- 1School of Mechanical Engineering, College of Engineering, University of Tehran, Tehran, Iran
- 2Department of Urology, School of Medicine, Tehran University of Medical Sciences, Tehran, Iran
- 3Pediatric Urology Research Center, Children’s Medical Center, Tehran, Iran
Due to the well-known biocompatibility, tunable biodegradability, and mechanical properties, silk fibroin hydrogel is an exciting material for localized drug delivery systems to decrease the therapy cost, decrease the negative side effects, and increase the efficiency of chemotherapy. However, the lack of remote stimuli response and active drug release behavior has yet to be analyzed comparatively. In this study, we developed magnetic silk fibroin (SF) hydrogel samples through the facile blending method, loaded with doxorubicin hydrochloride (DOX) and incorporated with different concentrations of iron oxide nanoparticles (IONPs), to investigate the presumable ability of controlled and sustained drug release under the various external magnetic field (EMF). The morphology and rheological properties of SF hydrogel and magnetic SF hydrogel were compared through FESEM images and rheometer analysis. Here, we demonstrated that adding magnetic nanoparticles (MNPs) into SFH decreased the complex viscosity and provided a denser porosity with a bigger pore size matrix structure, which allowed the drug to be released faster in the absence of an EMF. Release kinetic studies show that magnetic SF hydrogel could achieve controlled release of DOX in the presence of an EMF. Furthermore, the drug release from magnetic SF hydrogel decreased in the presence of a static magnetic field (SMF) and an alternating magnetic field (AMF), and the release rate decreased even more with the higher MNPs concentration and magnetic field strength. Subsequently, Wilms’ tumor and human fibroblast cells were cultured with almost the same concentration of DOX released in different periods, and cell viability was investigated using MTT assay. MTT results indicated that the Wilms’ tumor cells were more resistant to DOX than the human fibroblasts, and the IC50 values were calculated at 1.82
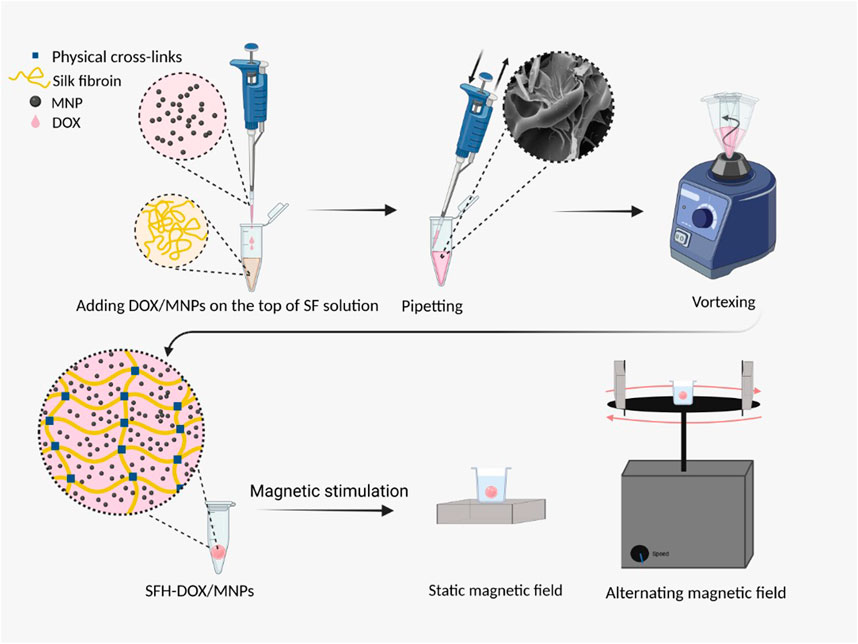
GRAPHICAL ABSTRACT Preparation and magnetic stimulation of SFH-DOX/MNPs for in vitro drug release study.
1 Introduction
Cancer is one of the leading causes of morbidity and mortality worldwide (Fitzmaurice et al., 2015), and Wilms’ tumor is the most common kidney cancer in young children, diagnosing 500 to 600 new cases each year in the united states alone (American Cancer Society, 2020). The main cancer treatments involve surgery, chemotherapy, and radiotherapy (Zitvogel et al., 2008; Bhatnagar, 2009). Despite many drugs and treatments developed, one of the problems in chemotherapy is the side effects of systemic chemotherapy, which is caused by free drug distribution, nonspecific targeting, short blood circulation half-life, fast in vivo metabolization, and drug resistance (Tian et al., 2014). By now, many nanometer-scale drug delivery systems based on natural and synthetic polymers have been developed to ameliorate these issues (Sung and Kim, 2020; Yao et al., 2020). Interestingly, silk is a natural, abundant, and reasonable cost polymeric source of biomaterials, demonstrating extraordinary mechanical properties, biocompatibility, and tunable biodegradability (Nguyen et al., 2019; Tomeh et al., 2019; Kochhar et al., 2021). Silk is composed of two main proteins, sericin, and fibroin. Despite having many biological activities, such as anti-bacterium, anti-coagulation, anti-oxidation, and promoting cell growth and differentiation (Wang et al., 2014), sericin is commonly extracted because of its combination with fibroin invokes an inflammatory response (Aramwit et al., 2009; Lam et al., 2021). Silk fibroin (SF) materials have been used as a desirable biomaterial, due to their physicochemical and mechanical properties, for several applications in biomedical and pharmaceutical through the past decades, such as small molecule drug delivery (Seib et al., 2013; Zhong et al., 2015; Gharehnazifam et al., 2021), gene delivery (Huang et al., 2016), biological drug delivery (Guziewicz et al., 2011), wound healing (Chouhan et al., 2018; He et al., 2019; Maaz Arif et al., 2021; Vidya and Rajagopal, 2021), cartilage regeneration (Cheng et al., 2018), and bone generation (Fini et al., 2005; Jin et al., 2015; Yan et al., 2019). SF as a biomaterial can be implemented in various forms of fabrication such as sponges, tubes, fibers, films, microspheres, and hydrogels as implantable and injectable systems (Rockwood et al., 2011). SF hydrogels have a shear-thinning behavior (Guziewicz et al., 2011) that provides the ability of injection and can be used as a career for a drug to provide bioavailability and sustainable release in the tumor site. Responding to the need to lower chemotherapy’s side effects, many researchers have utilized localized chemotherapy with a controlled and sustained drug release, using regulating endogenous parameters (Pritchard and Kaplan, 2011; de Moraes et al., 2015; Harris et al., 2016; Zuluaga-Vélez et al., 2019) or with SF’s different micro-environmental stimuli response properties hydrogel (Gou et al., 2020). However, controlling the release profile through remote stimuli is still an issue, leading to greater control to optimize the therapy. One strategy for remote controlling the drug release is using ferrogels, which can be prepared by introducing magnetic nanoparticles (MNPs) to the polymeric chain of the hydrogel to create a magneto-responsive hydrogel (Frachini and Petri, 2019). The previous researchers investigated the drug release profile of many different ferrogels (Liu et al., 2006; Hu et al., 2018). However, there have been no studies on magnetic silk-based hydrogel drug release behavior to the best of our knowledge.
This study aims to investigate the ability of sustained and remotely controlled release of the drug encapsulated in the magnetic SF hydrogel, using the regulation of external magnetic field (EMF) and MNPs concentration. For this purpose, Iron oxide nanoparticles (IONPs) were introduced to the SF solution through the blending method (Frachini and Petri, 2019), and the drug model was encapsulated in the composition using physical crosslinks. Physical crosslinking is safe, inexpensive, and has less toxicity for cells than crosslinking using chemical agents; thus, it is particularly of interest (Johari et al., 2020).
IONPs have different biomedical applications, such as diagnosis and treatment, and have been widely used as contrast agents for bioimaging, magnetic hyperthermia agents, and drug delivery (Xu C. et al., 2019). Moreover, IONPs have superparamagnetic properties (less than 50 nm) (Frachini and Petri, 2019) and are clinically approved (10–300 nm) (Jin et al., 2014). Doxorubicin hydrochloride (DOX) has been taken as a drug model, mainly used to treat Wilms’ tumor (Green et al., 2007; Malogolowkin et al., 2008; Spreafico et al., 2009; Oostveen and Pritchard-Jones, 2019), which usually causes issues like drug resistance and free drug distribution (Smith et al., 2006; Christowitz et al., 2019). Samples of SF hydrogel loaded with DOX and MNPs were developed to address these problems and were used as drug delivery systems to monitor the drug release rate profile. This approach makes magnetic SF hydrogel a multi-responsive system for sustained and controlled drug release.
A field emission scanning electron microscope (FESEM) was conducted to investigate the morphology of SF solution in comparison with SF/MNPs solution, which has a critical role in the release behavior of drugs from the SF scaffolds. In general, the morphology of hydrogels can reflect vital characteristics such as swelling behavior and viscosity (Park et al., 2013; Hu et al., 2017; Hu et al., 2018; Tanasić et al., 2021). Moreover, a rheometer was used for the study of the rheological properties of SF hydrogel and the effect of the addition of MNPs on its viscoelasticity and mechanical strength, which is mainly related to the crosslink density (Piluso et al., 2020). The rate of the drug released was monitored during different periods using spectrophotometry. Shortly, the effect of different parameters on the drug release rate was evaluated through different scenarios. Investigated parameters include; 1) the addition of MNPs to the SF hydrogel in passive mode, 2) applying different EMFs on the magnetic SF hydrogel, 3) the concentration of MNPs while applying an EMF, and 4) environmental pH. In the end, the bioactivity of the released DOX was accessed in vitro on human fibroblast and Wilms’ tumor cells through an MTT assay to evaluate and compare the cell death in the exposure of different Dox concentrations released in different time intervals from scaffolds. An overview of the present study is illustrated in Figure 1.
2 Materials and Methods
2.1 Materials
Bombyx Mori cocoons were supplied by a local market (from Chamkhaleh, Gilan, Iran). Collagenase type II, dispase, fetal bovine serum (FBS), Dulbecco’s Modified Eagle Medium and ham’s F12 medium (DMED-F12), Penicillin/streptomycin
2.2 Silk Fibroin Solution
The preparation of the SF solution was based on the previously described protocol by Kaplan and coworkers (Rockwood et al., 2011). Briefly, Bombyx mori cocoons were cut into small pieces after cleaning out of warm. Silk cocoons were boiled for 30 min in 0.02 M Na2CO3 solution to remove sericin protein. Then, degummed silk fibers were washed for 20 min three times with ultrapure water, squeezed, and allowed to air-dry overnight at room temperature. Parameters in the degumming process, such as time, Na2CO3 concentration, volume, and temperature, have an essential impact on resultant silk fibroin mechanical properties, molecular weight, and crystallinity, which can be optimized for different purposes (Seib et al., 2013; Bucciarelli et al., 2021). In the next step, SF fibers were dissolved in 9.3 M LiBr solution and were incubated at 62°C for 4 h, followed by dialyzing the solution against deionized water in a dialysis membrane [molecular weight cut-off (MWCO) 12,000 Da] for 48 h, following by centrifuging two times for 20 min in 4°C and 9,000 rpm. The resulting solution had an approximate concentration of 8%
2.3 DOX-Loaded Magnetic Silk Fibroin Hydrogel
DOX-loaded magnetic SF hydrogel was prepared in the fast and facile blending method (Li et al., 2013; Frachini and Petri, 2019). 25, 50, and 75
2.4 Field Emission Scanning Electron Microscope Observation
2.4.1 Silk Fibroin Sample
SF Solution was mixed with water in the ratio of 1:3 and freeze-dried using liquid N2 after being frozen. Water was added to the SF solution to liken the condition to the situation where SF hydrogels are loaded with a drug.
2.4.2 Magnetic Silk Fibroin Sample
25
2.4.3 Morphological Analysis
The morphology of SF and magnetic SF samples was observed using a HITACHI S4160 field emission scanning electron microscope (FESEM) (Tokyo, Japan). The samples were freeze-dried under identical conditions, coated with a conductive adhesive layer, and sprayed with a layer of gold to electrically conductive, for higher image resolution, photographed with an accelerating voltage of 20 kV at room temperature.
2.5 Rheological Characterization
The rheological properties of SFH-DOX and SFH-DOX/MNP1 were measured for comparison using an MCR 502 rheometer (Anton Paar, Hertford Herts, United Kingdom) at a controlled temperature of 37°C. 3 ml of SF-DOX and SF-DOX/MNP1 solutions were sonicated gelled (5 s at 20% amplitude) in a cylindrical mold with a diameter of 25 mm and a height of 3 mm and incubated at 37°C until the gelation occurred eventually (12 h). For the frequency sweeping test, complex viscosity
2.6 In Vitro Drug Release Study
To determine the release profile of DOX, 200
A total of eight different samples were used for evaluating the effect of the following parameters on the drug release rate.
1) Addition of MNPs: to independently evaluate the effects of the adding of MNPs on the passive DOX release, SFH-DOX, and SFH-DOX/MNP1 were incubated in the absence of any magnetic field in PBS 10X (pH 6.76) at 37°C.
2) EMF: to observe the effect of applying a magnetic field on the release rate, drug release from SFH-DOX/MNP1 in the absence of EMF is considered as control and compared with those of 0.18 T static magnetic field (SMF1), 0.28 T static magnetic field (SMF2), and 5 mT alternating magnetic field (AMF1) were applied. SMF1 and SMF2 were stationarily incubated with the hydrogel, while AMF1 was pulsed and applied for an hour (10 min on-5 min off) before each sample collection time point. The SMFs were created using neodymium magnets (30 cm length
3) MNPs concentration: to observe the effect of MNPs concentration on drug release profile, SFH-DOX/MNP1, SFH-DOX/MNP2, and SFH-DOX/MNP3 were compared in the presence of SMF2. Accurate details of sample components and conditions used in different scenarios are mentioned in Table 1.
4) Environmental pH: the effect of medium pH on in vitro drug release rate was investigated through soaking SFH-DOX/MNP1 in PBS 10X, with two different pHs 6.76 and 7.49.

TABLE 1. Nomenclature, composites, and properties of applied external actuation of silk fibroin hydrogel.
2.7 Cell Culture
Human fibroblast cells (isolated from the foreskin by enzymatic digestion) and Wilms’ tumor cells (by explant culture) were cultured in DMEM-F12 containing 10% (FBS) and 1% penicillin and streptomycin. All cells were incubated in a humid incubator at 37°C with 5% CO2 and 95% humidity, and trypsin-EDTA passaged at 70–90% confluence. Human fibroblast and Wilms’ tumor cells were used up to the third and fourth passage, respectively.
2.8 In vitro Cytotoxicity Assay
MTT assay was employed to quantification of cell viability. This method is based on the reduction of the tetrazolium salt solution (MTT) to purple formazan by metabolically active cells. Human fibroblast and Wilms’ tumor cells were plated in 96-well plates at an initial density of
In the next step, the culture medium was aspirated, 180
3 Results and Discussion
3.1 Morphology Observation
The freeze-dried SF solutions were prepared to observe the FESEM appearance. The morphology of the SF solution and SF/MNP1 solution is presented in Figure 2. The FESEM results exhibited that the internal structure of the SF and magnetic SF is a porous three-dimensional network structure with an irregular pore shape, and pore arrangement had no particular direction. FESEM images indicated that SF and magnetic SF have a porous fibrillary matrix, allowing the drug to release through the matrix. Furthermore, the average diameter of the pores and porosity seemed to increase with the addition of Fe3O4 nanoparticles in the SF solution. Quantitative measurements were assessed using ImageJ software. The porosity of SFH and SFH/MNP1 lyophilized solution was calculated at 14.306
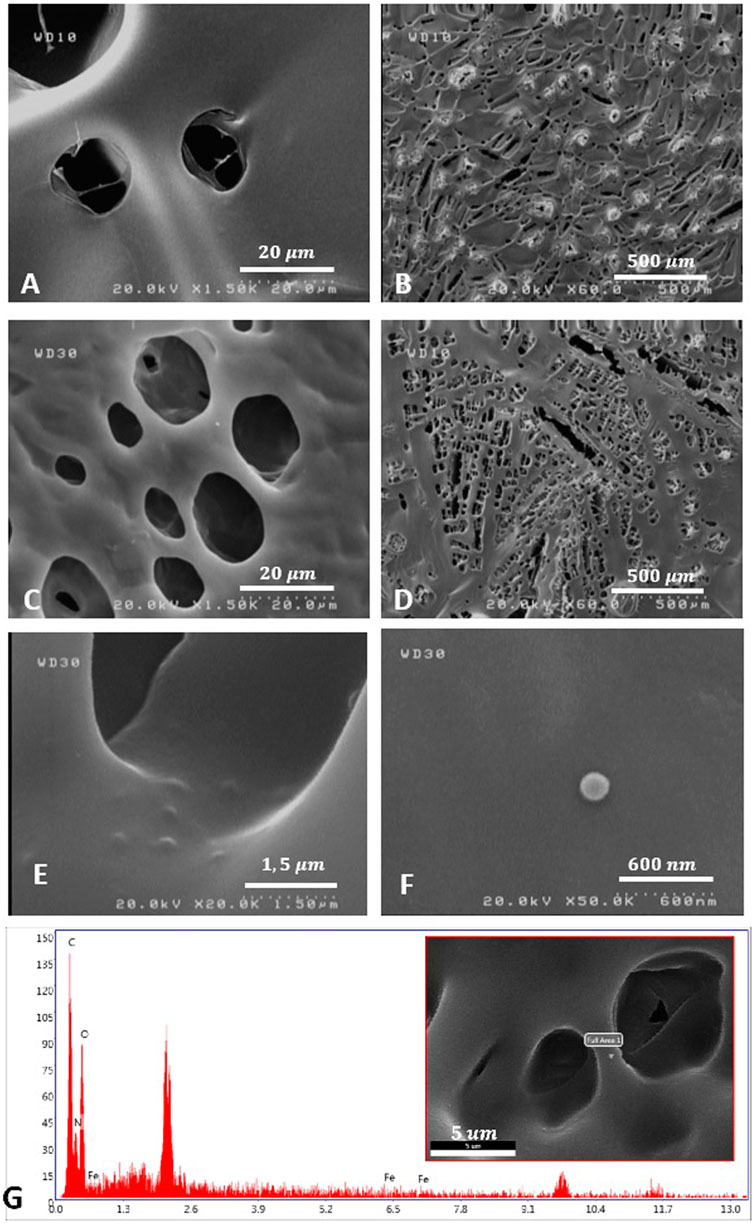
FIGURE 2. The morphology observation of SFH (A,B), and SFH/MNP1 (C–F) using FESEM. (G) Material characterization of SFH/MNP1 using Energy Dispersive X-Ray Analysis (EDAX) in the selected area.
3.2 Rheological Properties
An angular frequency sweep was performed to analyze the physical nature of hydrogels. The dynamic complex viscosity
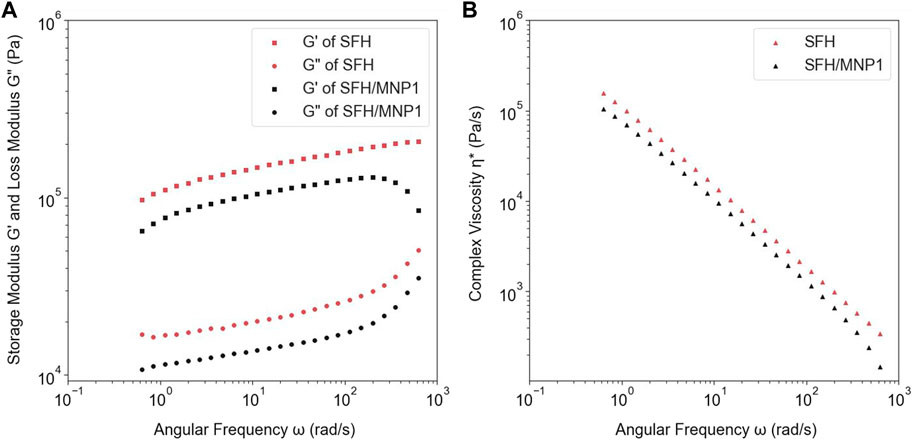
FIGURE 3. (A) The comparison between the storage and loss modulus of SFH-DOX and SFH-DOX/MNP1. (B) The changes of complex viscosity of SFH-DOX and SFH-DOX/MNP1 during the different angular frequencies.
3.3 In Vitro Releasing Profile
In vitro drug release pattern was investigated in eight different samples to study the effect of adding MNPs, the concentration of MNPs, applying an EMF, and pH in drug release from the SFH. In all samples, DOX was continuously released from the hydrogels for more than 35 days, representing the sustainable behavior of the drug release. No initial burst release was observed, meaning that all of the drugs loaded successfully in the SF scaffolds (Figure 4).
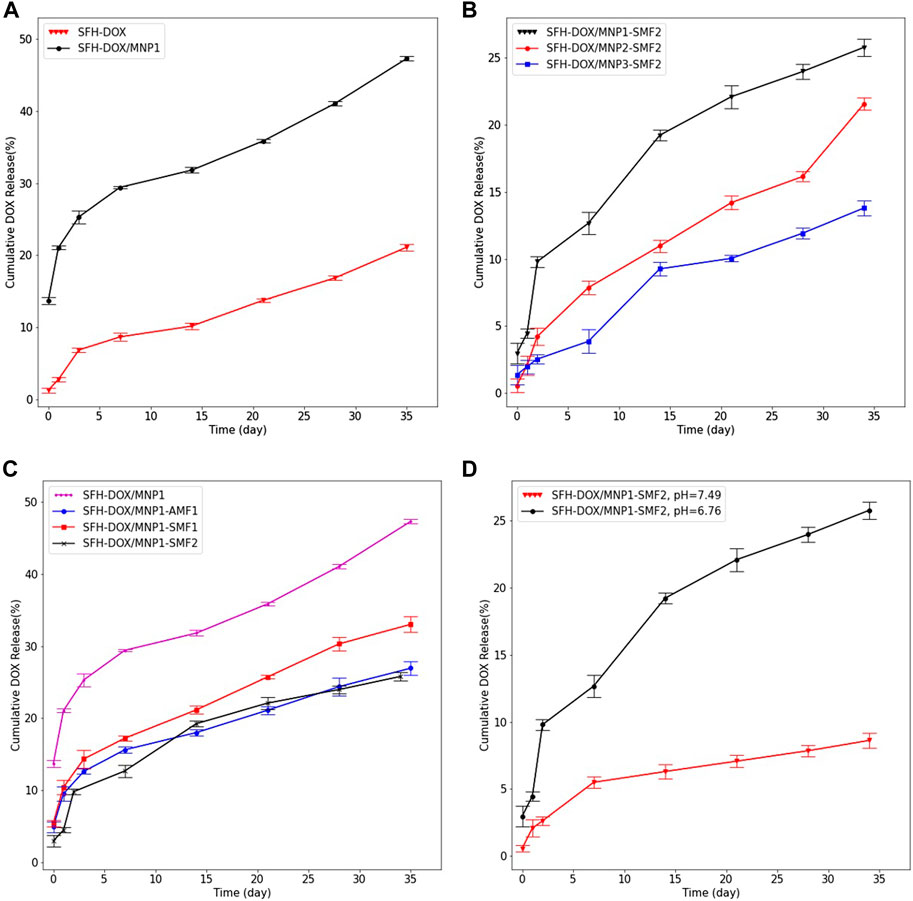
FIGURE 4. Evaluation of multiple stimuli-responsive properties of SFH. In vitro cumulative release profile of DOX from (A); SFH and SFH/MNP1 in the absence of an EMF, (B); different magnetic hydrogels containing different concentrations of MNPs, in the presence of 0.28 T SMF, (C); SFH/MNP1 under the various EMF, (D); SFH/MNP1 in two different release buffers acidity. Data are expressed as mean ± SD.
The influence of the addition of MNPs on the release of encapsulated DOX was investigated by studying the release of the DOX from SFH-DOX/MNP1 and compared with that of SFH-DOX. The result showed that the encapsulated DOX was released for a prolonged period from SFH-DOX compared to SFH-DOX/MNP1 in the absence of an EMF (Figure 4A). At 35 days, the cumulative release of DOX from SFH-DOX/MNP1 was more than twice faster (47.33%) than that without MNPs (21.12%). The difference in release patterns is closely associated with the viscosity and porosity, as described previously. The lower is the viscosity, the more accessible is the movement of drugs (Li et al., 2001). Moreover, diffusion from the pores is the primary mechanism of drug release (Peppas, 2000; Holback et al., 2011), and the addition of MNPs resulted in more porosity, thus, the drug release rate increased.
To study the effect of MNPs concentration on the release profile of DOX, three samples of SFH-DOX/MNP1, SFH-DOX/MNP2, and SFH-DOX/MNP3 were incubated in PBS 10X (pH 6.76), and SMF2 was applied for more than a month. Figure 4B shows that the cumulative release rate decreased with increasing MNPs in the formulation. We hypothesized that magnetic driven force caused direction movement of coated MNPs; thus, hydrogel pores were occupied because of the structure deformation, which reduced the drug release rate.
The SFH-DOX/MNP1 in the absence of EMF was taken as the control and compared with those under the SMF1, SMF2, and AMF1, to investigate the DOX cumulative release in the passive and EMF-induced mode (Figure 4C). The cumulative drug release was much higher in the absence of EMF than in the others. The in vitro release of DOX from SFH-DOX/MNP1 decreased with increasing the SMF strength. However, DOX release was more prevented in the presence of AMF1 compared with SMF1. This may contribute to coated magnetic nanoparticles’ orientation direction while applying a magnetic field. Pores are more likely to be blocked with the alternating direction of MNPs orientation than vertical orientation.
As presented in Figure 4D, after 34 days of incubation of SFH-DOX/MNP1 in the releasing media in the presence of SMF2, 8.62% of DOX was released in buffer (pH 7.49, mimicking blood plasma). However, much more DOX molecules (25.8%) were released in buffer (pH 6.76, extracellular environment in tumor site) compared to that in buffer with higher pH. These results demonstrated the inherent pH-responsibility of SFH-DOX (Gou et al., 2020), as investigated in previous studies. The enhancement in DOX release is due to weak electrostatic interactions between the drug and SF, in environments with lower pH (Duncan, 2003; Seib and Kaplan, 2012; Tian et al., 2014). Moreover, external factors like pH and temperature can affect drug release and polymer network degradation (de Moraes et al., 2015; Xu Z. et al., 2019; Gou et al., 2020). Therefore, the increase in drug release within lowering pH made this drug delivery system suitable for diminishing the side effects of chemotherapy by decreasing the drug accumulation in unfavorable sites.
3.4 Quantification of Cellular Viability
For evaluating cell viability and proliferation in the exposure of different DOX concentrations released from different hydrogel samples, Wilms’ tumor (Figures 5A,B) and human fibroblast (Figures 5C,D) cells were incubated for 48 h. The resulting absorbance was proportional to the number of viable cells at each concentration; thus, the viability could be assessed. The metabolic activities of human fibroblast cells gradually decreased over the increasing DOX concentration (Figure 5F). However, Wilms’ tumor cells viability almost did not change (not statistically significant) in the exposure of the highest concentration of 6.36 μg/ml (Figure 5E). These results suggest that Wilms’ tumor cancer cells should be exposed to a controlled DOX concentration to prevent cell resistance. The IC50 values of DOX for Wilms’ tumor were calculated at 2.73
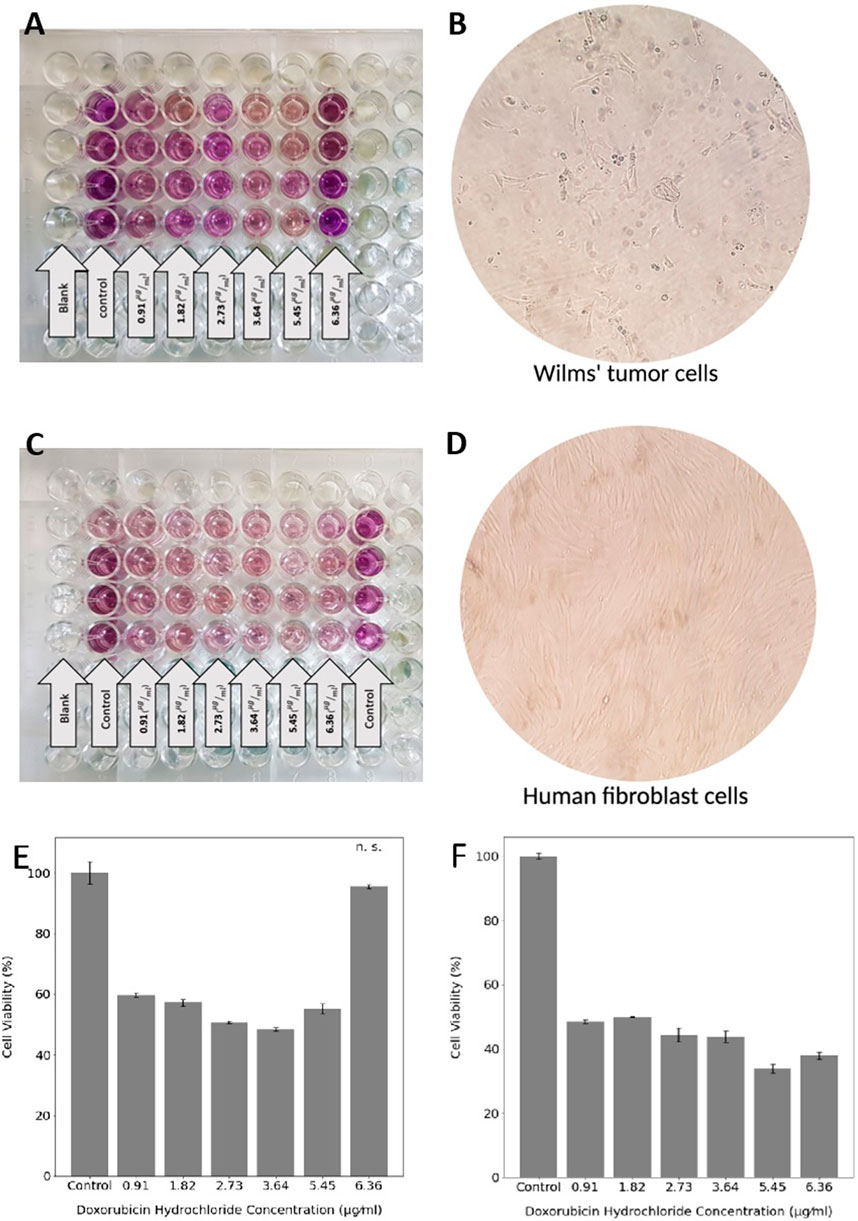
FIGURE 5. MTT assay of Wilms’ tumor and (A) and human fibroblast cells (C). The morphology of Wilms’ tumor (B) and human fibroblast (D) cells under the microscope. Cell viability of Wilms’ tumor (E) and human fibroblast (F) in the exposure of six different DOX concentrations. Statistical differences between the control and other groups were determined with ANOVA, mean ± SD; n = 4, p < 0.05, n. s. = not significant.
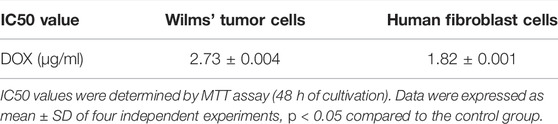
TABLE 2. IC50 values for Wilms’ tumor and human fibroblast cells, treated with different DOX concentrations.
4 Conclusion
The mechanical and rheological properties of SF hydrogel are reserved mainly with the addition of MNPs. The nanoparticle-assisted SF hydrogel introduces a 3D porous structure drug carrier with an extra function of magneto-responsive in addition to the pH and shear-responsive properties. These properties can be combined with governing the endogenous properties of SF, which significantly enable optimization of the drug release, providing more efficient therapy and minimizing the unwanted side effects. SF ferrogel with magneto-responsive ability has been prepared by introducing Fe3O4 nanoparticles to the SF solution through the blending method. This approach has developed as a drug delivery system with sustained and controlled release; this was achieved through simply tuning the concentration of MNPs and strength of the EMF. The in vitro drug release profile and MTT assay demonstrated that magnetic SF hydrogel works well as a novel drug delivery system in cancer therapy. This study proposes that controlled and sustained drug release can be achieved using optimization and regulation of magnetic SF hydrogels. Since SF hydrogels degrade faster in the conditions mimicking in vivo (Wang et al., 2008), such as the presence of enzymes and mechanical forces, this in vitro study was based on minimizing the drug release. Release assessment results pointed out that if magnetic SF hydrogel is used, an EMF should be applied; otherwise, it causes a higher drug release rate, which possibly leads to drug resistance. If lower drug release is favorable, the concentration of MNPs and the strength of the EMF should be maximized. Moreover, a low-frequency AMF with lower strength can reduce the release rate more in comparison to SMF. Future studies will focus on evaluating the drug release in mediums more mimicking the human body, in which the applied EMF is measurable in vitro. Numerical simulations also should be done for forecasting the drug release behavior, and the optimized magnetic hydrogels should be tested in vivo. Moreover, DOX can be combined with other chemotherapy drugs (both hydrophobic and hydrophilic) for Wilms’ tumor treatment, encapsulated in the hydrogels, and injected into the tumor site in vivo.
Data Availability Statement
The original contributions presented in the study are included in the article/Supplementary Material; further inquiries can be directed to the corresponding authors.
Author Contributions
MH: methodology, conceptualization, investigation, experimental, formal analysis, validation, visualization, and writing—review and editing. MOB: methodology, writing—original draft, validation, and supervision, AK: methodology, resources, validation, supervision, project administration, and writing—review and editing. ZG: formal analysis and writing—review and editing. BJ: methodology and experimental. MAB: methodology, resources, supervision, project administration, and writing—review and editing.
Conflict of Interest
The authors declare that the research was conducted in the absence of any commercial or financial relationships that could be construed as a potential conflict of interest.
Publisher’s Note
All claims expressed in this article are solely those of the authors and do not necessarily represent those of their affiliated organizations, or those of the publisher, the editors, and the reviewers. Any product that may be evaluated in this article, or claim that may be made by its manufacturer, is not guaranteed or endorsed by the publisher.
References
Aramwit, P., Kanokpanont, S., De-Eknamkul, W., and Srichana, T. (2009). Monitoring of Inflammatory Mediators Induced by Silk Sericin. J. Biosci. Bioeng. 107 (5), 556–561. doi:10.1016/j.jbiosc.2008.12.012
American Cancer Society (2020). Cancer Facts and Figures 2020. Atlanta, GA: American Cancer Society.
Barbucci, R., Rappuoli, R., Borzacchiello, A., and Ambrosio, L. (2000). Synthesis, Chemical and Rheological Characterization of New Hyaluronic Acid-Based Hydrogels. J. Biomaterials Sci. Polym. Ed. 11 (4), 383–399. doi:10.1163/156856200743779
Bhatnagar, S. (2009). Management of Wilms′ Tumor: NWTS vs SIOP. J. Indian Assoc. Pediatr. Surg. 14 (1), 6. doi:10.4103/0971-9261.54811
Bucciarelli, A., Greco, G., Corridori, I., Pugno, N. M., and Motta, A. (2021). A Design of Experiment Rational Optimization of the Degumming Process and its Impact on the Silk Fibroin Properties. ACS Biomater. Sci. Eng. 7 (4), 1374–1393. doi:10.1021/acsbiomaterials.0c01657
Cheng, G., Davoudi, Z., Xing, X., Yu, X., Cheng, X., Li, Z., et al. (2018). Advanced Silk Fibroin Biomaterials for Cartilage Regeneration. ACS Biomater. Sci. Eng. 4 (8), 2704–2715. doi:10.1021/acsbiomaterials.8b00150
Chouhan, D., Lohe, T. U., Samudrala, P. K., and Mandal, B. B. (2018). In Situ Forming Injectable Silk Fibroin Hydrogel Promotes Skin Regeneration in Full Thickness Burn Wounds. Adv. Healthc. Mater 7 (24), e1801092. doi:10.1002/adhm.201801092
Christowitz, C., Davis, T., Isaacs, A., van Niekerk, G., Hattingh, S., and Engelbrecht, A. M. (2019). Mechanisms of Doxorubicin-Induced Drug Resistance and Drug Resistant Tumour Growth in a Murine Breast Tumour Model. BMC cancer 19 (1), 1–10. doi:10.1186/s12885-019-5939-z
de Moraes, M. A., Mahl, C. R. A., Silva, M. F., and Beppu, M. M. (2015). Formation of Silk Fibroin Hydrogel and Evaluation of its Drug Release Profile. J. Appl. Polym. Sci. 132 (15). n/a-n/a. doi:10.1002/app.41802
Duncan, R. (2003). The Dawning Era of Polymer Therapeutics. Nat. Rev. Drug Discov. 2 (5), 347–360. doi:10.1038/nrd1088
Fini, M., Motta, A., Torricelli, P., Giavaresi, G., Nicoli Aldini, N., Tschon, M., et al. (2005). The Healing of Confined Critical Size Cancellous Defects in the Presence of Silk Fibroin Hydrogel. Biomaterials 26 (17), 3527–3536. doi:10.1016/j.biomaterials.2004.09.040
Fitzmaurice, C., Fitzmaurice, C., Dicker, D., Pain, A., Hamavid, H., Moradi-Lakeh, M., et al. (2015). The Global Burden of Cancer 2013. JAMA Oncol. 1 (4), 505–527. doi:10.1001/jamaoncol.2015.0735
Frachini, E., and Petri, D. (2019). Magneto-Responsive Hydrogels: Preparation, Characterization, Biotechnological and Environmental Applications. J. Braz. Chem. Soc, 1–19. doi:10.21577/0103-5053.20190074
Gharehnazifam, Z., Dolatabadi, R., Baniassadi, M., Shahsavari, H., Kajbafzadeh, A.-M., Abrinia, K., et al. (2021). Computational Analysis of Vincristine Loaded Silk Fibroin Hydrogel for Sustained Drug Delivery Applications: Multiphysics Modeling and Experiments. Int. J. Pharm. 609, 121184. doi:10.1016/j.ijpharm.2021.121184
Gou, S., Xie, D., Ma, Y., Huang, Y., Dai, F., Wang, C., et al. (2020). Injectable, Thixotropic, and Multiresponsive Silk Fibroin Hydrogel for Localized and Synergistic Tumor Therapy. ACS Biomater. Sci. Eng. 6 (2), 1052–1063. doi:10.1021/acsbiomaterials.9b01676
Green, D. M., Cotton, C. A., Malogolowkin, M., Breslow, N. E., Perlman, E., Miser, J., et al. (2007). Treatment of Wilms Tumor Relapsing after Initial Treatment with Vincristine and Actinomycin D: A Report from the National Wilms Tumor Study Group. Pediatr. Blood Cancer 48 (5), 493–499. doi:10.1002/pbc.20822
Guziewicz, N., Best, A., Perez-Ramirez, B., and Kaplan, D. L. (2011). Lyophilized Silk Fibroin Hydrogels for the Sustained Local Delivery of Therapeutic Monoclonal Antibodies. Biomaterials 32 (10), 2642–2650. doi:10.1016/j.biomaterials.2010.12.023
Harris, J. C., Coburn, J. M., Kajdacsy-Balla, A., Kaplan, D. L., and Chiu, B. (2016). Sustained Delivery of Vincristine inside an Orthotopic Mouse Sarcoma Model Decreases Tumor Growth. J. Pediatr. Surg. 51 (12), 2058–2062. doi:10.1016/j.jpedsurg.2016.09.040
Hasturk, O., Jordan, K. E., Choi, J., and Kaplan, D. L. (2020). Enzymatically Crosslinked Silk and Silk-Gelatin Hydrogels with Tunable Gelation Kinetics, Mechanical Properties and Bioactivity for Cell Culture and Encapsulation. Biomaterials 232, 119720. doi:10.1016/j.biomaterials.2019.119720
He, S., Shi, D., Han, Z., Dong, Z., Xie, Y., Zhang, F., et al. (2019). Heparinized Silk Fibroin Hydrogels Loading FGF1 Promote the Wound Healing in Rats with Full-Thickness Skin Excision. Biomed. Eng. Online 18 (1), 97. doi:10.1186/s12938-019-0716-4
Holback, H., Yeo, Y., and Park, K. (2011). Hydrogel Swelling Behavior and its Biomedical Applications. Biomed. Hydrogels, 3–24. doi:10.1533/9780857091383.1.3
Hu, X., Wang, Y., Zhang, L., and Xu, M. (2017). Morphological and Mechanical Properties of Tannic acid/PAAm Semi-IPN Hydrogels for Cell Adhesion. Polym. Test. 61, 314–323. doi:10.1016/j.polymertesting.2017.05.034
Hu, X., Wang, Y., Zhang, L., Xu, M., Zhang, J., and Dong, W. (2018). Magnetic Field-Driven Drug Release from Modified Iron Oxide-Integrated Polysaccharide Hydrogel. Int. J. Biol. Macromol. 108, 558–567. doi:10.1016/j.ijbiomac.2017.12.018
Huang, W., Tarakanova, A., Dinjaski, N., Wang, Q., Xia, X., Chen, Y., et al. (2016). Design of Multistimuli Responsive Hydrogels Using Integrated Modeling and Genetically Engineered Silk-Elastin‐Like Proteins. Adv. Funct. Mat. 26 (23), 4113–4123. doi:10.1002/adfm.201600236
Jin, R., Lin, B., Li, D., and Ai, H. (2014). Superparamagnetic Iron Oxide Nanoparticles for MR Imaging and Therapy: Design Considerations and Clinical Applications. Curr. Opin. Pharmacol. 18, 18–27. doi:10.1016/j.coph.2014.08.002
Jin, Y., Kundu, B., Cai, Y., Kundu, S. C., and Yao, J. (2015). Bio-inspired Mineralization of Hydroxyapatite in 3D Silk Fibroin Hydrogel for Bone Tissue Engineering. Colloids Surfaces B Biointerfaces 134, 339–345. doi:10.1016/j.colsurfb.2015.07.015
Johari, N., Moroni, L., and Samadikuchaksaraei, A. (2020). Tuning the Conformation and Mechanical Properties of Silk Fibroin Hydrogels. Eur. Polym. J. 134, 109842. doi:10.1016/j.eurpolymj.2020.109842
Kochhar, D., DeBari, M. K., and Abbott, R. D. (2021). The Materiobiology of Silk: Exploring the Biophysical Influence of Silk Biomaterials on Directing Cellular Behaviors. Front. Bioeng. Biotechnol. 9, 536. doi:10.3389/fbioe.2021.697981
Koetting, M. C., Peters, J. T., Steichen, S. D., and Peppas, N. A. (2015). Stimulus-responsive Hydrogels: Theory, Modern Advances, and Applications. Mater. Sci. Eng. R Rep. 93, 1–49. doi:10.1016/j.mser.2015.04.001
Lam, Y. T., Tan, R. P., Michael, P. L., Lau, K., Yang, N., Rnjak-Kovacina, J., et al. (2021). Bioengineering Silk into Blood Vessels. Biochem. Soc. Trans. 49 (5), 2271–2286. doi:10.1042/bst20210359
Li, M., Wu, Z., Zhang, C., Lu, S., Yan, H., Huang, D., et al. (2001). Study on Porous Silk Fibroin Materials. II. Preparation and Characteristics of Spongy Porous Silk Fibroin Materials. J. Appl. Polym. Sci. 79 (12), 2192–2199. doi:10.1002/1097-4628(20010321)79:12<2192::aid-app1027>3.0.co;2-0
Li, Y., Huang, G., Zhang, X., Li, B., Chen, Y., Lu, T., et al. (2013). Magnetic Hydrogels and Their Potential Biomedical Applications. Adv. Funct. Mat. 23 (6), 660–672. doi:10.1002/adfm.201201708
Liu, T.-Y., Hu, S.-H., Liu, T.-Y., Liu, D.-M., and Chen, S.-Y. (2006). Magnetic-sensitive Behavior of Intelligent Ferrogels for Controlled Release of Drug. Langmuir 22 (14), 5974–5978. doi:10.1021/la060371e
Maaz Arif, M., Khan, S. M., Gull, N., Tabish, T. A., Zia, S., Ullah Khan, R., et al. (2021). Polymer-based Biomaterials for Chronic Wound Management: Promises and Challenges. Int. J. Pharm. 598, 120270. doi:10.1016/j.ijpharm.2021.120270
Malogolowkin, M., Cotton, C. A., Green, D. M., Breslow, N. E., Perlman, E., Miser, J., et al. (2008). Treatment of Wilms Tumor Relapsing after Initial Treatment with Vincristine, Actinomycin D, and Doxorubicin. A Report from the National Wilms Tumor Study Group. Pediatr. Blood Cancer 50 (2), 236–241. doi:10.1002/pbc.21267
Nguyen, T. P., Nguyen, Q. V., Nguyen, V. H., Le, T. H., Huynh, V. Q. N., Vo, D. N., et al. (2019). Silk Fibroin-Based Biomaterials for Biomedical Applications: A Review. Polym. (Basel) 11 (12). doi:10.3390/polym11121933
Oostveen, R. M., and Pritchard-Jones, K. (2019). Pharmacotherapeutic Management of Wilms Tumor: An Update. Pediatr. Drugs 21 (1), 1–13. doi:10.1007/s40272-018-0323-z
Park, C. H., Jeong, L., Cho, D., Kwon, O. H., and Park, W. H. (2013). Effect of Methylcellulose on the Formation and Drug Release Behavior of Silk Fibroin Hydrogel. Carbohydr. Polym. 98 (1), 1179–1185. doi:10.1016/j.carbpol.2013.07.028
Peppas, N. (2000). Hydrogels in Pharmaceutical Formulations. Eur. J. Pharm. Biopharm. 50 (1), 27–46. doi:10.1016/s0939-6411(00)00090-4
Piluso, S., Flores Gomez, D., Dokter, I., Moreira Texeira, L., Li, Y., Leijten, J., et al. (2020). Rapid and Cytocompatible Cell-Laden Silk Hydrogel Formation via Riboflavin-Mediated Crosslinking. J. Mat. Chem. B 8 (41), 9566–9575. doi:10.1039/d0tb01731k
Pritchard, E. M., and Kaplan, D. L. (2011). Silk Fibroin Biomaterials for Controlled Release Drug Delivery. Expert Opin. Drug Deliv. 8 (6), 797–811. doi:10.1517/17425247.2011.568936
Rockwood, D. N., Preda, R. C., Yücel, T., Wang, X., Lovett, M. L., and Kaplan, D. L. (2011). Materials Fabrication from Bombyx mori Silk Fibroin. Nat. Protoc. 6 (10), 1612–1631. doi:10.1038/nprot.2011.379
Seib, F. P., and Kaplan, D. L. (2012). Doxorubicin-loaded Silk Films: Drug-Silk Interactions and In Vivo Performance in Human Orthotopic Breast Cancer. Biomaterials 33 (33), 8442–8450. doi:10.1016/j.biomaterials.2012.08.004
Seib, F. P., Pritchard, E. M., and Kaplan, D. L. (2013). Self-assembling Doxorubicin Silk Hydrogels for the Focal Treatment of Primary Breast Cancer. Adv. Funct. Mat. 23 (1), 58–65. doi:10.1002/adfm.201201238
Smith, L., Watson, M. B., O'Kane, S. L., Drew, P. J., Lind, M. J., and Cawkwell, L. (2006). The Analysis of Doxorubicin Resistance in Human Breast Cancer Cells Using Antibody Microarrays. Mol. Cancer Ther. 5 (8), 2115–2120. doi:10.1158/1535-7163.mct-06-0190
Spreafico, F., Pritchard Jones, K., Malogolowkin, M. H., Bergeron, C., Hale, J., de Kraker, J., et al. (2009). Treatment of Relapsed Wilms Tumors: Lessons Learned. Expert Rev. anticancer Ther. 9 (12), 1807–1815. doi:10.1586/era.09.159
Sung, Y. K., and Kim, S. W. (2020). Recent Advances in Polymeric Drug Delivery Systems. Biomater. Res. 24 (1), 12. doi:10.1186/s40824-020-00190-7
Tanasić, J., Erceg, T., Tanasić, L., Baloš, S., Klisurić, O., and Ristić, I. (2021). The Influence of Reaction Conditions on Structural Properties and Swelling Kinetics of Polyurethane Hydrogels Intended for Agricultural Purposes. React. Funct. Polym. 169, 105085. doi:10.1016/j.reactfunctpolym.2021.105085
Tian, Y., Jiang, X., Chen, X., Shao, Z., and Yang, W. (2014). Doxorubicin-loaded Magnetic Silk Fibroin Nanoparticles for Targeted Therapy of Multidrug-Resistant Cancer. Adv. Mat. 26 (43), 7393–7398. doi:10.1002/adma.201403562
Tomeh, M. A., Hadianamrei, R., and Zhao, X. (2019). Silk Fibroin as a Functional Biomaterial for Drug and Gene Delivery. Pharmaceutics 11 (10). doi:10.3390/pharmaceutics11100494
Vidya, M., and Rajagopal, S. (2021). Silk Fibroin: A Promising Tool for Wound Healing and Skin Regeneration. Int. J. Polym. Sci., 2021, 1–10. doi:10.1155/2021/9069924
Wang, X., Kluge, J. A., Leisk, G. G., and Kaplan, D. L. (2008). Sonication-induced Gelation of Silk Fibroin for Cell Encapsulation. Biomaterials 29 (8), 1054–1064. doi:10.1016/j.biomaterials.2007.11.003
Wang, Z., Zhang, Y., Zhang, J., Huang, L., Liu, J., Li, Y., et al. (2014). Exploring Natural Silk Protein Sericin for Regenerative Medicine: An Injectable, Photoluminescent, Cell-Adhesive 3D Hydrogel. Sci. Rep. 4 (1), 1–11. doi:10.1038/srep07064
Xu, C., Akakuru, O. U., Zheng, J., and Wu, A. (2019). Applications of Iron Oxide-Based Magnetic Nanoparticles in the Diagnosis and Treatment of Bacterial Infections. Front. Bioeng. Biotechnol. 7, 141. doi:10.3389/fbioe.2019.00141
Xu, Z., Tang, E., and Zhao, H. (2019). An Environmentally Sensitive Silk Fibroin/Chitosan Hydrogel and its Drug Release Behaviors. Polym. (Basel) 11 (12). doi:10.3390/polym11121980
Yan, Y., Cheng, B., Chen, K., Cui, W., Qi, J., Li, X., et al. (2019). Enhanced Osteogenesis of Bone Marrow-Derived Mesenchymal Stem Cells by a Functionalized Silk Fibroin Hydrogel for Bone Defect Repair. Adv. Healthc. Mater 8 (3), e1801043. doi:10.1002/adhm.201801043
Yao, Y., Zhou, Y., Liu, L., Xu, Y., Chen, Q., Wang, Y., et al. (2020). Nanoparticle-based Drug Delivery in Cancer Therapy and its Role in Overcoming Drug Resistance. Front. Mol. Biosci. 7, 193. doi:10.3389/fmolb.2020.00193
Zhong, T., Jiang, Z., Wang, P., Bie, S., Zhang, F., and Zuo, B. (2015). Silk Fibroin/copolymer Composite Hydrogels for the Controlled and Sustained Release of Hydrophobic/hydrophilic Drugs. Int. J. Pharm. 494 (1), 264–270. doi:10.1016/j.ijpharm.2015.08.035
Zitvogel, L., Apetoh, L., Ghiringhelli, F., and Kroemer, G. (2008). Immunological Aspects of Cancer Chemotherapy. Nat. Rev. Immunol. 8 (1), 59–73. doi:10.1038/nri2216
Zuluaga-Vélez, A., Cómbita-Merchán, D. F., Buitrago-Sierra, R., Santa, J. F., Aguilar-Fernández, E., and Sepúlveda-Arias, J. C. (2019). Silk Fibroin Hydrogels from the Colombian Silkworm Bombyx mori L: Evaluation of Physicochemical Properties. PLoS One 14 (3), e0213303. doi:10.1371/journal.pone.0213303
Keywords: silk fibroin, magnetic hydrogel, intelligent drug delivery, remote stimulation, sustained drug release
Citation: Haghighattalab M, Kajbafzadeh A, Baghani M, Gharehnazifam Z, Jobani BM and Baniassadi M (2022) Silk Fibroin Hydrogel Reinforced With Magnetic Nanoparticles as an Intelligent Drug Delivery System for Sustained Drug Release. Front. Bioeng. Biotechnol. 10:891166. doi: 10.3389/fbioe.2022.891166
Received: 07 March 2022; Accepted: 30 May 2022;
Published: 15 July 2022.
Edited by:
Hasan Uludag, University of Alberta, CanadaReviewed by:
Goutam Thakur, Manipal Institute of Technology, IndiaJiashen Li, The University of Manchester, United Kingdom
Copyright © 2022 Haghighattalab, Kajbafzadeh, Baghani, Gharehnazifam, Jobani and Baniassadi. This is an open-access article distributed under the terms of the Creative Commons Attribution License (CC BY). The use, distribution or reproduction in other forums is permitted, provided the original author(s) and the copyright owner(s) are credited and that the original publication in this journal is cited, in accordance with accepted academic practice. No use, distribution or reproduction is permitted which does not comply with these terms.
*Correspondence: Abdolmohammad Kajbafzadeh, a2FqYmFmemRAdHVtcy5hYy5pcg==; Majid Baniassadi, bS5iYW5pYXNzYWRpQHV0LmFjLmly