships that could be construed as a potential conflict of interest.
Publisher’s Note
All claims expressed in this article are solely those of the authors and do not necessarily represent those of their affiliated organizations, or those of the publisher, the editors and the reviewers. Any product that may be evaluated in this article, or claim that may be made by its manufacturer, is not guaranteed or endorsed by the publisher.
References
Abdolmaleki, H., Kidmose, P., and Agarwala, S. (2021). Droplet‐Based Techniques for Printing of Functional Inks for Flexible Physical Sensors. Adv. Mat. 33, 2006792. doi:10.1002/adma.202006792
Advincula, R. C., Dizon, J. R. C., Caldona, E. B., Viers, R. A., Siacor, F. D. C., Maalihan, R. D., et al. (2021). On the Progress of 3D-Printed Hydrogels for Tissue Engineering. Mrs Commun. 11, 539–553. doi:10.1557/s43579-021-00069-1
Ajdary, R., Huan, S., Zanjanizadeh Ezazi, N., Xiang, W., Grande, R., Santos, H. A., et al. (2019). Acetylated Nanocellulose for Single-Component Bioinks and Cell Proliferation on 3D-Printed Scaffolds. Biomacromolecules 20, 2770–2778. doi:10.1021/acs.biomac.9b00527
Alotaibi, A. M., Williamson, B. A. D., Sathasivam, S., Kafizas, A., Alqahtani, M., Sotelo-Vazquez, C., et al. (2020). Enhanced Photocatalytic and Antibacterial Ability of Cu-Doped Anatase TiO2 Thin Films: Theory and Experiment. ACS Appl. Mat. Interfaces 12, 15348–15361. doi:10.1021/acsami.9b22056
Barron, J. A., Spargo, B. J., and Ringeisen, B. R. (2004). Biological Laser Printing of Three Dimensional Cellular Structures. Appl. Phys. A 79, 1027–1030. doi:10.1007/s00339-004-2620-3
Benoit, D. S. W., Schwartz, M. P., Durney, A. R., and Anseth, K. S. (2008). Small Functional Groups for Controlled Differentiation of Hydrogel-Encapsulated Human Mesenchymal Stem Cells. Nat. Mater 7, 816–823. doi:10.1038/nmat2269
Boland, T., Tao, X., Damon, B. J., Manley, B., Kesari, P., Jalota, S., et al. (2007). Drop-on-demand Printing of Cells and Materials for Designer Tissue Constructs. Mater. Sci. Eng. C 27, 372–376. doi:10.1016/j.msec.2006.05.047
Cai, H., Zhang, T., Dai, R., Song, S., Han, R., Li, Y., et al. (2021). Effect of Poly(ε-Caprolactone) Microfibers in Poly(lactide-Co-Glycolide) Based Bone Fixation Plate on Preventing Dimensional Shrinkage and Promoting Cell Interactions. Compos. Sci. Technol. 216, 109051. doi:10.1016/j.compscitech.2021.109051
Chen, H., Wang, N., Di, J., Zhao, Y., Song, Y., and Jiang, L. (2010). Nanowire-in-microtube Structured Core/shell Fibers via Multifluidic Coaxial Electrospinning. Langmuir 26, 11291–11296. doi:10.1021/la100611f
Chen, J., Liu, X., Tian, Y., Zhu, W., Yan, C., Shi, Y., et al. (2022). 3D‐Printed Anisotropic Polymer Materials for Functional Applications. Adv. Mater. 34, 2102877. doi:10.1002/adma.202102877
Chen, J., Zhang, X., Huang, C., Cai, H., Hu, S., Wan, Q., et al. (2017). Osteogenic Activity and Antibacterial Effect of Porous Titanium Modified with Metal-Organic Framework Films. J. Biomed. Mat. Res. 105, 834–846. doi:10.1002/jbm.a.35960
Chen, W., Chen, S., Morsi, Y., El-Hamshary, H., El-Newhy, M., Fan, C., et al. (2016). Superabsorbent 3D Scaffold Based on Electrospun Nanofibers for Cartilage Tissue Engineering. ACS Appl. Mat. Interfaces 8, 24415–24425. doi:10.1021/acsami.6b06825
Cheng, G., Yin, C., Tu, H., Jiang, S., Wang, Q., Zhou, X., et al. (2019). Controlled Co-delivery of Growth Factors through Layer-By-Layer Assembly of Core-Shell Nanofibers for Improving Bone Regeneration. ACS Nano 13, 6372–6382. doi:10.1021/acsnano.8b06032
Cheng, L., Chen, Z., Cai, Z., Zhao, J., Lu, M., Liang, J., et al. (2020). Bioinspired Functional Black Phosphorus Electrospun Fibers Achieving Recruitment and Biomineralization for Staged Bone Regeneration. Small 16, 2005433. doi:10.1002/smll.202005433
Cheng, S., Jin, Y., Wang, N., Cao, F., Zhang, W., Bai, W., et al. (2017). Self-Adjusting, Polymeric Multilayered Roll that Can Keep the Shapes of the Blood Vessel Scaffolds during Biodegradation. Adv. Mat. 29, 1700171. doi:10.1002/adma.201700171
Cheptsov, V. S., Tsypina, S. I., Minaev, N. V., Yusupov, V. I., and Chichkov, B. N. (2019). New Microorganism Isolation Techniques with Emphasis on Laser Printing. Int. J. Bioprint 5, 165. doi:10.18063/ijb.v5i1.165
Cleeton, C., Keirouz, A., Chen, X., and Radacsi, N. (2019). Electrospun Nanofibers for Drug Delivery and Biosensing. ACS Biomater. Sci. Eng. 5, 4183–4205. doi:10.1021/acsbiomaterials.9b00853
Dias, A. D., Unser, A. M., Xie, Y., Chrisey, D. B., and Corr, D. T. (2014). Generating Size-Controlled Embryoid Bodies Using Laser Direct-Write. Biofabrication 6, 025007. doi:10.1088/1758-5082/6/2/025007
Dou, C., Perez, V., Qu, J., Tsin, A., Xu, B., and Li, J. (2021). A State‐of‐the‐Art Review of Laser‐Assisted Bioprinting and its Future Research Trends. Cben 8, 517–534. doi:10.1002/cben.202000037
Duocastella, M., Fernández-Pradas, J. M., Morenza, J. L., and Serra, P. (2009). Time-resolved Imaging of the Laser Forward Transfer of Liquids. J. Appl. Phys. 106, 084907. doi:10.1063/1.3248304
Duocastella, M., Fernández-Pradas, J. M., Serra, J. L. M. P., and Serra, P. (2010). Sessile Droplet Formation in the Laser-Induced Forward Transfer of Liquids: A Time-Resolved Imaging Study. Thin Solid Films 518, 5321–5325. doi:10.1016/j.tsf.2010.03.082
El-Ghazali, S., Khatri, M., Hussain, N., Khatri, Z., Yamamoto, T., Kim, S. H., et al. (2021). Characterization and Biocompatibility Evaluation of Artificial Blood Vessels Prepared from Pristine Poly (Ethylene-Glycol-Co-1,4-Cyclohexane Dimethylene-Co-Isosorbide Terephthalate), Poly (1, 4 Cyclohexane Di-methylene-co-isosorbide Terephthalate) Nanofibers and Their Blended Composition. Mater. Today Commun. 26, 102113. doi:10.1016/j.mtcomm.2021.102113
Gao, F., Xu, Z., Liang, Q., Liu, B., Li, H., Wu, Y., et al. (2018). Direct 3D Printing of High Strength Biohybrid Gradient Hydrogel Scaffolds for Efficient Repair of Osteochondral Defect. Adv. Funct. Mat. 28, 1706644. doi:10.1002/adfm.201706644
Geyer, F., D'acunzi, M., Yang, C.-Y., Müller, M., Baumli, P., Kaltbeitzel, A., et al. (2019). How to Coat the inside of Narrow and Long Tubes with a Super-liquid-repellent Layer-A Promising Candidate for Antibacterial Catheters. Adv. Mat. 31, 1801324. doi:10.1002/adma.201801324
Ghosal, K., Agatemor, C., Špitálsky, Z., Thomas, S., and Kny, E. (2019). Electrospinning Tissue Engineering and Wound Dressing Scaffolds from Polymer-Titanium Dioxide Nanocomposites. Chem. Eng. J. 358, 1262–1278. doi:10.1016/j.cej.2018.10.117
Goins, A., Webb, A. R., and Allen, J. B. (2019). Multi-layer Approaches to Scaffold-Based Small Diameter Vessel Engineering: A Review. Mater. Sci. Eng. C 97, 896–912. doi:10.1016/j.msec.2018.12.067
Guillemot, F., Souquet, A., Catros, S., and Guillotin, B. (2010). Laser-assisted Cell Printing: Principle, Physical Parameters versus Cell Fate and Perspectives in Tissue Engineering. Nanomedicine 5, 507–515. doi:10.2217/nnm.10.14
Guillotin, B., Souquet, A., Catros, S., Duocastella, M., Pippenger, B., Bellance, S., et al. (2010). Laser Assisted Bioprinting of Engineered Tissue with High Cell Density and Microscale Organization. Biomaterials 31, 7250–7256. doi:10.1016/j.biomaterials.2010.05.055
Guo, J., Nie, M., and Wang, Q. (2020). Self-Poling Polyvinylidene Fluoride-Based Piezoelectric Energy Harvester Featuring Highly Oriented β-Phase Structured at Multiple Scales. ACS Sustain. Chem. Eng. 9, 499–509. doi:10.1021/acssuschemeng.0c07802
Guo, Z., Poot, A. A., and Grijpma, D. W. (2021). Advanced Polymer-Based Composites and Structures for Biomedical Applications. Eur. Polym. J. 149, 110388. doi:10.1016/j.eurpolymj.2021.110388
Han, R., Zheng, L., Li, G., Chen, G., Ma, S., Cai, S., et al. (2021). Self-Poled Poly(vinylidene fluoride)/MXene Piezoelectric Energy Harvester with Boosted Power Generation Ability and the Roles of Crystalline Orientation and Polarized Interfaces. ACS Appl. Mat. Interfaces 13, 46738–46748. doi:10.1021/acsami.1c14007
Ho, M.-P., Wang, H., Lee, J.-H., Ho, C.-K., Lau, K.-T., Leng, J., et al. (2012). Critical Factors on Manufacturing Processes of Natural Fibre Composites. Compos. Part B Eng. 43, 3549–3562. doi:10.1016/j.compositesb.2011.10.001
Hu, X., Chen, A., Luo, Y., Zhang, C., and Zhang, E. (2018). Steerable Catheters for Minimally Invasive Surgery: a Review and Future Directions. Comput. Assist. Surg. 23, 21–41. doi:10.1080/24699322.2018.1526972
Hwang, J., Jeon, S., Kim, B., Kim, J. y., Jin, C., Yeon, A., et al. (2022). An Electromagnetically Controllable Microrobotic Interventional System for Targeted, Real‐Time Cardiovascular Intervention. Adv. Healthc. Mater., 2102529. doi:10.1002/adhm.202102529
Jang, S. R., Kim, J. I., Park, C. H., and Kim, C. S. (2020). Development of Y-Shaped Small Diameter Artificial Blood Vessel with Controlled Topography via a Modified Electrospinning Method. Mater. Lett. 264, 127113. doi:10.1016/j.matlet.2019.127113
Jeong, Y., Yao, Y., and Yim, E. K. F. (2020). Current Understanding of Intimal Hyperplasia and Effect of Compliance in Synthetic Small Diameter Vascular Grafts. Biomater. Sci. 8, 4383–4395. doi:10.1039/d0bm00226g
Jiang, H., Hu, Y., Li, Y., Zhao, P., Zhu, K., and Chen, W. (2005). A Facile Technique to Prepare Biodegradable Coaxial Electrospun Nanofibers for Controlled Release of Bioactive Agents. J. Control. Release 108, 237–243. doi:10.1016/j.jconrel.2005.08.006
Jiang, J., Wang, S., Hou, J., Zhang, K., Wang, X., Li, Q., et al. (2018). Effect of Injection Velocity on the Structure and Mechanical Properties of Micro Injection Molded Polycarbonate/poly(ethylene Terephthalate) Blends. Mater. Des. 141, 132–141. doi:10.1016/j.matdes.2017.12.024
Jones, J. R., and Hench, L. L. (2003). Regeneration of Trabecular Bone Using Porous Ceramics. Curr. Opin. Solid State Mater. Sci. 7, 301–307. doi:10.1016/j.cossms.2003.09.012
Jung, Y. H., Kim, J. U., Lee, J. S., Shin, J. H., Jung, W., Ok, J., et al. (2020). Injectable Biomedical Devices for Sensing and Stimulating Internal Body Organs. Adv. Mat. 32, 1907478. doi:10.1002/adma.201907478
Kandasamy, S., Narayanan, V., and Sumathi, S. (2020). Zinc and Manganese Substituted hydroxyapatite/CMC/PVP Electrospun Composite for Bone Repair Applications. Int. J. Biol. Macromol. 145, 1018–1030. doi:10.1016/j.ijbiomac.2019.09.193
Keriquel, V., Oliveira, H., Rémy, M., Ziane, S., Delmond, S., Rousseau, B., et al. (2017). In Situ Printing of Mesenchymal Stromal Cells, by Laser-Assisted Bioprinting, for In Vivo Bone Regeneration Applications. Sci. Rep. 7, 1778. doi:10.1038/s41598-017-01914-x
Kérourédan, O., Bourget, J.-M., Rémy, M., Crauste-Manciet, S., Kalisky, J., Catros, S., et al. (2019). Micropatterning of Endothelial Cells to Create a Capillary-like Network with Defined Architecture by Laser-Assisted Bioprinting. J. Mater. Sci. Mater. Med. 30, 1–12.
Kim, S. Y., Han, G., Hwang, D. B., Won, D. H., Shin, Y. S., Kim, C., et al. (2021). Design and Usability Evaluations of a 3D‐Printed Implantable Drug Delivery Device for Acute Liver Failure in Preclinical Settings. Adv. Healthc. Mat. 10, 2100497. doi:10.1002/adhm.202100497
Knowlton, S., Onal, S., Yu, C. H., Zhao, J. J., and Tasoglu, S. (2015). Bioprinting for Cancer Research. Trends Biotechnol. 33, 504–513. doi:10.1016/j.tibtech.2015.06.007
Kyle, S., Jessop, Z. M., Al-Sabah, A., and Whitaker, I. S. (2017). 'Printability' of Candidate Biomaterials for Extrusion Based 3D Printing: State-Of-The-Art. Adv. Healthc. Mat. 6, 1700264. doi:10.1002/adhm.201700264
Lee, J.-Y., An, J., and Chua, C. K. (2017). Fundamentals and Applications of 3D Printing for Novel Materials. Appl. Mater. Today 7, 120–133. doi:10.1016/j.apmt.2017.02.004
Lee, W. L., Wang, D., Wu, J., Ge, Q., and Low, H. Y. (2019). Injection Molding of Superhydrophobic Submicrometer Surface Topography on Macroscopically Curved Objects: Experimental and Simulation Studies. ACS Appl. Polym. Mat. 1, 1547–1558. doi:10.1021/acsapm.9b00312
Li, D., and Xia, Y. (2004a). Direct Fabrication of Composite and Ceramic Hollow Nanofibers by Electrospinning. Nano Lett. 4, 933–938. doi:10.1021/nl049590f
Li, D., and Xia, Y. (2004b). Electrospinning of Nanofibers Reinventing the Wheel? Adv. Mater. 16, 1151–1170. doi:10.1002/adma.200400719
Li, T., Zhai, D., Ma, B., Xue, J., Zhao, P., Chang, J., et al. (2019a). 3D Printing of Hot Dog‐Like Biomaterials with Hierarchical Architecture and Distinct Bioactivity. Adv. Sci. 6, 1901146. doi:10.1002/advs.201901146
Li, W., Luo, T., Yang, Y., Tan, X., and Liu, L. (2015a). Formation of Controllable Hydrophilic/hydrophobic Drug Delivery Systems by Electrospinning of Vesicles. Langmuir 31, 5141–5146. doi:10.1021/la504796v
Li, X., Liu, B., Pei, B., Chen, J., Zhou, D., Peng, J., et al. (2020). Inkjet Bioprinting of Biomaterials. Chem. Rev. 120, 10793–10833. doi:10.1021/acs.chemrev.0c00008
Li, X., Pi, L., Nie, M., and Wang, Q. (2015b). Joint Effects of Rotational Extrusion and TiO2 on Performance and Antimicrobial Properties of Extruded Polypropylene Copolymer Pipes. J. Appl. Polym. Sci. 132, 42410. doi:10.1002/app.42410
Li, Y., Nie, M., Pi, L., Bai, S., and Wang, Q. (2021a). A Novel Rotation-Extrusion Rheometer. Acta Polym. Sin. 52, 1547–1558. doi:10.11777/j.issn1000-3304.2021.21075
Li, Y., Zhang, K., Geng, Q., Nie, M., Wang, Q., Huang, Z., et al. (2021b). Helically Intersected Conductive Network Design for Wearable Electronic Devices: From Theory to Application. ACS Appl. Mat. Interfaces 13, 11480–11488. doi:10.1021/acsami.0c22086
Li, Y., Zhang, K., Nie, M., and Wang, Q. (2019b). Tubular Sensor with Multi-Axial Strain Sensibility and Heating Capability Based on Bio-Mimic Helical Networks. Ind. Eng. Chem. Res. 58, 22273–22282. doi:10.1021/acs.iecr.9b04783
Li, Y., Zhang, K., Zhang, L., Nie, M., and Wang, Q. (2021c). Highly Conductive and Durable Electrothermal Electrode through the Steric Confinement Effect of Graphene on Helically Intersected Carbon Fiber Network. Compos. Sci. Technol. 213, 108900. doi:10.1016/j.compscitech.2021.108900
Liao, Y., Loh, C.-H., Tian, M., Wang, R., and Fane, A. G. (2018). Progress in Electrospun Polymeric Nanofibrous Membranes for Water Treatment: Fabrication, Modification and Applications. Prog. Polym. Sci. 77, 69–94. doi:10.1016/j.progpolymsci.2017.10.003
Liu, W., Nie, M., and Wang, Q. (2015). Biaxial Reinforcements for Polybutene-1 Medical-Tubes Achieved via Flow-Design Controlled Morphological Development of Incorporated Polystyrene: In-Situ Microfibrillation, Alignment Manipulation and Performance Optimization. Compos. Sci. Technol. 119, 124–130. doi:10.1016/j.compscitech.2015.10.007
Liu, W., Nie, M., and Wang, Q. (2014). Polybutene-1 Tube with In Situ Microfibering Polystyrene via Helical Convergent Flow: an Economical Pathway to Continuously Fabricate Biaxially Reinforced Polyolefin Tubes for Medical Application. RSC Adv. 4, 47793–47796. doi:10.1039/c4ra07193j
Liu, Y., Zou, Y., Wang, J., Wang, S., and Liu, X. (2021). A Novel Cationic Waterborne Polyurethane Coating Modified by Chitosan Biguanide Hydrochloride with Application Potential in Medical Catheters. J. Appl. Polym. Sci. 138, 50290. doi:10.1002/app.50290
Lu, J., Qiang, Y., Wu, W., and Jiang, B. (2020). Experimental Study on Viscosity Properties of Cyclic Olefin Copolymer (COC) Flowing through Micro Capillary Dies. Polym. Test. 89, 106635. doi:10.1016/j.polymertesting.2020.106635
Ma, H., Feng, C., Chang, J., and Wu, C. (2018). 3D-printed Bioceramic Scaffolds: From Bone Tissue Engineering to Tumor Therapy. Acta biomater. 79, 37–59. doi:10.1016/j.actbio.2018.08.026
Ma, L., Yu, Y., Liu, H., Sun, W., Lin, Z., Liu, C., et al. (2021). Berberine-releasing Electrospun Scaffold Induces Osteogenic Differentiation of DPSCs and Accelerates Bone Repair. Sci. Rep. 11, 1–12. doi:10.1038/s41598-020-79734-9
Mandrycky, C., Wang, Z., Kim, K., and Kim, D.-H. (2016). 3D Bioprinting for Engineering Complex Tissues. Biotechnol. Adv. 34, 422–434. doi:10.1016/j.biotechadv.2015.12.011
Matschuk, M., Bruus, H., and Larsen, N. B. (2010). Nanostructures for All-Polymer Microfluidic Systems. Microelectron. Eng. 87, 1379–1382. doi:10.1016/j.mee.2009.11.167
Mei, J., Lovell, M. R., and Mickle, M. H. (2005). Formulation and Processing of Novel Conductive Solution Inks in Continuous Inkjet Printing of 3-D Electric Circuits. IEEE Trans. Electron. Packag. Manuf. 28. doi:10.1109/tepm.2005.852542
Motealleh, A., Kart, D., Czieborowski, M., and Kehr, N. S. (2021). Functional Nanomaterials and 3D-Printable Nanocomposite Hydrogels for Enhanced Cell Proliferation and for the Reduction of Bacterial Biofilm Formation. ACS Appl. Mat. Interfaces 13, 43755–43768. doi:10.1021/acsami.1c13392
Murphy, S. V., and Atala, A. (2014). 3D Bioprinting of Tissues and Organs. Nat. Biotechnol. 32, 773–785. doi:10.1038/nbt.2958
Nakamura, M., Iwanaga, S., Henmi, C., Arai, K., and Nishiyama, Y. (2010). Biomatrices and Biomaterials for Future Developments of Bioprinting and Biofabrication. Biofabrication 2, 014110. doi:10.1088/1758-5082/2/1/014110
Neoh, K. G., Li, M., Kang, E.-T., Chiong, E., and Tambyah, P. A. (2017). Surface Modification Strategies for Combating Catheter-Related Complications: Recent Advances and Challenges. J. Mat. Chem. B 5, 2045–2067. doi:10.1039/c6tb03280j
Nie, M. W. Q., and Wang, Q. (2013). Control of Rotation Extrusion over Shish-Kebab Crystal Alignment in Polyethylene Pipe and its Effect on the Pipe's Crack Resistance. J. Appl. Polym. Sci. 128, 3149–3155. doi:10.1002/app.38512
Niklason, L. E., and Lawson, J. H. (2020). Bioengineered Human Blood Vessels. Science 370. doi:10.1126/science.aaw8682
Palla-Papavlu, A., Paraico, I., Shaw-Stewart, J., Dinca, V., Savopol, T., Kovacs, E., et al. (2011). Liposome Micropatterning Based on Laser-Induced Forward Transfer. Appl. Phys. A 102, 651–659. doi:10.1007/s00339-010-6114-1
Park, C., Park, S., Kim, J., Han, A., Ahn, S., Min, S.-K., et al. (2020). Enhanced Endothelial Cell Activity Induced by Incorporation of Nano-Thick Tantalum Layer in Artificial Vascular Grafts. Appl. Surf. Sci. 508, 144801. doi:10.1016/j.apsusc.2019.144801
Pi, L., Nie, M., and Wang, Q. (2019). Crystalline Composition and Morphology in Isotactic Polypropylene Pipe under Combining Effects of Rotation Extrusion and Fibril β-nucleating Agent. J. Vinyl Addit. Technol. 25, E195–E202. doi:10.1002/vnl.21686
Pi, L., Shi, Y., Nie, M., and Li, Y. (2020). Polyethylene/TiO 2 Medical Tube with Comprehensive Mechanical Performances via Bio‐Mimic Multiscale Helical Structures. J. Vinyl Addit. Technol. 26, 405–412. doi:10.1002/vnl.21755
Piccolo, L., Puleo, K., Sorgato, M., Lucchetta, G., and Masato, D. (2021). Modeling the Replication of Submicron-Structured Surfaces by Micro Injection Molding. Mater. Des. 198, 109272. doi:10.1016/j.matdes.2020.109272
Ringeisen, B. R., Lizewski, S. E., Fitzgerald, L. A., Biffinger, J. C., Knight, C. L., Crookes-Goodson, W. J., et al. (2010). Single Cell Isolation of Bacteria from Microbial Fuel Cells and Potomac River Sediment. Electroanalysis 22, 875–882. doi:10.1002/elan.200880012
Saunders, R. E., and Derby, B. (2014). Inkjet Printing Biomaterials for Tissue Engineering: Bioprinting. Int. Mater. Rev. 59, 430–448. doi:10.1179/1743280414y.0000000040
Séon-Lutz, M., Couffin, A. C., Vignoud, S., Schlatter, G., and Hébraud, A. (2019). Electrospinning in Water and In Situ Crosslinking of Hyaluronic Acid/Cyclodextrin Nanofibers: Towards Wound Dressing with Controlled Drug Release. Carbohydr. Polym. 207, 276–287. doi:10.1016/j.carbpol.2018.11.085
Serra, P., Duocastella, M., Fernández-Pradas, J. M., and Morenza, J. L. (2009). Liquids Microprinting through Laser-Induced Forward Transfer. Appl. Surf. Sci. 255, 5342–5345. doi:10.1016/j.apsusc.2008.07.200
Shi, S., Wang, L., Pan, Y., Liu, C., Liu, X., Li, Y., et al. (2019). Remarkably Strengthened Microinjection Molded Linear Low-Density Polyethylene (LLDPE) via Multi-Walled Carbon Nanotubes Derived Nanohybrid Shish-Kebab Structure. Compos. Part B Eng. 167, 362–369. doi:10.1016/j.compositesb.2019.03.007
Shiu, J.-Y., Aires, L., Lin, Z., and Vogel, V. (2018). Nanopillar Force Measurements Reveal Actin-Cap-Mediated YAP Mechanotransduction. Nat. Cell Biol. 20, 262–271. doi:10.1038/s41556-017-0030-y
Sorkio, A., Koch, L., Koivusalo, L., Deiwick, A., Miettinen, S., Chichkov, B., et al. (2018). Human Stem Cell Based Corneal Tissue Mimicking Structures Using Laser-Assisted 3D Bioprinting and Functional Bioinks. Biomaterials 171, 57–71. doi:10.1016/j.biomaterials.2018.04.034
Sun, B., Long, Y. Z., Zhang, H. D., Li, M. M., Duvail, J. L., Jiang, X. Y., et al. (2014). Advances in Three-Dimensional Nanofibrous Macrostructures via Electrospinning. Prog. Polym. Sci. 39, 862–890. doi:10.1016/j.progpolymsci.2013.06.002
Sun, F., Guo, J., Li, Y., Bai, S., and Wang, Q. (2019). Preparation of High-Performance Polyethylene Tubes under the Coexistence of Silicone Cross-Linked Polyethylene and Rotation Extrusion. R. Soc. open Sci. 6, 182095. doi:10.1098/rsos.182095
Sun, Z., Zussman, E., Yarin, A. L., Wendorff, J. H., and Greiner, A. (2003). Compound Core-Shell Polymer Nanofibers by Co-electrospinning. Adv. Mat. 15, 1929–1932. doi:10.1002/adma.200305136
Szewczyk, P. K., Metwally, S., Karbowniczek, J. E., Marzec, M. M., Stodolak-Zych, E., Gruszczyński, A., et al. (2018). Surface-potential-controlled Cell Proliferation and Collagen Mineralization on Electrospun Polyvinylidene Fluoride (PVDF) Fiber Scaffolds for Bone Regeneration. ACS Biomater. Sci. Eng. 5, 582–593. doi:10.1021/acsbiomaterials.8b01108
Szewczyk, P. K., Metwally, S., Karbowniczek, J. E., Marzec, M. M., Stodolak-Zych, E., Gruszczyński, A., et al. (2019). Surface-Potential-Controlled Cell Proliferation and Collagen Mineralization on Electrospun Polyvinylidene Fluoride (PVDF) Fiber Scaffolds for Bone Regeneration. ACS Biomater. Sci. Eng. 5, 582–593. doi:10.1021/acsbiomaterials.8b01108
Tang, D., Chen, S., Hou, D., Gao, J., Jiang, L., Shi, J., et al. (2018). Regulation of Macrophage Polarization and Promotion of Endothelialization by NO Generating and PEG-YIGSR Modified Vascular Graft. Mater. Sci. Eng. C 84, 1–11. doi:10.1016/j.msec.2017.11.005
Unal, A. Z., and West, J. L. (2020). Synthetic ECM: Bioactive Synthetic Hydrogels for 3D Tissue Engineering. Bioconjugate Chem. 31, 2253–2271. doi:10.1021/acs.bioconjchem.0c00270
Unger, C., Gruene, M., Koch, L., Koch, J., and Chichkov, B. N. (2011). Time-resolved Imaging of Hydrogel Printing via Laser-Induced Forward Transfer. Appl. Phys. A 103, 271–277. doi:10.1007/s00339-010-6030-4
Wang, C., Yan, K.-W., Lin, Y.-D., and Hsieh, P. C. H. (2010). Biodegradable Core/shell Fibers by Coaxial Electrospinning: Processing, Fiber Characterization, and its Application in Sustained Drug Release. Macromolecules 43, 6389–6397. doi:10.1021/ma100423x
Wang, D., Xu, Y., Wang, L., Wang, X., Ren, C., Zhang, B., et al. (2020b). Expanded Poly(tetrafluoroethylene) Blood Vessel Grafts with Embedded Reactive Oxygen Species (ROS)-Responsive Antithrombogenic Drug for Elimination of Thrombosis. ACS Appl. Mater Interfaces 12, 29844–29853. doi:10.1021/acsami.0c07868
Wang, D., Xu, Y., Li, Q., and Turng, L.-S. (2020a). Artificial Small-Diameter Blood Vessels: Materials, Fabrication, Surface Modification, Mechanical Properties, and Bioactive Functionalities. J. Mat. Chem. B 8, 1801–1822. doi:10.1039/c9tb01849b
Wang, D., Xu, Y., Wang, L., Wang, X., Yan, S., Yilmaz, G., et al. (2020c). Long-term Nitric Oxide Release for Rapid Endothelialization in Expanded Polytetrafluoroethylene Small-Diameter Artificial Blood Vessel Grafts. Appl. Surf. Sci. 507, 145028. doi:10.1016/j.apsusc.2019.145028
Wang, L., Zhang, Y., Jiang, L., Yang, X., Zhou, Y., Wang, X., et al. (2019). Effect of Injection Speed on the Mechanical Properties of Isotactic Polypropylene Micro Injection Molded Parts Based on a Nanoindentation Test. J. Appl. Polym. Sci. 136, 47329. doi:10.1002/app.47329
Wang, X., Jiang, M., Zhou, Z., Gou, J., and Hui, D. (2017). 3D Printing of Polymer Matrix Composites: A Review and Prospective. Compos. Part B Eng. 110, 442–458. doi:10.1016/j.compositesb.2016.11.034
Wang, Z., Wang, Y., Yan, J., Zhang, K., Lin, F., Xiang, L., et al. (2021). Pharmaceutical Electrospinning and 3D Printing Scaffold Design for Bone Regeneration. Adv. drug Deliv. Rev. 174, 504–534. doi:10.1016/j.addr.2021.05.007
Wang, Z., Xu, L., Wu, X., and Chen, J. (2018). A Designable Surface via the Micro-molding Process. Microsystems Nanoeng. 4, 1–8. doi:10.1038/micronano.2017.99
Wen, M., Zhi, D., Wang, L., Cui, C., Huang, Z., Zhao, Y., et al. (2020). Local Delivery of Dual MicroRNAs in Trilayered Electrospun Grafts for Vascular Regeneration. ACS Appl. Mat. Interfaces 12, 6863–6875. doi:10.1021/acsami.9b19452
Xue, J., Wu, T., Dai, Y., and Xia, Y. (2019). Electrospinning and Electrospun Nanofibers: Methods, Materials, and Applications. Chem. Rev. 119, 5298–5415. doi:10.1021/acs.chemrev.8b00593
Xue, J., Xie, J., Liu, W., and Xia, Y. (2017). Electrospun Nanofibers: New Concepts, Materials, and Applications. Acc. Chem. Res. 50, 1976–1987. doi:10.1021/acs.accounts.7b00218
Xue, X., Hu, Y., Deng, Y., and Su, J. (2021). Recent Advances in Design of Functional Biocompatible Hydrogels for Bone Tissue Engineering. Adv. Funct. Mat. 31, 2009432. doi:10.1002/adfm.202009432
Yahia, S., Khalil, I. A., and El-Sherbiny, I. M. (2019). Sandwich-like Nanofibrous Scaffolds for Bone Tissue Regeneration. ACS Appl. Mat. Interfaces 11, 28610–28620. doi:10.1021/acsami.9b06359
Yang, C., Li, Q., Nie, M., and Wang, Q. (2021a). Preferential Alignment of Two-Dimensional Montmorillonite in Polyolefin Elastomer Tube via Rotation Extrusion Featuring Biaxial Stress Field. Compos. Sci. Technol. 215, 109034. doi:10.1016/j.compscitech.2021.109034
Yang, G., Li, X., He, Y., Ma, J., Ni, G., and Zhou, S. (2018). From Nano to Micro to Macro: Electrospun Hierarchically Structured Polymeric Fibers for Biomedical Applications. Prog. Polym. Sci. 81, 80–113. doi:10.1016/j.progpolymsci.2017.12.003
Yang, H., Luo, X., Shen, K., Yuan, Y., Fu, Q., Gao, X., et al. (2019). The Role of Mandrel Rotation Speed on Morphology and Mechanical Properties of Polyethylene Pipes Produced by Rotational Shear. Polymer 184, 121915. doi:10.1016/j.polymer.2019.121915
Yang, X., Wang, Y., Zhou, Y., Chen, J., and Wan, Q. (2021b). The Application of Polycaprolactone in Three-Dimensional Printing Scaffolds for Bone Tissue Engineering. Polymers 13, 2754. doi:10.3390/polym13162754
Yatsushiro, S., Yamamoto, T., Yamamura, S., Abe, K., Obana, E., Nogami, T., et al. (2016). Application of a Cell Microarray Chip System for Accurate, Highly Sensitive, and Rapid Diagnosis for Malaria in Uganda. Sci. Rep. 6, 30136–30139. doi:10.1038/srep30136
Yim, E. K. F., Darling, E. M., Kulangara, K., Guilak, F., and Leong, K. W. (2010). Nanotopography-induced Changes in Focal Adhesions, Cytoskeletal Organization, and Mechanical Properties of Human Mesenchymal Stem Cells. Biomaterials 31, 1299–1306. doi:10.1016/j.biomaterials.2009.10.037
Yu, D.-G., Li, X.-Y., Wang, X., Yang, J.-H., Bligh, S. W. A., and Williams, G. R. (2015). Nanofibers Fabricated Using Triaxial Electrospinning as Zero Order Drug Delivery Systems. ACS Appl. Mat. Interfaces 7, 18891–18897. doi:10.1021/acsami.5b06007
Yue, Y., Gong, X., Jiao, W., Li, Y., Yin, X., Si, Y., et al. (2021). In-situ Electrospinning of Thymol-Loaded Polyurethane Fibrous Membranes for Waterproof, Breathable, and Antibacterial Wound Dressing Application. J. Colloid Interface Sci. 592, 310–318. doi:10.1016/j.jcis.2021.02.048
Zanchetta, E., Guidi, E., Della Giustina, G., Sorgato, M., Krampera, M., Bassi, G., et al. (2015). Injection Molded Polymeric Micropatterns for Bone Regeneration Study. ACS Appl. Mat. Interfaces 7, 7273–7281. doi:10.1021/acsami.5b00481
Zhang, B., Pei, X., Song, P., Sun, H., Li, H., Fan, Y., et al. (2018a). Porous Bioceramics Produced by Inkjet 3D Printing: Effect of Printing Ink Formulation on the Ceramic Macro and Micro Porous Architectures Control. Compos. Part B Eng. 155, 112–121. doi:10.1016/j.compositesb.2018.08.047
Zhang, C., Li, Y., Kang, W., Liu, X., and Wang, Q. (2021). Current Advances and Future Perspectives of Additive Manufacturing for Functional Polymeric Materials and Devices. SusMat 1, 127–147. doi:10.1002/sus2.11
Zhang, H., Cong, Y., Osi, A. R., Zhou, Y., Huang, F., Zaccaria, R. P., et al. (2020). Direct 3D Printed Biomimetic Scaffolds Based on Hydrogel Microparticles for Cell Spheroid Growth. Adv. Funct. Mat. 30, 1910573. doi:10.1002/adfm.201910573
Zhang, K., Li, X., Nie, M., and Wang, Q. (2018b). Helical Flow-Driven Alignment of Off-Axial Silver-Functionalized Titanium Dioxide Fibers in Polypropylene Tube Suitable for Medical Applications. Compos. Sci. Technol. 158, 121–127. doi:10.1016/j.compscitech.2018.02.022
Zhang, X., Xu, Y., Zhang, X., Wu, H., Shen, J., Chen, R., et al. (2019). Progress on the Layer-By-Layer Assembly of Multilayered Polymer Composites: Strategy, Structural Control and Applications. Prog. Polym. Sci. 89, 76–107. doi:10.1016/j.progpolymsci.2018.10.002
Zhao, L., Li, X., Yang, L., Sun, L., Mu, S., Zong, H., et al. (2021). Evaluation of Remodeling and Regeneration of Electrospun PCL/fibrin Vascular Grafts In Vivo. Mater. Sci. Eng. C 118, 111441. doi:10.1016/j.msec.2020.111441
Zhao, X., Liao, T., Yang, X., Coates, P., Whiteside, B., Barker, D., et al. (2022). Mold Temperature- and Molar Mass-dependent Structural Formation in Micro-injection Molding of Isotactic Polypropylene. Polymer 248, 124797. doi:10.1016/j.polymer.2022.124797
Zhao, Y., Cao, X., and Jiang, L. (2007). Bio-mimic Multichannel Microtubes by a Facile Method. J. Am. Chem. Soc. 129, 764–765. doi:10.1021/ja068165g
Keywords: advanced polymer processing, micro extrusion, micro injection molding, 3D printing, bone repair, biomedical engineering
Citation: Zhang T, Nie M and Li Y (2022) Current Advances and Future Perspectives of Advanced Polymer Processing for Bone and Tissue Engineering: Morphological Control and Applications. Front. Bioeng. Biotechnol. 10:895766. doi: 10.3389/fbioe.2022.895766
Received: 15 March 2022; Accepted: 11 May 2022;
Published: 26 May 2022.
Edited by:
Muhammad Wajid Ullah, Jiangsu University, ChinaReviewed by:
Ismat Ullah, Suzhou Institute of Nano-tech and Nano-bionics (CAS), ChinaDietmar Drummer, University of Erlangen Nuremberg, Germany
Copyright © 2022 Zhang, Nie and Li. This is an open-access article distributed under the terms of the Creative Commons Attribution License (CC BY). The use, distribution or reproduction in other forums is permitted, provided the original author(s) and the copyright owner(s) are credited and that the original publication in this journal is cited, in accordance with accepted academic practice. No use, distribution or reproduction is permitted which does not comply with these terms.
*Correspondence: Yijun Li, ruddph@gmail.com