- 1Department of Plastic Surgery, The First Hospital of China Medical University, Shenyang, China
- 2Department of Breast Surgery, The First Hospital of China Medical University, Shenyang, China
- 3Liaoning Provincial Key Laboratory of Oral Diseases, School of Stomatology and Department of Oral Pathology, School of Stomatology, China Medical University, Shenyang, China
Implant-associated infection (IAI) is one of the major challenges in orthopedic surgery. The development of implants with inherent antibacterial properties is an effective strategy to resolve this issue. In recent years, biodegradable alloy materials have received considerable attention because of their superior comprehensive performance in the field of orthopedic implants. Studies on biodegradable alloy orthopedic implants with antibacterial properties have gradually increased. This review summarizes the recent advances in biodegradable magnesium- (Mg-), iron- (Fe-), and zinc- (Zn-) based alloys with antibacterial properties as orthopedic implant materials. The antibacterial mechanisms of these alloy materials are also outlined, thus providing more basis and insights on the design and application of biodegradable alloys with antibacterial properties as orthopedic implants.
1 Introduction
Currently, orthopedic implants have been broadly utilized to treat orthopedic and maxillofacial diseases, including deformity, osteoarthritis, and fracture (Kynaston-Pearson et al., 2013; Agarwal and García, 2015; Key et al., 2021; Farjam et al., 2022). Nevertheless, these implants are at risk of bacterial infection (Oliva et al., 2021). Actually, implant-associated infections (IAI) are among the most prevalent and severe complications in orthopedic surgery (Pfang et al., 2019). The occurrence of IAI not only means the failure of implant surgery but also requires secondary surgical repair and antibacterial therapy, which will inevitably increase the mental and economic pressure on patients (Peng et al., 2021a). IAI is primarily caused by bacteria located in the surgical approach and surgical site or brought in through blood and open wounds (Sendi et al., 2011; Tian et al., 2021). Biofilms that exert a protective effect on bacteria are formed on the surface of implants after the attachment and colonization of bacteria (Wagner and Hänsch, 2017). Clinically, antibiotic therapy remains the mainstay of treatment (Zimmerli and Sendi, 2017). Antibiotic-eluting strategies based on local diffusion are developed to address the issue of failure to achieve adequate concentrations at the site of infection by systemic use of antibiotics (Fei et al., 2011; Makarov et al., 2013). Although it is a big leap in antibacterial treatment, the ever-increasing occurrence of IAI remains unsolved. On the one hand, the formation of bacterial biofilm will be resistant to antibiotic treatment (Wagner and Hänsch, 2017). On the other hand, the release period of antibiotic-eluting devices can be short. Besides, the excessive use of antibiotics may greatly contribute to the emergence of drug-resistant bacteria (Gullberg et al., 2011). Therefore, an alternative is urgently needed to avoid the short life cycles of antibiotic-eluting devices and provide the implants with a lasting antibacterial effect. In light of this, the inherent antibacterial properties of some implant materials have gained increasing attention from researchers (Tran et al., 2015; Xu et al., 2016; Bee et al., 2021; Calabrese et al., 2021; Chen et al., 2021).
Alloy materials have always been favored in the area of orthopedic implants for their both excellent mechanical strength and mature fabrication process. There are numerous studies on traditional alloy materials, including stainless steel, cobalt-chromium (Co-Cr) alloys, and Ti alloys (Yamanaka et al., 2013; Al Jabbari, 2014; Li et al., 2014a; Bekmurzayeva et al., 2018; Xue et al., 2020). Researchers focused on enhancing the antibacterial effect of these traditional alloys during the early stage and attained fruitful results (Resnik et al., 2020; Guo et al., 2021a; Wang et al., 2021a; Lu et al., 2021; Watanabe et al., 2021). However, as permanent implants, these traditional alloy materials have many problems that need to be overcome. For instance, they carry the risk of complications such as intoxications and allergies, stress shielding problems, and secondary surgeries for implant removal (Sumner, 2015; Seyhan et al., 2018). In contrast, biodegradable alloys possess sufficient mechanical strength and can progressively degrade in vivo. Besides, a smaller host response ensues (Aghion, 2018). Moreover, the degradation process allows for shifting loads to healing tissues gradually, which resolves the stress shielding issues (Yuan et al., 2022). Furthermore, after full healing of tissues, the complete degradation of alloy materials obviates the need for secondary surgery (Zheng et al., 2014). Thus, biodegradable alloys have recently received significant attention, and there is a gradual increase in studies of biodegradable alloys with antibacterial properties.
At present, biodegradable alloys that are widely studied in the field of orthopedic implants include magnesium- (Mg-), iron- (Fe-), and zinc- (Zn-) based alloys. Although it is promising for the applications of biodegradable orthopedic implants with antibacterial properties, the development and application are still at an initial and exploratory stage. This review summarizes the recent advances in biodegradable Mg-, Fe-, and Zn-based alloys with antibacterial properties as orthopedic implant materials. The antibacterial mechanisms of these alloy materials are also outlined, thus providing more basis and insights on the design and application of biodegradable alloys with antibacterial properties as orthopedic implants.
2 The Main Pathogens and Prevention Strategies of Implant-Associated Infections
In general, the Gram-positive strains Staphylococcus aureus (S. aureus) and Staphylococcus epidermidis (S. epidermidis) are the most common causative agents of IAI in orthopedics (Arciola et al., 2005; Montanaro et al., 2011). They account for more than 70% of various causative agents (Arciola et al., 2005), followed by Gram-negative strains (Pseudomonas genus and Enterobacteriaceae) (Arciola et al., 2005). Depending on the site and type of implant and the timing of the infection, the cause of IAI will vary. For example, implants in the pelvis are more susceptible to be infected by Enterobacteriaceae, while S. aureus is still the main cause of implant surgery in other parts (Arciola et al., 2005). In addition, according to recent classification criteria, the manifestation of infections associated with orthopedic implants within 1 month after surgery is defined as early infection (Zimmerli, 2014). The virulent S. aureus is the main pathogen of this early perioperative infection and hematogenous infection. In most cases, chronic infections are caused by low-virulence bacteria such as coagulase-negative staphylococci (Trampuz and Widmer, 2006). No matter what kind of bacteria invade the implantation site, they will experience the process of adhesion and colonization on the implant surface, eventually persisting through the formation of stubborn biofilms (Masters et al., 2022). Clinically, systemic antibiotic therapy remains the mainstay of treatment (Zimmerli and Sendi, 2017). However, at the site of infection, antibiotics cannot reach effective concentrations (Noukrati et al., 2016). Biofilm formation often leads to the failure of antibiotic therapy (Wagner and Hänsch, 2017). At the same time, with the emergence of drug-resistant strains such as methicillin-resistant Staphylococcus aureus (MRSA), the treatment of IAI faces more challenges (Li and Webster, 2018).
Strategies for IAI mainly start from three aspects: 1) preventing the initial adhesion of bacteria, 2) destroying the biofilm that is just starting to form, and 3) destroying the mature biofilm. Many research studies have been devoted to improvements in antibiotic therapy, such as bone cement (Ismat et al., 2021), biopolymers (Kasza et al., 2021), ceramic materials (Cyphert et al., 2021), hydrogels (Garg et al., 2021), and nanomaterials (Keskin et al., 2021; Nag et al., 2021). They are designed as local drug delivery vehicles or coatings. This local drug delivery system successfully overcomes the problem of low blood drug concentration at the site of infection. However, there are still disadvantages, such as uneven drug release and short life cycles (Li et al., 2021). In addition, in order to solve the problem of bacterial resistance, many novel antibacterial substances, including antimicrobial peptides (Rai et al., 2022), bacteriophages (Kim et al., 2021), and nanoparticles (Nag et al., 2021), have been developed for the loading of drug delivery systems. Recently, the design of implants with antibacterial properties has begun to attract researchers’ attention. Surface modification (Narayana and Srihari, 2019; Khalid et al., 2020) and coating (Narayana and Srihari, 2019; Ahmadabadi et al., 2020) of implants are methods that have been extensively studied. These two methods are used to modulate the antibacterial properties of the implant surface. Sometimes, there are disadvantages, such as the problem of antibacterial aging. Unlike these two methods, metal alloying can achieve the overall adjustment of the implant. Antibacterial alloys can bring durable and stable antibacterial properties (Ren and Yang, 2017). At present, there are many studies on the alloying and antibacterial modification of traditional alloy materials such as stainless steel, Co-Cr alloys, and Ti alloys, and fruitful results have been achieved (Resnik et al., 2020; Guo et al., 2021a; Wang et al., 2021a; Lu et al., 2021; Watanabe et al., 2021). However, these permanently implanted alloys still suffer from unresolved drawbacks, including the risk of poisoning and allergies, stress shielding issues, and secondary surgery for implant removal (Sumner, 2015; Seyhan et al., 2018). In contrast, biodegradable alloy orthopedic implants have recently been favored by researchers due to their acceptable mechanical properties and in vivo degradability (Aghion, 2018; Yuan et al., 2022). Degradable Mg-, Zn-, and Fe-based alloy orthopedic implants with antibacterial properties have also been studied more, which will be described in detail below.
3 Research Progress of Mg-, Zn-, and Fe-Based Alloy Orthopedic Implants With Antibacterial Properties
3.1 Mg-Based Alloys With Antibacterial Properties
Biodegradable Mg-based alloys have been attracting much attention as orthopedic implants due to their similar mechanical properties to native bone and excellent biocompatibility (Razavi and Huang, 2019). Mg-based alloys can not only address the problem of stress-shielding related to Ti and Co-Cr alloys but also exhibit positive effects on bone regeneration (Cipriano et al., 2013; Zhang et al., 2017; Shahin et al., 2019). Additionally, Mg-based alloys can degrade naturally in the physiological condition to avoid secondary surgery to remove the implants. Thus, Mg-based alloys can be considered as a promising material for orthopedic implants (Razavi and Huang, 2019). Antibacterial properties of Mg have been gradually confirmed in recent years. A high PH environment due to degradation of Mg exhibits significant inhibition to bacteria (Robinson et al., 2010; Li et al., 2014b; Rahim et al., 2015). Nevertheless, the results of the antibacterial ability of Mg in vivo are not optimistic (Hou et al., 2016; Rahim et al., 2016). A reduction in antimicrobial efficacy is shown when Mg-based implants are placed in vivo because a high PH value is more likely to be buffered by body fluids (Bartsch et al., 2014; Zhao et al., 2020). To solve this issue, investigators are committed to adding bactericidal metal elements into Mg-based alloys in order to manufacture Mg-based alloy implants with excellent bactericidal properties.
3.1.1 Antibacterial Properties
Compared with Zn- and Fe-based alloys, there are more studies on the antibacterial properties of Mg-based alloys. The research on the addition of antibacterial elements Ag and Cu is dominant. At the same time, the effects of processing methods and the addition of new antibacterial elements on the antibacterial properties of alloys are also the focus of researchers. Table 1 summarizes the antibacterial properties of existing magnesium alloys.
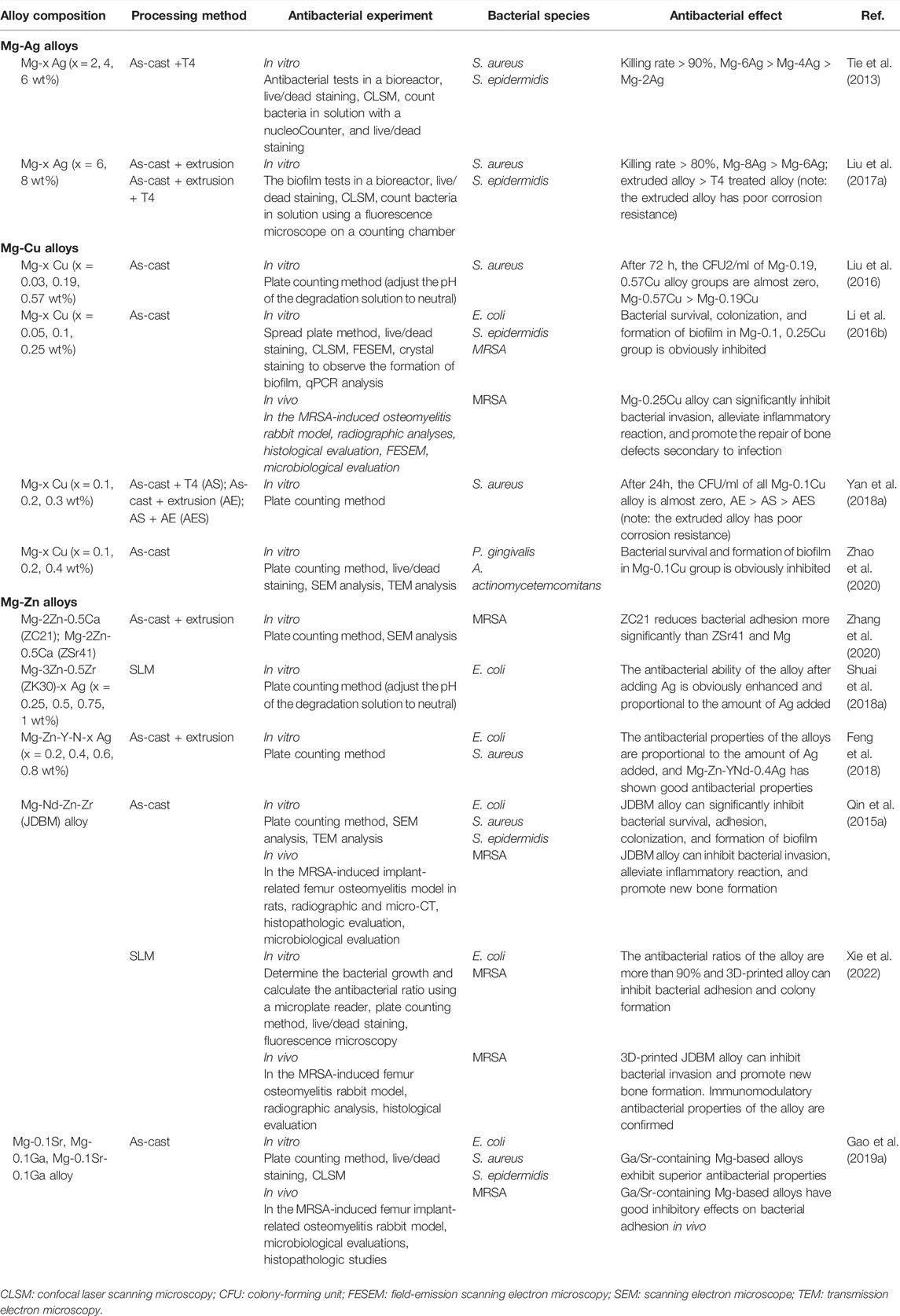
TABLE 1. Antibacterial properties of Mg-based alloys with antibacterial properties as orthopedic implants.
Mg-Ag alloys are among the first implants studied with available antibacterial activities. As is well known, silver (Ag) has resistance to many bacterial species and was used as an essential metal fungicide very early in the past (Besinis et al., 2014). Tie et al. attempted to alloy Mg with Ag element and then manufactured three kinds of solution- (T4-) treated Mg-Ag alloys with Ag mass fractions of 1.87%, 3.82, and 6.00%, respectively. In vitro experiments revealed that the killing rate of three kinds of alloys on S. aureus and S. epidermidis all exceeded 90%. With the increase in silver content, the antibacterial properties of the alloys were enhanced (Tie et al., 2013). The high silver content Mg-x Ag (x = 6, 8 wt%) prepared by Liu et al. showed strong antibacterial ability in the medium containing many bacteria. However, compared with T4-treated Mg-6Ag alloy, the inhibitory effect of T4-treated Mg-8Ag alloy on bacterial viability was slightly enhanced. In addition, the as-extruded Mg-Ag alloys had stronger antibacterial properties than the T4-treated Mg-Ag alloys (Liu et al., 2017a). Unfortunately, there is no further in vivo translational research on Mg-Ag alloys as orthopedic implants. Several recent studies have focused on the application of Ag as an antimicrobial additive for the microalloying of other magnesium alloys, which will be mentioned below.
Mg-Cu alloys are regarded as a promising candidate for orthopedic implants because of their dual antibacterial and osteogenesis properties (Jacobs et al., 2020). Copper (Cu) is an antibacterial metal that was applied to medical treatment long ago (Szymański et al., 2012; Vincent et al., 2018). Besides, as an essential trace element in human tissue, Cu exhibits reliable physiological safety (Mitra et al., 2020). More importantly, Cu is confirmed to bring a beneficial effect on promoting osteogenesis and angiogenesis potential (Wu et al., 2013; Li et al., 2016a). This dual performance has been well applied to orthopedic implants such as Cu-doped stainless steel and Ti alloys (Ren et al., 2015; Zhao et al., 2019; Moniri Javadhesari et al., 2020; Yang et al., 2021a). Therefore, it is of great attraction for researchers to incorporate Cu into pure Mg and fabricate Mg-Cu alloys. In earlier in vitro studies, Mg-Cu alloys demonstrated excellent antibacterial effectiveness. The antibacterial abilities of the Mg-x Cu (x = 0.03, 0.19, 0.57 wt%) alloys prepared by Liu et al. were significantly better than those of pure Mg. With the increase in the Cu content, the antibacterial properties of the alloys were enhanced (Liu et al., 2016). Subsequently, Li et al. developed cast Mg-Cu alloys with Cu addition of 0.05, 0.1, and 0.25 wt%, respectively, in which all demonstrated broad-spectrum antimicrobial activity against Escherichia coli (E. coli), S. epidermidis, and MRSA and remarkably resisted bacterial adhesion and biofilm formation. Mg-0.25Cu alloy, with the best antibacterial activities and biocompatibility, was applied to a rabbit tibia model with chronic osteomyelitis. The results revealed that Mg-0.25Cu alloy could significantly inhibit the invasion of bacteria and stimulate the repair of bone defects secondary to infection (Li et al., 2016b). Regrettably, although the Mg-Cu alloys show a certain application prospect in treating osteomyelitis, they are not suitable for use as a filling material for bone defects due to their rapid degradation rate. In addition, the processing technology will affect the antibacterial effect of Mg-Cu alloys. The T4-treated Mg-0.1Cu alloy showed a delayed sterilization effect after 6 h. In contrast, the as-cast Mg-0.1Cu alloy achieved a rapid and potent killing effect on S. aureus, which may be attributed to higher and faster OH− release than the T4-treated alloy (Yan et al., 2018a). In fact, the variability in Cu adding amounts and processing conditions enables Mg-Cu alloys to possess adjustable mechanical properties and degradation rates to adapt to diverse environments, thereby broadening the applications. Moreover, Mg-Cu alloys can not only play a role in the common causative agents of IAI, such as S. aureus as described above but also have a killing effect on other bacteria. In a related study, Mg–x Cu (x = 0.1, 0.2, 0.3 wt%) alloys exhibited antibacterial efficiency of up to 99.9% against Candida albicans (C. albicans) (Chen et al., 2018). Mg-Cu alloys, regarded as periodontal bone substitutes, have been used to treat periodontitis related to alveolar bone defects. It is corroborated that Mg-Cu alloys significantly decreased the survival ratios of key pathogens such as Porphyromonas gingivalis (P. gingivalis) and Aggregatibacter actinomycetemcomitans (A. actinomycetemcomitans) in periodontal diseases and peri-implantitis (Zhao et al., 2020).
Mg-Zn alloys have long been receiving substantial attention in the field of orthopedic implants because of their excellent mechanical and biomedical properties (Zhang et al., 2010; Chen et al., 2011; Seyedraoufi and Mirdamadi, 2013; Han et al., 2014; Hofstetter et al., 2015). Considered an essential element for our bodies, zinc (Zn) is safe and reliable (Zhang et al., 2021a). Moreover, Zn is verified to facilitate bone mineralization (Luo et al., 2014). However, the clinical application of Mg-Zn alloys is restricted due to rapid degradation (González et al., 2012). Several studies have put their effort into adding the third kind of alloying element for further modification of Mg-Zn alloys (Fazel Anvari-Yazdi et al., 2016; Bian et al., 2018; Prakash et al., 2018; Song et al., 2018; Miao et al., 2019). With the increasing attention to the antibacterial properties of alloys, the antimicrobial performance of some developed Mg-Zn alloys started to be explored (Zhang et al., 2020). At the same time, several novel Mg-Zn alloys with antibacterial alloying elements emerge (Shuai et al., 2018a; Feng et al., 2018). Zhang et al. evaluated the antibacterial performance of alloy pins made out of Mg-2Zn-0.5Ca (named ZC21) alloy and Mg-4Zn-1Sr (named ZSr41) alloy with excellent degradable properties and biocompatibility in vitro. It was revealed that ZC21 showed better antimicrobial activities than ZSr41 and pure Mg (Zhang et al., 2020). Excellent antibacterial performance is also shown in Zn. With this in mind, coupled with the antibacterial performance of zircon (Zr), both Mg-3Zn-0.5Zr (ZK30) (Shuai et al., 2018a) and Mg-6Zn-0.5Zr (ZK60) alloys (Shuai et al., 2018b) have been confirmed to have a certain antibacterial ability. Similarly, Qin et al. evaluated the antibacterial potency of Mg-Nd-Zn-Zr alloy (named JDBM) that had been developed before. The results confirmed that JDBM showed strong bacteriostatic activity against E. coli, S. epidermidis, and S. aureus. Moreover, JDBM appears to be a potential antibacterial orthopedic implant because of its capability of preventing infection and promoting the formation of new bones in rat models (Qin et al., 2015a). Recently, Xie et al. prepared 3D-printing JDBM implants with porous structure using selective laser melting (SLM) technology (Xie et al., 2022). The antibacterial rates of JDBM implants against S. aureus and E. coli reached 90.0% and 92.1%, respectively. Moreover, 3D-printed JDBM implants performed excellently in the rabbit femoral osteomyelitis model (Xie et al., 2022). The first attempt to apply 3D-printing technology to Mg-based alloys exhibits its potential in the field of Mg-based alloy orthopedic implants with antibacterial properties.
Adding Ag or Cu elements to the existing Mg-based alloys with superior properties has also been shown to impart or improve the antibacterial properties of the alloys. This antibacterial effect is also proportional to the amount of Ag or Cu elements added (Shuai et al., 2018a; Shuai et al., 2018b; Feng et al., 2018; Bakhsheshi-Rad et al., 2019). For example, Dai et al.’s study, which added 1 wt% Ag to Mg-4Y alloy, made the alloy’s antibacterial rate against S. aureus reach 92.93% (Dai et al., 2018). In Feng et al.’s, the Mg-Zn-Y-Nd-x Ag (x = 0.2, 0.4, 0.8 wt%) alloys exhibited broad-spectrum antibacterial properties against S. aureus and E. coli. The alloy already showed strong antibacterial efficacy when the Ag content reached 0.4 wt% (Feng et al., 2018). Although the ZK30 alloy already has some antibacterial properties, the addition of 0.25 to 1 wt% Ag or 0.1 to 0.3 wt% Cu will significantly improve its antibacterial ability (Shuai et al., 2018a). The addition of Cu to the ZK60 alloy was also confirmed to significantly improve the antibacterial properties of the alloy. The extracts of ZK60-0.8Cu alloy eliminated bacterial colonies within 48 h, while the extracts of ZK60-0.2Cu alloy needed 96 to achieve this effect (Shuai et al., 2018b).
The addition of electrochemically inert elements such as Ag and Cu into Mg-based alloys triggers galvanic corrosion and accelerates degradation, harming the biocompatibility and life span of Mg-based alloy implants. With this in mind, researchers tried to find new alloying elements with antibacterial properties to fabricate Mg-based alloy implants with superior corrosion resistance, antibacterial properties, and osteogenic capabilities. Along this line, Mg-based alloys containing trace content of Ga/Sr (0.1 wt%) have been developed (Gao et al., 2019a). Adding Ga/Sr shows an improvement in corrosion resistance of Mg-based alloys and displays broad-spectrum antibacterial activity against S. aureus, S. epidermidis, and E. coli. In addition, Mg-based alloys with Ga/Sr effectively inhibited bacterial infections in the mouse femoral osteomyelitis model (Gao et al., 2019a). The findings may shed new light on the development of antibacterial orthopedic implants. We might also shift the focus to novel, high-quality alloying elements with antibacterial properties and even osteogenic ability.
Overall, most studies on Mg-based alloy orthopedic implants with antibacterial properties are focused on classical antibacterial elements Ag and Cu. The influence of their addition on the antibacterial properties of alloys is still a subject of concern. Mg-Cu alloys are supposed to be potential orthopedic implants with double antibacterial and osteogenic effects. At the same time, it seems quite promising to further alloy Mg-Zn alloys to develop multi-element antibacterial Mg-based alloys. It is supposed to be a good idea to apply novel, high-quality alloying elements with antibacterial properties and even osteogenic ability to Mg-based alloys. Additionally, the processing technology also affects the antibacterial properties of Mg-based alloys. The application of new fabrication processes, such as 3D printing, also has expectable perspectives. However, it should be noted that the balance between antibacterial properties, mechanical properties, corrosion resistance, and biocompatibility of alloys is always an issue to be properly addressed, regardless of which way of thinking we choose.
3.1.2 Mechanical Properties
During the development of antibacterial Mg-based alloys, the alloying of metal elements can not only improve their antibacterial properties but also show significant influences on their mechanical properties. Considering the “stress shielding” problem, a discussion on the mechanical properties of Mg-based alloys is warranted. The mechanical parameters of existing antimicrobial Mg-based alloys are summarized in Table 2.
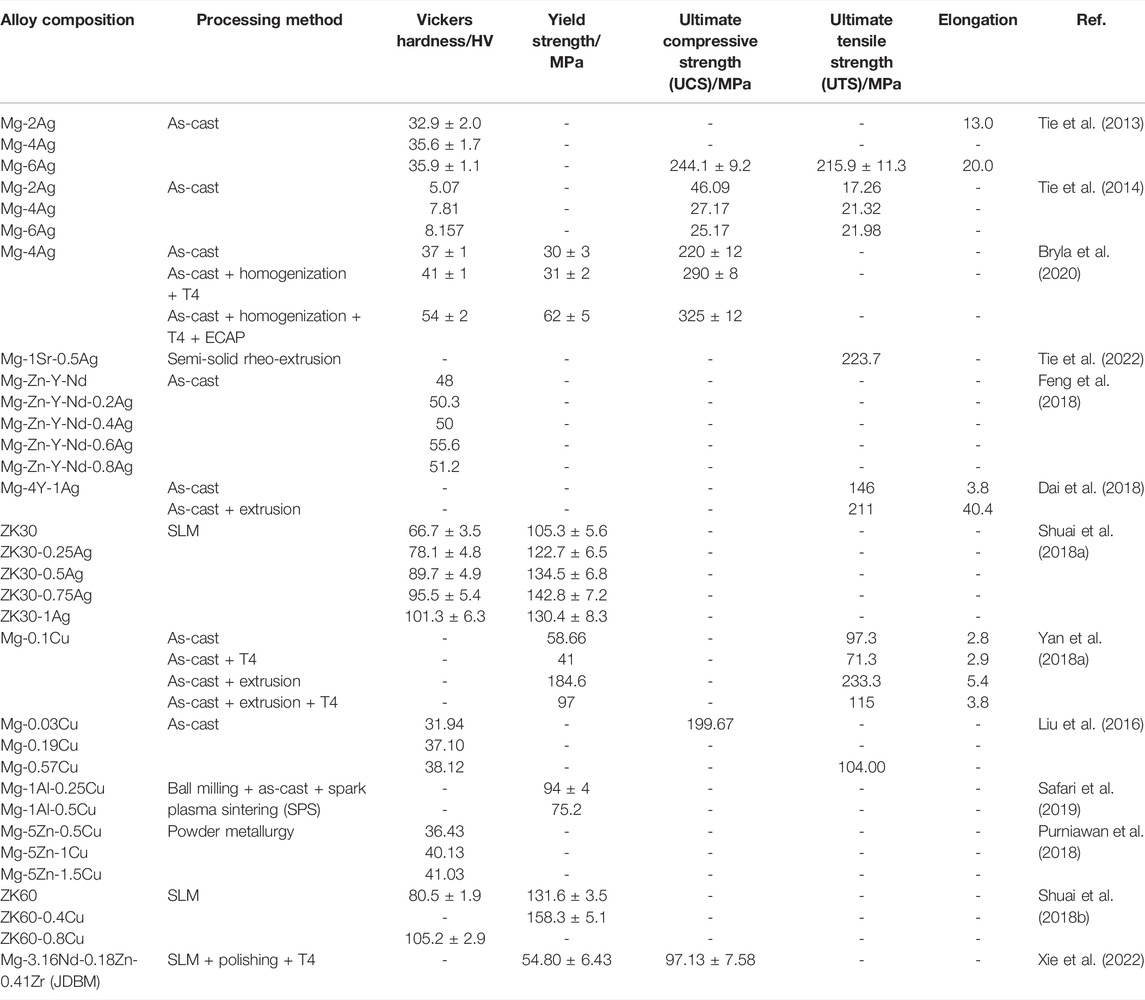
TABLE 2. Mechanical properties of Mg-based alloys with antibacterial properties as orthopedic implants.
The addition of the Ag element exhibits a significant improvement in the mechanical properties of Mg-based alloys through grain refinement strengthening and precipitation strengthening (Tie et al., 2013; Shuai et al., 2018a; Feng et al., 2018). Ag-containing Mg binary alloys, whatever processing pathway, will result in a decrease in the average grain size of alloys as Ag content increases (Tie et al., 2013; Tie et al., 2014; Liu et al., 2017a). According to the Hall–Petch relationship, grain refinement brought by Ag enables alloys with better mechanical properties (Shuai et al., 2018a). Tie et al. confirmed this by investigating the mechanical properties of as-cast Mg2Ag, Mg4Ag, and Mg6Ag alloys (Tie et al., 2013; Tie et al., 2014). Based on such properties of Ag, several studies attempted to add a trace amount of Ag element to other Mg alloys to improve the mechanical and antimicrobial properties. In Feng et al.’s study, Mg-Zn-Y-Nd-xAg alloys (x = 0.2, 0.4, 0.6, 0.8 wt%) presented an increase in micro-hardness as Ag content increased (Feng et al., 2018) because of the addition of Ag, which leading to the grain refinement in alloys, an increase in the volume fraction of alloys in second phase, and a more scattered distribution pattern in Mg matrix (Shuai et al., 2018a; Feng et al., 2018). Nevertheless, it should be noted that when Ag is added to reach 1wt% in the Mg-3Zn-0.5Zr (ZK30) alloy, the alloy is likely to show lower compressive yield strength (CYS) due to a rougher precipitate phase and a lower binding strength on the interface between Mg matrix and precipitate phase (Shuai et al., 2018a).
Similarly, adding Cu is confirmed to have a favorable effect on the mechanical properties of Mg-based alloys. The hardness of Cu-containing Mg-based alloys significantly increases as Cu content rises. Shuai et al. established that the incorporation of Cu enabled the hardness of alloys to grow from 80.5 ± 1.9 HV of ZK60 alloy to 105.2 ± 2.9 HV of ZK60-0.8Cu alloy (Shuai et al., 2018b). Similar to the Ag element, the addition of Cu promotes the grain refinement of alloys and the formation of intermetallic phases with a higher stiffness than the Mg matrix (Shuai et al., 2018b; Xu et al., 2019). Grain refinement and uniformly distributed intermetallic phases bring high compressive strength. The compressive strength of ZK60 alloy increases to 158.3 ± 5.1 MPa after adding 0.4 wt% Cu (Shuai et al., 2018b). Moreover, due to the pinning effects by intermetallic phases along grain boundaries, more addition of Cu leads to an increase in tensile strength of Mg alloy. The ultimate tensile strength (UTS) of Mg-0.57Cu alloy is nearly twofold higher than that of pure Mg (Liu et al., 2016). It is important to remark that low supplement with Cu may not function apparently to the grain refinement of Mg-based alloys due to the low growth restriction factor value of Cu. This is corroborated in the investigation of Mg-xCu (x = 0.1, 0.2, 0.3 wt%) alloys by Yan et al., (2018a) and Chen et al., (2018). In this case, the slight improvement in the hardness of alloys by adding Cu is achieved mainly through increasing intermetallic phases (Xu et al., 2019). Nevertheless, adding too much Cu attenuates the improvement of mechanical properties. Besides the number of intermetallic phases, their size and distribution also affect the mechanical behavior (Golafshan et al., 2017). In the study of Mg-1Al-xCu alloys, Mg–1Al-0.25Cu with more uniformly distributed Al2Cu grains doubled the compressive and yield strength compared to Mg–1Al-0.5 Cu alloy (Safari et al., 2019). Shuai et al. also confirmed that as Cu content reached 0.6 and 0.8 wt%, excessive MgZnCu phase in ZK60-xCu alloy interconnected and formed networks along grain boundaries. This contributes to the disruption of continuity in the Mg matrix. During deformation, stress builds up at the junction of the intermetallic phase and Mg matrix, leading to a reduction in compressive strength (Shuai et al., 2018b).
The procedure of processing also affects the mechanical behavior of Mg-based alloys. It has been previously reported that solution (T4) treatment can dissolve the intermetallic phases in as-cast alloys, causing the hardness of alloys to decrease slightly (Tie et al., 2013; Yan et al., 2018a). Bryla et al. also confirmed that the high-temperature condition increased the Ag solubility in Mg during the T4 treatment, leading to the dissolution of dendritic structures in Mg-Ag alloys. However, they discovered that the solid solution strengthened elevated stiffness, compression strength, and CSF of as-cast Mg-Ag alloy after the homogeneous treatment process (Bryla et al., 2020). Extrusion treatment improves the hardness of alloys by structural refinement. During extrusion, high-temperature and high-pressure change coarse dendrites into equiaxed grains in alloys and lead to the dissolution or conversion into equiaxed grains of the second phase (Dai et al., 2018; Feng et al., 2018). More refined grains decrease stress concentration. Meanwhile, the increase in grain boundary after structural refinement impedes crack propagation, which remarkably improves the extensibility of Mg-based alloys (Yan et al., 2018a). Dai et al. showed that compared to as-cast Mg-4Y-1Ag alloy, yield stress, UTS, and elongation of extruded Mg-4Y-1Ag alloy all get improved (Dai et al., 2018). Equal-channel angular pressing (ECAP) is also an effective means of grain refinement in alloys. Bryla et al. stated that Mg–4% Ag alloy was subjected to twice ECAP treatment, and its average grain size decreased from 350 to 15 μm. The refinement significantly improves the hardness, CYS, and UCS of alloy (Bryla et al., 2020). In contrast, T6 aging treatment has a limited role in improving mechanical properties, although aging strengthening of Mg-based alloys can be realized as the precipitated phase is re-precipitated. For instance, the hardness of Mg–6% Ag after T6 treatment slightly increases from 36 HV5 to 43 HV5, while even a slight decline occurs in UCS (Tie et al., 2013).
3.1.3 Corrosion Resistance
The corrosion resistance, biocompatibility, and antibacterial properties of Mg-based alloys are intimately interlinked. Mg-based alloys degrade with a concomitant elevation of pH value, osmotic pressure, and release of other metal elements. Sometimes, due to concerns about antimicrobial properties, a higher pH value and more release of ions are expected. Nonetheless, the non-negligible thing is that hyperosmolarity and excessive released ions brought by rapid degradation may result in cellular toxicity. To meet the demand for biocompatibility, the corrosion resistance of Mg-based alloys requires improvement to tightly control the degradation rate. Table 3 lists a summary of the corrosion-resistant performance of antimicrobial Mg-based alloys with various compositions and procedures of processing.
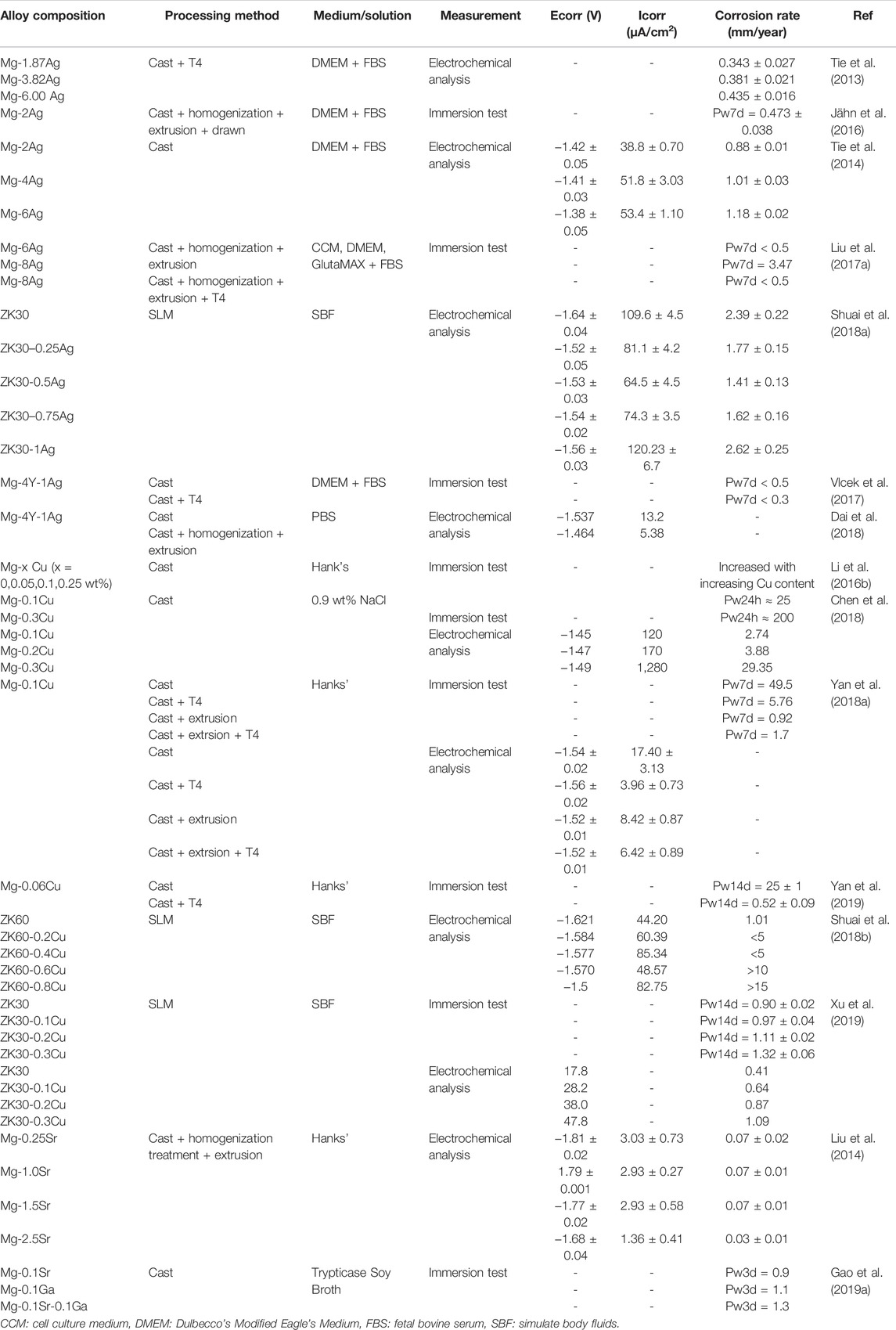
TABLE 3. Corrosion resistance of Mg-based alloys with antibacterial properties as orthopedic implants.
The addition of Ag and Cu elements increases the antimicrobial behavior, with a concomitant decrease in corrosion resistance. In Ag-containing binary Mg alloys, the occurrence of corrosion is more prone to exist as Ag content increases. Tie et al. found that Mg-x Ag (x = 2, 4, 6 wt%) alloys degraded more rapidly with the increase in Ag content, whether or not hot treatment was performed (Tie et al., 2013). Liu Z et al. suggested that the degradation rate of alloy reached 3.47 mm/year as the concentration of Ag increased up to 8.51 wt%, compared with that of pure Mg (0.5 mm/year) (Liu et al., 2017a). Cu-containing binary Mg alloys also demonstrate the same tendency (Li et al., 2016b; Liu et al., 2016; Yan et al., 2018a; Chen et al., 2018). For example, the corrosion rate of Mg-0.1Cu alloy manufactured by Li et al. is double that of pure Mg. Besides, the corrosion rate increases from 25 mm/y to nearly 200 mm/y, with Cu content growing from 0.1 to 0.3 wt% (Li et al., 2016b). The dominant reason for this phenomenon lies in the solubility of Ag and Cu in Mg. More secondary phases precipitate in alloy with increasing the Ag or Cu content (Tie et al., 2013; Li et al., 2016b; Liu et al., 2016; Liu et al., 2017a). There is a variation in electrochemical potentials between inert Mg-Ag or Mg-Cu particles and Mg matrix in these alloys (Li et al., 2016b; Bryla et al., 2020). Bulk secondary phases act as highly active micro-cathodes, coupled with α-Mg anodes, thus forming numerous micro corrosion cells leading to the accelerated corrosion of the Mg matrix (Li et al., 2016b; Liu et al., 2017a; Bryla et al., 2020). Furthermore, pitting corrosion occurs due to the different corrosion rates between the α-Mg phase and secondary phase, resulting in accelerating the non-uniform degradation process of alloy. In contrast, a slight addition of Ag or Cu element in other Mg-based alloys results in different outcomes. In the study of Shuai et al., the corrosion rate of ZK30-xAg (x = 0, 0.25, 0.5, 0.75, 1 wt%) alloy increased initially and then diminished with increased Ag content. When the Ag content reached 1 wt%, the corrosion resistance of alloy tended to be adversely affected (Shuai et al., 2018a). Zhang et al. stated that the modified Mg-based alloys were obtained by adding Ag into Mg-3.0Nd-0.2Zn-0.4Zr (named JDBM) alloys. The degradation rate of the alloys substantially accelerated with 0.4 wt% loading of the Ag content (Zhang et al., 2013). Similar results were obtained when adding the Cu element into Mg-based alloys. The corrosion resistance improves as the Mg-1Al alloy is modified by adding 0.025 wt%Cu. In contrast, the degradation rate is significantly accelerated as the Cu content reaches 0.1 wt% (Safari et al., 2019) because, on the one hand, the addition of Cu and Ag elements results in structural refinement and increases the density of grain boundaries. Fine-grain size is favorable for the formation of dense corrosion product film, while the high-density distribution of grain boundaries acts as a corrosion barrier to suppress the corrosion process (Zhang et al., 2013; Shuai et al., 2018a; Shuai et al., 2018b). On the other hand, when the addition of Ag or Cu elements reaches a certain content, more precipitate phases occur, and alloys are more likely to degrade rapidly because of the exacerbation of galvanic and pitting corrosion (Zhang et al., 2013; Shuai et al., 2018b; Feng et al., 2018; Safari et al., 2019).
As a commonly used alloying element in Mg-based alloys, Zn is corroborated to elevate corrosion resistance significantly through effective grain refinement in antibacterial Mg-based alloys (He et al., 2015; Zhang et al., 2020). Mg-2Zn-0.5Ca (named ZC21) alloys and Mg-4Zn-1Sr (named ZSr41) alloys present excellent corrosion resistance that outperform pure Mg (Zhang et al., 2020). Because of adding Zn, Nn, and Zr, the corrosion rate of JDBM alloys was even reduced fivefold compared with pure Mg (Qin et al., 2015a). After Qin et al. added 2∼4 wt% Zn into the Mg-1Ca-0.5Sr alloy, the corrosion resistance of the alloy got improved. More uniform corrosion appeared on the surface of Zn-containing alloys, with no apparent corrosion pits. Nevertheless, Mg-1Ca-0.5Sr-6Zn alloy implied a great hydrogen evolution rate (He et al., 2015). This is possibly explained by the reduction in Zn solubility in the Mg matrix due to the appearance of other alloying elements. Excessive Zn promotes the precipitation of Zn-containing intermetallic phases, thereby accelerating galvanic erosion (He et al., 2015). Moreover, the improvement of corrosion resistance in Mg-based alloys can also be observed with the addition of biocompatible elements such as Sr and Ga. However, due to the low solubility of Sr in the Mg matrix, the corrosion resistance of the Mg-Sr alloy declined due to more precipitation phases when the Sr content reached over 1wt% (Liu et al., 2014). Thus, Gao et al. added 0.1 wt% Sr and/or Ga for microalloying treatment on Mg-based alloys. The obtained Mg-0.1Sr, Mg-0.1Ga, and Mg-0.1Sr-0.1Ga showed much slower corrosion rates than pure Mg (Gao et al., 2019a).
Meanwhile, the processing procedure has a great impact on corrosion resistance. T4 treatment effectively promotes the performance of corrosion resistance. Yan et al. stated that the corrosion rate of the Mg-0.1Cu alloy (0.92 mm/y) via solution processing was reduced over 50-fold compared with that of the as-cast Mg-0.1Cu alloy (49.5 mm/y) (Yan et al., 2018a). T4 treatment can dissolve most of the secondary dendrites or precipitate phase, causing the surface corrosion potential to distribute more evenly and a significant reduction in the number of micro-galvanic cells (Liu et al., 2017a; Vlcek et al., 2017; Yan et al., 2018a; Feng et al., 2018; Yan et al., 2019). Meanwhile, the dissolution of precipitated particles and homogenization of solute bring a more homogenous, compact, and flat degradation surface and a lower trend toward pitting corrosion (Tie et al., 2013; Liu et al., 2017a). It is not difficult to understand that the Mg alloys exhibit more excellent corrosion resistance and degradation behavior after T4 treatment. Hot extrusion can also improve the corrosion resistance of alloys for refining grain structures and distributing intermetallic phases evenly (Yan et al., 2018a). However, there are still many intermetallic phases in alloys after extrusion. The intermetallic phases spreading along crush-bands and Mg matrix form a large cathode-to-anode area, leading to severe corrosion. Thus, the improvement of extrusion on corrosion resistance of Mg-based alloys is not obvious (Yan et al., 2018a; Yan et al., 2018b).
There is another issue that cannot be overlooked for the corrosion resistance of Mg-based alloys. Many studies have established that a clear difference is observed between in vivo and in vitro degradation of Mg-based alloys (Kumar and Katyal, 2021). Multiple factors in the in vivo environment may impact the degradation of Mg-based alloys. The studies on in vivo degradation in terms of antibacterial Mg-based alloys is of great importance (Kumar and Katyal, 2022). Jahn et al. studied the intramedullary Mg2Ag nails, finding it took 617 days for in vitro degradation, while only 210 days for the in vivo test. Although there is a certain difference in degradation between in vivo and in vitro, the in vivo degradation rate of Mg2Ag nails was still acceptable (Jähn et al., 2016). The shape of the ZC21 alloy was still largely maintained after 12 weeks of implantation on mouse femurs (Zhang et al., 2020). The degradation rate of JDBM in rat femur was merely 0.092 mm/y (Qin et al., 2015a). The studies on in vivo degradation of antibacterial Mg-based alloys are so far limited, and additional research is needed.
3.1.4 Biocompatibility
As discussed above, the addition of alloying elements indeed improved the antimicrobial properties of Mg-based alloys. Nevertheless, the issue of cytotoxic effects caused by changing the pH values and releasing the metal ions has always been a concern. It is reported that most antibacterial Mg alloys present good in vitro biocompatibility. Table 4 summarizes the results of studies on in vitro biocompatibility of various antibacterial Mg-based alloys.
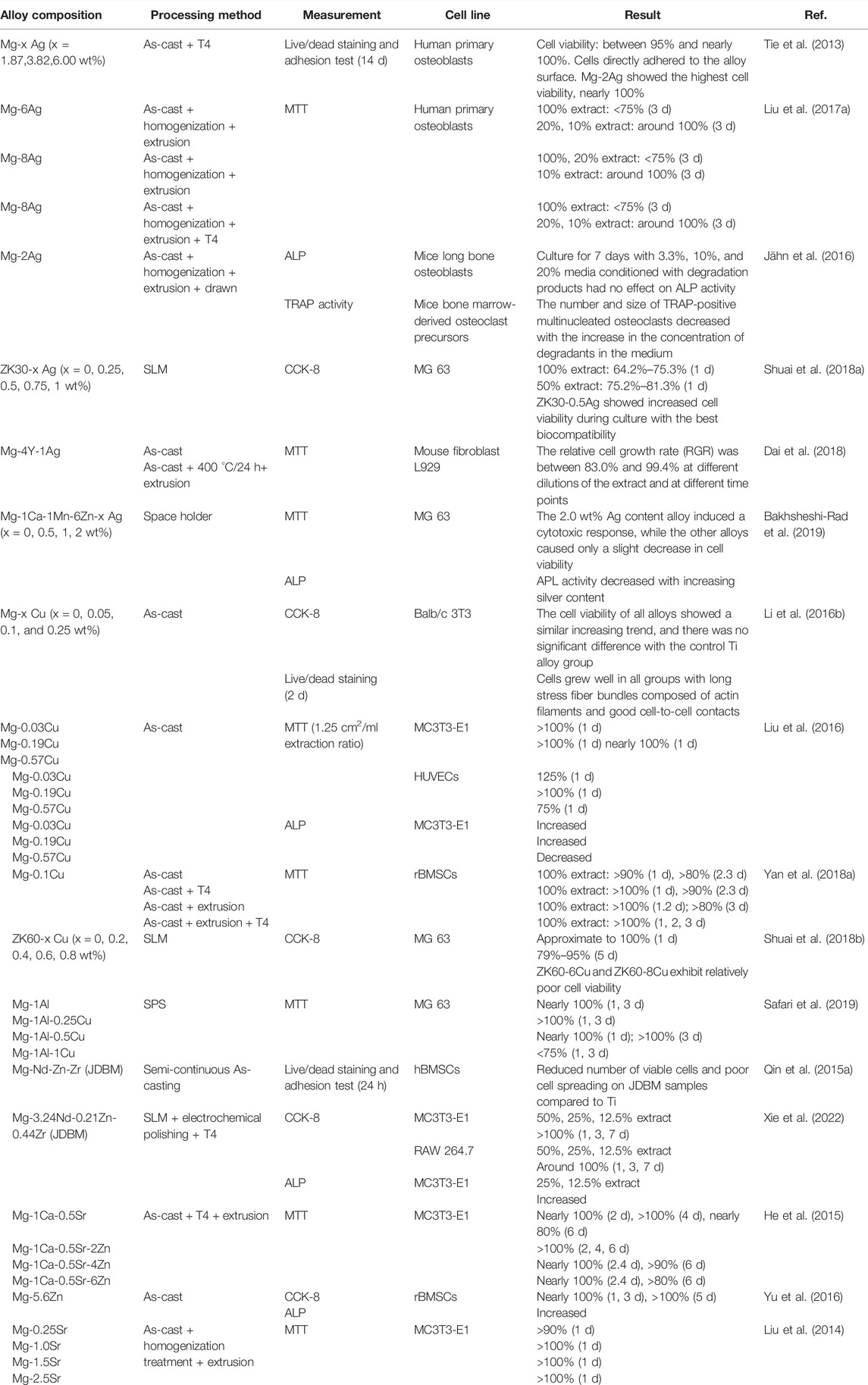
TABLE 4. In vitro biocompatibility of Mg-based alloys with antibacterial properties as orthopedic implants.
Adding moderate Ag and Cu content does not seem to have influenced the biocompatibility of alloys. Antimicrobial Mg alloys containing Ag or Cu show no cytotoxic effects on human primary osteoblasts (Tie et al., 2013; Liu et al., 2017a), mice long bone osteoblasts (Jähn et al., 2016), MG 63 cells (Shuai et al., 2018a; Shuai et al., 2018b; Bakhsheshi-Rad et al., 2019; Safari et al., 2019), mouse fibroblast L929 cells (Dai et al., 2018), Balb/c 3T3 cells (Li et al., 2016b), MC3T3-E1 cells (Liu et al., 2016), HUVECs (Liu et al., 2016), and rBMSCs (Yan et al., 2018a). The addition of low Cu content even induces the osteogenic differentiation of osteogenic precursor cells, mineralization of extracellular matrix, and collagen secretion (Liu et al., 2016). Cu in low concentration is also conducive to enhancing the activity, proliferation, migration, and angiogenesis-related markers expression of HUVECs (Liu et al., 2016). Mg-Cu alloys under T4 treatment also present good biocompatibility because of the optimized performance of corrosion resistance (Yan et al., 2018a). Nonetheless, continued attention is required that excessive addition of Ag and Cu may adversely affect the survival, proliferation, and adhesion of cells, especially for Cu-containing Mg alloys, as confirmed by several studies (Liu et al., 2016; Shuai et al., 2018a; Yan et al., 2018a; Shuai et al., 2018b; Bakhsheshi-Rad et al., 2019; Safari et al., 2019). It is essential to consider biocompatibility, antibacterial properties, mechanical behavior, and corrosion resistance when probing the optimal addition amount of Ag and Cu in different Mg-based alloys.
The addition of biocompatible elements, such as Zn, Ca, and Sr, has been demonstrated to enhance the biocompatibility of antimicrobial Mg-based alloys. Compared with Mg-1Ca-0.5Sr, alloys with 2∼6 wt%Zn exhibit higher biocompatibility (He et al., 2015). Zhang C et al. pinyed out that because of the addition of Zn and Ca, the ZC21 alloy presented a stronger stimulatory effect on the adhesion and proliferation of BMSCs than the Ti alloy (Zhang et al., 2020). Adding Zn to Mg-Zn binary alloys is also confirmed to promote osteogenic differences in rBMSC and extracellular matrix calcium deposition (Yu et al., 2016). Furthermore, the Sr element is confirmed to contribute positively to the survival rate of hMSCs, which may counterbalance the potential adverse effects of over-releasing Mg ions (Gao et al., 2019a). Mg-Sr alloys exhibit an obvious positive promotion in the survival, proliferation, adhesion, and spreading of MC3T3-E1cells (Liu et al., 2014).
There are few studies on the in vivo biocompatibility of antimicrobial Mg-based alloys. Table 5 summarizes the results of studies on in vivo biocompatibility of several antibacterial Mg-based alloys. Additional in vivo researches are indispensable for better clinical translation.
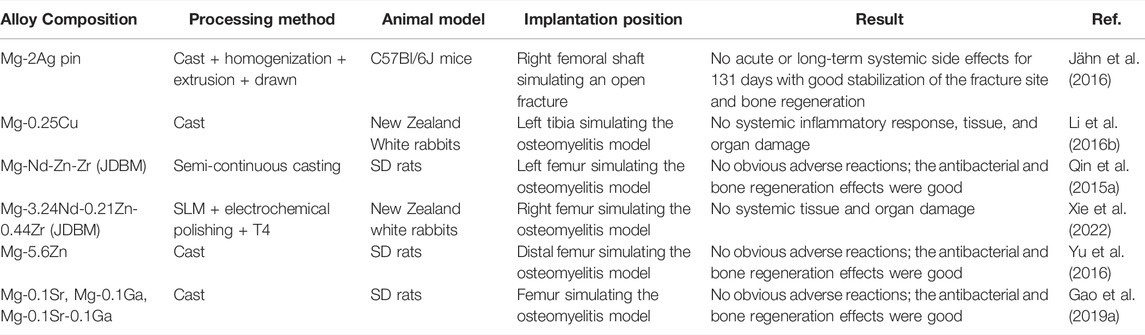
TABLE 5. In vivo biocompatibility of Mg-based alloys with antibacterial properties as orthopedic implants.
3.2 Fe-Based Alloys With Antibacterial Properties
Fe-based alloys are highly valuable in the field of orthopedic implants because of their excellent biocompatibility, degradability, and mechanical properties (Gorejová et al., 2019). Higher mechanical strength of Fe, compared with Mg and Zn, is essential for orthopedic implants that require shearing enough stress and loads (Heiden and Walker, 2015). Nevertheless, unlike Mg-based alloys that degrade rapidly, the slow degradation reactivity of Fe alloys restricts its clinical application (Chen et al., 2020). The degradation rate can be improved by adding alloying elements that form galvanic corrosion (Schinhammer et al., 2010; Liu and Zheng, 2011; Heiden and Walker, 2015). This is also required for the preparation of antibacterial Fe-based alloys. Unfortunately, there are relatively few studies on Fe-based alloys with antibacterial properties.
3.2.1 Antibacterial Properties
Some existing studies on Fe-based alloys with antibacterial properties mainly focus on the addition of the antibacterial elements, such as Cu and Ag. Table 6 summarizes the antibacterial properties of existing Fe-based alloys.
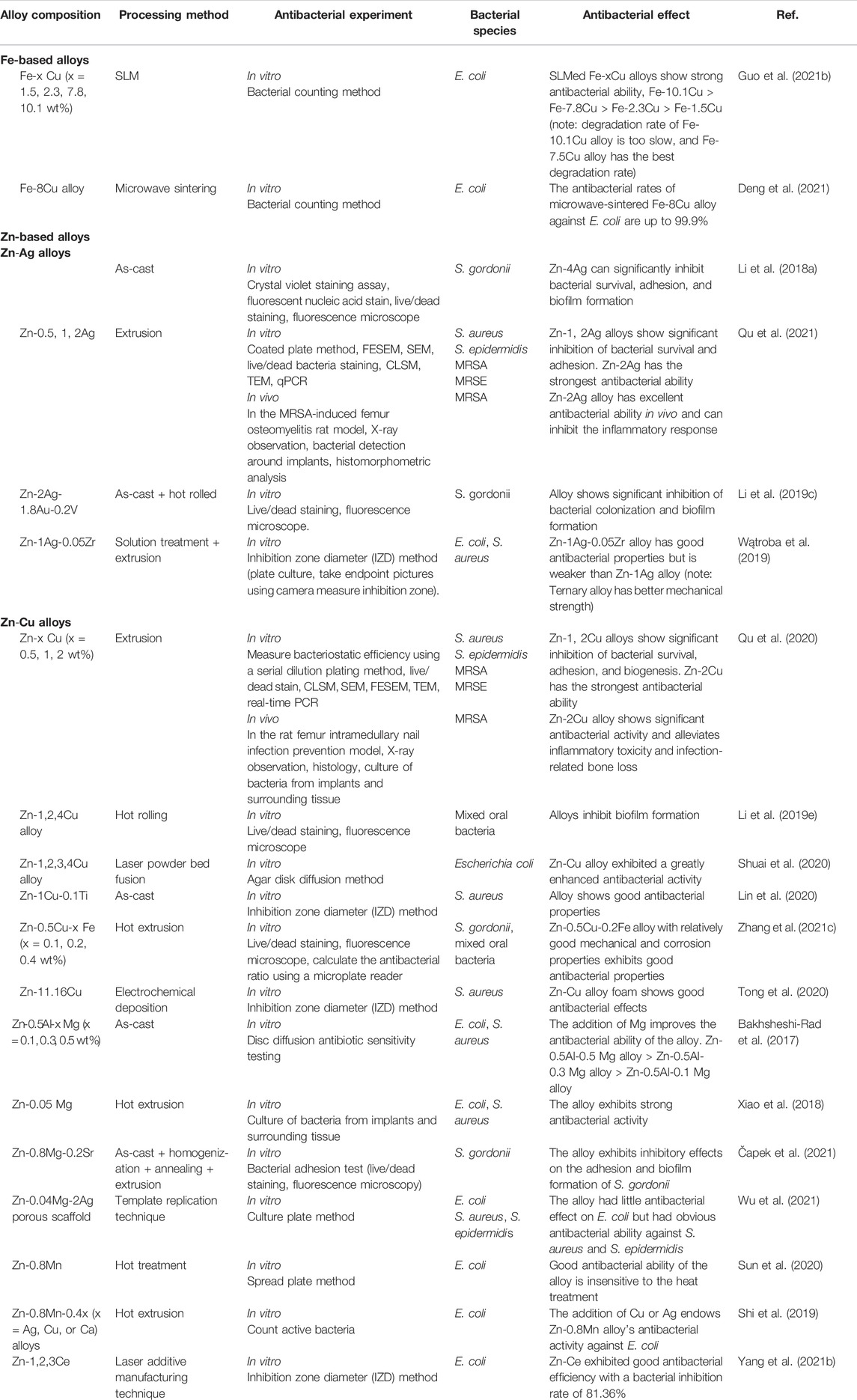
TABLE 6. Antibacterial properties of Fe- and Zn-based alloys with antibacterial properties as orthopedic implants.
The addition of Cu element was confirmed to impart antibacterial properties to Fe-based alloys. Guo et al. suggested that Fe-x Cu (x = 0, 1.5, 2.3, 7.8, and 10.1 wt%) alloys prepared by SLM exhibited superior antibacterial properties. The antibacterial ability of the alloys was enhanced with the increase in the Cu content. The antibacterial rate of the SLMed Fe-1.5 Cu alloy against E. coli was about 96.5% (Guo et al., 2021b). The antibacterial rates of other high-content Fe-Cu alloys were all greater than 99.9%. Deng et al. also confirmed the antibacterial efficacy of Cu-containing Fe-based alloys. They used microwave sintering to prepare porous Fe-8Cu alloy with an antibacterial rate of 99.9% against E. coli (Deng et al., 2021). In addition, the excellent antibacterial properties of Cu-containing Fe-Mn alloys are unanimously affirmed. Although Fe-Mn alloys have a certain antibacterial effect or promote bacterial growth, there is some controversy (Sotoudehbagha et al., 2018; Mandal et al., 2019; Mandal et al., 2021). Mandal et al. stated that the Fe-Mn-0.9Cu alloy did not have antibacterial properties. However, when the Cu addition amount was further increased (5 and 10 wt%), the Fe-Mn-Cu alloy exhibited obvious bacterial growth inhibition with the increase of Cu content (Mandal et al., 2021). Similarly, Fe-(35-x) Mn-x Cu (x = 0, 1, 3, 5, 10 wt%) also showed an enhanced bactericidal effect on E. coli with the increase in copper content (Mandal et al., 2019).
However, little research has been done on Ag-doped Fe-based alloys. Sotoudehbagha et al. confirmed that when 1 wt% Ag was added to the Fe-30Mn alloy, the antibacterial rate of the alloy against E. coli and S. aureus rose to 77% and 90%, respectively. When the silver content reached 3wt%, the antibacterial rate of the alloy against E. coli and S. aureus could reach 99%.
In conclusion, although the studies on the antibacterial properties of Fe-based alloys are relatively scarce, the existing results are promising. Fe-based alloys with antibacterial properties deserve in-depth research.
3.2.2 Mechanical Properties
The addition of Ag and Cu elements in the antibacterial Fe-based alloys not only gives the Fe-based alloys antibacterial properties but also improves the mechanical properties of the alloys. Table 7 summarizes the mechanical properties of antibacterial Fe-based alloys containing Cu or Ag and highlights the effects of the amount of Ag or Cu added on the mechanical properties of antibacterial Fe-base alloys.
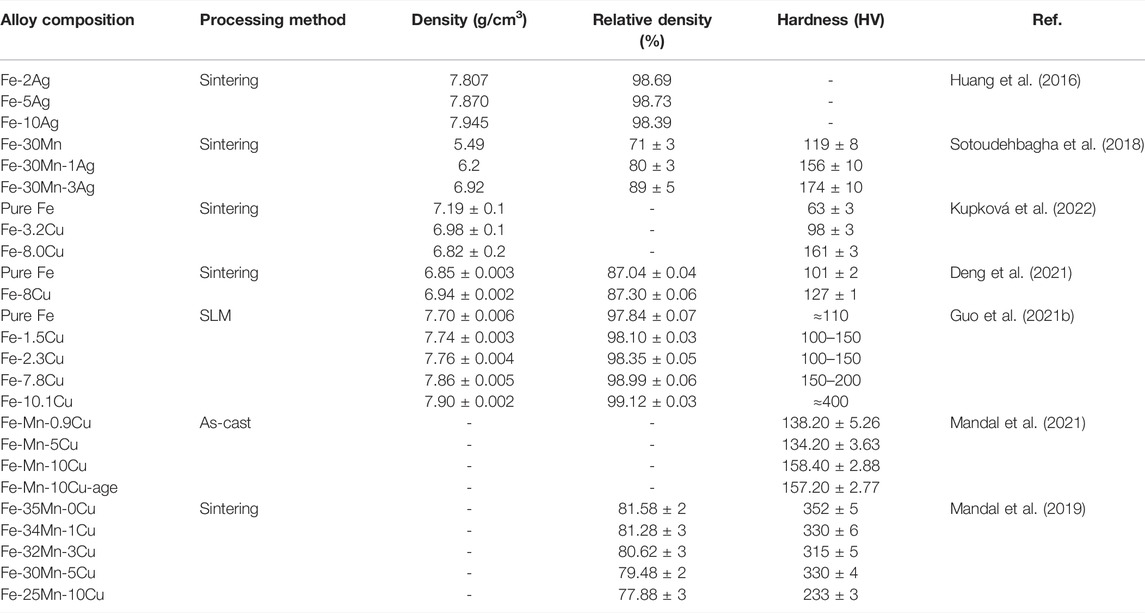
TABLE 7. Mechanical properties of Fe-based alloys with antibacterial properties as orthopedic implants.
The improvement of the mechanical properties of pure iron by Ag and Cu elements is mainly attributed to their solid solution strengthening and precipitation strengthening ability. After the addition of Ag and Cu, the iron matrix will form a Cu-rich or Ag-rich second phase due to precipitation (Zhang et al., 2016; Sotoudehbagha et al., 2018; Zhang et al., 2021b; Mandal et al., 2021). These second phases are distributed along the grain boundaries, effectively fill the structural gaps, thereby increasing the overall density and hardness of the alloys (Cao et al., 2006). For instance, adding Ag to the Fe-30Mn alloy enabled the hardness of the alloy to increase from 119 HV of Fe-30Mn to 174 HV of Fe-30Mn-3Ag. At the same time, Fe-30Mn-3Ag also showed three times the shear strength, suggesting that densification and grain refinement can also improve the shear strength of the alloy (Sotoudehbagha et al., 2018). However, it is important to note that the strength of Ag is lower than that of Fe. When the Ag content is too high, the strength of Fe-Ag alloys will decrease (Cao et al., 2006; Huang et al., 2016). Compared with pure iron, only Fe-5Ag alloy in the Fe-x Ag (x = 2, 5, 10 wt%) alloys exhibited better mechanical properties (Huang et al., 2016). As for Cu-containing Fe-based alloys, the changing trend of mechanical properties is similar to Ag-containing Fe-based alloys. Deng et al. stated that the hardness of microwave sintered Fe-8Cu (∼127 HV) was slightly improved compared to the hardness of pure Fe (about 101 HV) (Deng et al., 2021). Guo et al. found out that the Fe-x Cu (x = 0, 1.5, 2.3, 7.8, 10.1 wt%) alloy prepared by SLM exhibited a gradually increasing hardness with the increase in Cu content. What is more, the hardness of the SLMed Fe-10.1Cu alloy increased sharply to 400 HV (Guo et al., 2021b). Similar results were obtained by Mandal et al., suggesting that the addition of 0.9 and 5 wt%Cu did not achieve a significant improvement in the hardness of the alloy. However, when the Cu addition reached 10 wt%, the hardness of the alloy increased significantly (Mandal et al., 2021). The changes in the mechanical properties of the Fe-Mn alloys with the addition of Cu are slightly more complicated. In the study of Mandal et al., as the added amount of Cu increased to 3 wt%, the hardness of Fe-Mn-Cu alloy did not increase but decreased. When the added amount of copper reached 5 wt%, the hardness of the alloy increased. However, when the added amount of Cu reached 10 wt%, the hardness of the alloy decreased again (Mandal et al., 2019). They believed that the decrease in the hardness of the alloy was due to the increase in the accumulation of failure energy (SFE) when a small amount of Cu was added. With the increase in the Cu content, the solid solution strengthening and precipitation strengthening effect of Cu on the alloy overcame the SFE effect and increased the hardness of the alloy (Mandal et al., 2019).
The metal preparation and metal forming processes also have a great influence on the mechanical properties of the alloy. Fe-Cu alloys produced by SLM have high mechanical strength due to their distinctly refined grain structure. However, the microstructures of the iron matrix of all SLMed Fe-xCu (x = 0, 1.5, 2.3, 7.8, 10.1 wt%) alloys are quite compact without any obvious pores (Guo et al., 2021b). In contrast, the Fe-Cu binary alloys produced by sintering have a porous structure closer to the natural bone tissue. With the increase in the Cu content, the size of the alloy pores increases. Although the strength of the alloy is partially lost due to the presence of pores, sintered Fe-Cu alloys can still show acceptably enhanced hardness with the increase in Cu due to the counteracting effect of precipitation hardening (Kupková et al., 2022).
3.2.3 Corrosion Resistance
As mentioned above, the corrosion rate of pure iron is very low and is not suitable for orthopedic implant applications. The alloying treatment and the application of new preparation technology are the main methods to improve the degradation properties of Fe-based materials. Table 8 summarizes the corrosion resistance of Fe-based alloys with antibacterial properties manufactured by different alloying elements and processing processes.
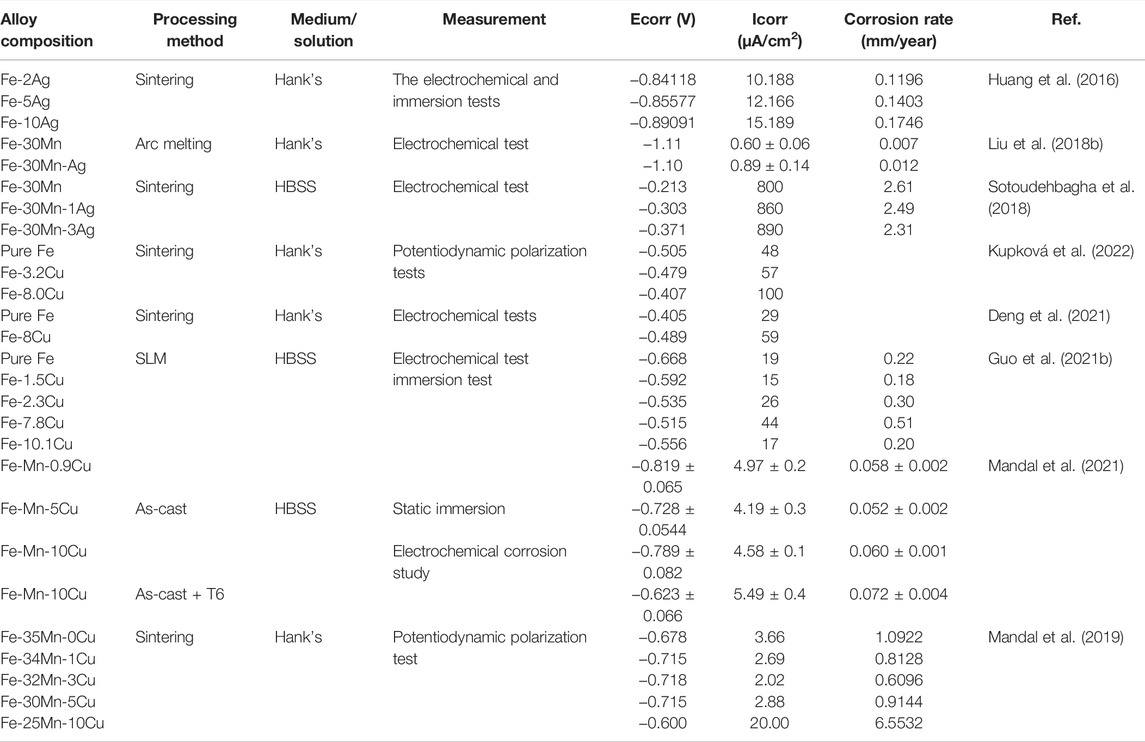
TABLE 8. Corrosive properties of Fe-based alloys with antibacterial properties as orthopedic implants.
Generally, the addition of antibacterial metal elements, such as Ag and Cu, accelerates the corrosion of Fe-based alloys (Sotoudehbagha et al., 2018; Guo et al., 2021b; Deng et al., 2021; Mandal et al., 2021). The standard electrode potential of Ag (+0.7996 V) and Cu (+0.337V) is much higher than that of Fe (−0.44 V) (Huang et al., 2016). The Cu-containing or Ag-containing second phase with high corrosion potential in Fe-based alloys can be used as independent cathodes, and the iron matrix acts as an anode, forming many micro corrosion cells to accelerate the electrochemical corrosion of Fe-based alloys (Sotoudehbagha et al., 2018). Huang et al. found out that the corrosion rate of Fe-x Ag (x = 2, 5, 10 wt%) alloys increased with increased silver content. Much precipitation of the second phase of sterling Ag brought about by the increase in Ag content significantly accelerated the degradation of the alloys (Huang et al., 2016). For Fe-Cu binary alloys, the corrosion rate varying with copper content is not unidirectional. Guo et al. confirmed that adding a small amount of Cu (1.5 wt%) to pure iron reduced the degradation rate of the alloy compared with pure iron. When the Cu addition reached 2.3 wt%, the alloy showed a significantly increased corrosion rate with the increase in copper addition. The degradation rate of Fe-7.8 wt%Cu alloy (0.51 mm/y) was almost 2.5 times that of pure iron (0.22 mm/y) (Guo et al., 2021b), consistent with the performance trend of sintered Fe-xCu (x = 0, 3.2, 8.0 wt%) alloys prepared by Kupková et al. (2022). However, when the added amount of Cu was further increased to 10.1 wt%, the degradation rate of the alloy reduced to 0.086 mm/y (Guo et al., 2021b) because, in addition to considering the galvanic corrosion induced by the precipitation phase, it is also necessary to pay attention to the influence of the formation of the passivation film and the distribution of the precipitation phase on the degradation of the alloys (Guo et al., 2021b). During the degradation of Fe-Cu alloys, the iron oxide layer forms a passivation film on the surface of the alloys, which will significantly inhibit the continued degradation of the alloys. The deterioration of galvanic corrosion caused by the addition of a small amount of Cu is counteracted by the protective effect of the passivation film. Moreover, the addition of excess Cu makes the alloy surface form more copper-rich phases, and the release of more Cu2+ will significantly promote the formation of passivation films. In addition, an excessive Cu-rich phase tends to form a network. The dense reticular copper-rich phase is also a layer of protection of the iron matrix (Guo et al., 2021b). It is not difficult to understand that Fe-based alloys with high Cu content exhibit slow corrosive properties. The corrosion resistance of Fe-Mn-Cu alloys is special. Mandal et al. confirmed that no passivation film is formed during the degradation of Fe-Mn-Cu alloys (Mandal et al., 2021). When the amount of Cu added to the casted Fe-Mn-Cu alloy was 5wt%, the alloy showed improved corrosion resistance due to the formation of a solid solution between Cu and Fe. When the amount of Cu content further increases, the degradation rate will be accelerated due to the intensification of galvanic corrosion (Mandal et al., 2021). The same trend was also found by Mandal et al. The Fe-25Mn-10Cu alloy prepared by powder sintering technology had a corrosion rate of 0.258 mmpy, which was six times that of the Fe-35Mn alloy (Mandal et al., 2019).
The metal preparation and metal forming processes also have a great impact on the corrosive properties of antibacterial Fe alloy. The microwave sintered alloy shows a porous structure. Porous alloys exhibit a larger surface area than as-cast ones with high density (Deng et al., 2021). What is more, Gap erosion is more prone to be developed in porous structures. The degradation rate of microwave sintered Fe-8Cu alloy manufactured by Deng et al. reaches up to 0.69 mm/y (Deng et al., 2021). The space holder method can produce alloys with a highly porous structure. Zhang et al. pointed out that the porosity of FePd2 alloy in this process reaches up to 60%, with a corrosion rate up to 1.162 mm/a (Čapek et al., 2017). Furthermore, the Fe alloy under SLM treatment is confirmed to present with excellent degradable behavior. The SLMed Fe-7.8 Cu alloy exhibits a rapid degradation rate, approximately 2.5 times higher than pure Fe (Guo et al., 2021b). The FePd2 alloy under Spark plasma sintering (SPS) treatment presents a better degradation behavior than the as-cast one because of the grain microstructure. Thus, rational development and utilization of the fabrication process are feasible to the improvement on the degradation behavior of Fe alloys (Čapek et al., 2017).
3.2.4 Biocompatibility
Developing degradable biomaterials with enhanced antibacterial properties is a challenging task because it requires a delicate balance between degradation rate, cell compatibility, and antibacterial properties (Mandal et al., 2021). The addition of Ag, Cu, and other metal elements to iron is conducive to antibacterial efficiency. However, it should be noted that the metal ion concentration released by the alloys should be lower than the cytotoxic limit so that the damage to mammalian cells is minimized (Mandal et al., 2021). Table 9 summarizes the in vitro cell compatibility of antibacterial Fe-based alloys with different contents of Ag or Cu.
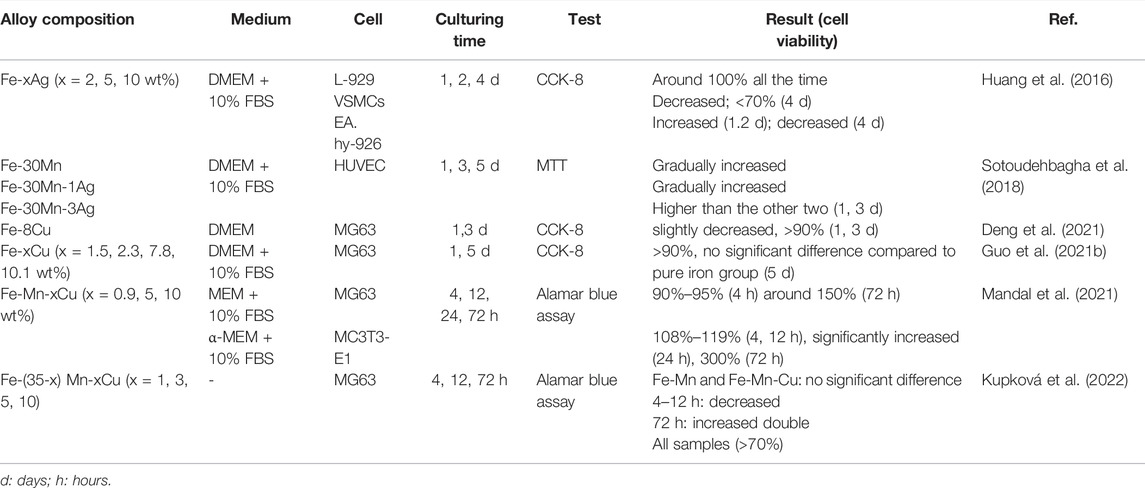
TABLE 9. In vitro biocompatibility of Fe-based alloys with antibacterial properties as orthopedic implants.
Antibacterial Fe-based alloys containing Cu or Ag have been reported as non-toxic to L-929 cells (Huang et al., 2016), MG 63 cells (Guo et al., 2021b; Deng et al., 2021; Mandal et al., 2021), and mc3T3-E1 (Mandal et al., 2019) cells. The addition of an appropriate amount of Cu to antibacterial Fe-based alloys has a positive effect on alloy biocompatibility. In Guo et al.’s study, MG63 cells adhered well and developed well on the surface of the SLMed Fe-xCu (x = 1.5, 2.3, 7.8, 10.1 wt%) alloys (Guo et al., 2021b). Fe-xMn-y Cu (x = 35, 34, 32, 30, 25 wt%; y = 0, 1, 3, 5, 10 wt%) alloys also exhibited good biocompatibility, and the extract of alloys showed a significant promotion of MG 63 cell proliferation (Mandal et al., 2019). Cu is an essential microelement that plays an important role in many processes of cellular metabolism (Huang et al., 2016). The positive impact of the addition of Cu on the biocompatibility of Fe-based alloys is not difficult to understand. However, it should be noted that excessive Cu is toxic to cells by promoting the formation of free radicals in cells (Mandal et al., 2021). The Cu content of the reported antibacterial Fe-based alloys is acceptable and does not produce significant cytotoxic effects (Mandal et al., 2019; Guo et al., 2021b; Deng et al., 2021; Mandal et al., 2021; Kupková et al., 2022). In vitro biocompatibility of Ag-containing Fe-based alloys is also acceptable. Huang et al. stated that the cell viability of L-929 cells remained at around 100% for 4 days in the extract of sintering Fe-x Ag (x = 2, 5, 10 wt%) (Gao et al., 2019b). Moreover, adding excessive Fe ions was also known to have adverse effects on cell proliferation (Guo et al., 2021b). A recent study confirmed that cell viability could not be inhibited when Fe ion concentrations are below 50 μgmL−1 (Kupková et al., 2022; Zhu et al., 2009). Guo et al. showed that, in the extract of SLMed Fe-Cu alloy, the release of Fe ions is within the acceptable range, and the SLMed Fe-Cu alloy had no obvious cytotoxicity to MG63 cells and good cytocompatibility (Guo et al., 2021b).
3.3 Zn-Based Alloys With Antibacterial Properties
Zn-based alloys have been increasingly favored as promising orthopedic implants in recent years (Xiao et al., 2021a; Zhang et al., 2021b). As an essential trace element, Zn is involved in the formation of bone and has perfect biocompatibility (Solomons, 2013). The antibacterial activity of Zn has been corroborated (Zhao et al., 2016a; Bakhsheshi-Rad et al., 2017). What is more, Zn is more dominant compared to Mg and Fe because the degradation rate of Zn is between that of Mg and Fe, and degradation products can be fully absorbed (Bowen et al., 2013). Nevertheless, the poor mechanical properties of pure Zn fail to meet the requirements for orthopedic implants (Hernández-Escobar et al., 2019; Peng et al., 2021b). Besides, cytotoxicity is prone to be induced due to a high concentration of Zn ions by inhibiting ECM mineralization (Li et al., 2019a; Wang et al., 2021b). Thus, a growing number of studies have focused on alloying Zn-based materials to ameliorate mechanical properties and biocompatibility (Li et al., 2019b; Hernández-Escobar et al., 2019). Recently, with the antibacterial effect of implants receiving much more attention, several Zn-based alloy orthopedic implants with antibacterial properties have been reported.
3.3.1 Antibacterial Properties
Zn-Ag alloys are the most studied Zn alloys with antibacterial activity. As expected, Zn-Ag alloys have been verified to be promising in vitro antibacterial activity against Gram-negative bacteria (E. coli) (Xie et al., 2018), Gram-positive and multi-resistant bacteria, including a potential strain of infection after maxillofacial surgery with an intraoral approach called Streptococcus gordonii (S. gordonii) (Loo et al., 2000; Li et al., 2018a), S. epidermidis, S. aureus, MRSA, and methicillin-resistant Staphylococcus epidermidis (MRSE) (Qu et al., 2021). Similar to Mg-Ag alloys, the antibacterial properties of Zn-Ag alloys enhance gradually with an increase in the Ag content (Xie et al., 2018; Qu et al., 2021). It is worth noting that the Zn-2Ag alloy demonstrated significant in vivo antibacterial activity against MRSA and inhibition of osteomyelitis in the rat femoral osteomyelitis prevention model (Qu et al., 2021). In addition, porous Zn-Ag alloy exhibited a stronger antibacterial effect than bulk Zn-Ag alloy (Xie et al., 2018). Given the biomimetic effect and osteogenic ability of porous structures, this finding adds further evidence and motivation for the development of porous alloy implants.
Other studies attempt to further add other alloying elements to manufacture ternary and quaternary Zn-Ag alloys, to improve the performance of alloy implants. Given that Mg is the most effective element to enhance the comprehensive performance of Zn-based materials among numerous alloying elements (Venezuela and Dargusch, 2019), Xiao et al. added Mg to the Zn-Ag alloy and developed Zn-0.05Mg-1.0Ag alloy with both superior mechanical properties and antibacterial capacity. This kind of ternary alloy exhibits a powerful antibacterial ability against S. aureus and E. coli (over 99%) (Xiao et al., 2019). Similarly, the Zn-0.04Mg-2Ag alloy prepared by Wu et al. also has strong antibacterial properties against S. aureus and S. epidermidis. However, in their study, the inhibitory effect of the alloy on E. coli is weak (Wu et al., 2021). The reason for such a discrepancy requires further studies and explanation. In addition, the quaternary Zn-2Ag-1.8Au-0.2V(wt%) alloy demonstrated enhanced antibacterial behaviors against S. gordonii, which was manufactured with the antibacterial ability of Ag (Li et al., 2019c). Combined with the antibacterial ability of Ag and Zr, the Zn-1Ag-0.05Zr alloy revealed ascendant inhibitory action against E. coli and S. aureus (Wątroba et al., 2019). However, it should be noted that the antibacterial ability of this ternary alloy seemed to be weaker than that of the Zn-1Ag alloy. This might be attributed to the low degradation rate and its impact on ions releasing, which is crucial for the generation of antibacterial ability (Wątroba et al., 2019). More studies are clearly required to fully understand this phenomenon.
Zn-Cu alloys are also confirmed to have great potential as orthopedic implants with antibacterial properties. They have been confirmed effectively against Gram-negative (E. coli) (Shuai et al., 2020), Gram-positive, and drug-resistant strains (S. aureus, S. epidermidis, MRSA, and MRSE) (Qu et al., 2020). The antibacterial ability of the alloy is proportional to the Cu content (Qu et al., 2020; Shuai et al., 2020). In addition, Li et al. demonstrated the significant inhibitory effect of Zn-4Cu alloy on the biofilm formation of mixed oral bacteria, the main causative agents of craniomaxillofacial osteosynthesis (Li et al., 2019d). Zn-Cu also exhibited excellent in vivo antibacterial properties. In a rat femoral intramedullary nail MRSA infection model, the Zn-2Cu alloy implants suppressed inflammation and toxicities caused by MRSA and played a beneficial role in preventing infection-related bone loss (Qu et al., 2020). Further alloying and processing on Zn-Cu alloys led to satisfactory outcomes. For instance, the ternary Zn-1Cu-0.1Ti alloy presented significantly improved antibacterial properties, which are manufactured by adding Ti element and dealing with hot rolling and cold rolling (Lin et al., 2020). The ternary Zn-0.5Cu-0.2Fe alloy guided bone regeneration (GBR) films obtained by introducing the Fe element and dealing with hot extrusion showed extensive inhibition of S. gordonii and mixed oral bacteria (Zhang et al., 2021c). Moreover, the Zn-Cu bimetallic foam obtained by electrochemical deposition and subordinate diffusion heat treatment also has excellent antibacterial performance (Tong et al., 2020). Besides, the unique porous architecture of this bimetallic foam plays a critical role in osseointegration and vessel ingrowth. By adjusting the pore structure, orthopedic implants with different properties and suitable for different body sites can also be customized (Tong et al., 2020). Overall, the application prospect of Zn-Cu alloys and Cu-containing zinc alloys prepared by a special process in orthopedic implants is very broad.
In addition, it has been reported that Zn-0.5Mg alloy shows a good antibacterial effect on E. coli and S. aureus (Xiao et al., 2018). The Zn-0.8Mg-0.2Sr alloy prepared by Capek et al. exhibited inhibitory effects on the adhesion and biofilm formation of S. gordonii (Čapek et al., 2021). Moreover, Bakhsheshi-Rad et al. confirmed that the addition of Mg element to Zn-Al alloys could improve the antibacterial properties of the alloys, which was expected to be further improved with higher Mg content (Bakhsheshi-Rad et al., 2017). As can be seen, Mg is an alloying element that is worthy of attention for fabricating Zn alloys with antibacterial properties.
From the current status of research, the development of Zn-based alloy orthopedic implants with antibacterial is still in the elementary stage, and most studies are primarily concentrated on the application of classical antibacterial metal elements, such as Ag and Cu (Table 6). However, there is no doubt that Zn-based alloys hold great potential as orthopedic implants with antibacterial properties. The research on processing technology and alloying elements, especially those with antibacterial properties, will promote the usage of Zn alloys in the field of orthopedic implants.
3.3.2 Mechanical Properties
As load-bearing implants, pure Zn exhibits poor behavior on mechanical strength and stretchability (Xiao et al., 2020). It has been shown that the tensile strength of pure Zn ranges from 10–110 MPa, elongation is 0.32%–36%, and Vickers hardness is 38–39 HV1 (Li et al., 2018b). The mechanical properties of alloys can be significantly improved by adding alloying elements and the fabrication process (Xiao et al., 2020). Parameters on mechanical properties of antibacterial Zn alloys are summarized in Table 10.
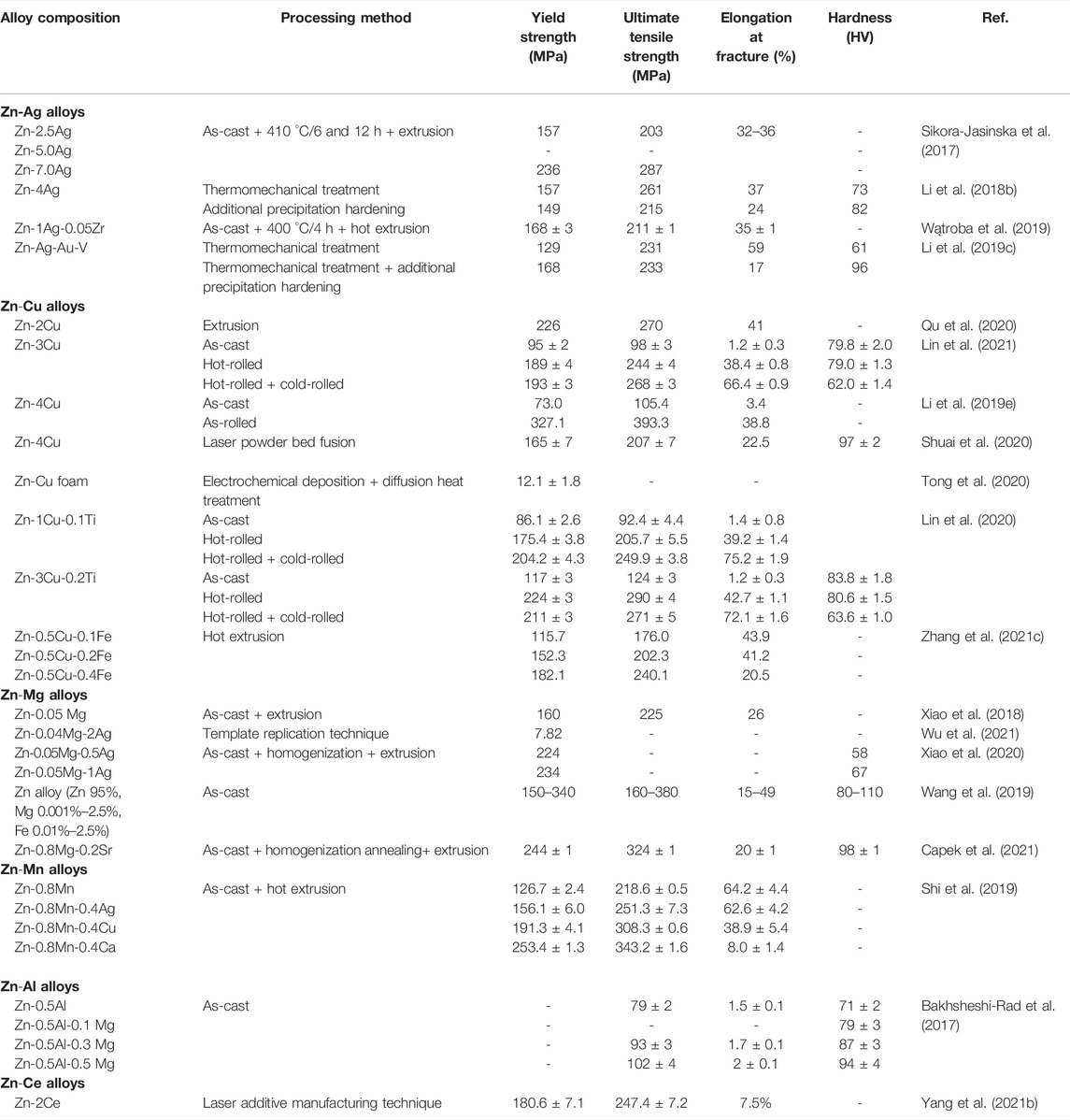
TABLE 10. Mechanical properties of Zn-based alloys with antibacterial properties as orthopedic implants.
It is reported that the addition of Ag and Cu can not only activate slip systems to maintain preponderant elongation of Zn alloys (Sikora-Jasinska et al., 2017; Lin et al., 2020) but also contribute to solution strengthening and effective grain refinement for the enhancement of mechanical behavior (Wątroba et al., 2019; Qu et al., 2020). Based on the Hall–Petch strengthening mechanism, the smaller particle size alloys are, the stronger yield strength they present (Lin et al., 2020). Precipitation of AgZn3 and ε-CuZn5 is a critical factor in improving the hardness and strength of alloys (Xie et al., 2018; Li et al., 2019e; Wątroba et al., 2019; Lin et al., 2020). When the Ag content reaches 3.5%, porous Zn-3.5Ag scaffold precipitates secondary phase AgZn3 (Xie et al., 2018), which decreases the grain size and helps further grain refinement (Wątroba et al., 2019). Nevertheless, the improvement in mechanical properties of alloys is not apparently observed when the size of precipitate phases reaches a certain level. Shuai et al. fabricated the Zn-Cu alloy by laser powder bed fusion, finding that when the Cu content was up to 4 wt%, the mechanical behavior of alloys appeared to be slightly decreased due to the stress concentration of the ε-CuZn5 phase in a larger size (Shuai et al., 2020). Moreover, Shi et al. suggested that although adding Cu increased the mechanical properties substantially, its stretchability declined from 64.2% to 38.9% compared with the Zn-0.8Mn alloy. However, the Cu alloy shows sufficiently high ductile properties (Shi et al., 2019). As a commonly used alloying element, Mg is also added to the antibacterial Zn alloy to enhance mechanical properties (Xiao et al., 2018). Similar to the effects of Cu and Ag, adding Mg remarkably improves the mechanical properties of antimicrobial Zn alloy mainly because of the formation of solid solution, grain refinement, and the obstacle of grain boundary sliding by intermetallic particles (Bakhsheshi-Rad et al., 2017; Čapek et al., 2021). During this process, the fine Mg2Zn11 particles with uniform distribution are pivotal in the excellent hardness and plasticity of the antibacterial Zn-based alloys (Xiao et al., 2018). Furthermore, the addition of alloying elements such as Ti (Lin et al., 2021), Ca (Shi et al., 2019), and Ce (Yang et al., 2021b) is proved to be effective in improving the antibacterial Zn alloy.
Besides adding alloying elements, improving mechanical properties can also be realized by proper fabrication processes such as extrusion, rolling, forcing, and annealing (Li et al., 2018b). The same as the addition of alloying elements, the purpose of these processes is all grain refinement, thus obtaining alloys with higher strength (Wątroba et al., 2019). As one of the most commonly used fabrication processes, extrusion has been extensively exploited for its drastic improvement of the mechanical properties of alloys (Sikora-Jasinska et al., 2017; Xiao et al., 2018; Čapek et al., 2021). Sikora-Jasinska et al. fabricated several kinds of binary Zn-x Ag alloys (x = 2.5, 5.0, 7.0 wt%) by hot extrusion (Sikora-Jasinska et al., 2017). Microscopic analysis indicated that hot extrusion brought a marked decrease in the grain size of alloys. The higher the Ag content, the smaller grain. What is more, yield strength and ultimate tensile strength of alloy were improved (Zn-7.0%Ag alloy corresponded to 236 and 287 MPa, respectively) due to the precipitation of fine AgZn3 particles along grain boundaries (Sikora-Jasinska et al., 2017). Similar results are seen in the other Zn alloys by hot extrusion (Li et al., 2018b; Xiao et al., 2018; Kodetová et al., 2019; Čapek et al., 2021). This may be related to the occurrence of dynamic recrystallization (DRX) during the extrusion process. Capek et al. found out that the microstructure of extruded materials consisted of complete recrystallized grains with a size of merely 2.4 μm (Čapek et al., 2021). Besides significant enhancement of the strength of alloys, hot extrusion can also eliminate the fragility of as-cast alloys and improve plasticity. Shi et al. suggested that the elongation of extruded Zn-0.8Mn alloys arrived at 64.2%, while it was solely 1.0% for as-cast ones (Xiao et al., 2018). The solution heat-treatment is also an approach for enhancing the mechanical properties of alloys (Sun et al., 2020). By this process, Sun et al. manufactured the Zn-0.8Mn alloy and found that solution heat-treatment at 380°C enabled MnZn13 particles to dissolve into the Zn matrix, leading to a solid solution hardening effect. This effect appeared to become more significant with elongated treatment time (Sun et al., 2020). This is likely because heat treatment (solution annealing) transforms a dendritic cast structure into a globular structure, resulting in a more stable structure, unlike the dendritic presence of anisotropy in as-cast alloys (Kodetová et al., 2019). Rolling is also a frequent process. Lin et al. compared Zn-Cu alloy under the treatment of hot and cold rolling with the as-cast and hot-rolled one, finding a significant enhancement in strength and plasticity because, with the process of hot and cold rolling, precipitated hard and brittle ε- CuZn5 particles were evenly distributed in the η-Zn matrix after fragmentation into minute particles (Lin et al., 2020). Nonetheless, processing in such a manner leads to the reduction in the Cu content in the η-Zn matrix and thus a weakening of solid-solution strengthening, causing a dramatic decline in hardness (Lin et al., 2020; Lin et al., 2021). Furthermore, 3D printing and additive manufacturing are also suited to the fabrication of Zn alloys with outstanding mechanical properties (Shuai et al., 2020; Yang et al., 2021b).
Alloys with porous structures offer desirable structural conditions for the proliferation and differentiation of osteoblasts because their pore-size range is consistent with that of the cancellous bone pore (400–600 mm) in the human body (Wu et al., 2021). What is more, the unique degradable characteristics enable bone healing without subsequent operating surgeons after implantation (Tong et al., 2020). For this reason, the development of porous structure quickly gains popularity for antibacterial Zn and its alloys as bone implants. Compared with bulk structure, the mechanical behavior of porous structure (compressive plateau stress and elastic modulus) gets poor because of increased porosity, which restricts its high load-bearing applications (Zhao et al., 2016b). The current fabrication of porous scaffolds mainly concentrates on pure Zn (Zhao et al., 2016b; Zhao et al., 2018; Cockerill et al., 2020). In contrast, only Zn-0.04Mg-2Ag is successfully developed and proved with excellent biological performance among porous antimicrobial Zn alloys as bone implants (Wu et al., 2021). Thus, the fabrication of more porous scaffolds of antibacterial Zn alloys represents new research directions in the future.
3.3.3 Corrosion Resistance
Compared with Mg and Fe, Zn has moderate corrosion resistance, because its standard corrosion potential (−0.762 VSCE) ranges between Fe (−0.440 VSCE) and Mg (−2.372 VSCE) (Li et al., 2018b), which avoids hydrogen accumulation caused by rapid corrosion rate and strong corrosion resistance to hinder clinical applications of alloys. Nonetheless, the corrosion rate of pure Zn is 9.6 μm/a (Wątroba et al., 2019), and there is a clinical need for adding alloying elements to improve the degradation rate. Table 11 summarizes the corrosion resistance performance of antibacterial Zn alloys in in vitro studies.
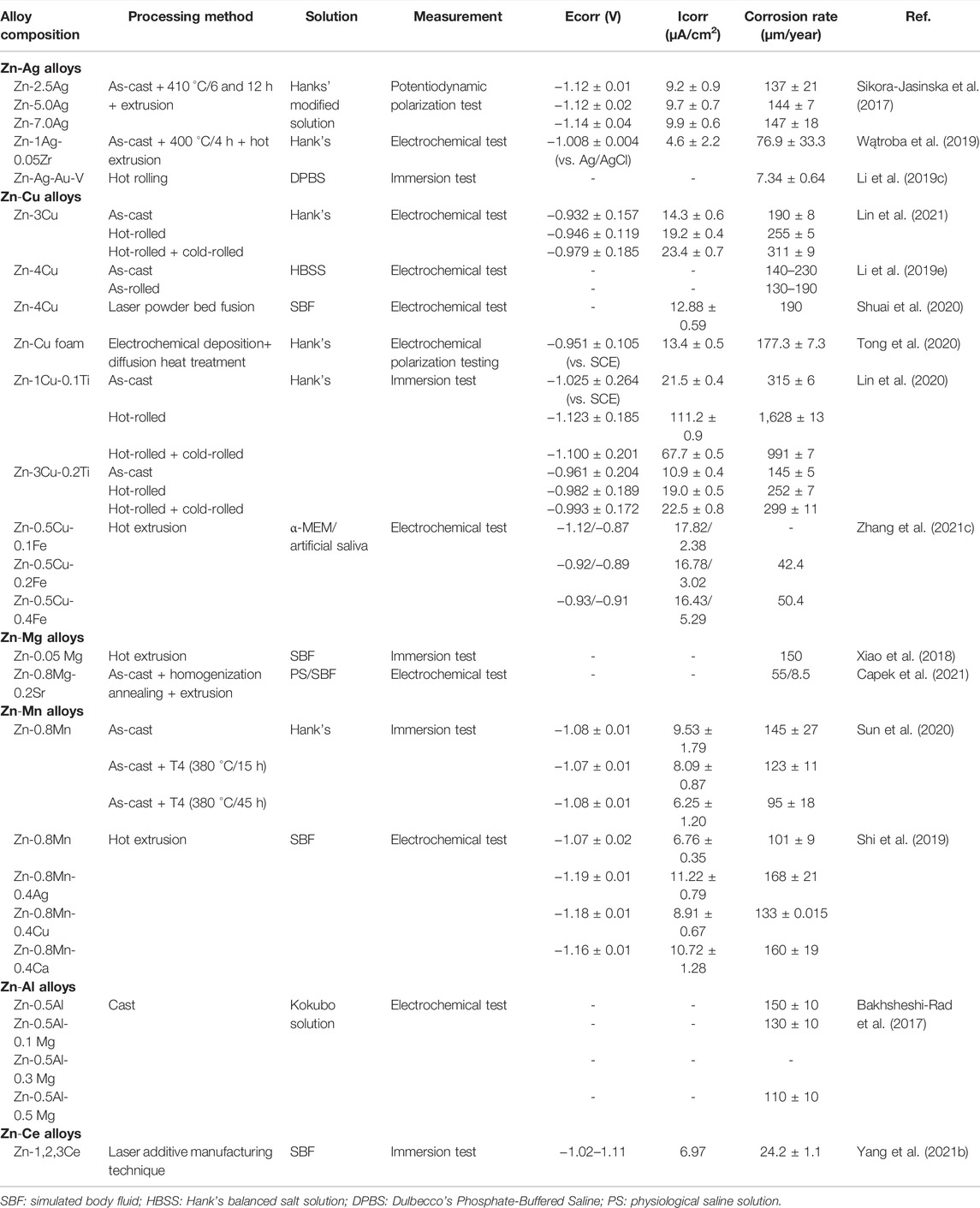
TABLE 11. Corrosion resistance of Zn-based alloys with antibacterial properties as orthopedic implants.
The addition of the Ag and Cu elements remarkably decreases the corrosion resistance of Zn alloys, thus substantially improving the degradation rate (Li et al., 2018b; Qu et al., 2021) because possibly a higher standard electrode potential of Ag and Cu than Zn declines the Ecorr value (Shi et al., 2019), and the formation of AgZn3 and −CuZn5 phases induces galvanic corrosion (Qu et al., 2020; Shuai et al., 2020), which is confirmed to be more apparent in the samples with high Cu contents (Zn-7.0Ag, Zn-4.0Cu) (Sikora-Jasinska et al., 2017; Shuai et al., 2020). The AgZn3 phase can damage the densified surface of the matrix (Xie et al., 2018), such as ZnO, Zn(OH)2, and Ca3(PO4)2 (Sikora-Jasinska et al., 2017; Wątroba et al., 2019), thereby further accelerating the degradation rate. However, it is not absolute. The Zn-Ag-Au-v alloy exhibits a lower degradation rate than pure Zn in the test carried out in phosphate-buffered saline (PBS) due to the formation of zinc phosphate, a more densified passivation film than Zn(OH)2 (Kodetová et al., 2019). Unlike the AgZn3 phase, the ε- CuZn5 phase is demonstrated to have twofold implications on the corrosion behavior. The continuous reticular structure formed by the ε- CuZn5 phase in the matrix functions as a protective barrier to hinder corrosion (Lin et al., 2020). Adding other elements exerts a certain influence on the degradation rate of alloys. For instance, the addition of 0.2 wt% Fe to Zn-0.5Cu alloys accelerates the degradation of alloys (Zhang et al., 2021c). Ti (Lin et al., 2021), Ce (Yang et al., 2021b), and Mn (Sun et al., 2020) are also confirmed to enhance corrosion resistance when added to antibacterial Zn alloys. In contrast, the corrosion resistance of Zn alloys is not particularly affected by adding trace amounts of Mg. The corrosion rate is approximately 0.15 mm/a, consistent with that of pure Zn (Xiao et al., 2018).
The fabrication process also exerts a dramatic effect on the corrosion rate. Compared to as-cast alloys, hot rolled ones present more uniform corrosion characteristics due to grain refinement and even distribution (Li et al., 2019e; Kodetová et al., 2019). Furthermore, hot rolling tends to accelerate corrosion, which may be related to the galvanic corrosion occurring between secondary phases and the Zn matrix, as well as the destruction of the natural oxide layer (Li et al., 2019e) and reticular structure formed by secondary phases in the matrix (Lin et al., 2021). In the study on Zn-3Cu and Zn-3Cu-0.2Ti alloys by Lin et al., compared with hot rolling, the hot-rolled + cold-rolled samples exhibited a more rapid corrosion rate due to the higher content of ε-CuZn5 secondary phases and increased micro-battery reaction with η-Zn phases in the matrix (Lin et al., 2021). However, the results of the alloy foam with porous structure appear to be in contrast to those of bulk alloys. The Zn-Cu foam alloy manufactured by Tong et al. is proven with excellent corrosion properties (Tong et al., 2020). Nyquist plots show that the radius of the capacitive arc of foam increases after heat treatment, leading to larger corrosion resistance and a lower corrosion rate (Tong et al., 2020).
Ideally, the strength of antimicrobial Zn alloy in vivo as implants diminishes over time, which is crucial for reducing stress shielding and recovering the physiological stress of bones (Wang et al., 2019). Thus, in vivo studies on biodegradable materials are critical. Nevertheless, recent in vivo studies on the biodegradation of Zn alloys are rare (Wang et al., 2019). Clearly, future studies will need to focus on degradation and changes in mechanical properties of alloys in vivo to obtain antibacterial Zn alloys with outstanding comprehensive performance as bone implants.
3.3.4 Biocompatibility
As bone implants, the evaluation of the biocompatibility of antibacterial Zn-based alloys has major clinical implications (Wu et al., 2021). According to the reference to the human body, the recommended intake levels of Zn, Cu, Mg, and Ag are 12–16 Mg/d, 0.9–1.2 Mg/d, 240–400 Mg/d, and 0.4–27 μg/d (Xiao et al., 2020; Lin et al., 2021). There are no toxic side effects to human tissues and organs when the amount of ions release is lower than that of the daily recommended intake. Otherwise, over-releasing of metal ions tends to trigger cytotoxicity, and then inflammation response, carcinogenic stimulation, autoimmunity, and allergy (Xiao et al., 2020). Currently, studies on the biocompatibility of Zn alloys mainly focus on in vitro analysis. Table 12 summarizes the biocompatible performance of antibacterial Zn alloys in in vitro studies.
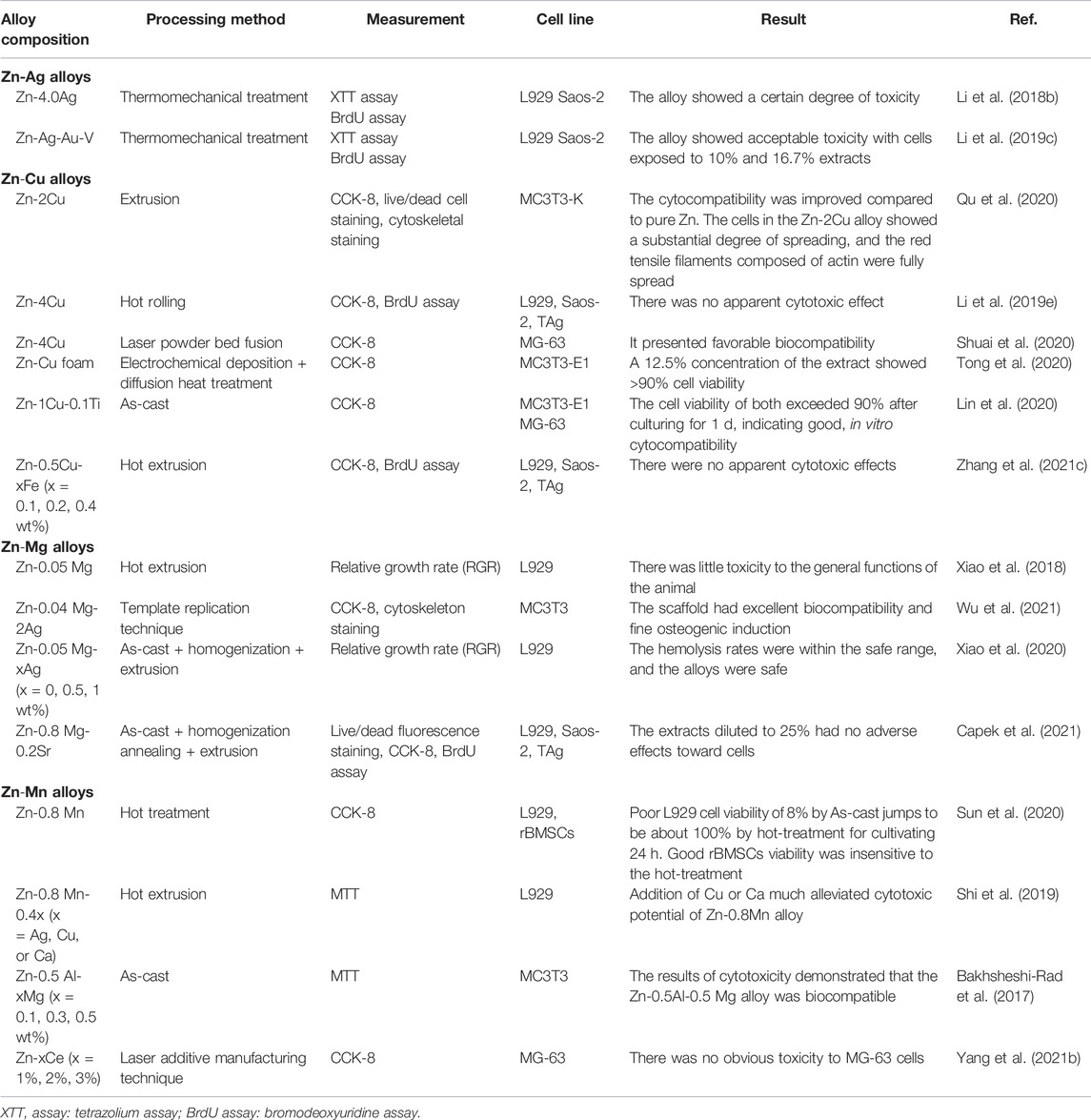
TABLE 12. In vitro biocompatibility of Zn-based alloys with antibacterial properties as orthopedic implants.
The addition of Ag (Li et al., 2018b) and Cu (Shi et al., 2019; Shuai et al., 2020) substantially increases the biocompatibility of pure Zn without producing extra cytotoxicity and even exhibits a significant improvement in the cell metabolic and proliferative activity (Li et al., 2018b; Li et al., 2019e). Xiao et al. analyzed the cell morphology of L-929 and relative growth rate (RGR), confirming that adding 0.5 and 1 wt% Ag to the Zn-0.05wt.%Mg alloy largely increased RGR (Xiao et al., 2020). Another study demonstrated that the cellular survival rate exceeded 80% when the concentration in Zn-3Cu and Zn-3Cu-0.2 Ti alloy extracts was no more than 25% (Lin et al., 2021). Except for the concentration of Zn and Cu ions in extracts within the recommended amounts, it is also correlative with the high cellular tolerance of ions (Li et al., 2019e; Zhang et al., 2021c). The Zn-Mg alloy is a non-toxic material with good biocompatibility. Its extracts show excellent cell morphology, tolerance, and adherence (Wang et al., 2019; Wu et al., 2021; Čapek et al., 2021). What is more, the in vivo degradation of alloys causes no harm to important organs and cellular structures (Xiao et al., 2018). However, it should be noted that extracts without dilution usually exhibit apparent cytotoxicity, which adversely affects the survival, proliferation, and adherence of cells. This is mainly due to the inhibition of high ions concentration and high osmolarity on cellular adherence and growth (Lin et al., 2021). Generally, metal ions in low concentrations are beneficial to cells, but an opposite trend is observed in high concentrations (Shi et al., 2019; Sun et al., 2020). In undiluted extracts of Zn-4Ag (Li et al., 2018b) and Zn-Ag-Au-V alloys (Kodetová et al., 2019), apparent cytotoxicity is observed, which almost completely inhibits the cellular activity and proliferation. The dilution of extracts shows no toxicity to cells, enabling a gradual increase in cellular activity (Kodetová et al., 2019; Lin et al., 2020; Tong et al., 2020).
Zn is confirmed with osteogenesis activities, promoting bone formation by increasing calcium content, collagen content, and alkaline phosphate activity (Xiao et al., 2018). The addition of Cu, Ag, and Mg elements enables the further proliferation of osteoblasts, thus presenting better osteogenic induction in antibacterial Zn alloys (Xiao et al., 2018; Qu et al., 2020; Wu et al., 2021). Mg ions significantly upregulate the expression of OSX, OPN, and OC9 (Wu et al., 2021), leading to more adhesion and proliferation of cells, thereby promoting bone healing (Xiao et al., 2018). Similarly, releasing Cu ions enhances the expression of osteogenesis-related genes ALP, COL 1, OCN, and Runx-2 (Qu et al., 2020).
Currently, there is not much in vivo research on the biosafety of antimicrobial Zn alloys, most of which are focused on Zn-Mg alloys (Xiao et al., 2018; Wang et al., 2019; Xiao et al., 2021b). Nonetheless, due to the differences in the degradable environment and sensitivity (Xiao et al., 2018), there is an apparent discrepancy between in vivo and in vitro results on the biocompatibility of alloys (Čapek et al., 2021). Even current standardized tests (ISO 10993: −5 and −12) cannot mimic the physiological metabolism in vivo (Čapek et al., 2021). Therefore, further studies on in vivo biocompatibility are greatly warranted.
4 Antibacterial Mechanism of Mg-, Zn-, and Fe-Based Alloys as Orthopedic Implants
The exact antibacterial mechanisms of alloys have still not been completely elucidated due to their complexity. Nonetheless, a thorough comprehension of antibacterial mechanisms is indispensable for the improvement of the design and application of alloy materials with antibacterial properties as orthopedic implants. Presently, the proposed antibacterial mechanisms mainly include four aspects as follows (Figure 1).
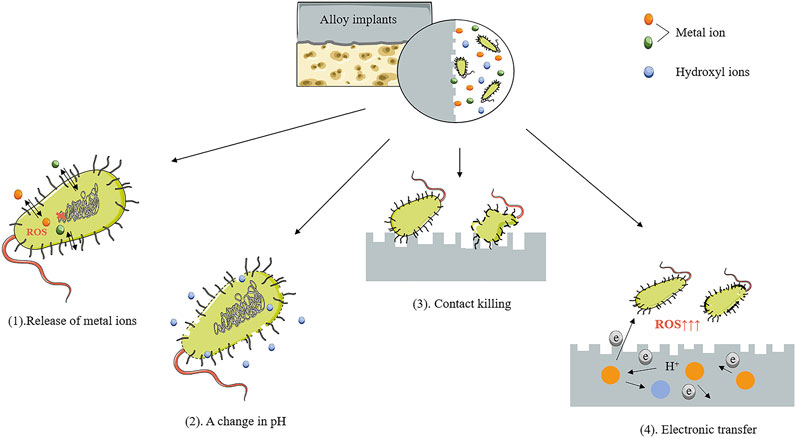
FIGURE 1. Antibacterial mechanism of Mg-, Zn-, and Fe-based alloys as orthopedic implants. Presently, the proposed antibacterial mechanisms mainly include four aspects: (1) release of metal ions, (2) a change in pH, (3) contact killing, and (4) electronic transfer.
4.1 Release of Metal Ions
Metal ions, including Ag+, Cu2+, and Zn2+, released gradually from alloys during the degradation process, are confirmed to inhibit or even kill bacteria via multiple pathways. These metal ions are available to bind to bacterial membranes and proteins, thus resulting in greatly increased cell membrane permeability (Feng et al., 2018), which may cause the loss of large volumes of cytoplasm. Simultaneously, these metal ions enter the cytoplasm of bacteria and interact with sulfhydryl groups of proteins, causing irreversible inactivation of proteins owing to the breakage of ionic bonds. These metal ions also produce large quantities of reactive oxygen species (ROS). Ultimately, these events induce the collapse of bacteria’s respiratory and material transport and degradation of DNA (Marambio-Jones and Hoek, 2010; Amin Yavari et al., 2016; Ma et al., 2018). What has been corroborated by in vitro studies of Zn-Ag and Mg-Cu alloys is that the expression of genes linked to biofilm formation, bacterial adhesion, autolysis, cell wall biosynthesis, cytotoxicity, and drug resistance is interfered with due to the existence of metal ions (Li et al., 2016b; Li et al., 2018a).
Notably, recent studies have confirmed that Zn2+ and Mg2+ can also produce immunomodulatory antibacterial activity by influencing the local immune microenvironment. It has been demonstrated that the addition of Zn to hydroxyapatite (HA) can reduce the expression of pro-inflammatory mediator interleukin- (IL-) 8 and the matrix metalloproteinase-9 (Velard et al., 2010). Moreover, under the modulation of the microenvironment created by the implanted scaffolds, macrophages undergo polarization to the anti-inflammatory (M2) phenotype or pro-inflammatory (M1) phenotype and subsequently release a wide series of bioactive molecules, leading to active regeneration of bone or induction of persistent inflammation, respectively. To date, most studies have mainly identified that Zn2+ deficiency can aggravate the inflammatory response. Huang et al. demonstrated that Zn2+ could promote the polarization of macrophages from M1 to M2 through PI3K/Akt/mTOR pathway. The M2 phenotype polarization of macrophages and the subsequent biological events will create a favorable osteogenic microenvironment (Huang et al., 2021). The immunomodulatory action of Mg2+ is related to its concentration. Mg2+ at high concentrations has been corroborated to play a role in promoting macrophages polarization to M1 phenotype, increasing the phagocytic ability of the bacteria and expression of TNF-αand iNOS that are crucial to bacterial clearance (Xie et al., 2022). However, persistent and hyperactivated inflammation has an unfavorable effect on bone regeneration (Schmidt-Bleek et al., 2012; Liu et al., 2018a). The corrosion layer produced by the degradation of implants can impede the release of Mg ions (Xie et al., 2022). The environment containing low concentrations of Mg ions are likely to exhibit anti-inflammatory function by suppressing activation of NF-κB, thereby reducing the expression of proinflammatory cytokines in macrophages such as TNF-α, IL-6, and IL-1β (Hu et al., 2018).
Accordingly, the release of metal ions in alloy implants has an integral role in determining the antibacterial activities of alloys (Figure 2). However, it should not be overlooked that although most ions have been confirmed with antibacterial activities that are positively correlated with ions concentration, excessive released ions elicit cytotoxic effects or show safety issues in vivo. Therefore, reasonable metal content and metal ion release of the alloys are critically essential.
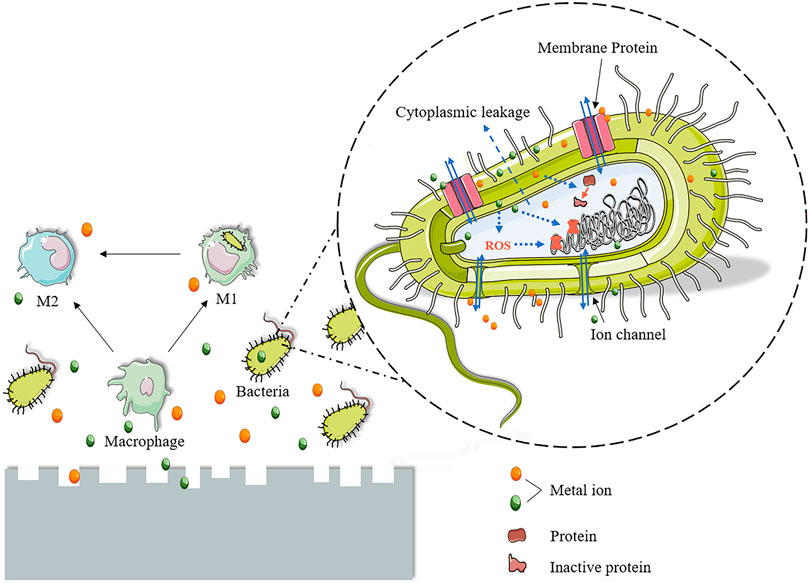
FIGURE 2. Antibacterial mechanism of metal ions. The effects of metal ions released by alloys on bacteria include two major aspects. On the one hand, these metal ions can bind to bacterial membranes and proteins, resulting in a greatly increased cell membrane permeability, which may lead to massive cytoplasmic loss. At the same time, these metal ions enter the bacterial cytoplasm and interact with the sulfhydryl group of the protein, resulting in irreversible inactivation of the protein due to the cleavage of the ionic bond. Moreover, these metal ions also generate a large amount of reactive oxygen species (ROS). Ultimately, these events lead to the breakdown of bacterial respiration and material transport and the degradation of DNA. On the other hand, metal ions, Zn and Mg, have immunomodulatory antibacterial mechanisms. They can modulate the immune microenvironment by modulating the polarization of macrophages and ultimately play a role in bacterial clearance and killing and the control of inflammation.
4.2 A Change in pH Value (Creation of an Alkaline Environment)
During the degradation process of Mg-, Zn-, and Fe-based alloys, the pH value of the surrounding environment elevates, accompanied by a massive generation of hydroxyl ions (Li et al., 2019c; Luque-Agudo et al., 2020; Guo et al., 2021c). The pH value appropriate for bacterial survival ranges from 6.0 to 8.0. Both over-acid and over-alkaline environments are detrimental to bacterial growth (Krulwich et al., 2011). The existence of an alkaline environment further enhances the antibacterial property of alloys, which appears prominently in Mg-based alloys. It is regarded as the major antibacterial mechanism of Mg alloys (Qin et al., 2015a; Feng et al., 2018; Luque-Agudo et al., 2020). It has even been demonstrated that the bactericidal effect of Mg is entirely due to the elevation of pH values rather than the release of Mg2+ because bacterial growth is not inhibited at all when the pH of the supernatant in the corrosive solution of Mg-based alloys is regulated to neutral or performing bacteriostatic experiment with Mg2+ solely (Robinson et al., 2010; Rahim et al., 2015). Besides, the antibacterial property of Mg increases gradually with the augment of pH during the degradation process, suggesting that the antibacterial property of the Mg-based alloys has a positive correlation with the PH value (Robinson et al., 2010; Qin et al., 2015a). According to the in vitro experiments by Qin et al. and Rahim et al., when relying solely on high alkaline pH to inhibit bacteria, the pH value of the solution needs to reach 9. When the pH value is greater than 10, it can produce higher antibacterial efficiency. However, the medium with a pH value of 8 cannot produce an antibacterial effect (Qin et al., 2015b; Rahim et al., 2015). In the in vitro immersion tests of antibacterial alloys, due to the large differences in the degradation rates of various alloys and the use of different culture solutions, the obtained pH change curves are also different. For some alloys, the pH value of the immersion solution can exceed 9 after immersing for a few hours (Li et al., 2016b; Yan et al., 2019). For example, the pH value of the as-cast Mg-x Cu (x = 0.1, 0.25 wt%) alloy immersion solution exceeded 9 after only 3 h of immersion in vitro (Li et al., 2016b). However, the pH value of the immersion solution of some alloys rises slowly, and it takes several days to reach an effective bacteriostatic pH (Qin et al., 2015a; He et al., 2015; Liu et al., 2017b). The zinc-containing Mg-Ca-Sr alloy reported by He et al. did not reach a pH value of 10 until 5 days of immersion (He et al., 2015). In summary, the time an alloy takes to reach an effective bacteriostatic pH ranges from a few hours to a few days, depending on its degradation rate. At the same time, as briefly mentioned above, there may be large differences in the in vitro and in vivo degradation behavior of alloys. The changes induced by alloy degradation in vivo remain to be investigated. Moreover, when Mg-based alloys are used in vivo, their antibacterial property will decrease because the high pH value is prone to be gradually buffered by body fluid (Bartsch et al., 2014; Zhao et al., 2020). Bacteria in a high alkalinity environment will release a large amount of H+ to partially neutralize the high environmental pH (Qin et al., 2015b). Thus, in vivo, the elevation of pH value may only exert bacteriostasis at the early stages (Liu et al., 2016).
4.3 Contact Killing (Direct Contact Sterilization)
In addition to fatal killing effects on bacteria caused by metal ions and hydroxyl ions released from alloy degradation, the surface of alloys can also cause damage by direct contact with bacteria. Exposure of bacteria to the surface of alloys leads to the destruction of bacterial membrane and structure, as well as the repression of adhesion (Jiao et al., 2021). Qin et al. confirmed that Zn and Zr on the surface of Mg-Nd-Zn-Zr alloy could suppress the bacterial colonization and exhibited a direct contact killing effect on MRSA (Qin et al., 2015a). The surface of Sr or Ag-doped alloys shows resistance to bacterial adhesion and biofilm formation as well in the experiment of simulating competitive surface colonization by co-culture with cells and bacteria (Cochis et al., 2020). Deng et al. stated that the surface of porous microwave sintered Fe-Cu alloy could cause perforation, deformation, and damage to cell membranes, thus inducing bacterial cell death. Porous structure and Cu-rich precipitation phase play an important role in the mechanical killing process (Deng et al., 2021). The in vivo anti-osteomyelitis assays of Mg-Cu alloys showed that bacterial adhesion was not observable on the surface of alloys, which also verified the inhibition of bacterial adhesion on alloy surface (Li et al., 2016b).
4.4 Electron Transfer
Electron transfer is essential for bacterial energy metabolism and survival (Gomaa et al., 2022). Electron transfer is an essential link in bacterial respiration (Shi et al., 2016). Disrupting this process will stimulate the massive production of ROS in the bacteria and produce a killing effect on the bacteria (Wang et al., 2018). Metal ions released from alloy implants affect bacterial electron transfer. For example, the interaction between Ag+ and bacterial sulfhydryl groups interferes with essential enzymes in the respiratory chain and prevents sufficient electron transfer to oxygen, ultimately leading to the production of large amounts of ROS (Park et al., 2009). In addition, Wang et al. confirmed that active metal ions such as Zn2+ and Cu2+ might catalyze electron transfer. Moreover, various metal ions can promote each other and coordinately enhance the interference effect on the electron transfer of bacterial ion channels, as suggested by Wang et al. (2016). In their study, the antibacterial effect of multi-component solutions containing Ag+, Zn2+, and Cu2+ was much stronger than that of single-component solutions with the same ionic concentration (Wang et al., 2016), suggesting that multi-component alloys may have better anti-infective properties due to the synergistic antibacterial effect of multiple metal ions. Interestingly, the increase in the pH value caused by alloy degradation also affects the electron transfer of bacteria. As mentioned above, bacteria in a high alkalinity environment produce large amounts of H+ to neutralize the high environmental pH value (Qin et al., 2015b). This net transfer of protons to the extracellular compartment disrupts the bacterial transmembrane electrochemical gradient and results in ATP synthesis disorder. Eventually, bacteria die due to abnormal proliferation and metabolism. Furthermore, the potential difference between the components in the alloy implants will lead to electron transfer. Excessive consumption of H+ during electron transfer can affect the activity of cellular proton pumps, resulting in a massive release of ROS and the killing of bacteria (Zhang et al., 2021b). Potential differences between the components in alloys result in electron transfer. H+ will be consumed in the process of electron transfer, which will affect the activity of the proton pump. This ultimately induces the abundant release of ROS and the killing of bacteria (Lemire et al., 2013).
5 Conclusion
It is a feasible and efficient strategy to develop biodegradable alloys with antibacterial properties as orthopedic implants to solve the issues on IAI. Mg-, Zn-, and Fe-based alloys with antibacterial properties hold great application prospects. Nonetheless, studies on Mg-, Zn-, and Fe-based alloys with antibacterial properties as orthopedic implants are still at the exploratory stage. The evaluation of alloy performance is far from perfect, and most alloys still stay at the stage of in vitro antibacterial assay. Therefore, in vivo evaluation of the alloys needs further refinement to facilitate translation toward clinical application. Furthermore, comparing Mg- and Zn-based alloys, the development of Fe-based alloys with antibacterial properties as orthopedic implants is obviously insufficient. Future studies could devote additional attention to the manufacturing of Fe-based alloys with antibacterial properties. Because the antibacterial mechanisms of alloys remain unclear, studies on mechanisms are still indispensable for further design of alloy implants with antibacterial properties. Overall, there are still some unknowns about biodegradable alloys with antibacterial properties as orthopedic implants, which await exploration in future research, whether from the perspective of design and development or studies on antibacterial mechanisms.
Author Contributions
NW and YM wrote the original manuscript. SY contributed to financial support, correction, supervision, and editing of the manuscript. HS and YS reviewed and edited the manuscript. SG supervised and edited the manuscript. All authors contributed to manuscript revision and read and approved the submitted version.
Funding
The work was supported by grants from the Natural Science Foundation of Liaoning Province (2021-BS-103) and the China Postdoctoral Science Foundation Grant (2020M681020).
Conflict of Interest
The authors declare that the research was conducted in the absence of any commercial or financial relationships that could be construed as a potential conflict of interest.
Publisher’s Note
All claims expressed in this article are solely those of the authors and do not necessarily represent those of their affiliated organizations or those of the publisher, the editors, and the reviewers. Any product that may be evaluated in this article, or claim that may be made by its manufacturer, is not guaranteed or endorsed by the publisher.
References
Agarwal, R., and García, A. J. (2015). Biomaterial Strategies for Engineering Implants for Enhanced Osseointegration and Bone Repair. Adv. Drug Deliv. Rev. 94, 53–62. doi:10.1016/j.addr.2015.03.013
Ahmadabadi, H. Y., Yu, K., and Kizhakkedathu, J. N. (2020). Surface Modification Approaches for Prevention of Implant Associated Infections. Colloids Surf. B: Biointerfaces 193, 111116. doi:10.1016/j.colsurfb.2020.111116
Al Jabbari, Y. S. (2014). Physico-mechanical Properties and Prosthodontic Applications of Co-cr Dental Alloys: a Review of the Literature. J. Adv. Prosthodont. 6 (2), 138–145. doi:10.4047/jap.2014.6.2.138
Amin Yavari, S., Loozen, L., Paganelli, F. L., Bakhshandeh, S., Lietaert, K., Groot, J. A., et al. (2016). Antibacterial Behavior of Additively Manufactured Porous Titanium with Nanotubular Surfaces Releasing Silver Ions. ACS Appl. Mater. Inter. 8 (27), 17080–17089. doi:10.1021/acsami.6b03152
Arciola, C. R., An, Y. H., Campoccia, D., Donati, M. E., and Montanaro, L. (2005). Etiology of Implant Orthopedic Infections: a Survey on 1027 Clinical Isolates. Int. J. Artif. Organs 28 (11), 1091–1100. doi:10.1177/039139880502801106
Bakhsheshi-Rad, H. R., Dayaghi, E., Ismail, A. F., Aziz, M., Akhavan-Farid, A., and Chen, X. (2019). Synthesis and In-Vitro Characterization of Biodegradable Porous Magnesium-Based Scaffolds Containing Silver for Bone Tissue Engineering. Trans. Nonferrous Met. Soc. China 29 (5), 984–996. doi:10.1016/s1003-6326(19)65007-7
Bakhsheshi-Rad, H. R., Hamzah, E., Low, H. T., Kasiri-Asgarani, M., Farahany, S., Akbari, E., et al. (2017). Fabrication of Biodegradable Zn-Al-Mg alloy: Mechanical Properties, Corrosion Behavior, Cytotoxicity and Antibacterial Activities. Mater. Sci. Eng. C 73, 215–219. doi:10.1016/j.msec.2016.11.138
Bartsch, I., Willbold, E., Rosenhahn, B., and Witte, F. (2014). Non-invasive pH Determination Adjacent to Degradable Biomaterials In Vivo. Acta Biomater. 10 (1), 34–39. doi:10.1016/j.actbio.2013.08.047
Bee, S.-L., Bustami, Y., Ul-Hamid, A., Lim, K., and Abdul Hamid, Z. A. (2021). Synthesis of Silver Nanoparticle-Decorated Hydroxyapatite Nanocomposite with Combined Bioactivity and Antibacterial Properties. J. Mater. Sci. Mater. Med. 32 (9), 106. doi:10.1007/s10856-021-06590-y
Bekmurzayeva, A., Duncanson, W. J., Azevedo, H. S., and Kanayeva, D. (2018). Surface Modification of Stainless Steel for Biomedical Applications: Revisiting a century-old Material. Mater. Sci. Eng. C 93, 1073–1089. doi:10.1016/j.msec.2018.08.049
Besinis, A., De Peralta, T., and Handy, R. D. (2014). Inhibition of Biofilm Formation and Antibacterial Properties of a Silver Nano-Coating on Human Dentine. Nanotoxicology 8 (7), 745–754. doi:10.3109/17435390.2013.825343
Bian, D., Deng, J., Li, N., Chu, X., Liu, Y., Li, W., et al. (2018). In Vitro and In Vivo Studies on Biomedical Magnesium Low-Alloying with Elements Gadolinium and Zinc for Orthopedic Implant Applications. ACS Appl. Mater. Inter. 10 (5), 4394–4408. doi:10.1021/acsami.7b15498In
Bowen, P. K., Drelich, J., and Goldman, J. (2013). Zinc Exhibits Ideal Physiological Corrosion Behavior for Bioabsorbable Stents. Adv. Mater. 25 (18), 2577–2582. doi:10.1002/adma.201300226
Bryla, K., Horky, J., Krystian, M., Litynska-Dobrzynska, L., and Mingler, B. (2020). Microstructure, Mechanical Properties, and Degradation of Mg-Ag alloy after Equal-Channel Angular Pressing. Mater. Sci. Eng. C Mater. Biol. Appl. 109, 110543.
Calabrese, G., Franco, D., Petralia, S., Monforte, F., Condorelli, G. G., Squarzoni, S., et al. (2021). Dual-Functional Nano-Functionalized Titanium Scaffolds to Inhibit Bacterial Growth and Enhance Osteointegration. Nanomaterials (Basel, Switzerland) 11 (10), 2634. doi:10.3390/nano11102634
Cao, Y., Allameh, S., Nankivil, D., Sethiaraj, S., Otiti, T., and Soboyejo, W. (2006). Nanoindentation Measurements of the Mechanical Properties of Polycrystalline Au and Ag Thin Films on Silicon Substrates: Effects of Grain Size and Film Thickness. Mater. Sci. Eng. A 427 (1-2), 232–240. doi:10.1016/j.msea.2006.04.080
Čapek, J., Kubásek, J., Pinc, J., Fojt, J., Krajewski, S., Rupp, F., et al. (2021). Microstructural, Mechanical, In Vitro Corrosion and Biological Characterization of an Extruded Zn-0.8Mg-0.2Sr (Wt%) as an Absorbable Material. Mater. Sci. Eng. C Mater. Biol. Appl. 122, 111924. doi:10.1016/j.msec.2021.111924
Capek, J., Kubazek, J., Pinc, J., Fojt, J., Krajewski, S., Rupp, F., et al. (2021). Microstructural, Mechanical, In Vitro Corrosion and Biological Characterization of an Extruded Zn-0.8Mg-0.2Sr (Wt%) as an Absorbable Material. Mater. Sci. Eng. C-Materials Biol. Appl. 122, 111924. doi:10.1016/j.msec.2021.111924
Čapek, J., Msallamová, Š., Jablonská, E., Lipov, J., and Vojtěch, D. (2017). A Novel High-Strength and Highly Corrosive Biodegradable Fe-Pd alloy: Structural, Mechanical and In Vitro Corrosion and Cytotoxicity Study. Mater. Sci. Eng. C 79, 550–562. doi:10.1016/j.msec.2017.05.100
Chen, D., He, Y., Tao, H., Zhang, Y., Jiang, Y., Zhang, X., et al. (2011). Biocompatibility of Magnesium-Zinc alloy in Biodegradable Orthopedic Implants. Int. J. Mol. Med. 28 (3), 343–348. doi:10.3892/ijmm.2011.707
Chen, J., Peng, W., Zhu, L., Tan, L., Etim, I. P., Wang, X., et al. (2018). Effect of Copper Content on the Corrosion Behaviors and Antibacterial Properties of Binary Mg-Cu Alloys. Mater. Technology 33 (2), 145–152. doi:10.1080/10667857.2018.1432170
Chen, K., Lu, Y., Tang, H., Gao, Y., Zhao, F., Gu, X., et al. (2020). Effect of Strain on Degradation Behaviors of WE43, Fe and Zn Wires. Acta Biomater. 113, 627–645. doi:10.1016/j.actbio.2020.06.028
Chen, Z.-Y., Gao, S., Zhang, Y.-W., Zhou, R.-B., and Zhou, F. (2021). Antibacterial Biomaterials in Bone Tissue Engineering. J. Mater. Chem. B 9 (11), 2594–2612. doi:10.1039/d0tb02983a
Cipriano, A. F., Zhao, T., Johnson, I., Guan, R.-G., Garcia, S., and Liu, H. (2013). In Vitro degradation of Four Magnesium-Zinc-Strontium Alloys and Their Cytocompatibility with Human Embryonic Stem Cells. J. Mater. Sci. Mater. Med. 24 (4), 989–1003. doi:10.1007/s10856-013-4853-1
Cochis, A., Barberi, J., Ferraris, S., Miola, M., Rimondini, L., Vernè, E., et al. (2020). Competitive Surface Colonization of Antibacterial and Bioactive Materials Doped with Strontium And/or Silver Ions. Nanomaterials (Basel) 10 (1), 120. doi:10.3390/nano10010120
Cockerill, I., Su, Y., Sinha, S., Qin, Y. X., Zheng, Y., Young, M. L., et al. (2020). Porous Zinc Scaffolds for Bone Tissue Engineering Applications: A Novel Additive Manufacturing and Casting Approach. Mater. Sci. Eng. C Mater. Biol. Appl. 110, 110738. doi:10.1016/j.msec.2020.110738
Cyphert, E. L., Zhang, N., Learn, G. D., Hernandez, C. J., and von Recum, H. A. (2021). Recent Advances in the Evaluation of Antimicrobial Materials for Resolution of Orthopedic Implant-Associated Infections In Vivo. ACS Infect. Dis. 7 (12), 3125–3160. doi:10.1021/acsinfecdis.1c00465
Dai, Y., Liu, H., Tang, Y., Xu, X., Long, H., Yan, Y., et al. (2018). A Potential Biodegradable Mg-Y-Ag Implant with Strengthened Antimicrobial Properties in Orthopedic Applications. Metals 8 (11), 948. doi:10.3390/met8110948
Deng, B., Guo, Y., Zhao, M. C., Li, Q. F., Ma, B., Duan, B., et al. (2021). Study on a Novel Biodegradable and Antibacterial Fe-Based Alloy Prepared by Microwave Sintering. Materials (Basel) 14 (14), 3784. doi:10.3390/ma14143784
Farjam, P., Hekman, E. E. G., Rouwkema, J., and Verkerke, G. J. (2022). Bone Fixation Techniques for Managing Joint Disorders and Injuries: A Review Study. J. Mech. Behav. Biomed. Mater. 126, 104982. doi:10.1016/j.jmbbm.2021.104982
Fazel Anvari-Yazdi, A., Tahermanesh, K., Hadavi, S. M. M., Talaei-Khozani, T., Razmkhah, M., Abed, S. M., et al. (2016). Cytotoxicity Assessment of Adipose-Derived Mesenchymal Stem Cells on Synthesized Biodegradable Mg-Zn-Ca Alloys. Mater. Sci. Eng. C 69, 584–597. doi:10.1016/j.msec.2016.07.016
Fei, J., Liu, G.-d., Pan, C.-j., Chen, J.-y., Zhou, Y.-g., Xiao, S.-h., et al. (2011). Preparation, Release Profiles and Antibacterial Properties of Vancomycin-Loaded Ca-P Coating Titanium alloy Plate. J. Mater. Sci. Mater. Med. 22 (4), 989–995. doi:10.1007/s10856-011-4277-8
Feng, Y., Zhu, S., Wang, L., Chang, L., Hou, Y., and Guan, S. (2018). Fabrication and Characterization of Biodegradable Mg-Zn-Y-Nd-Ag alloy: Microstructure, Mechanical Properties, Corrosion Behavior and Antibacterial Activities. Bioactive Mater. 3 (3), 225–235. doi:10.1016/j.bioactmat.2018.02.002
Gao, C., Yao, M., Li, S., Feng, P., Peng, S., and Shuai, C. (2019). Highly Biodegradable and Bioactive Fe-Pd-Bredigite Biocomposites Prepared by Selective Laser Melting. J. Adv. Res. 20, 91–104. doi:10.1016/j.jare.2019.06.001
Gao, Z., Song, M., Liu, R.-L., Shen, Y., Ward, L., Cole, I., et al. (2019). Improving In Vitro and In Vivo Antibacterial Functionality of Mg Alloys through Micro-alloying with Sr and Ga. Mater. Sci. Eng. C 104, 109926. doi:10.1016/j.msec.2019.109926
Garg, D., Matai, I., and Sachdev, A. (2021). Toward Designing of Anti-infective Hydrogels for Orthopedic Implants: From Lab to Clinic. ACS Biomater. Sci. Eng. 7 (6), 1933–1961. doi:10.1021/acsbiomaterials.0c01408
Golafshan, N., Kharaziha, M., and Fathi, M. (2017). Tough and Conductive Hybrid Graphene-PVA: Alginate Fibrous Scaffolds for Engineering Neural Construct. Carbon 111, 752–763. doi:10.1016/j.carbon.2016.10.042
Gomaa, O. M., Costa, N. L., and Paquete, C. M. (2022). Electron Transfer in Gram-Positive Bacteria: Enhancement Strategies for Bioelectrochemical Applications. World J. Microbiol. Biotechnol. 38 (5), 83. doi:10.1007/s11274-022-03255-y
González, S., Pellicer, E., Fornell, J., Blanquer, A., Barrios, L., Ibáñez, E., et al. (2012). Improved Mechanical Performance and Delayed Corrosion Phenomena in Biodegradable Mg-Zn-Ca Alloys through Pd-Alloying. J. Mech. Behav. Biomed. Mater. 6, 53–62. doi:10.1016/j.jmbbm.2011.09.014
Gorejová, R., Haverová, L., Oriňaková, R., and Oriňak, A. (2019). Recent Advancements in Fe-Based Biodegradable Materials for Bone Repair. J. Mater. Sci. 54 (3), 1913–1947. doi:10.1007/s10853-018-3011-z
Gullberg, E., Cao, S., Berg, O. G., Ilbäck, C., Sandegren, L., Hughes, D., et al. (2011). Selection of Resistant Bacteria at Very Low Antibiotic Concentrations. Plos Pathog. 7 (7), e1002158. doi:10.1371/journal.ppat.1002158
Guo, J., Cao, G., Wang, X., Tang, W., Diwu, W., Yan, M., et al. (2021). Coating CoCrMo Alloy with Graphene Oxide and ϵ-Poly-L-Lysine Enhances its Antibacterial and Antibiofilm Properties. Ijn 16, 7249–7268. doi:10.2147/ijn.s321800
Guo, Y., Zhao, M-C., Xie, B., Zhao, Y-C., Yin, D., Gao, C., et al. (2021). In Vitro Corrosion Resistance and Antibacterial Performance of Novel Fe–xCu Biomedical Alloys Prepared by Selective Laser Melting. Adv. Eng. Mater. 23 (4), 1. doi:10.1002/adem.202001000
Guo, Y., Zhao, M.-C., Xie, B., Zhao, Y.-C., Yin, D., Gao, C., et al. (2021). In Vitro Corrosion Resistance and Antibacterial Performance of Novel Fe- X Cu Biomedical Alloys Prepared by Selective Laser Melting. Adv. Eng. Mater. 23 (4), 2001000. doi:10.1002/adem.202001000
Han, P., Tan, M., Zhang, S., Ji, W., Li, J., Zhang, X., et al. (2014). Shape and Site Dependent In Vivo Degradation of Mg-Zn Pins in Rabbit Femoral Condyle. Ijms 15 (2), 2959–2970. doi:10.3390/ijms15022959
He, G., Wu, Y., Zhang, Y., Zhu, Y., Liu, Y., Li, N., et al. (2015). Addition of Zn to the Ternary Mg-Ca-Sr Alloys Significantly Improves Their Antibacterial Properties. J. Mater. Chem. B 3 (32), 6676–6689. doi:10.1039/c5tb01319d
Heiden, M., and Walker, E. (2015). Magnesium, Iron and Zinc Alloys, the Trifecta of Bioresorbable Orthopaedic and Vascular Implantation-A Review. J. Biotechnol. Biomater. 5 (2), 1. doi:10.4172/2155-952X.1000178
Hernández-Escobar, D., Champagne, S., Yilmazer, H., Dikici, B., Boehlert, C. J., and Hermawan, H. (2019). Current Status and Perspectives of Zinc-Based Absorbable Alloys for Biomedical Applications. Acta Biomater. 97, 1–22. doi:10.1016/j.actbio.2019.07.034
Hofstetter, J., Martinelli, E., Pogatscher, S., Schmutz, P., Povoden-Karadeniz, E., Weinberg, A. M., et al. (2015). Influence of Trace Impurities on the In Vitro and In Vivo Degradation of Biodegradable Mg-5Zn-0.3Ca Alloys. Acta Biomater. 23, 347–353. doi:10.1016/j.actbio.2015.05.004
Hou, P., Zhao, C., Cheng, P., Wu, H., Ni, J., Zhang, S., et al. (2016). Reduced Antibacterial Property of Metallic Magnesium In Vivo. Biomed. Mater. 12 (1), 015010. doi:10.1088/1748-605x/12/1/015010
Hu, T., Xu, H., Wang, C., Qin, H., and An, Z. (2018). Magnesium Enhances the Chondrogenic Differentiation of Mesenchymal Stem Cells by Inhibiting Activated Macrophage-Induced Inflammation. Sci. Rep. 8 (1), 3406. doi:10.1038/s41598-018-21783-2
Huang, T., Cheng, J., Bian, D., and Zheng, Y. (2016). Fe-Au and Fe-Ag Composites as Candidates for Biodegradable Stent Materials. J. Biomed. Mater. Res. 104 (2), 225–240. doi:10.1002/jbm.b.33389
Huang, X., Huang, D., Zhu, T., Yu, X., Xu, K., Li, H., et al. (2021). Sustained Zinc Release in Cooperation with CaP Scaffold Promoted Bone Regeneration via Directing Stem Cell Fate and Triggering a Pro-healing Immune Stimuli. J. Nanobiotechnol 19 (1), 207. doi:10.1186/s12951-021-00956-8
Ismat, A., Walter, N., Baertl, S., Mika, J., Lang, S., Kerschbaum, M., et al. (2021). Antibiotic Cement Coating in Orthopedic Surgery: a Systematic Review of Reported Clinical Techniques. J. Orthop. Traumatol. 22 (1), 56. doi:10.1186/s10195-021-00614-7
Jacobs, A., Renaudin, G., Forestier, C., Nedelec, J.-M., and Descamps, S. (2020). Biological Properties of Copper-Doped Biomaterials for Orthopedic Applications: A Review of Antibacterial, Angiogenic and Osteogenic Aspects. Acta Biomater. 117, 21–39. doi:10.1016/j.actbio.2020.09.044
Jähn, K., Saito, H., Taipaleenmäki, H., Gasser, A., Hort, N., Feyerabend, F., et al. (2016). Intramedullary Mg2Ag Nails Augment Callus Formation during Fracture Healing in Mice. Acta Biomater. 36, 350–360. doi:10.1016/j.actbio.2016.03.041
Jiao, J., Zhang, S., Qu, X., and Yue, B. (2021). Recent Advances in Research on Antibacterial Metals and Alloys as Implant Materials. Front. Cel. Infect. Microbiol. 11, 693939. doi:10.3389/fcimb.2021.693939
Kasza, K., Gurnani, P., Hardie, K. R., Cámara, M., and Alexander, C. (2021). Challenges and Solutions in Polymer Drug Delivery for Bacterial Biofilm Treatment: A Tissue-By-Tissue Account. Adv. Drug Deliv. Rev. 178, 113973. doi:10.1016/j.addr.2021.113973
Keskin, D., Zu, G., Forson, A. M., Tromp, L., Sjollema, J., and van Rijn, P. (2021). Nanogels: A Novel Approach in Antimicrobial Delivery Systems and Antimicrobial Coatings. Bioactive Mater. 6 (10), 3634–3657. doi:10.1016/j.bioactmat.2021.03.004
Key, B. M., Symanski, J., Scheidt, M. J., and Tutton, S. M. (2021). Vertebroplasty, Kyphoplasty, and Implant-Based Mechanical Vertebral Augmentation. Semin. Musculoskelet. Radiol. 25 (6), 785–794. doi:10.1055/s-0041-1739531
Khalid, S., Gao, A., Wang, G., Chu, P. K., and Wang, H. (2020). Tuning Surface Topographies on Biomaterials to Control Bacterial Infection. Biomater. Sci. 8 (24), 6840–6857. doi:10.1039/d0bm00845a
Kim, H. Y., Chang, R. Y. K., Morales, S., and Chan, H. K. (2021). Bacteriophage-Delivering Hydrogels: Current Progress in Combating Antibiotic Resistant Bacterial Infection. Antibiotics (Basel) 10 (2), 130. doi:10.3390/antibiotics10020130
Kodetová, V., Smola, B., Stulíková, I., Kudrnová, H., Vlach, M., and Neubert, V. (2019). Mechanical and thermal Properties and Corrosion Behaviour of Heat-Treated Mg–Y–Nd–Ag Alloys. J. Therm. Anal. Calorim. 138 (3), 2167–2174. doi:10.1007/s10973-019-08782-9
Krulwich, T. A., Sachs, G., and Padan, E. (2011). Molecular Aspects of Bacterial pH Sensing and Homeostasis. Nat. Rev. Microbiol. 9 (5), 330–343. doi:10.1038/nrmicro2549
Kumar, R., and Katyal, P. (2021). Effects of Alloying Elements on Performance of Biodegradable Magnesium alloy. Mater. Today Proc. 1, 1. doi:10.1016/j.matpr.2021.08.233
Kumar, S., and Katyal, P. (2022). Factors Affecting Biocompatibility and Biodegradation of Magnesium Based Alloys. Mater. Today Proc. 52, 1092–1107. doi:10.1016/j.matpr.2021.10.499
Kupková, M., Kupka, M., Morovská Turoňová, A., and Oriňaková, R. (2022). Microstructural, Mechanical and Corrosion Characteristics of Degradable PM Biomaterials Made from Copper-Coated Iron Powders. Materials (Basel) 15 (5), 1913. doi:10.3390/ma15051913
Kynaston-Pearson, F., Ashmore, A. M., Malak, T. T., Rombach, I., Taylor, A., Beard, D., et al. (2013). Primary Hip Replacement Prostheses and Their Evidence Base: Systematic Review of Literature. Bmj 347, f6956. doi:10.1136/bmj.f6956
Lemire, J. A., Harrison, J. J., and Turner, R. J. (2013). Antimicrobial Activity of Metals: Mechanisms, Molecular Targets and Applications. Nat. Rev. Microbiol. 11 (6), 371–384. doi:10.1038/nrmicro3028
Li, B., and Webster, T. J. (2018). Bacteria Antibiotic Resistance: New Challenges and Opportunities for Implant-Associated Orthopedic Infections. J. Orthop. Res. 36 (1), 22–32. doi:10.1002/jor.23656
Li, G., Yang, H., Zheng, Y., Chen, X.-H., Yang, J.-A., Zhu, D., et al. (2019). Challenges in the Use of Zinc and its Alloys as Biodegradable Metals: Perspective from Biomechanical Compatibility. Acta Biomater. 97, 23–45. doi:10.1016/j.actbio.2019.07.038
Li, J., Zhai, D., Lv, F., Yu, Q., Ma, H., Yin, J., et al. (2016). Preparation of Copper-Containing Bioactive Glass/eggshell Membrane Nanocomposites for Improving Angiogenesis, Antibacterial Activity and Wound Healing. Acta Biomater. 36, 254–266. doi:10.1016/j.actbio.2016.03.011
Li, P., Schille, C., Schweizer, E., Kimmerle-Müller, E., Rupp, F., Han, X., et al. (2019). Evaluation of a Zn-2Ag-1.8Au-0.2V Alloy for Absorbable Biocompatible Materials. Materials (Basel) 13 (1), 56. doi:10.3390/ma13010056
Li, P., Schille, C., Schweizer, E., Rupp, F., Heiss, A., Legner, C., et al. (2018). Mechanical Characteristics, In Vitro Degradation, Cytotoxicity, and Antibacterial Evaluation of Zn-4.0Ag Alloy as a Biodegradable Material. Int. J. Mol. Sci. 19 (3), 755. doi:10.3390/ijms19030755
Li, P., Schille, C., Schweizer, E., Rupp, F., Heiss, A., Legner, C., et al. (2018). Mechanical Characteristics, In Vitro Degradation, Cytotoxicity, and Antibacterial Evaluation of Zn-4.0Ag Alloy as a Biodegradable Material. Int. J. Mol. Sci. 19 (3), 755. doi:10.3390/ijms19030755
Li, P., Zhang, W., Dai, J., Xepapadeas, A. B., Schweizer, E., Alexander, D., et al. (2019). Investigation of Zinc-copper A-lloys as P-otential M-aterials for C-raniomaxillofacial O-steosynthesis I-mplants. Mater. Sci. Eng. C Mater. Biol. Appl. 103, 109826. doi:10.1016/j.msec.2019.109826
Li, P., Gao, Z., Tan, Z., Xiao, J., Wei, L., and Chen, Y. (2021). New Developments in Anti-biofilm Intervention towards Effective Management of Orthopedic Device Related Infections (ODRI's). Biofouling 37 (1), 1–35. doi:10.1080/08927014.2020.1869725
Li, P., Zhang, W., Dai, J., Xepapadeas, A. B., Schweizer, E., Alexander, D., et al. (2019). Investigation of Zinc-copper A-lloys as P-otential M-aterials for C-raniomaxillofacial O-steosynthesis I-mplants. Mater. Sci. Eng. C 103, 109826. doi:10.1016/j.msec.2019.109826
Li, S., Chen, X., Wang, X., Xiong, Y., Yan, Y., Tan, Z., et al. (2019). Simonkolleite Coating on Poly(Amino Acids) to Improve Osteogenesis and Suppress Osteoclast Formation In Vitro. Polymers (Basel) 11 (9), 1505. doi:10.3390/polym11091505
Li, Y., Liu, G., Zhai, Z., Liu, L., Li, H., Yang, K., et al. (2014). Antibacterial Properties of Magnesium In Vitro and in an In Vivo Model of Implant-Associated Methicillin-Resistant Staphylococcus aureus Infection. Antimicrob. Agents Chemother. 58 (12), 7586–7591. doi:10.1128/aac.03936-14
Li, Y., Liu, L., Wan, P., Zhai, Z., Mao, Z., Ouyang, Z., et al. (2016). Biodegradable Mg-Cu alloy Implants with Antibacterial Activity for the Treatment of Osteomyelitis: In Vitro and In Vivo Evaluations. Biomaterials 106, 250–263. doi:10.1016/j.biomaterials.2016.08.031
Li, Y., Yang, C., Zhao, H., Qu, S., Li, X., and Li, Y. (2014). New Developments of Ti-Based Alloys for Biomedical Applications. Materials 7 (3), 1709–1800. doi:10.3390/ma7031709
Lin, J., Tong, X., Shi, Z., Zhang, D., Zhang, L., Wang, K., et al. (2020). A Biodegradable Zn-1Cu-0.1Ti alloy with Antibacterial Properties for Orthopedic Applications. Acta Biomater. 106, 410–427. doi:10.1016/j.actbio.2020.02.017
Lin, J., Tong, X., Wang, K., Shi, Z., Li, Y., Dargusch, M., et al. (2021). Biodegradable Zn-3Cu and Zn-3Cu-0.2Ti Alloys with Ultrahigh Ductility and Antibacterial Ability for Orthopedic Applications. J. Mater. Sci. Technology 68, 76–90. doi:10.1016/j.jmst.2020.06.052
Liu, B., and Zheng, Y. F. (2011). Effects of Alloying Elements (Mn, Co, Al, W, Sn, B, C and S) on Biodegradability and In Vitro Biocompatibility of Pure Iron. Acta Biomater. 7 (3), 1407–1420. doi:10.1016/j.actbio.2010.11.001
Liu, C., Fu, X., Pan, H., Wan, P., Wang, L., Tan, L., et al. (2016). Biodegradable Mg-Cu Alloys with Enhanced Osteogenesis, Angiogenesis, and Long-Lasting Antibacterial Effects. Sci. Rep. 6, 27374. doi:10.1038/srep27374
Liu, C., Wan, P., Tan, L. L., Wang, K., and Yang, K. (2014). Preclinical Investigation of an Innovative Magnesium-Based Bone Graft Substitute for Potential Orthopaedic Applications. J. Orthopaedic Translation 2 (3), 139–148. doi:10.1016/j.jot.2014.06.002
Liu, H., Li, D., Zhang, Y., and Li, M. (2018). Inflammation, Mesenchymal Stem Cells and Bone Regeneration. Histochem. Cel Biol 149 (4), 393–404. doi:10.1007/s00418-018-1643-3
Liu, R.-Y., He, R.-G., Xu, L.-Q., and Guo, S.-F. (2018). Design of Fe-Mn-Ag Alloys as Potential Candidates for Biodegradable Metals. Acta Metall. Sin. (Engl. Lett. 31 (6), 584–590. doi:10.1007/s40195-018-0702-z
Liu, W., Wang, J., Jiang, G., Guo, J., Li, Q., Li, B., et al. (2017). The Improvement of Corrosion Resistance, Biocompatibility and Osteogenesis of the Novel Porous Mg-Nd-Zn alloy. J. Mater. Chem. B 5 (36), 7661–7674. doi:10.1039/c7tb00920h
Liu, Z., Schade, R., Luthringer, B., Hort, N., Rothe, H., Müller, S., et al. (2017). Influence of the Microstructure and Silver Content on Degradation, Cytocompatibility, and Antibacterial Properties of Magnesium-Silver Alloys In Vitro. Oxid Med. Cel Longev 2017, 8091265. doi:10.1155/2017/8091265
Loo, C. Y., Corliss, D. A., and Ganeshkumar, N. (2000). Streptococcus Gordonii Biofilm Formation: Identification of Genes that Code for Biofilm Phenotypes. J. Bacteriol. 182 (5), 1374–1382. doi:10.1128/jb.182.5.1374-1382.2000
Lu, X., Wu, Z., Xu, K., Wang, X., Wang, S., Qiu, H., et al. (2021). Multifunctional Coatings of Titanium Implants toward Promoting Osseointegration and Preventing Infection: Recent Developments. Front. Bioeng. Biotechnol. 9, 783816. doi:10.3389/fbioe.2021.783816
Luo, X., Barbieri, D., Davison, N., Yan, Y., de Bruijn, J. D., and Yuan, H. (2014). Zinc in Calcium Phosphate Mediates Bone Induction: In Vitro and In Vivo Model. Acta Biomater. 10 (1), 477–485. doi:10.1016/j.actbio.2013.10.011
Luque-Agudo, V., Fernández-Calderón, M. C., Pacha-Olivenza, M. A., Pérez-Giraldo, C., Gallardo-Moreno, A. M., and González-Martín, M. L. (2020). The Role of Magnesium in Biomaterials Related Infections. Colloids Surf. B: Biointerfaces 191, 110996. doi:10.1016/j.colsurfb.2020.110996
Ma, Y., Liu, J., Yin, H., Xu, X., Xie, Y., Chen, D., et al. (2018). Remarkably Improvement in Antibacterial Activity of Carbon Nanotubes by Hybridizing with Silver Nanodots. j nanosci nanotechnol 18 (8), 5704–5710. doi:10.1166/jnn.2018.15383
Makarov, C., Berdicevsky, I., Raz-Pasteur, A., and Gotman, I. (2013). In Vitro antimicrobial Activity of Vancomycin-Eluting Bioresorbable β-TCP-polylactic Acid Nanocomposite Material for Load-Bearing Bone Repair. J. Mater. Sci. Mater. Med. 24 (3), 679–687. doi:10.1007/s10856-012-4832-y
Mandal, S., Kishore, V., Bose, M., Nandi, S. K., and Roy, M. (2021). In Vitro and In Vivo Degradability, Biocompatibility and Antimicrobial Characteristics of Cu Added Iron-Manganese alloy. J. Mater. Sci. Technology 84, 159–172. doi:10.1016/j.jmst.2020.12.029
Mandal, S., Ummadi, R., Bose, M., Balla, V. K., and Roy, M. (2019). Fe-Mn-Cu alloy as Biodegradable Material with Enhanced Antimicrobial Properties. Mater. Lett. 237, 323–327. doi:10.1016/j.matlet.2018.11.117
Marambio-Jones, C., and Hoek, E. M. V. (2010). A Review of the Antibacterial Effects of Silver Nanomaterials and Potential Implications for Human Health and the Environment. J. Nanopart Res. 12 (5), 1531–1551. doi:10.1007/s11051-010-9900-y
Masters, E. A., Ricciardi, B. F., Bentley, K. L. M., Moriarty, T. F., Schwarz, E. M., and Muthukrishnan, G. (2022). Skeletal Infections: Microbial Pathogenesis, Immunity and Clinical Management. Nat. Rev. Microbiol. 1, 1–16. doi:10.1038/s41579-022-00686-0
Miao, H., Zhang, D., Chen, C., Zhang, L., Pei, J., Su, Y., et al. (2019). Research on Biodegradable Mg-Zn-Gd Alloys for Potential Orthopedic Implants: In Vitro and In Vivo Evaluations. ACS Biomater. Sci. Eng. 5 (3), 1623–1634. doi:10.1021/acsbiomaterials.8b01563
Mitra, D., Kang, E.-T., and Neoh, K. G. (2020). Antimicrobial Copper-Based Materials and Coatings: Potential Multifaceted Biomedical Applications. ACS Appl. Mater. Inter. 12 (19), 21159–21182. doi:10.1021/acsami.9b17815
Moniri Javadhesari, S., Alipour, S., and Akbarpour, M. R. (2020). Biocompatibility, Osseointegration, Antibacterial and Mechanical Properties of Nanocrystalline Ti-Cu alloy as a New Orthopedic Material. Colloids Surf. B: Biointerfaces 189, 110889. doi:10.1016/j.colsurfb.2020.110889
Montanaro, L., Speziale, P., Campoccia, D., Ravaioli, S., Cangini, I., Pietrocola, G., et al. (2011). Scenery of Staphylococcus Implant Infections in Orthopedics. Future Microbiol. 6 (11), 1329–1349. doi:10.2217/fmb.11.117
Nag, M., Lahiri, D., Sarkar, T., Ghosh, S., Dey, A., Edinur, H. A., et al. (2021). Microbial Fabrication of Nanomaterial and its Role in Disintegration of Exopolymeric Matrices of Biofilm. Front. Chem. 9, 690590. doi:10.3389/fchem.2021.690590
Narayana, P. S. V. V. S., and Srihari, P. S. V. V. (2019). A Review on Surface Modifications and Coatings on Implants to Prevent Biofilm. Regenerative Eng. Translational Med. 6 (3), 330–346.
Noukrati, H., Cazalbou, S., Demnati, I., Rey, C., Barroug, A., and Combes, C. (2016). Injectability, Microstructure and Release Properties of Sodium Fusidate-Loaded Apatitic Cement as a Local Drug-Delivery System. Mater. Sci. Eng. C 59, 177–184. doi:10.1016/j.msec.2015.09.070
Oliva, A., Miele, M. C., Al Ismail, D., Di Timoteo, F., De Angelis, M., Rosa, L., et al. (2021). Challenges in the Microbiological Diagnosis of Implant-Associated Infections: A Summary of the Current Knowledge. Front. Microbiol. 12, 750460. doi:10.3389/fmicb.2021.750460
Park, H.-J., Kim, J. Y., Kim, J., Lee, J.-H., Hahn, J.-S., Gu, M. B., et al. (2009). Silver-ion-mediated Reactive Oxygen Species Generation Affecting Bactericidal Activity. Water Res. 43 (4), 1027–1032. doi:10.1016/j.watres.2008.12.002
Peng, F., Cheng, S., Zhang, R., Li, M., Zhou, J., Wang, D., et al. (2021). Zn-contained Mussel-Inspired Film on Mg alloy for Inhibiting Bacterial Infection and Promoting Bone Regeneration. Regen. Biomater. 8 (1), rbaa044. doi:10.1093/rb/rbaa044
Peng, F., Lin, Y., Zhang, D., Ruan, Q., Tang, K., Li, M., et al. (2021). Corrosion Behavior and Biocompatibility of Diamond-like Carbon-Coated Zinc: An In Vitro Study. ACS omega 6 (14), 9843–9851. doi:10.1021/acsomega.1c00531
Pfang, B. G., García-Cañete, J., García-Lasheras, J., Blanco, A., Auñón, Á., Parron-Cambero, R., et al. (2019). Orthopedic Implant-Associated Infection by Multidrug Resistant Enterobacteriaceae. J. Clin. Med. 8 (2), 220. doi:10.3390/jcm8020220
Prakash, C., Singh, S., Gupta, M. K., Mia, M., Królczyk, G., and Khanna, N. (2018). Synthesis, Characterization, Corrosion Resistance and In-Vitro Bioactivity Behavior of Biodegradable Mg⁻Zn⁻Mn⁻(Si⁻HA) Composite for Orthopaedic Applications. Materials (Basel) 11 (9), 1602. doi:10.3390/ma11091602
Purniawan, A., Maulidiah, H. M., and Purwaningsih, H. (2018). Influence of Copper Composition on Mechanical Properties of Biodegradable Material Mg-Zn-Cu for Orthopedic Application. AIP Conf. Proc. 1945, 020010. doi:10.1063/1.5030232
Qin, H., Zhao, Y., An, Z., Cheng, M., Wang, Q., Cheng, T., et al. (2015). Enhanced Antibacterial Properties, Biocompatibility, and Corrosion Resistance of Degradable Mg-Nd-Zn-Zr alloy. Biomaterials 53, 211–220. doi:10.1016/j.biomaterials.2015.02.096
Qin, H., Zhao, Y., Cheng, M., Wang, Q., Wang, Q., Wang, J., et al. (2015). Anti-biofilm Properties of Magnesium Metal via Alkaline pH. RSC Adv. 5 (28), 21434–21444. doi:10.1039/c5ra00027k
Qu, X., Yang, H., Jia, B., Wang, M., Yue, B., Zheng, Y., et al. (2021). Zinc alloy-based Bone Internal Fixation Screw with Antibacterial and Anti-osteolytic Properties. Bioactive Mater. 6 (12), 4607–4624. doi:10.1016/j.bioactmat.2021.05.023
Qu, X., Yang, H., Jia, B., Yu, Z., Zheng, Y., and Dai, K. (2020). Biodegradable Zn-Cu Alloys Show Antibacterial Activity against MRSA Bone Infection by Inhibiting Pathogen Adhesion and Biofilm Formation. Acta Biomater. 117, 400–417. doi:10.1016/j.actbio.2020.09.041
Rahim, M. I., Eifler, R., Rais, B., and Mueller, P. P. (2015). Alkalization Is Responsible for Antibacterial Effects of Corroding Magnesium. J. Biomed. Mater. Res. 103 (11), 3526–3532. doi:10.1002/jbm.a.35503
Rahim, M. I., Rohde, M., Rais, B., Seitz, J.-M., and Mueller, P. P. (2016). Susceptibility of Metallic Magnesium Implants to Bacterial Biofilm Infections. J. Biomed. Mater. Res. 104 (6), 1489–1499. doi:10.1002/jbm.a.35680
Rai, A., Ferrao, R., Palma, P., Patricio, T., Parreira, P., Anes, E., et al. (2022). Antimicrobial Peptide-Based Materials: Opportunities and Challenges. J. Mater. Chem. B 10, 2384. doi:10.1039/d1tb02617h
Razavi, M., and Huang, Y. (2019). Assessment of Magnesium-Based Biomaterials: from Bench to Clinic. Biomater. Sci. 7 (6), 2241–2263. doi:10.1039/c9bm00289h
Ren, L., Wong, H. M., Yan, C. H., Yeung, K. W. K., and Yang, K. (2015). Osteogenic Ability of Cu-Bearing Stainless Steel. J. Biomed. Mater. Res. 103 (7), 1433–1444. doi:10.1002/jbm.b.33318
Ren, L., and Yang, K. (2017). “Antibacterial Design for Metal Implants,” in Metallic Foam Bone (Elsevier), 203–216. doi:10.1016/b978-0-08-101289-5.00008-1
Resnik, M., Benčina, M., Levičnik, E., Rawat, N., Iglič, A., and Junkar, I. (2020). Strategies for Improving Antimicrobial Properties of Stainless Steel. Materials (Basel) 13 (13), 2944. doi:10.3390/ma13132944
Robinson, D. A., Griffith, R. W., Shechtman, D., Evans, R. B., and Conzemius, M. G. (2010). In Vitro antibacterial Properties of Magnesium Metal against Escherichia coli, Pseudomonas aeruginosa and Staphylococcus aureus☆. Acta Biomater. 6 (5), 1869–1877. doi:10.1016/j.actbio.2009.10.007
Safari, N., Toroghinejad, M. R., and Kharaziha, M. (2019). Influence of Copper on the Structural, Mechanical, and Biological Characteristics of Mg–1Al–Cu alloy. Mater. Chem. Phys. 237, 121838. doi:10.1016/j.matchemphys.2019.121838
Schinhammer, M., Hänzi, A. C., Löffler, J. F., and Uggowitzer, P. J. (2010). Design Strategy for Biodegradable Fe-Based Alloys for Medical Applications☆. Acta Biomater. 6 (5), 1705–1713. doi:10.1016/j.actbio.2009.07.039
Schmidt-Bleek, K., Schell, H., Schulz, N., Hoff, P., Perka, C., Buttgereit, F., et al. (2012). Inflammatory Phase of Bone Healing Initiates the Regenerative Healing cascade. Cell Tissue Res 347 (3), 567–573. doi:10.1007/s00441-011-1205-7
Sendi, P., Banderet, F., Graber, P., and Zimmerli, W. (2011). Periprosthetic Joint Infection Following Staphylococcus aureus Bacteremia. J. Infect. 63 (1), 17–22. doi:10.1016/j.jinf.2011.05.005
Seyedraoufi, Z. S., and Mirdamadi, S. (2013). Synthesis, Microstructure and Mechanical Properties of Porous Mg‐ ‐Zn Scaffolds. J. Mech. Behav. Biomed. Mater. 21, 1–8. doi:10.1016/j.jmbbm.2013.01.023
Seyhan, M., Guler, O., Mahirogullari, M., Donmez, F., Gereli, A., and Mutlu, S. (2018). Complications during Removal of Stainless Steel versus Titanium Nails Used for Intramedullary Nailing of Diaphyseal Fractures of the Tibia. Ann. Med. Surg. (Lond) 26, 38–42. doi:10.1016/j.amsu.2017.12.012
Shahin, M., Munir, K., Wen, C., and Li, Y. (2019). Magnesium Matrix Nanocomposites for Orthopedic Applications: A Review from Mechanical, Corrosion, and Biological Perspectives. Acta Biomater. 96, 1–19. doi:10.1016/j.actbio.2019.06.007
Shi, L., Dong, H., Reguera, G., Beyenal, H., Lu, A., Liu, J., et al. (2016). Extracellular Electron Transfer Mechanisms between Microorganisms and Minerals. Nat. Rev. Microbiol. 14 (10), 651–662. doi:10.1038/nrmicro.2016.93
Shi, Z.-Z., Yu, J., Liu, X.-F., Zhang, H.-J., Zhang, D.-W., Yin, Y.-X., et al. (2019). Effects of Ag, Cu or Ca Addition on Microstructure and Comprehensive Properties of Biodegradable Zn-0.8Mn alloy. Mater. Sci. Eng. C 99, 969–978. doi:10.1016/j.msec.2019.02.044
Shuai, C., Zhou, Y., Yang, Y., Gao, C., Peng, S., and Wang, G. (2018). Ag-Introduced Antibacterial Ability and Corrosion Resistance for Bio-Mg Alloys. Biomed. Res. Int. 2018, 6023460. doi:10.1155/2018/6023460
Shuai, C., Dong, Z., He, C., Yang, W., Peng, S., Yang, Y., et al. (2020). A Peritectic Phase Refines the Microstructure and Enhances Zn Implants. J. Mater. Res. Technology 9 (3), 2623–2634. doi:10.1016/j.jmrt.2020.04.037
Shuai, C., Liu, L., Zhao, M., Feng, P., Yang, Y., Guo, W., et al. (2018). Microstructure, Biodegradation, Antibacterial and Mechanical Properties of ZK60-Cu Alloys Prepared by Selective Laser Melting Technique. J. Mater. Sci. Technology 34 (10), 1944–1952. doi:10.1016/j.jmst.2018.02.006
Sikora-Jasinska, M., Mostaed, E., Mostaed, A., Beanland, R., Mantovani, D., and Vedani, M. (2017). Fabrication, Mechanical Properties and In Vitro Degradation Behavior of Newly Developed Zn Ag Alloys for Degradable Implant Applications. Mater. Sci. Eng. C 77, 1170–1181. doi:10.1016/j.msec.2017.04.023
Solomons, N. W. (2013). Update on Zinc Biology. Ann. Nutr. Metab. 62 (Suppl. 1), 8–17. doi:10.1159/000348547
Song, X., Chang, L., Wang, J., Zhu, S., Wang, L., Feng, K., et al. (2018). Investigation on the In Vitro Cytocompatibility of Mg-Zn-Y-Nd-Zr Alloys as Degradable Orthopaedic Implant Materials. J. Mater. Sci. Mater. Med. 29 (4), 44. doi:10.1007/s10856-018-6050-8
Sotoudehbagha, P., Sheibani, S., Khakbiz, M., Ebrahimi-Barough, S., and Hermawan, H. (2018). Novel Antibacterial Biodegradable Fe-Mn-Ag Alloys Produced by Mechanical Alloying. Mater. Sci. Eng. C 88, 88–94. doi:10.1016/j.msec.2018.03.005
Sumner, D. R. (2015). Long-term Implant Fixation and Stress-Shielding in Total Hip Replacement. J. Biomech. 48 (5), 797–800. doi:10.1016/j.jbiomech.2014.12.021
Sun, J., Zhang, X., Shi, Z. Z., Gao, X. X., Liu, X. F., Wang, J. Q., et al. (2020). Adjusting Comprehensive Properties of Biodegradable Zn-Mn alloy through Solution Heat-Treatment. Mater. Today Commun. 23, 101150. doi:10.1016/j.mtcomm.2020.101150
Szymański, P., Frączek, T., Markowicz, M., and Mikiciuk-Olasik, E. (2012). Development of Copper Based Drugs, Radiopharmaceuticals and Medical Materials. Biometals : Int. J. role metal ions Biol. Biochem. Med. 25 (6), 1089–1112.
Tian, X., Lu, Z., Ma, C., Wu, M., Zhang, C., Yuan, Y., et al. (2021). Antimicrobial Hydroxyapatite and its Composites for the Repair of Infected Femoral Condyle. Mater. Sci. Eng. C 121, 111807. doi:10.1016/j.msec.2020.111807
Tie, D., Feyerabend, F., Feyerabend, F., Müller, W.-D., Schade, R., Liefeith, K., et al. (2013). Antibacterial Biodegradable Mg-Ag Alloys. eCM 25, 284–298. doi:10.22203/ecm.v025a20
Tie, D., Feyerabend, F., Hort, N., Hoeche, D., Kainer, K. U., Willumeit, R., et al. (2014). In Vitro mechanical and Corrosion Properties of Biodegradable Mg-Ag Alloys. Mater. Corrosion 65 (6), 569–576. doi:10.1002/maco.201206903
Tie, D., Hort, N., Chen, M., Guan, R., Ulasevich, S., Skorb, E. V., et al. (2022). In Vivo urinary Compatibility of Mg-Sr-Ag alloy in Swine Model. Bioactive Mater. 7, 254–262. doi:10.1016/j.bioactmat.2021.05.046
Tong, X., Shi, Z., Xu, L., Lin, J., Zhang, D., Wang, K., et al. (2020). Degradation Behavior, Cytotoxicity, Hemolysis, and Antibacterial Properties of Electro-Deposited Zn-Cu Metal Foams as Potential Biodegradable Bone Implants. Acta Biomater. 102, 481–492. doi:10.1016/j.actbio.2019.11.031
Trampuz, A., and Widmer, A. F. (2006). Infections Associated with Orthopedic Implants. Curr. Opin. Infect. Dis. 19 (4), 349–356. doi:10.1097/01.qco.0000235161.85925.e8
Tran, N., Kelley, M. N., Tran, P. A., Garcia, D. R., Jarrell, J. D., Hayda, R. A., et al. (2015). Silver Doped Titanium Oxide-PDMS Hybrid Coating Inhibits Staphylococcus aureus and Staphylococcus Epidermidis Growth on PEEK. Mater. Sci. Eng. C 49, 201–209. doi:10.1016/j.msec.2014.12.072
Velard, F., Laurent-Maquin, D., Braux, J., Guillaume, C., Bouthors, S., Jallot, E., et al. (2010). The Effect of Zinc on Hydroxyapatite-Mediated Activation of Human Polymorphonuclear Neutrophils and Bone Implant-Associated Acute Inflammation. Biomaterials 31 (8), 2001–2009. doi:10.1016/j.biomaterials.2009.11.066
Venezuela, J., and Dargusch, M. S. (2019). The Influence of Alloying and Fabrication Techniques on the Mechanical Properties, Biodegradability and Biocompatibility of Zinc: A Comprehensive Review. Acta Biomater. 87, 1–40. doi:10.1016/j.actbio.2019.01.035
Vincent, M., Duval, R. E., Hartemann, P., and Engels‐Deutsch, M. (2018). Contact Killing and Antimicrobial Properties of Copper. J. Appl. Microbiol. 124 (5), 1032–1046. doi:10.1111/jam.13681
Vlcek, M., Lukac, F., Kudrnova, H., Smola, B., Stulikova, I., Luczak, M., et al. (2017). Microhardness and In Vitro Corrosion of Heat-Treated Mg-Y-Ag Biodegradable Alloy. Materials (Basel) 10 (1), 55. doi:10.3390/ma10010055
Wagner, C., and Hänsch, G. M. (2017). Mechanisms of Bacterial Colonization of Implants and Host Response. Adv. Exp. Med. Biol. 971, 15–27. doi:10.1007/5584_2016_173
Wang, G., Feng, H., Hu, L., Jin, W., Hao, Q., Gao, A., et al. (2018). An Antibacterial Platform Based on Capacitive Carbon-Doped TiO2 Nanotubes after Direct or Alternating Current Charging. Nat. Commun. 9 (1), 2055. doi:10.1038/s41467-018-04317-2
Wang, S., Li, R., Xia, D., Zhao, X., Zhu, Y., Gu, R., et al. (2021). The Impact of Zn-Doped Synthetic Polymer Materials on Bone Regeneration: a Systematic Review. Stem Cel Res Ther 12 (1), 123. doi:10.1186/s13287-021-02195-y
Wang, X., Liu, S., Li, M., Yu, P., Chu, X., Li, L., et al. (2016). The Synergistic Antibacterial Activity and Mechanism of Multicomponent Metal Ions-Containing Aqueous Solutions against Staphylococcus aureus. J. Inorg. Biochem. 163, 214–220. doi:10.1016/j.jinorgbio.2016.07.019
Wang, X., Shao, X., Dai, T., Xu, F., Zhou, J. G., Qu, G., et al. (2019). In Vivo study of the Efficacy, Biosafety, and Degradation of a Zinc alloy Osteosynthesis System. Acta Biomater. 92, 351–361. doi:10.1016/j.actbio.2019.05.001
Wang, Z., Wang, X., Wang, Y., Zhu, Y., Liu, X., and Zhou, Q. (2021). NanoZnO-modified Titanium Implants for Enhanced Anti-bacterial Activity, Osteogenesis and Corrosion Resistance. J. Nanobiotechnol 19 (1), 353. doi:10.1186/s12951-021-01099-6
Watanabe, K., Fukuzaki, S., Sugino, A., Benson, N., Metcalf, N., Nakamura, M., et al. (2021). Cobalt-Chromium Alloy Has Superior Antibacterial Effect Than Titanium Alloy. Spine 46 (17), E911–e915. doi:10.1097/brs.0000000000003970
Wątroba, M., Bednarczyk, W., Kawałko, J., Mech, K., Marciszko, M., Boelter, G., et al. (2019). Design of Novel Zn-Ag-Zr alloy with Enhanced Strength as a Potential Biodegradable Implant Material. Mater. Des. 183, 108154. doi:10.1016/j.matdes.2019.108154
Wu, C., Zhou, Y., Xu, M., Han, P., Chen, L., Chang, J., et al. (2013). Copper-containing Mesoporous Bioactive Glass Scaffolds with Multifunctional Properties of Angiogenesis Capacity, Osteostimulation and Antibacterial Activity. Biomaterials 34 (2), 422–433. doi:10.1016/j.biomaterials.2012.09.066
Wu, H., Xie, X., Wang, J., Ke, G., Huang, H., Liao, Y., et al. (2021). Biological Properties of Zn-0.04Mg-2Ag: a New Degradable Zinc alloy Scaffold for Repairing Large-Scale Bone Defects. J. Mater. Res. Technology 13, 1779–1789. doi:10.1016/j.jmrt.2021.05.096
Xiao, C., Shi, X. Y., Yu, W. T., Wei, X. W., Cheng, L. L., Qiu, X., et al. (2021). In Vivo biocompatibility Evaluation of Zn-0.05Mg-(0, 0.5, 1wt%)Ag Implants in New Zealand Rabbits. Mater. Sci. Eng. C-Materials Biol. Appl. 119, 111435. doi:10.1016/j.msec.2020.111435
Xiao, C., Su, Y., Zhu, X., Yu, W., Cui, D., Wei, X., et al. (2020). Mechanical Performance and Biocompatibility Assessment of Zn-0.05wt%Mg-(0.5, 1 Wt%) Ag Alloys. J. Biomed. Mater. Res. B Appl. Biomater. 108 (7), 2925–2936. doi:10.1002/jbm.b.34623
Xiao, C., Wang, L., Ren, Y., Sun, S., Zhang, E., Yan, C., et al. (2018). Indirectly Extruded Biodegradable Zn-0.05wt%Mg alloy with Improved Strength and Ductility: In Vitro and In Vivo Studies. J. Mater. Sci. Technology 34 (9), 1618–1627. doi:10.1016/j.jmst.2018.01.006
Xiao, C., Zhao, D., Sun, Q., Su, Y., Cui, D., Zhang, X., et al. (2019). Morphology and Mechanical, Corrosive, and Antibacterial Behaviors of Indirectly Extruded Zn-0.05 wt.% Mg-(0.5, 1 wt.%) Ag Alloys. J. Mater. Eng. Perform. 28 (11), 6864–6872. doi:10.1007/s11665-019-04297-4
Xiao, X., Liu, E., Shao, J., and Ge, S. (2021). Advances on Biodegradable Zinc-Silver-Based Alloys for Biomedical Applications. J. Appl. Biomater. Funct. Mater. 19, 22808000211062407. doi:10.1177/22808000211062407
Xie, K., Wang, N., Guo, Y., Zhao, S., Tan, J., Wang, L., et al. (2022). Additively Manufactured Biodegradable Porous Magnesium Implants for Elimination of Implant-Related Infections: An In Vitro and In Vivo Study. Bioactive Mater. 8, 140–152. doi:10.1016/j.bioactmat.2021.06.032
Xie, Y., Zhao, L., Zhang, Z., Wang, X., Wang, R., and Cui, C. (2018). Fabrication and Properties of Porous Zn-Ag alloy Scaffolds as Biodegradable Materials. Mater. Chem. Phys. 219, 433–443. doi:10.1016/j.matchemphys.2018.08.023
Xu, R., Zhao, M.-C., Zhao, Y.-C., Liu, L., Liu, C., Gao, C., et al. (2019). Improved Biodegradation Resistance by Grain Refinement of Novel Antibacterial ZK30-Cu Alloys Produced via Selective Laser Melting. Mater. Lett. 237, 253–257. doi:10.1016/j.matlet.2018.11.071
Xu, Z.-L., Lei, Y., Yin, W.-J., Chen, Y.-X., Ke, Q.-F., Guo, Y.-P., et al. (2016). Enhanced Antibacterial Activity and Osteoinductivity of Ag-Loaded Strontium Hydroxyapatite/chitosan Porous Scaffolds for Bone Tissue Engineering. J. Mater. Chem. B 4 (48), 7919–7928. doi:10.1039/c6tb01282e
Xue, T., Attarilar, S., Liu, S., Liu, J., Song, X., Li, L., et al. (2020). Surface Modification Techniques of Titanium and its Alloys to Functionally Optimize Their Biomedical Properties: Thematic Review. Front. Bioeng. Biotechnol. 8, 603072. doi:10.3389/fbioe.2020.603072
Yamanaka, K., Mori, M., and Chiba, A. (2013). Nanoarchitectured Co-cr-mo Orthopedic Implant Alloys: Nitrogen-Enhanced Nanostructural Evolution and its Effect on Phase Stability. Acta Biomater. 9 (4), 6259–6267. doi:10.1016/j.actbio.2012.12.013
Yan, X., Wan, P., Tan, L., Zhao, M., Qin, L., and Yang, K. (2018). Corrosion and Biological Performance of Biodegradable Magnesium Alloys Mediated by Low Copper Addition and Processing. Mater. Sci. Eng. C 93, 565–581. doi:10.1016/j.msec.2018.08.013
Yan, X., Wan, P., Tan, L., Zhao, M., Shuai, C., and Yang, K. (2018). Influence of Hybrid Extrusion and Solution Treatment on the Microstructure and Degradation Behavior of Mg-0.1Cu alloy. Mater. Sci. Eng. B 229, 105–117. doi:10.1016/j.mseb.2017.12.033
Yan, X., Zhao, M.-C., Yang, Y., Tan, L., Zhao, Y.-C., Yin, D.-F., et al. (2019). Improvement of Biodegradable and Antibacterial Properties by Solution Treatment and Micro-arc Oxidation (MAO) of a Magnesium alloy with a Trace of Copper. Corrosion Sci. 156, 125–138. doi:10.1016/j.corsci.2019.05.015
Yang, J., Qin, H., Chai, Y., Zhang, P., Chen, Y., Yang, K., et al. (2021). Molecular Mechanisms of Osteogenesis and Antibacterial Activity of Cu-Bearing Ti alloy in a Bone Defect Model with Infection In Vivo. J. orthopaedic translation 27, 77–89. doi:10.1016/j.jot.2020.10.004
Yang, Y. W., Yang, M. L., He, C. X., Qi, F. W., Wang, D., Peng, S. P., et al. (2021). Rare Earth Improves Strength and Creep Resistance of Additively Manufactured Zn Implants. Composites B-Engineering 216, 108882. doi:10.1016/j.compositesb.2021.108882
Yu, W., Chen, D., Ding, Z., Qiu, M., Zhang, Z., Shen, J., et al. (2016). Synergistic Effect of a Biodegradable Mg-Zn alloy on Osteogenic Activity and Anti-biofilm Ability: an In Vitro and In Vivo Study. RSC Adv. 6 (51), 45219–45230. doi:10.1039/c6ra03998g
Yuan, W., Xia, D., Wu, S., Zheng, Y., Guan, Z., and Rau, J. V. (2022). A Review on Current Research Status of the Surface Modification of Zn-Based Biodegradable Metals. Bioactive Mater. 7, 192–216. doi:10.1016/j.bioactmat.2021.05.018
Zhang, C., Lin, J., Nguyen, N.-Y. T., Guo, Y., Xu, C., Seo, C., et al. (2020). Antimicrobial Bioresorbable Mg-Zn-Ca Alloy for Bone Repair in a Comparison Study with Mg-Zn-Sr Alloy and Pure Mg. ACS Biomater. Sci. Eng. 6 (1), 517–538. doi:10.1021/acsbiomaterials.9b00903
Zhang, E., Wang, X., Chen, M., and Hou, B. (2016). Effect of the Existing Form of Cu Element on the Mechanical Properties, Bio-Corrosion and Antibacterial Properties of Ti-Cu Alloys for Biomedical Application. Mater. Sci. Eng. C 69, 1210–1221. doi:10.1016/j.msec.2016.08.033
Zhang, E., Zhao, X., Hu, J., Wang, R., Fu, S., and Qin, G. (2021). Antibacterial Metals and Alloys for Potential Biomedical Implants. Bioactive Mater. 6 (8), 2569–2612. doi:10.1016/j.bioactmat.2021.01.030
Zhang, Q., Xue, Y., Fu, Y., Bao, B., and Guo, M. Y. (2021). Zinc Deficiency Aggravates Oxidative Stress Leading to Inflammation and Fibrosis in Lung of Mice. Biol. trace Elem. Res. 1, 1. doi:10.1007/s12011-021-03011-7
Zhang, S., Zhang, X., Zhao, C., Li, J., Song, Y., Xie, C., et al. (2010). Research on an Mg-Zn alloy as a Degradable Biomaterial. Acta Biomater. 6 (2), 626–640. doi:10.1016/j.actbio.2009.06.028
Zhang, W., Li, P., Shen, G., Mo, X., Zhou, C., Alexander, D., et al. (2021). Appropriately Adapted Properties of Hot-Extruded Zn-0.5Cu-xFe Alloys Aimed for Biodegradable Guided Bone Regeneration Membrane Application. Bioactive Mater. 6 (4), 975–989. doi:10.1016/j.bioactmat.2020.09.019
Zhang, X., Ba, Z., Wang, Z., He, X., Shen, C., and Wang, Q. (2013). Influence of Silver Addition on Microstructure and Corrosion Behavior of Mg-Nd-Zn-Zr Alloys for Biomedical Application. Mater. Lett. 100, 188–191. doi:10.1016/j.matlet.2013.03.061
Zhang, X., Zu, H., Zhao, D., Yang, K., Tian, S., Yu, X., et al. (2017). Ion Channel Functional Protein Kinase TRPM7 Regulates Mg Ions to Promote the Osteoinduction of Human Osteoblast via PI3K Pathway: In Vitro Simulation of the Bone-Repairing Effect of Mg-Based alloy Implant. Acta Biomater. 63, 369–382. doi:10.1016/j.actbio.2017.08.051
Zhao, C., Wu, H., Hou, P., Ni, J., Han, P., and Zhang, X. (2016). Enhanced Corrosion Resistance and Antibacterial Property of Zn Doped DCPD Coating on Biodegradable Mg. Mater. Lett. 180, 42–46. doi:10.1016/j.matlet.2016.04.035
Zhao, J., Zhai, Z., Sun, D., Yang, C., Zhang, X., Huang, N., et al. (2019). Antibacterial Durability and Biocompatibility of Antibacterial-Passivated 316L Stainless Steel in Simulated Physiological Environment. Mater. Sci. Eng. C 100, 396–410. doi:10.1016/j.msec.2019.03.021
Zhao, L., Xie, Y., Zhang, Z., Wang, X., Qi, Y., Wang, T., et al. (2018). Fabrication and Properties of Biodegradable ZnO Nano-Rods/porous Zn Scaffolds. Mater. Characterization 144, 227–238. doi:10.1016/j.matchar.2018.07.023
Zhao, L., Zhang, Z., Song, Y., Liu, S., Qi, Y., Wang, X., et al. (2016). Mechanical Properties and In Vitro Biodegradation of Newly Developed Porous Zn Scaffolds for Biomedical Applications. Mater. Des. 108, 136–144. doi:10.1016/j.matdes.2016.06.080
Zhao, X., Wan, P., Wang, H., Zhang, S., Liu, J., Chang, C., et al. (2020). An Antibacterial Strategy of Mg-Cu Bone Grafting in Infection-Mediated Periodontics. Biomed. Res. Int. 2020, 7289208. doi:10.1155/2020/7289208
Zheng, Y. F., Gu, X. N., and Witte, F. (2014). Biodegradable Metals. Mater. Sci. Eng. R: Rep. 77, 1–34. doi:10.1016/j.mser.2014.01.001
Zhu, S., Huang, N., Xu, L., Zhang, Y., Liu, H., Sun, H., et al. (2009). Biocompatibility of Pure Iron: In Vitro Assessment of Degradation Kinetics and Cytotoxicity on Endothelial Cells. Mater. Sci. Eng. C 29 (5), 1589–1592. doi:10.1016/j.msec.2008.12.019
Zimmerli, W. (2014). Clinical Presentation and Treatment of Orthopaedic Implant-Associated Infection. J. Intern. Med. 276 (2), 111–119. doi:10.1111/joim.12233
Keywords: magnesium-based alloys, zinc-based alloys, iron-based alloys, degradable alloys, orthopedic implants, antibacterial
Citation: Wang N, Ma Y, Shi H, Song Y, Guo S and Yang S (2022) Mg-, Zn-, and Fe-Based Alloys With Antibacterial Properties as Orthopedic Implant Materials. Front. Bioeng. Biotechnol. 10:888084. doi: 10.3389/fbioe.2022.888084
Received: 02 March 2022; Accepted: 11 April 2022;
Published: 23 May 2022.
Edited by:
Tairong Kuang, Zhejiang University of Technology, ChinaReviewed by:
Ulrich Klotz, Forschungsinstitut für Edelmetalle und Metallchemie, GermanyShokouh Attarilar, Shanghai Jiao Tong University, China
Copyright © 2022 Wang, Ma, Shi, Song, Guo and Yang. This is an open-access article distributed under the terms of the Creative Commons Attribution License (CC BY). The use, distribution or reproduction in other forums is permitted, provided the original author(s) and the copyright owner(s) are credited and that the original publication in this journal is cited, in accordance with accepted academic practice. No use, distribution or reproduction is permitted which does not comply with these terms.
*Correspondence: Shu Guo, c2d1b0BjbXUuZWR1LmNu; Shude Yang, c2R5YW5nQGNtdS5lZHUuY24=
†These authors have contributed equally to this work