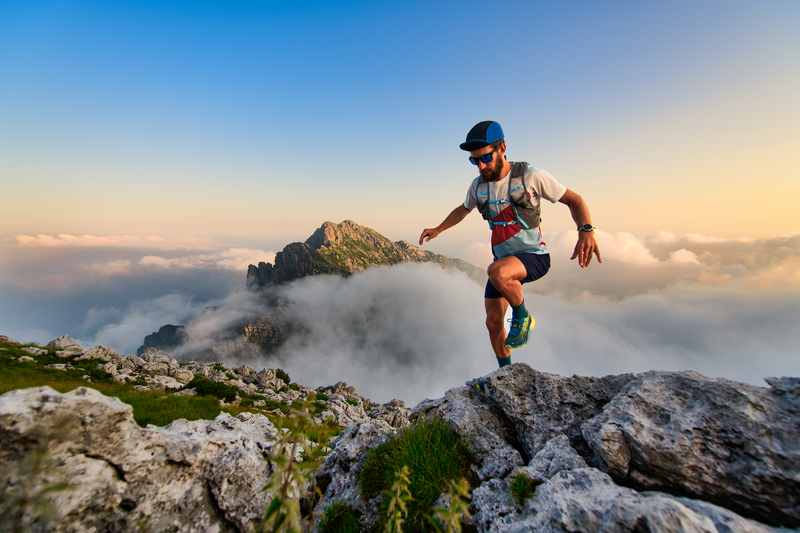
95% of researchers rate our articles as excellent or good
Learn more about the work of our research integrity team to safeguard the quality of each article we publish.
Find out more
REVIEW article
Front. Bioeng. Biotechnol. , 18 May 2022
Sec. Nanobiotechnology
Volume 10 - 2022 | https://doi.org/10.3389/fbioe.2022.882100
This article is part of the Research Topic Quantum Dots for Biological Applications View all 5 articles
The understanding of the genesis of life-threatening cancer and its invasion calls for urgent development of novel technologies for real-time observations, early diagnosis, and treatment. Quantum dots (QDs) grabbed the spotlight in oncology owing to their excellent photostability, bright fluorescence, high biocompatibility, good electrical and chemical stability with minimum invasiveness. Recently, carbon QDs (CQDs) have become popular over toxic inorganic QDs in the area of bioimaging, biosensing, and drug delivery. Further, CQDs derived from natural sources like biomolecules and medicinal plants have drawn attention because of their one-pot, low-cost and ease of synthesis, along with remarkable tunable optical properties and biocompatibility. This review introduces the synthesis and properties of CQDs derived from natural sources, focusing on the applicability of CQD-based technologies as nano-theranostics for the diagnosis and treatment of cancer. Furthermore, the current issues and future directions for the transformation of CQDs-based nanotechnologies to clinical applications are highlighted.
Cancer is one of the most severe diseases in the lives of humankind. As per estimates from the world health organization, it is the first or second leading genesis of demise before the age of 70 years in almost all developed countries (Sung et al., 2021). By 2040, 27.5 million new cancer cases and 16.2 million deaths are projected exclusively due to cancer (Ferlay 2019). Cancer is caused by the uncontrolled growth of cells, ultimately these extra abnormal cells produce an unusual mass of tissues i.e., tumors. Despite the advances in medical science, cancer is still a major challenge to early diagnosis and treatment due to the high mutation rate and metastasis of cancer cells (Sun et al., 2018). Radiotherapy and chemotherapy are the typical ways to fight cancer. Several serious side effects have been observed with these treatments, like the death of normal cells, hair loss, neutropenia, blood clotting, and many more (Pearce et al., 2017) (Meirow and Nugent 2001). Therefore, there is an urgent requirement for a more targeted, and painless strategy to detect as well as cure cancer.
In recent years, nanotechnology has become the most promising interdisciplinary field to solve major challenges in almost all sectors like health, energy, and the environment. Similarly, nanotechnology has played a vital role in the treatment and diagnosis of cancer in the past years. Specifically, over the years carbon quantum dot (CQD)-based strategy provides a wide range of applications such as fluorescence imaging(C. Shi et al., 2019), drug delivery (Su et al., 2020), photoinduced therapy (Zhang et al., 2017), and nanomedicine (Bayda et al., 2021). CQDsare given attention in biomedical applications due to their promising properties such as tunable fluorescence (Wang C. et al., 2020), biocompatibility (Teradal and Jelinek 2017), low toxicity, good electrical and chemical stability (Tian et al., 2021). The clinical applications of CQDs are summarized in Table 1. However, there are certain drawbacks associated with traditional CQDs such as the use of toxic precursors, harsh synthesis methods, high cost, and poor reproducibility. Thus cost-effective, sustainable, and eco-friendly avenue has to be designed. In this context, bioinspired CQDs have attracted great attention in the last few years. Bioinspired, also known as Biodots are CQDs derived from natural sources, like biological materials. These CQDs possess excellent inherent doped structure, tunable size, and promising medicinal properties. Apart from these unique properties, the presence of multifunctional groups and heteroatoms and large abundance offer more capabilities in the biomedical field. Plenty of precursors are available in nature for the synthesis of CQDs, low molecular weight biomolecules, biopolymers, biomass such as plant leaves (Shukla et al., 2020) (Li et al., 2021), fruits (Ramezani, Qorbanpour, and Rahbar 2018) (Tadesse et al., 2020), vegetables (Sachdev and Gopinath 2015), flowers (Wang Y. et al., 2020; Rojas-valencia et al., 2021) and milk (Han et al., 2015; Wang D. et al., 2016). In this review, we discuss recent advances of CQDs derived from biomolecules and medicinal plants for their application in oncology research and treatment, including diagnosis of the tumor, cell imaging, photoinduced therapy and drug delivery (Figure 1). A systematic overview is given on classification, synthesis routes, unique properties, present challenges and future perspectives of natural source-derived CQDs.
FIGURE 1. Schematic representation of applications of CQDs derived from biomolecules and medicinal plants in cancer diagnosis and treatment.
CQDs are defined as a special class of carbon nanomaterial made up of carbon nanoparticles with sizes below 10 nm. CQDs synthesized from naturally occurring material have gained keen interest in the last decades not only due to their biocompatibility and non-toxicity but also due to their presence of heteroatoms, fluorescence, and surface functionalization. As the molecular structures and inherent functionalities of natural precursors can potentially affect the physiochemical properties of CQDs, a closer look at the precursors used in CQDs synthesis is essential for a better understanding of CQD synthesis. As discussed in the previous part, in this review we particularly focus on biomolecules, biopolymers, and medicinal plants as a precursor for the synthesis of CQDs, as schematically shown in Figure 2. Since biomolecules (and biopolymers) are good sources of heteroatoms, CQDs constructed from these precursors are often doped with nitrogen, sulfur, or phosphorus. The doping of nitrogen and sulfur in CQDs results in changes in molecular structures and optical properties (Wang and Hu, 2014). In addition, biomass from plants has gained great attention as a CQD precursor. Many plants have been used to produce CQDs of different sizes and emission wavelengths. In addition, some medicinal plants have anti-cancer therapeutic properties as well. CQDs from different plants exhibit vastly different surface properties and morphology due to their different chemical compositions and concentrations of molecular building blocks.
FIGURE 2. Schematic representation of precursors for the synthesis of CQDs from biomolecules and medicinal plants.
A wide range of biomolecules and biopolymers such as proteins(Lapshina et al., 2019), amino acids (Pei et al., 2015), nucleic acids (T. Song et al., 2015; Wang et al., 2021),carbohydrates (Li Y. et al., 2014; Wang and Wallace 2015; Niino et al., 2016), and vitamins (Carneiro et al., 2019) (Nasrin et al., 2020) has been used for the fabrication of CQDs. The selection of precursor is based on the intended application, because it affects the variation of heteroatoms (doped atom) and functional groups in the CQDs, which influences the optical and electrical properties of CQDs. Therefore, in this section we first discuss various precursor biomolecules that have been used for the synthesis of CQDs and their distinctive properties.
Amino acids are biomolecules containing amine (-NH2) and carboxylic acid (-COOH) functional groups attached to the same Carbon atom. Amino acids are an attractive precursor for the synthesis of CQDs due to their abundance, low price, good solubility and biocompatibility. For example, Pei. et al. constructed CQDs from hydrothermal reactions of amino acids at low temperatures (Pei et al., 2015). Recently, Xu et al. reported a systematic analysis of material design for the synthesis of amino acid-derived CQDs (AA dots) (Xu et al., 2018). It is revealed that various physiochemical properties like optical properties, stability, and morphology of CQDs vary according to the functional group (R-group) attached to the central α-carbon atom. In another work, Kafra et al. applied a similar strategy and synthesized CQDs of high quantum yield from 8 amino acids: arginine, cysteine, glutamic acid, glutamine, aspartic acid, lysine, tyrosine, and methionine. Among all the CQDs, the cysteine-derived CQDs have shown optimistic properties like cytocompatibility, photocatalytic activity with potential applications in sensing, cell imaging, and anti-bacterial activity. Protein is one of the most abundant biomolecules widely regarded for its complex structure and versatile functionalities. Proteins are polymers of amino acids which are organic compounds containing carbon, oxygen, hydrogen, Sulphur, and nitrogen. The presence of the amide group and carboxyl group make them a prominent precursor for the synthesis of CQDs. Proteins can easily unfold under certain reaction and environmental conditions like pH and temperature (Otzen 2002). Albumin is the most promising protein for the synthesis of CQDs due to its excellent biocompatibility, biodegradability, and water solubility. bovine serum albumin (BSA), human serum albumin (HSA), hemoglobin and gelatin have been widely used to synthesize highly fluorescent CQDs. Liang et al. reported blue fluorescent CQDs made from gelatin (Liang et al., 2013). These CQDs have shown excitation-dependent, pH-sensitive, and up-converted PL properties. Surface passivation has numerous pitfalls such as toxicity, the requirement of further purification, and complex synthesis techniques. Therefore, Tan et al. synthesized Nitrogen-doped CQDs (N-doped C-dots) from BSA and formic acid without any surface passivation. The product exhibited good photostability and biocompatibility (Tan et al., 2015). β-Lactoglobulin is a major whey protein that is smaller, less hydrophobic, and more tolerant against degradation than BSA. Sai et al. reported CQDs synthesized from β-lactoglobulin with excitation independent emission and high quantum yield (QY) for sensing purposes. (Sai et al., 2017). Also, Huang’s group synthesized CQDs from histidine with strong blue fluorescence. The CQDs can be used as an imaging probe (Huang et al., 2014). Moreover, Chakraborty et al. synthesized Fe-doped CQDs (Blood dots) with a size of 4 nm from hemoglobin (Chakraborty, Sarkar, and Das 2018). In another work, Yadav et al. utilized lysozyme as the carbon source to construct pH-sensitive protein nanodots (PND) with excellent biocompatibility at pH 7, as shown in Figure 3A (Yadav et al., 2021). PNDs have also been synthesized by the combination of amino acids with other molecules. For example, Nawaz et al. synthesized blue emissive CQDs from l-lysine and ascorbic acid as the precursor. Strong blue fluorescence is arrived due to the presence of doped nitrogen. When lysine is replaced by phenol, this blue color is significantly affected(Faisal et al., 2013). In short, Proteins and amino acids are excellent sources to produce fluorescent and biocompatible CQDs that can be used for sensing and bioimaging applications.
FIGURE 3. Schematic of Synthesis of CQDs from (A) lysozyme via hydrothermal method(Yadav et al., 2021), (B) DNA via sonication and hydrothermal method (Ding et al., 2015), (C) Ocimumtenuiflorum via hydrothermal method(Shukla et al., 2020), (D) Gynostemma via Calcination (Wei et al., 2019), (E) nucleotides via hydrothermal method (Zheng et al., 2019)and (F) hemoglobin via simple heating (Chakraborty et al., 2018).
Carbohydrate is a hydrophilic biomolecule also known as saccharide consisting of carbon, hydrogen, and an oxygen atom. Sugar, starch, and cellulose are also included in this category. Sugars are the smallest and low molecular weight carbohydrate also called monosaccharides while oligosaccharides and polysaccharides are other classes of these molecules. Carbohydrates play a vital role in the functioning and regulating various processes in living organisms like energy storage, preventing blood coagulation, immune system, and fertilization. Thus, Carbohydrates have been extensively used for the synthesis of carbon-based nanomaterial due to their sustainability (Demir-Cakan et al., 2009) and strong multicolor fluorescence (Peng and Travas-Sejdic, 2009; Zhu et al., 2009). For example, Wang et Al. reported the time-saving synthesis of multicolor photoluminescent (Carbon dots) from carbohydrates such as glycerol, glucose, glycol and sucrose without the addition of surface passivation reagent (Wang et al., 2011). Biomass such as obtained from algae is a rich source of lipids as well as carbohydrates. Thus, it would be a great potential source for the cost-effective construction of CQDs. For example, Usain et al. constructed CQDs (Carbon dots) from lipids and carbohydrates extracted from microbial biomass (microalga Acutodesmus obliquus) (Gusain et al., 2021). Interestingly addition of acetone results in a redshift in the absorption spectra which explores potential biomedical applications such as cell-imaging, fluorescent ink, and drug delivery.
Glucose is widely used as a precursor to synthesize CQDs due to its high-water solubility, low cost, and non-toxicity. Ma et al. synthesized nitrogen-doped CQDs (NCDs) via ultrasonication treatment by reaction between glucose and ammonium hydroxide (Ma et al., 2012). Since glucose can cross blood-brain barriers, researchers have started to construct glucose-based CQDs to penetrate the blood-brain barrier for various applications related to brain diseases. For example, Zheng et al. derived CQDs from D-Glucose for imaging cancerous Glioma cells (Qiao et al., 2018). Apart from that, fructose, maltose, sucrose lactose, and their derivatives have also been used as carbon sources. Furthermore, polysaccharides such as chitin, chitosan (Liu et al., 2016a) and cellulose are the main precursors reported to derive CQDs.
Nucleic acids are an important class of biomolecules that refers to DNA and RNA. The usual functions of them are to store and encode information that comes in different molecular forms to synthesize protein. Nucleic acids consist of nitrogenous nucleobases, a phosphate group, and pentose sugar. These molecules of different nucleotide sequences have been reported as the carbon source for the synthesis of CQDs. For example, Guo et al. reported blue fluorescent CQDs from DNA of different lengths via self-assembly at low temperatures (80°) (Guo et al., 2013). Ding et al. constructed CQDs from DNA via sonication and hydrothermal treatment at 180°C for 12 h(Ding et al., 2015). It is also reported that most of the covalent bonds in DNA molecules remain as it is in CQDs. Also, DNA CQDs are formed by crosslinking DNA strands due to interaction between amino groups of the bases and with the phosphate group of other DNA strands which causes dehydration, polymerization, carbonization, and condensation. Also, Zheng et al. constructed Single oxygen generating CQDs from four basic nucleotides of DNA via the hydrothermal method as schematically shown in Figure 3E (Zheng et al., 2019). Furthermore, Luo et al. reported CQDs synthesized from cytosine, a DNA base by heating at 160°C (Luo et al., 2018). It is shown that these CQDs have excellent photostability and are stable in a wide range of pH, offering potential application in cell imaging and sensing.
Other biomolecules such as folic acid, vitamins, and glutathione are also used to synthesize CQDs. For example, Zeng and co-workers reported highly water-soluble and bright blue fluorescent CQDs from L-Glutathione with a quantum yield of 40% (Zeng, Zhang, and Yang 2017). Also, Gong and co-workers synthesized highly luminescent CQDs from ascorbic acid as the precursor (Gong et al., 2014). These highly biocompatible CQDs have shown excellent results for imaging human breast cancer cell Bcap 37.
From ancient times, humankind use various plants as medicine due to captivating properties such as self-healing (Chah et al., 2006), anti-inflammatory (Ravipati et al., 2012), anti-bacterial (Lekhak and Sharma 2009), anti-venom (Kumarapppan, Jaswanth, and Kumarasunderi 2011), anti-aging (Ndlovu et al., 2013), and many more (Jamshidi-kia, Lorigooini, and Amini-khoei 2018). Due to such inherent medicinal properties, medicinal plants have huge potential for diagnosis and treatment of cancer. It is proven that certain plants have excellent anti-cancer properties like tumor inhabitation (H. Park et al., 2017), induce apoptosis (Kumar et al., 2017b), and induced cell cytotoxicity (Itharat et al., 2004). Thus, medicinal plant-based CQDs are developing a new fascinating and sustainable approach in the biomedical sector. Additionally, medicinal plants based CQDs have simple synthesis routes and easy availability of plants. Therefore, CQDs obtained from medicinal plants have drawn attention in the last decade. Medicinal plant-based carbon quantum dots have shown excellent photostability, nontoxicity, high PL, and water solubility(Naik et al., 2021). However, no systematic study has so far been reported on the synthesis of medicinal plants-based CQDs. In this review, we discuss the importance and unique properties of medicinal plants-based CQDs.
Various parts of plants such as leaves, stems, roots, fruits, flowers, seeds and many have been used as medicine for a long time. These parts are rich in biomolecules, metals, nonmetals, and complex functional groups. Thus, CQDs derived from certain plants show interesting theranostic properties. For example, Li et al. synthesized CQDs from Ginger juice and used them as it is without the addition of any drug (Li C.-L. et al., 2014). Similarly, Hsu et al. constructed biocompatible and highly photoluminescent CQDs from Green Tea (He et al., 2021). These carbon dots show excellent efficiency for inhabitation of breast cancer. In addition, Arkan et al. synthesized CQDs from walnut oil which showed apoptotic activity towards breast cancer cells (Arkan et al., 2018). Furthermore, Meena et al. reported CQDs synthesized from plants Viz. (Azadirachta Indica), (Ocimum Tenuiflorum) and (Tridax Procumbens) which have widely been used in Ayurveda for medicinal purposes. Ginseng is the root of the plant’s genus Panax and is widely used as medicine and functional food. It has extremely beneficial properties like anti-oxidant and anti-inflammatory and is widely used as medicine in neurodegenerative disease and anti-cancer activities (Radad et al., 2006). Yao et al. constructed CQDs from Ginsenoside Re, an active compound of Ginseng via one-pot hydrothermal treatment (Yao et al., 2018). Ocimum sanctumis also known as Holy Basil or Tulasi which is widely popular for its medicinal and spiritual properties in Asian tropics. As is greatly used as medicine for cough, asthma, diarrhea, fever, dysentery, arthritis, eye diseases, indigestion (Srinivas Naik et al., 2015), etc., Shukla et al. constructed CQDs from Ocimumtenui florum via one-step hydrothermal method at reaction temperature of 200°C as shown in Figure 3C. Thus, medicinal plants as a precursor are the most cost-effective, environment-friendly, and efficient strategy for the synthesis of non-toxic, biocompatible, and highly luminescent CQDs.
Researchers have explored a variety of techniques to synthesize nanomaterials with desired size, shape, and orientation. These technical approaches may be grouped as top-down (Limosani et al., 2021) or bottom-up (Guo et al., 2018). In a top-down process, CQDs are typically manufactured from bulk materials like carbon powder. This approach is cost-effective and does not require a lot of experimental conditions or harsh chemicals. It, however, produces a substantial amount of waste material, and it is also difficult to control the size and morphology of CQDs and hence their physiochemical properties. An alternative is to use a bottom-up approach, which could result in less waste and therefore be more economically feasible. The bottom-up approach refers to building up material from the bottom-up, atom-by-atom, molecule-by-molecule, or cluster-by-cluster. Some of these techniques are still under development or have just begun to be used for commercial production. Among the top-down and bottom-up approaches, the bottom-up approach is widely accepted in the synthesis of CQDs due to many merits including fewer defects, more homogenous chemical composition, and more ordered structure. It is also reported that the Gibbs free energy, thermodynamic equilibrium, and kinetics are the main factors to consider for CQD synthesis in the bottom-up approach (Mahmoud et al., 2020). Here we discuss some widely used techniques for the synthesis of CQDs.
Hydrothermal synthesis is one of the most widely used methods due to its low cost and easy operation. This method covers all four critical processes involved in the synthesis of CQDs: carbonization, dehydration, passivation, and polymerization. In this method, the precursor is mixed with suitable solvent in a sealed autoclave at an elevated pressure, and heated in an oven to a specific temperature. In this method, particle morphology, surface chemistry, and crystalline phase can be tuned by controlling temperature, pressure, solvent, and reaction time. For example, Yoshinaga et al. reported CQDs from D-Glucose at 200°C. It is also reported that the size of the CQDs increases with an increase in hydrothermal reaction time from 5 to 60 min(Yoshinaga et al., 2018). Song et al. synthesized fluorescent CQDs from DNA in an aqueous medium by heating at 160°C for 4 h (T. Song et al., 2015). Similarly, a wide range of biomolecules and biomass like plant leaves (Alam et al., 2015; Li et al., 2022), fruit juice (Sahu et al., 2012), proteins (Tan et al., 2015; Kumar et al., 2016) and amino acids (Huang et al., 2014, Y.; Jiang et al., 2015) have been used to synthesize CQDs.
Microwave heating is a simple and efficient technique for rapid and efficient synthesis of material with higher reproducibility. In this method, there is a transfer of electromagnetic energy to thermal energy when the solution is placed in a microwave chamber. The microwave effect arises due to material-wave interaction and due to the dipolar polarization of molecules in solution. After irradiation, a large number of molecules move to reorient themselves leading to collision and rise in temperature of the solution. The effect of this technique is greatly affected by the polarity of the reacting molecules. Precursors such as sugars, amino acids (J. Jiang et al., 2012), proteins (Liu et al., 2016b) and other green precursors (C. Liu et al., 2011) are widely used to synthesize CQDs by this technique.
The pyrolysis technique is simple and economical for the bulk production of carbon dots. In this technique, the materials are subjected to heat in the presence of controlled pressure above the melting point in the absence of oxygen. Under this atmosphere, physical and chemical changes occur in organic precursor substances which results in carbon-containing solid residues. CQDs are in general obtained after further condensation and nucleation. This technique is widely used to synthesis specifically from hydrocarbon-rich organic sources such as glycerol, citric acid, plant leaves (Zhu L. et al., 2013) and organic waste (Zhou et al., 2012; Xue et al., 2015). For example, Zheng et al. reported CQDs from L-aspartic acid and D-glucose via pyrolysis method at 200°C. The obtained CQDs have an average size of 2.28 nm (Zheng et al., 2015).
The use of ultrasonication energy to synthesis nanomaterial has become quite interesting and growing due to the low temperature and environment-friendly approach. Acoustic waves induced by ultrasonic radiation produce bubbles (cavity) in the solution. These bubbles restore energy during this process and collapse after overgrowing and releasing energy and temperature to the solution, leading to the breaking of chemical bonds to produce CQDs. This method is used for a wide range of precursors such as biomolecules (Li et al., 2010), biowastes (Park et al., 2014), microorganisms (Lee et al., 2014) and fruit juices (Balcı et al., 2015).
This method is comparatively efficient for bulk production due to its simplicity and low cost. In this method, precursors are mixed at room temperature and then heated up to a particularly high temperature to form CQDs by crystallization reaction. Many biomolecules such as sugars (Gong et al., 2016), amino acids (Wu et al., 2013), and other biological materials such as plant leaves (Wang et al., 2011) and fruit juices (Hsu et al., 2012) have been reported to synthesize CQDs via simple heating.
In this process, various chemical reagents such as acids and bases are used as reducing agents for the synthesis of CQDs. Glucose (Peng and Travas-Sejdic 2009), fructose, and maltose (Li Y. et al., 2014) have been used with various chemicals in the synthesis of CQDs.
In this technique, high intensity laser beam incident on precursor, usually prefabricated nanomaterial to break into small parts and remove certain portion (ablation) to fabricate CQDs. An intense and energetic pulsed laser, femtosecond laser is widely used for this strategy due to high precision and minimum damage of surrounding. This technique is eco-friendly and efficient due to no chemicals required and contactless preparation (Singh et al., 2021). For example, Ren et al. fabricated CQDs from biowaste Platanus with average size of 1.26 nm using pulsed laser ablation (Ren et al., 2019).
Characterization of synthesized CQDs is a crucial factor to design CQDs of pertinent properties according to required applications. Various parameters or properties of CQDs such as size, bonding, structure, elemental composition, fluorescence, and many other physical and chemical properties have been tuned by using fine characterization techniques. Various characterization techniques such as ultraviolet-visible (UV-Vis) spectroscopy, Fourier transform infrared radiation (FTIR) spectroscopy, X-ray photon spectroscopy (XPS), Transmission Electron Microscopy (TEM), and photoluminescence (PL) spectroscopy is widely used. In this section, we discussed these major characterization techniques in brief.
As discussed earlier, CQDs contain amorphous carbon structures along with functional groups formed by carbonization and polymerization of organic molecules. CQDs display excellent and unique absorption properties due to sp2 carbon core and surface states. UV-Vis spectroscopy is a technique that is based on the measurement of absorption of electromagnetic radiation in the region of UV-Vis (800–200 nm). Usually, CQDs show excellent absorption in the range of 280–380 nm. When CQDs absorb waves in the UV or visible region, they shine with a very small stoke shift (a few nanometers). In general, CQDs have absorption peaks due to π-π* transition of aromatic sp2 domain and n-π* transition of various functional groups such as carboxyl, hydroxyl, amine, and ester. For example, Zang et al. reported nitrogen-doped CQDs (N-CQDs) with three absorbance bands at around 235, 285, and 380 nm (Zhang and Chen, 2013). The peak at 235 nm is responsible for π-π* transition while the other two are due to the excited state energy of the surface which is responsible for high fluorescence intensity. In addition, Chakraborty et al. have shown that oxidative damage of DNA in photoinduced therapy can investigate using UV-Vis spectroscopy, in which absorption maxima of DNA gradually decreased with the addition of H2O2 and hemoglobin derived blood dots (BDs) (Chakraborty, Sarkar and Das, 2018). In the recent past, broad absorption spectra, absorbance in the visible range (450 nm) is also reported which occurs due to various surface states corresponding to a small bandgap within the n-π* band gap(Schneider et al., 2022).
The infrared spectrum represents a fingerprint of a sample whose absorption peaks correspond to frequencies of oscillation between the bonds of the atoms that make up the material. Because each different material is a unique combination of atoms, no two compounds produce the same infrared spectrum. Therefore, FTIR spectroscopy can give a positive determination (qualitative analysis) of different materials. In addition, the size of the peaks in the spectrum is a direct indicator of the amount of matter present. With modern software algorithms, the FTIR is an excellent tool for the quantitative analysis of CQDs. For example, Yadav et al. constructed protein nanodots (PND) from Lysozyme protein (Yadav et al., 2021). The characteristics of amide bands of precursor protein are not observed in the spectrum as shown in Figure 4A. which shows that the globular structure of protein gets converted into PNDs. While in another work Atchudan et al. reported a band of nitrogen-doped CQDs (N-CDs) at 1,680, 1,590, 1,400, 1,290, and 1,072 cm-1 which corresponds to C=O, C=C, C=N, C-OH, C-O-C/C-N stretching vibrations respectively (Atchudan et al., 2018). This result is supported by excellent solubility N-CDs in an aqueous medium due presence of a polar functional group.
FIGURE 4. Depiction for characterization of PNDs: (A) FTIR spectra (B) XPS spectrum showing peaks for oxygen, nitrogen, and carbon and (C) Deconvoluted high-resolution spectra for C1s, N1s, and O1s (Yadav et al., 2021).
X-ray Photoelectron Spectroscopy (XPS), also known as Electron Spectroscopy for Chemical Analysis (ESCA), is a technique based on photoelectric effect for quantitative analysis of surface composition. XPS can give solid knowledge about surface functionalization, electronic structure, and elemental composition of CQDs. The XPS scan as shown in fig of PNDs concludes that PNDs contain carbon, oxygen, and nitrogen (Yadav et al., 2021). This result also supported that high fluorescence intensity is due to high nitrogen content compared to oxygen as shown in high-resolution spectra of C1s, N1s, and O1s as depicted in Figure 4C–E. In another work of Liang et al., XPS was studied in detail for Gelatin-derived CQDs (Liang et al., 2013). Four peaks observed at 168.2, 284.8, 399.1, and 531.5 eV corresponds to S2p, C1s, N1s, and O1s which indicate the presence of sulfur, carbon, nitrogen, and oxygen.
TEM is a microscopy technique in which a beam of electrons is passed through an ultra-thin material and interacts with the sample as it passes.
An image is formed from the interaction of electrons transmitted through the sample; the image is enlarged and focused on an imaging device such as a fluorescent screen on a layer of photographic film or to be captured by a sensor. It has a wide demand in nanotechnology to explore morphology and size distribution due to its high resolution up to 1 nm. For example, Mohajeri et al. constructed DNA CQDs (DNA dots) with sizes 4.5–5 nm(Mohajeri et al., 2020). From TEM images show that DNA dots are spherical in shape and well separated from each other i.e., no aggregation is formed. In another work Simsek et al. constructed CQDs from Nerium Oleander with an average size of 2.05 nm measured by TEM (Şimşek et al., 2020). It is also revealed that CQDs possess a high crystalline structure with 0.21 nm spacing which corresponds to sp2 carbon.
Photoluminescence or specifically saying fluorescence is the most fascinating property of CQDs which has a wide range of applications. CQDs possess excitation wavelength-dependent emission spectra ranging from visible to near IR. Generally, CQDs show an obvious red shift in emission on increasing excitation wavelength. Also, emission spectra depend on size, functional groups, and presence of Heteroatoms. Several studies have reported different approaches to the fluorescence mechanism of CQD, but the origin of fluorescence is still not completely understood. Many reasons are related to the origin of the fluorescence behavior of CQD, such as the quantum confinement effect due to the defects and different functional groups, surface passivation, zig-zag edges, degree of conjugation, and recombination of electron-hole pairs localized within sp2 clusters of carbon aromatic moieties (Zu et al., 2017). At lower temperatures, the luminescence effect is due to molecular fluorophores, while at higher temperatures the effect is due to the predominant carbon nucleus (Ehtesabi et al., 2020). Often multiple levels of fluorescence intensity are observed with a single CQD indicating the presence of multiple chromogenic units in the core and surface emission states. Reduced CQD appears to have multiple levels compared with oxidized CQD exhibiting a single emission level. Therefore, surface chemistry is largely involved in these processes with size effect playing some role and it has been hypothesized to reduce the gap between Highest occupied molecular orbit (HOMO) and Lowest unoccupied molecular orbit (LUMO) with the size reduction of the CQD (Zhu B. et al., 2013). These CQDs often exhibit excitation-dependent fluorescence. CQD also shows a broad emission peak with a large stokes shift relative to organic dyes, which may be due to multiple narrow peaks caused by a heterogeneously prepared solution containing CQD of different sizes, different PL, and surface chemistry (Wang et al., 2013). Surface and functional group oxidation largely determine the emission peak of CQD due to the presence of defects that cause redshift and affect the frequency band in addition to quantum confinement. Multiple synthesis steps and surface modifications have been reported to help achieve higher QY. Most CQDs emit blue or green fluorescence. Blue fluorescence can arise from the n-π* transition of the carbon-oxygen groups or the π-π* transition of the central carbon states. While green fluorescence can arise from n-π* transitions of edge states. Fluorescence of CQDs in the Near-Infra-red region has been used for in vivo imaging. The multiphoton fluorescence of CQDs in the visible and NIR range surpasses the usable wavelength range offered by organic dyes by many orders of magnitudes. Furthermore, by altering the functional groups on their surface, their composition, and their surroundings, their emission may be controlled across the visible spectral range. The PL QY of CDs varies greatly, with figures as low as 1% and as high as 94.5% percent observed (Hu et al., 2015). Kim et al. reported CQDs (S-CDs) from Camellia japonica with NIR absorbance (Kim et al., 2021). It is also, claimed that high green/blue fluorescence at 440 nm is excitation wavelength-independent due to various surface states. Dehghani et al. reported collagen-derived CQDs with excitation wavelength and pH-dependent emission spectra (Dehghani et al., 2018). It is shown that the maximum intensity of emission spectra decreases under high acidic or basic conditions due to induced changes in the functional group and electronic transition in surface defects by H+ or OH−. Guo et al. constructed DNA CQDs (Biodots) with pH and excitation wavelength-independent high fluorescence as shown in Figure 5A (Guo et al., 2013). It is reported that the Cytosine base is responsible for PL properties due to the pyrimidine structure. By controlling the synthesizing conditions, different PL peaks and intensities are observed as shown in Figure 5B,C.
FIGURE 5. Schematic for optical characterization of DNA biodots: (A) UV-Vis absorption spectra for precursor DNA solution and biodots and excitation independent PL spectra for biodots. (B) PL spectra for biodots derived from different precursors and (C) Biodots with different emission spectra (Guo et al., 2013).
Diagnosis of cancer at an early stage is the crucial factor for the cure. Biopsy, computerized tomography (CT) scan, magnetic resonance image (MRI), endoscopy, and X-rays are widely used for the detection of malignant tumors for several decades. However, there are certain limitations with these traditional diagnosis tools such as high cost, imprecision (false negative or positive), lack of awareness, unnecessary treatments, and misuse of medical resources. The result of diagnosis is also subjected to the size of the tumor leading to an increase in the death rate. In addition, there are very few diagnostic tests available for a particularly rare type of cancer. Thus, there is an urgent need for an advanced, cost-effective, sensitive, and biocompatible approach to the early detection of tumor cells. Moreover, conventional treatments like surgery, chemotherapy and radiotherapy have many drawbacks such as harmful side effects including infertility, menopause, emotional difficulties, and shortened life span. Therefore, non-invasive and reliable treatment is highly demanded. Nanotechnology has played a significant role in cancer theranostics. Particularly, CQDs exhibit specific advantages in cancer imaging and therapy due to their large surface area to volume ratio, photoluminescence (PL), water solubility, and biocompatibility. Herein we discuss the CQD-based strategy for anticancer activities in recent years. Table 2 summarizes the precursors, properties, and applications of CQDs derived from biomolecules and medicinal plants.
Fluorescence cell imaging is a widely accepted and most powerful tool in biomedical applications. CQDs exhibit bright, tunable and longtime fluorescence which makes them a suitable candidate for bioimaging. In this part, we mainly discuss the recent progress of CQDs in cancerous cell imaging. It is found that the properties of CQDs, particularly their fluorescence and Quantum yield depend on the physical properties of the precursors like the polarity, the functional groups, and the chemical bonding (L. Shi et al., 2016). CQDs have performed very well for in vitro as well as in vivo imaging with very low toxicity, good biocompatibility with better penetration, and bright fluorescence. Cytotoxicity is one of the most important parameters to consider when selecting a CQD for use as a fluorescent imaging agent. Several studies show that CQDs do not show any cytotoxicity up to a concentration of 0.4 mg/ml (Zhao et al., 2008). They are non-toxic to cells at a concentration necessary for fluorescence imaging. The primary detection mechanism is based on an interaction between CQDs and surface groups of cancer cells. The CQDs internalize into the cells by endocytosis. Upon exposure to CQDs, cancer cells tend to accumulate within the nuclei or the cytoplasm. Targeted staining using CQDs for specific cell imaging has been utilized by attaching special ligands to them such as Transferrin, folic acid, and hyaluronic acid (Peng et al., 2017). For in vitro studies, different types of cancerous cells such as MCF-7, HeLa, c6 Glioma have been successfully imaged with CQDs. Guo et al. developed CQDs from DNA molecules using the self-assembly principle. These CQDs have shown bright fluorescence with a long lifetime (Guo et al., 2013). The fascinating photoluminescence (PL) properties are very useful in bioimaging applications. It is reported that the presence of cytosine base pair is the key factor for excellent PL properties. Hence these CQDs have shown very good fluorescent signals with MCF-7 cancer cells. Bhunia et al. derived blue and green fluorescent CQDs (carbon nanoparticles) from vitamin B1 (thiamine hydrochloride) with an average size of less than 10 nm (Bhunia, Pradhan, and Jana 2014). The presence of Sulphur, nitrogen, and phosphorus created significant bright fluorescence which makes them an efficient imaging probe. Interestingly these CQDs change their fluorescence color with the change in reaction temperature and solvent as shown in Figure 6.
FIGURE 6. Synthesis of tunable fluorescent carbon nanoparticles from vitamin B1. Fluorescent properties changed with reaction temperature and solvent (Bhunia, Pradhan, and Jana 2014).
Folate receptor is overexpressed in cancer cells and tumors such as breast, brain, cervical, and ovarian cancer. Folic acid is an ideal ligand for targeting and imaging cancer cells. Liu and coworkers synthesized luminescent CQDs with a high quantum yield (95%) using folic acid (vitamin B9) as a precursor (H. Liu et al., 2018). These CQD exhibit outstanding properties such as high photostability, photoluminescent activity, and good biocompatibility due to that they can easily penetrate cancer cells.
Choi et al. synthesized fluorescent Folic acid-functionalized CQDs (CDs) from thermal decomposition of α-cyclodextrin and loaded with photosensitizer (PS)zinc phthalocyanine (Znpc) via π-πstacking interaction for imaging as well as PDT. (Choi et al., 2014). Fluorescent CQDs were synthesized from nucleotides by the hydrothermal method (Zheng et al., 2019). Among four types of CQDs (dAMP, dGMP, dCMP, dTMP), dAMP (2′-deoxyadenosine 5′-monophosphate) derived CQDs exhibit excellent properties like high PL intensity, generation of singlet oxygen due to the presence of adenine base. Also, dAMP dots reveal bright fluorescence and high quantum yield due to the existence of an extra phosphate group. In-vitro study was performed by applying dAMP CQDs to HeLa cancer cells. It is reported that the size of the cell decreased compared to non-treated HeLa cells with 95% cell viability at high concentration (Figures 7A,B). Also, under different excitations, dAMP CQDs show green, red and blue fluorescence (Figure 7C), which makes them a promising agent to penetrate the cell membrane and enter the cell for excellent fluorescence labeling.
FIGURE 7. (A) MTT cell proliferation assay along with A-dots,(B) Cellular toxicity of dAMP-CQDs when white light is irradiated at the different concentrations for 10 min, and (C) Confocal microscope images of Hela cells with dAMP CQDs with excitation at 405, 488 and 564 nm (from left to right) resulting in different emission colors blue, red and green, respectively(Zheng et al., 2019).
Though one-pot hydrothermal synthesis is the most popular method to synthesize carbon dots, it takes a high temperature for a very long time. Wang et al. reported that the synthesis of acid catalyzes CQDs from tryptophane and phenylalanine using hydrochloric acid as a catalyst (Wang D. et al., 2016). Due to the presence of hydrochloric acid, the reaction time was reduced significantly by 80%. For specificity, these carbon dots were functionalized with MUC-1 aptamer. These functionalized carbon dots efficiently targeted MCF-7 human breast cancer cells due to the binding between the DNA aptamer and the MUC-1 cancer protein. A comparative study was reported by Xu et al. to reveal various properties of CQDs by synthesizing from 20 natural amino acids separately via a hydrothermal process (Xu et al., 2018). It is reported that the PL properties of CQDs depend on the R group of the precursor molecules. It is also claimed that the presence of hydroxyl group in serine and threonine-based CQDs exhibit excellent photostability, high QY, cellular uptake, and biocompatibility compared to other CQDs, which makes them a good candidate for cell imaging. While in another study, Kafra et al. synthesized a series of CQDs from eight amino acids (Karfa et al., 2015) via the hydrothermal method with the addition of extra sulfuric acid. Among all the CQDs, cysteine (Cys) CQDs were reported with exceptional high QY and PL intensity. This result is in contradiction to the study by Xu et al. The CQDs made by Karfa et al. have shown excellent stability and good fluorescence during cell imaging with MCF7 cells.
Brain cancer is the most dangerous and challenging among all types of cancer. Detection and treatment of brain cancer are greatly affected by the blood-brain barrier. The blood-brain barrier is a physiological barrier that protects brain and brain tissues from foreign substances and allows them to pass specific molecules. Zheng et al. reported CQDs (CD-Asp) as smart nanomedicine synthesized from D-glucose and L-aspartic acid (Zheng et al., 2015). These CQDs can cross the blood-brain barrier and act as a fluorescent imaging probe for c6 glioma cells. Furthermore, Qiao et al. have shown that when D-glucose and L-aspartic acid has been taken in a molar ratio of 7:3, the synthesized CQDs have the highest selectivity toward glioma cells (Qiao et al., 2018).
The targeting cell nucleus is gaining more attention in cancer treatment. Some CQDs have shown potential for imaging cell nuclei. Jung and coworkers reported zwitterionic CQDs synthesized from citric acid and β-analine with multicolor fluorescence (Jung, Shin, and Kim 2015). These CQDs can penetrate the cytoplasm of Hela cells by endocytosis due to their positively charged moieties. Song et al. prepared transactivator of transcription (TAT) peptides conjugated CQDs from tryptophan (Y. Song et al., 2019). They performed one and two-photon fluorescence nucleus imaging with mouse melanoma B16-F10 cells.
Betel leaves have been used as a mouth freshener fora very long time as it is loaded with antioxidants, vitamins, and minerals (Guha and Guha 2017). Atchudan et al. derived nitrogen-doped CQDs (B-NCD) from betel leaves. The CQDs showed multicolor fluorescence (Atchudan et al., 2018). The authors also reported multicolor imaging on HCT 116 colon cancer cells. Aloe Vera is a medicinal plant that has inherent anti-cancer properties along with self-healing, anti-aging properties. It has been widely used in various cosmetics, medicines, and as a preservative (Raksha 2014). Prasad et al. constructed amorphous CQDs using Aloe vera extract as a precursor (Prasad et al., 2021). Interestingly these CQDs have shown apoptosis effects on MCF-7 cancer cells, and are useful as a fluorescent probe for live imaging.
The development of nano-drug carriers for anti-cancer treatment has attracted great interest in recent years. Most of the current anti-cancer drugs are non-precise, poorly soluble, and have many side effects. CQDs can efficiently be used in drug delivery systems by incorporating them into as anti-cancer nano-drug carriers due to excellent water solubility and biodegradation. They can lend their excellent fluorescence imaging capability for tracking drug response, delivery, and activity by combining ‘therapy’ as well as diagnostics known as theranostic approaches. The release of attached drug moiety leads to fluorescence recovery of the CQDs which can be monitored over time. These molecules are attached via covalent or non-covalent bonds due to the presence of various functional groups such as amine, carboxyl, and hydroxyl as depicted in fig. The former provides more control for targeted release through pH changes or light irradiation. For example, Zeng et al. constructed Carbon dots (CD) by microwave synthesis of citric acid and urea providing excellent surface chemistry to form CD- Doxorubicin (DOX) drug conjugate (Zeng et al., 2016). Carboxyl group of CD formed hydrogen bonding with the amine group of DOX, an anti-cancer drug. This non-covalent interaction utilizes the difference in pH of cancerous and normal cells to kill cancer cells effectively as depicted in Figure 8. Another work by Gong et al. reported phosphorous and nitrogen-doped carbon dots (PNHCD) with enhanced drug loading capacity due to the presence of a central hollow cavity and multiple functional groups on a surface such as phosphoric, pyridinic, pyrrolic, and hydroxyl(Gong et al., 2016). CQDs enhance the intracellular concentration of drugs in cancer cells and keep away normal cells from toxicity. For example, Simsek et al. synthesized carbon dots (CD) of size 2.05 nm from Nerium Oleander (Şimşek et al., 2020). CQDs can penetrate cell nuclei to react with genes resulting in DNA damage, as explained in Figure 9. Ding et al. developed CQDs from DNA isolated from Escherichia coli. These Carbon dots are successfully loaded with Rh 6G and DOX within prokaryotic and eukaryotic cells (Figure 10) (Ding et al., 2015). It was seen that from Figure 10A–C, CQDs evenly transported Rh 6G into cells while Figure 10D–F showed Dox was distributed in eukaryotic cells seen as a dotted pattern.
FIGURE 8. Overall presentation of synthesis and application of multifunctional CQDs (nanodots) (BCCGH) from BSA, carbon dots, and metal ions
FIGURE 9. Depiction of CD-DOX conjugate: Amine group of DOX binds with carboxylic group of CD, CD–DOX conjugates deliver to HepG2 cancer cells and HL-7702 normal cells. The CD–DOX conjugates will release DOX in HepG2 cancer cells, but not in HL-7702 normal liver cells, due to a difference in pH i.e., a cancer cell has low pH(Zeng et al., 2016).
FIGURE 10. Schematic of CQDs impact on human breast cancer (MCF-7) cell line (Şimşek et al., 2020).
Jha et al. synthesized CDs (Biodots) from DNA for the treatment of non-small lung cancer (Jha et al., 2020). They developed DNA CDs and ETP loaded and cetuximab conjugated liposomes for effective targeting of tumor cells. Yadav et al. reported the synthesis of protein CDs (PND) from lysozyme (Yadav et al., 2021). These protein CQDs are loaded with melatonin, a potent antioxidant with anti-tumor properties. Melatonin-loaded PNDs (MPND) were treated with breast cancer cells and they showed good cellular uptake. Ma and co-workers developed ternary nanoparticles from peptide dendritic carbon dots. These CQDs are synthesized from glucose (Ma et al., 2018). To increase the efficacy of Gemcitabine (GEM), a chemotherapy drug, Yunus et al. conjugate it with CQDs derived from sucrose via the ultrasonication method (Yunus et al., 2020). In-vitro and in vivo studies have shown that the CDs-GEM conjugate works more selectively towards cancerous cells and penetrates cell membranes efficiently.
Photoinduced therapy has received great interest in recent years due to no invasiveness, high specificity, and minimum damage to normal tissues (Ferroni et al., 2019). Typically, this therapy includes photothermal therapy (PTT) and photodynamic therapy (PDT). In PTT, Near-Infrared light is used to generate heat to kill cancer cells by elevation of tissue temperature as explained in Figure 11. NIR absorbents are used for efficient heat generation. Similarly, the photodynamic strategy for cancer treatment is processed by using the interaction between light and photosensitizing agents. In this therapy, when photosensitizers are exposed to the light of a particular wavelength, they generate reactive oxygen species and kill cancer cells by oxidative DNA damage.
FIGURE 11. DNA–CQDs as a vehicle for the anti-cancer drug. (A,D) Confocal microscopy images for CQD alone, (B,E) Rh 6G or Dox, and (C,F) Overlay in S. cerevisie cells (Ding et al., 2015).
Experimentally it is observed that overexpression of H2O2 is the major concern of cancer cells (Yang et al., 2020). An increase in the cellular level of H2O2in cancer cells when compared to normal cells is due to the presence of a high level of superoxide dismutase produced in mitochondria. This overexpressed H2O2 can act as a pro-drug in the presence of an appropriate activator by producing hydroxyl and superoxide radicals to kill the cancerous cell by oxidative DNA damage. For example, Chakraborty et al. derived Blood dots, Fe2+ containing carbon dots from hemoglobin (Chakraborty, Sarkar, and Das 2018). Blood dots sense H2O2 as well as split into hydroxyl (OH−) and superoxide radicals. These highly reactive radicals can kill the cancerous cell by oxidative DNA damage. However, PTT is still challenging due to poor photothermal conversion of CQDs.
Though the photoinduced strategy has huge potential and is of great interest in cancer treatment, it is often reported that photosensitizers possess issues like limited solubility and poor selectivity. So, a carrier or delivery system must increase its solubility and cellular internalization in body fluid. For example, Choi et al. synthesized folic acid functionalized carbon dots from α-cyclodextrin and loaded them with zinc phthalocyanine (Znpc), a photosensitizer for in vivo and in vitro photodynamic therapy (Choi et al., 2014). Carbon dots-based PD agent enhanced efficacy of photodynamic therapy with relatively small amount of (Znpc). Similarly, Hua et al. fabricated multifunctional nanodots using BSA, carbon dots, and metal ions and conjugated them with 2-(1-hexyloxyethyl)-2-devinyl pyropheophorbide-a (HPPH), a photosensitizer (Hua et al., 2018a). Nanodots have shown excellent photothermal conversion efficiency (68.4%) for both PTT and PDT performance as explained in detail in Figure 12.
FIGURE 12. Green fluorescent carbon quantum dots (CQDs) for cell labeling and Near Infra-Red (NIR) light exposed therapy (Meena et al., 2019).
Camellia Japonica is a nutrients and medicina plant which is rich in various bioactive compounds with numerous benefits (Yoon et al., 2017). Here Kim et al. synthesized sulfur-doped CQD (S-CDs) from Camellia Japonica flowers for efficient photothermal therapy (Kim et al., 2021). These CQD exhibits efficient PTT activity in vivo as well as in vitro, with 55.4% efficiency. In addition, Zhao et al. prepared multifunctional CQD from seaweed plants. They reported PTT activity with a high photothermal conversion efficiency (42.2%).
Ginsenosides, an asteroid-like compound is the main constituent of the Panax ginseng plant. It is a widely used herbal medicine in Asia and western countries. Ginsenoside has unique medicinal and anticancer properties such as anti-tumor, anti-oxidation, and anti-inflammatory effect. Yao et al. developed multifunctional carbon dots from ginsenoside. These CQDs precisely inhibit various cancer cells by increasing Reactive Oxygen Species (ROS) (Yao et al., 2018). The CQDs also trigger apoptosis through a ROS-mediated pathway. In addition, Wei et al. synthesized fluorescent carbon dots (CDs) from the Gynostemma plant by calcination at 400°C as shown in Figure 3D. The plant is rich in various bioactive compounds (Wei et al., 2019). The synthesized CDs also displayed antioxidative stress properties by inducing H2O2 in HeLa cells. The CDs can also elevate mRNA expression to control oxidative damage of normal cells. Meena et al. utilized CQDs from Ayurvedic medicinal Plants, as discussed in the precursor section, for photoinduced therapy by applying the CQDs to kill bacteria. The material showed promising results along with colors-imaging. Thus, these CDs have huge potential in tumor inhibition through oxidative DNA damage without harming healthy cells.
Due to the presence of various multifunctional groups, CQDs are quite suitable for the conjugation of different biomolecules specifically antibodies, genes, and antigens. The immunofluorescent technique has gotten wide attention due to its high sensitivity, specificity, and ultrafast result. In this technique, CQDs can be used to immobilize different molecules resulting in the formation of nanocomposites to detect antigen (tumor marker) responsible for cancer. For example, Alarfaj et al. constructed a nanocomposite of CQDs synthesized from glucose and gold for the detection of CA19-9 pancreatic tumor, a carbohydrate antigen (Alarfaj et al., 2018). In another work, they reported CQDs made from citrus lemon for detection of cytokeratin19 fragment (Cyfra21-1) for early detection of lung cancer (Alarfaj et al., 2020).
The fluorescence of CQDs can be quenched by binding with fluorophores via various mechanisms including resonance energy transfer, charge transfer, and electron transfer. Therefore, CQDs are the best candidate for sensing drugs and enzymes. Tracking of concentration of drugs in patients’ blood serum is necessary to observe various effects on the body as well for optimum therapeutic effect. Recently Shashahanipour et al. synthesized carbon dots from Lawsoniainermis (Henna) leave for highly sensitive detection of methotrexate (MTX), a widely used chemotherapy agent through fluorescence resonance energy transfer (FRET) (Shahshahanipour et al., 2019). Breast cancer is one of the most heterogeneous types of cancer. In this cancer, tumors exist with different morphology and gene expression. These phenomena affect the therapeutic and diagnostic practice significantly. So, there is an urgent need to track and monitor heterogenicity to avoid resistance to drugs as well as the therapeutic process. Pramanik et al. constructed CQDs from mango and prune for conjugation with Anti-HER2 antibodies for tracking heterogenicity in breast cancer (Pramanik et al., 2019).
The present review discusses and enlightens the recent applications of CQDs in cancer theranostics such as cell imaging, drug delivery, photoinduced therapy, and drug sensing. Although the applications of CQDs in cancer theranostics have grown rapidly in recent years, several challenges restrict their clinical uses in human due to toxicity and poor biocompatibility. Therefore, more eco-friendly, biocompatible, biodegradable, and one-step synthesis methods need to be discovered. Another major concern is the lack of study regarding genotoxicity, which must be investigated extensively so that CQDs can be directed into cell nuclei to destroy maladious genetic material and enhance anti-tumor therapy. Further, photoinduced therapy requires an imaging agent with a high absorption coefficient within the biological transparency window (650–950 nm), so there is an urgent requirement for non-toxic, biocompatible CQDs with emission in the NIR region. Also, targeting and inhibiting cancer tumors in the brain is still a major issue due to the blood-brain barrier which does not allow it to penetrate the central nervous system for most CQDs. So, there is a need to design highly specific CQDs for blood-brain barriers penetration. Moreover, obstacles such as bulk industrial production and cost-effective synthesis need to be solved urgently.
Apart from the above challenges, structural design and molecular interactions between biomolecules need to be studied to design more functional and tunable CQDs using self-assembly. Due to tremendous deforestation, we are losing priceless medicinal species of herbs and plants every day. So, it is essential to protect and preserve rare species of medicinal plants and herbs so that more of them can be investigated to synthesize CQDs with various anti-cancer properties.
Overall, in this review, we have discussed distinctive properties, synthesis methods, characterization techniques and cancer-targeting applications of CQDs derived from biomolecules and medicinal plants. These CQDs have a radiant future and scope in the biomedical field due to non-invasive treatment, tunable optical properties, and water solubility. Through the combination of scientists, industry, and the health sector, more focus should be on the fabrication of more biocompatible CQDs for clinical applications. Despite these challenges, we can conclude that intensive research, a sustainable approach, and an increase in the health consciousness of society will result in the application of CQDs almost everywhere in the biomedical sector.
KN collected the literature, designed figures and drafted the manuscript. SC and LY reviewed and modified draft. ASP edited final draft and supervised the research. All the authors contributed to the article and approved the submitted version.
The authors declare that the research was conducted in the absence of any commercial or financial relationships that could be construed as a potential conflict of interest.
All claims expressed in this article are solely those of the authors and do not necessarily represent those of their affiliated organizations, or those of the publisher, the editors and the reviewers. Any product that may be evaluated in this article, or claim that may be made by its manufacturer, is not guaranteed or endorsed by the publisher.
The authors would like to thanks the Department of Science and Technology (SERB), India-CRG/2019/000903(Core Research Grant) & SB/S2/RJN-140/2014 (Ramanujan Fellowship Award) for the financial support.
Alam, A.-M., Park, B.-Y., Ghouri, Z. K., Park, M., and Kim, H.-Y. (2015). Synthesis of Carbon Quantum Dots from Cabbage with Down- and Up-Conversion Photoluminescence Properties: Excellent Imaging Agent for Biomedical Applications. Green Chem. 17, 3791–3797. doi:10.1039/c5gc00686d
Alarfaj, N. A., El-Tohamy, M. F., and Oraby, H. F. (2020). New Immunosensing-Fluorescence Detection of Tumor Marker Cytokeratin-19 Fragment (CYFRA 21-1) via Carbon Quantum Dots/Zinc Oxide Nanocomposite. Nanoscale Res. Lett. 15, 12. doi:10.1186/s11671-020-3247-9
Alarfaj, N., El-Tohamy, M., and Oraby, H. (2018). CA 19-9 Pancreatic Tumor Marker Fluorescence Immunosensing Detection via Immobilized Carbon Quantum Dots Conjugated Gold Nanocomposite. Int. J. Mol. Sci. 19, 1162. doi:10.3390/ijms19041162
Arkan, E., Barati, A., Rahmanpanah, M., Hosseinzadeh, L., Moradi, S., and Hajialyani, M. (2018). Green Synthesis of Carbon Dots Derived from Walnut Oil and an Investigation of Their Cytotoxic and Apoptogenic Activities toward Cancer Cells. Adv. Pharm. Bull. 8, 149–155. doi:10.15171/apb.2018.018
Arumugham, T., Alagumuthu, M., Amimodu, R. G., Munusamy, S., and Iyer, S. K. (2020). A Sustainable Synthesis of Green Carbon Quantum Dot (CQD) from Catharanthus Roseus (White Flowering Plant) Leaves and Investigation of its Dual Fluorescence Responsive Behavior in Multi-Ion Detection and Biological Applications. Sustain. Mater. Technol. 23, e00138. doi:10.1016/j.susmat.2019.e00138
Aslandaş, A. M., Balcı, N., Arık, M., Şakiroğlu, H., Onganer, Y., and Meral, K. (2015). Liquid Nitrogen-Assisted Synthesis of Fluorescent Carbon Dots from Blueberry and Their Performance in Fe 3+ Detection. Appl. Surf. Sci. 356, 747–752. doi:10.1016/j.apsusc.2015.08.147
Atchudan, R., Edison, T. N. J. I., Aseer, K. R., Perumal, S., and Lee, Y. R. (2018). Hydrothermal Conversion of Magnolia Liliiflora into Nitrogen-Doped Carbon Dots as an Effective Turn-Off Fluorescence Sensing, Multi-Colour Cell Imaging and Fluorescent Ink. Colloids Surfaces B Biointerfaces 169, 321–328. doi:10.1016/j.colsurfb.2018.05.032
Bayda, S., Amadio, E., Cailotto, S., Frión-Herrera, Y., Perosa, A., and Rizzolio, F. (2021). Carbon Dots for Cancer Nanomedicine: a Bright Future. Nanoscale Adv. 3, 5183–5221. doi:10.1039/d1na00036e
Bhunia, S. K., Pradhan, N., and Jana, N. R. (2014). Vitamin B1 Derived Blue and Green Fluorescent Carbon Nanoparticles for Cell-Imaging Application. ACS Appl. Mat. Interfaces 6, 7672–7679. doi:10.1021/am500964d
Carneiro, S. V., de Queiroz, V. H. R., Cruz, A. A. C., Fechine, L. M. U. D., Denardin, J. C., Freire, R. M., et al. (2019). Sensing Strategy Based on Carbon Quantum Dots Obtained from Riboflavin for the Identification of Pesticides. Sensors Actuators B Chem. 301, 127149. doi:10.1016/j.snb.2019.127149
Chah, K. F., Eze, C. A., Emuelosi, C. E., and Esimone, C. O. (2006). Antibacterial and Wound Healing Properties of Methanolic Extracts of Some Nigerian Medicinal Plants. J. Ethnopharmacol. 104, 164–167. doi:10.1016/j.jep.2005.08.070
Chakraborty, D., Sarkar, S., and Das, P. K. (2018). Blood Dots: Hemoglobin-Derived Carbon Dots as Hydrogen Peroxide Sensors and Pro-drug Activators. ACS Sustain. Chem. Eng. 6, 4661–4670. doi:10.1021/acssuschemeng.7b03691
Chiu, S.-H., Gedda, G., Girma, W. M., Chen, J.-K., Ling, Y.-C., Ghule, A. V., et al. (2016). Rapid Fabrication of Carbon Quantum Dots as Multifunctional Nanovehicles for Dual-Modal Targeted Imaging and Chemotherapy. Acta Biomater. 46, 151–164. doi:10.1016/j.actbio.2016.09.027
Choi, Y., Kim, S., Choi, M.-H., Ryoo, S.-R., Park, J., Min, D.-H., et al. (2014). Highly Biocompatible Carbon Nanodots for Simultaneous Bioimaging and Targeted Photodynamic Therapy In Vitro and In Vivo. Adv. Funct. Mat. 24, 5781–5789. doi:10.1002/adfm.201400961
Dehghani, A., Ardekani, S. M., Hassan, M., and Gomes, V. G. (2018). Collagen Derived Carbon Quantum Dots for Cell Imaging in 3D Scaffolds via Two-Photon Spectroscopy. Carbon 131, 238–245. doi:10.1016/j.carbon.2018.02.006
Demir-Cakan, R., Baccile, N., Antonietti, M., and Titirici, M.-M. (2009). Carboxylate-rich Carbonaceous Materials via One-step Hydrothermal Carbonization of Glucose in the Presence of Acrylic Acid. Chem. Mat. 21, 484–490. doi:10.1021/cm802141h
Ding, H., Du, F., Liu, P., Chen, Z., and Shen, J. (2015). DNA-carbon Dots Function as Fluorescent Vehicles for Drug Delivery. ACS Appl. Mat. Interfaces 7, 6889–6897. doi:10.1021/acsami.5b00628
Ehtesabi, H., Hallaji, Z., Najafi Nobar, S., and Bagheri, Z. (2020). Carbon Dots with pH-Responsive Fluorescence: a Review on Synthesis and Cell Biological Applications. Microchim. Acta 187, 150. doi:10.1007/s00604-019-4091-4
Ferlay, J., Colombet, M., Soerjomataram, I., Mathers, C., Parkin, D. M., Piñeros, M., et al. (2019). Estimating the Global Cancer Incidence and Mortality in 2018: GLOBOCAN Sources and Methods. Int. J. Cancer 144, 1941–1953. doi:10.1002/ijc.31937
Ferroni, C., Del Rio, A., Martini, C., Manoni, E., and Varchi, G. (2019). Light-Induced Therapies for Prostate Cancer Treatment. Front. Chem. 7, 719. doi:10.3389/fchem.2019.00719
Gaddam, R. R., Mukherjee, S., Punugupati, N., Vasudevan, D., Patra, C. R., Narayan, R., et al. (2017). Facile Synthesis of Carbon Dot and Residual Carbon Nanobeads: Implications for Ion Sensing, Medicinal and Biological Applications. Mater. Sci. Eng. C 73, 643–652. doi:10.1016/j.msec.2016.12.095
Gong, J., An, X., and Yan, X. (2014). A Novel Rapid and Green Synthesis of Highly Luminescent Carbon Dots with Good Biocompatibility for Cell Imaging. New J. Chem. 38, 1376–1379. doi:10.1039/c3nj01320k
Gong, X., Zhang, Q., Gao, Y., Shuang, S., Choi, M. M. F., and Dong, C. (2016). Phosphorus and Nitrogen Dual-Doped Hollow Carbon Dot as a Nanocarrier for Doxorubicin Delivery and Biological Imaging. ACS Appl. Mat. Interfaces 8, 11288–11297. doi:10.1021/acsami.6b01577
Guha, P., and Guha, P. (2017). Betel Leaf: The Neglected Green Gold of India. J. Hum. Ecol. 19, 87–93. doi:10.1080/09709274.2006.11905861
Guo, C. X., Xie, J., Wang, B., Zheng, X., Yang, H. B., and Li, C. M. (2013). A New Class of Fluorescent-Dots: Long Luminescent Lifetime Bio-Dots Self-Assembled from DNA at Low Temperatures. Sci. Rep. 3, 2957. doi:10.1038/srep02957
Guo, L., Li, L., Liu, M., Wan, Q., Tian, J., Huang, Q., et al. (2018). Bottom-up Preparation of Nitrogen Doped Carbon Quantum Dots with Green Emission under Microwave-Assisted Hydrothermal Treatment and Their Biological Imaging. Mater. Sci. Eng. C 84, 60–66. doi:10.1016/j.msec.2017.11.034
Gusain, D., Renuka, N., Guldhe, A., and Bux, F. (2021). Use of Microalgal Lipids and Carbohydrates for the Synthesis of Carbon Dots via Hydrothermal Microwave Treatment. Inorg. Chem. Commun. 134, 109021. doi:10.1016/j.inoche.2021.109021
Han, S., Zhang, H., Xie, Y., Liu, L., Shan, C., Li, X., et al. (2015). Application of Cow Milk-Derived Carbon dots/Ag NPs Composite as the Antibacterial Agent. Appl. Surf. Sci. 328, 368–373. doi:10.1016/j.apsusc.2014.12.074
He, Z., Cheng, J., Yan, W., Long, W., Ouyang, H., Hu, X., et al. (2021). One-step Preparation of Green Tea Ash Derived and Polymer Functionalized Carbon Quantum Dots via the Thiol-Ene Click Chemistry. Inorg. Chem. Commun. 130, 108743. doi:10.1016/j.inoche.2021.108743
Hsu, P.-C., Shih, Z.-Y., Lee, C.-H., and Chang, H.-T. (2012). Synthesis and Analytical Applications of Photoluminescent Carbon Nanodots. Green Chem. 14, 917–920. doi:10.1039/c2gc16451e
Hu, S., Trinchi, A., Atkin, P., and Cole, I. (2015). Tunable Photoluminescence across the Entire Visible Spectrum from Carbon Dots Excited by White Light. Angew. Chem. Int. Ed. 54, 2970–2974. doi:10.1002/anie.201411004
Hua, X.-W., Bao, Y.-W., and Wu, F.-G. (2018a). Fluorescent Carbon Quantum Dots with Intrinsic Nucleolus-Targeting Capability for Nucleolus Imaging and Enhanced Cytosolic and Nuclear Drug Delivery. ACS Appl. Mat. Interfaces 10, 10664–10677. doi:10.1021/acsami.7b19549
Hua, X.-W., Bao, Y.-W., Zeng, J., and Wu, F.-G. (2018b). Ultrasmall All-In-One Nanodots Formed via Carbon Dot-Mediated and Albumin-Based Synthesis: Multimodal Imaging-Guided and Mild Laser-Enhanced Cancer Therapy. ACS Appl. Mat. Interfaces 10, 42077–42087. doi:10.1021/acsami.8b16065
Huang, H., Li, C., Zhu, S., Wang, H., Chen, C., Wang, Z., et al. (2014). Histidine-Derived Nontoxic Nitrogen-Doped Carbon Dots for Sensing and Bioimaging Applications. Langmuir 30, 13542–13548. doi:10.1021/la503969z
Itharat, A., Houghton, P. J., Eno-amooquaye, E., Burke, P. J., Sampson, J. H., and Raman, A. (2004). In Vitro cytotoxic Activity of Thai Medicinal Plants Used Traditionally to Treat Cancer. J. Ethnopharmacol. 90, 33–38. doi:10.1016/j.jep.2003.09.014
Jamshidi-kia, F., Lorigooini, Z., and Amini-khoei, H. (2018). Medicinal Plants: Past History and Future Perspective. J. Herbmed Pharmacol. 7, 1–7. doi:10.15171/jhp.2018.01
Jha, A., Viswanadh, M. K., Burande, A. S., Mehata, A. K., Poddar, S., Yadav, K., et al. (2020). DNA Biodots Based Targeted Theranostic Nanomedicine for the Imaging and Treatment of Non-small Cell Lung Cancer. Int. J. Biol. Macromol. 150, 413–425. doi:10.1016/j.ijbiomac.2020.02.075
Jian, H.-J., Yu, J., Li, Y.-J., Unnikrishnan, B., Huang, Y.-F., Luo, L.-J., et al. (2020). Highly Adhesive Carbon Quantum Dots from Biogenic Amines for Prevention of Biofilm Formation. Chem. Eng. J. 386, 123913. doi:10.1016/j.cej.2019.123913
Jiang, J., He, Y., Li, S., and Cui, H. (2012). Amino Acids as the Source for Producing Carbon Nanodots: Microwave Assisted One-step Synthesis, Intrinsic Photoluminescence Property and Intense Chemiluminescence Enhancement. Chem. Commun. 48, 9634–9636. doi:10.1039/c2cc34612e
Jiang, Y., Han, Q., Jin, C., Zhang, J., and Wang, B. (2015). A Fluorescence Turn-Off Chemosensor Based on N-Doped Carbon Quantum Dots for Detection of Fe3+ in Aqueous Solution. Mater. Lett. 141, 366–368. doi:10.1016/j.matlet.2014.10.168
Jung, Y. K., Shin, E., and Kim, B.-S. (2015). Cell Nucleus-Targeting Zwitterionic Carbon Dots. Sci. Rep. 5, 1–9. doi:10.1038/srep18807
Karfa, P., Roy, E., Patra, S., Kumar, S., Tarafdar, A., Madhuri, R., et al. (2015). Retracted Article: Amino Acid Derived Highly Luminescent, Heteroatom-Doped Carbon Dots for Label-free Detection of Cd2+/Fe3+, Cell Imaging and Enhanced Antibacterial Activity. RSC Adv. 5, 58141–58153. doi:10.1039/c5ra09525e
Kim, D., Jo, G., Chae, Y., Subramani, S., Lee, B. Y., Kim, E. J., et al. (2021). Bioinspired Camellia Japonica Carbon Dots with High Near-Infrared Absorbance for Efficient Photothermal Cancer Therapy. Nanoscale 13, 14426–14434. doi:10.1039/d1nr03999g
Kumar, A., Chowdhuri, A. R., Laha, D., Mahto, T. K., Karmakar, P., and Sahu, S. K. (2017a). Green Synthesis of Carbon Dots from Ocimum Sanctum for Effective Fluorescent Sensing of Pb2+ Ions and Live Cell Imaging. Sensors Actuators B Chem. 242, 679–686. doi:10.1016/j.snb.2016.11.109
Kumar, V. B., Sheinberger, J., Porat, Z., Shav-Tal, Y., and Gedanken, A. (2016). A Hydrothermal Reaction of an Aqueous Solution of BSA Yields Highly Fluorescent N Doped C-Dots Used for Imaging of Live Mammalian Cells. J. Mat. Chem. B 4, 2913–2920. doi:10.1039/c6tb00519e
Kumarapppan, C., Jaswanth, A., and Kumarasunderi, K. (2011). Antihaemolytic and Snake Venom Neutralizing Effect of Some Indian Medicinal Plants. Asian Pac. J. Trop. Med. 4, 743–747. doi:10.1016/S1995-7645(11)60185-5
Lapshina, N., Shishkin, I. I., Nandi, R., Noskov, R. E., Barhom, H., Joseph, S., et al. (2019). Bioinspired Amyloid Nanodots with Visible Fluorescence. Adv. Opt. Mater. 7, 1801400. doi:10.1002/adom.201801400
Lee, H. U., Park, S. Y., Park, E. S., Son, B., Lee, S. C., Lee, J. W., et al. (2014). Photoluminescent Carbon Nanotags from Harmful Cyanobacteria for Drug Delivery and Imaging in Cancer Cells. Sci. Rep. 4, 1–7. doi:10.1038/srep04665
Lekhak, S., and Sharma, A. (2009). Antibacterial Property of Different Medicinal Plants : Ocimum Sanctum , Cinnamomum Zeylanicum. Xanthoxylum armatum Origanum majorana 5, 143–150.
Li, C.-L., Ou, C.-M., Huang, C.-C., Wu, W.-C., Chen, Y.-P., Lin, T.-E., et al. (2014). Carbon Dots Prepared from Ginger Exhibiting Efficient Inhibition of Human Hepatocellular Carcinoma Cells. J. Mat. Chem. B 2, 4564–4571. doi:10.1039/c4tb00216d
Li, H., He, X., Liu, Y., Huang, H., Lian, S., Lee, S.-T., et al. (2011). One-step Ultrasonic Synthesis of Water-Soluble Carbon Nanoparticles with Excellent Photoluminescent Properties. Carbon 49, 605–609. doi:10.1016/j.carbon.2010.10.004
Li, P., Han, F., Cao, W., Zhang, G., Li, J., Zhou, J., et al. (2020). Carbon Quantum Dots Derived from Lysine and Arginine Simultaneously Scavenge Bacteria and Promote Tissue Repair. Appl. Mater. Today 19, 100601. doi:10.1016/j.apmt.2020.100601
Li, Y., Li, W., Yang, X., Kang, Y., Zhang, H., Liu, Y., et al. (2021). Salvia Miltiorrhiza-Derived Carbon Dots as Scavengers of Reactive Oxygen Species for Reducing Oxidative Damage of Plants. ACS Appl. Nano Mat. 4, 113–120. doi:10.1021/acsanm.0c02419
Li, Y., Tang, Z., Pan, Z., Wang, R., Wang, X., Zhao, P., et al. (2022). Calcium-Mobilizing Properties of Salvia Miltiorrhiza-Derived Carbon Dots Confer Enhanced Environmental Adaptability in Plants. ACS Nano 16, 4357–4370. doi:10.1021/acsnano.1c10556
Li, Y., Zhong, X., Rider, A. E., Furman, S. A., and Ostrikov, K. (2014). Fast, Energy-Efficient Synthesis of Luminescent Carbon Quantum Dots. Green Chem. 16, 2566–2570. doi:10.1039/c3gc42562b
Liang, Q., Ma, W., Shi, Y., Li, Z., and Yang, X. (2013). Easy Synthesis of Highly Fluorescent Carbon Quantum Dots from Gelatin and Their Luminescent Properties and Applications. Carbon 60, 421–428. doi:10.1016/j.carbon.2013.04.055
Limosani, F., Bauer, E. M., Cecchetti, D., Biagioni, S., Orlando, V., Pizzoferrato, R., et al. (2021). Top-Down N-Doped Carbon Quantum Dots for Multiple Purposes: Heavy Metal Detection and Intracellular Fluorescence. Nanomaterials 11, 2249. doi:10.3390/nano11092249
Liu, C., Zhang, P., Tian, F., Li, W., Li, F., and Liu, W. (2011). One-step Synthesis of Surface Passivated Carbon Nanodots by Microwave Assisted Pyrolysis for Enhanced Multicolor Photoluminescence and Bioimaging. J. Mat. Chem. 21, 13163–13167. doi:10.1039/c1jm12744f
Liu, H., Li, Z., Sun, Y., Geng, X., Hu, Y., Meng, H., et al. (2018). Synthesis of Luminescent Carbon Dots with Ultrahigh Quantum Yield and Inherent Folate Receptor-Positive Cancer Cell Targetability. Sci. Rep. 8, 1086. doi:10.1038/s41598-018-19373-3
Liu, X., Li, T., Hou, Y., Wu, Q., Yi, J., and Zhang, G. (2016a). Microwave Synthesis of Carbon Dots with Multi-Response Using Denatured Proteins as Carbon Source. RSC Adv. 6, 11711–11718. doi:10.1039/c5ra23081k
Liu, X., Pang, J., Xu, F., and Zhang, X. (2016b). Simple Approach to Synthesize Amino-Functionalized Carbon Dots by Carbonization of Chitosan. Sci. Rep. 6, 31100. doi:10.1038/srep31100
Luo, L., Song, T., Wang, H., Yuan, Q., and Zhou, S. (2018). A Highly Selective Fluorescence Sensing Platform for Nanomolar Hg(II) Detection Based on Cytosine Derived Quantum Dot. Spectrochimica Acta Part A Mol. Biomol. Spectrosc. 193, 95–101. doi:10.1016/j.saa.2017.11.044
Ma, J., Kang, K., Zhang, Y., Yi, Q., and Gu, Z. (2018). Detachable Polyzwitterion-Coated Ternary Nanoparticles Based on Peptide Dendritic Carbon Dots for Efficient Drug Delivery in Cancer Therapy. ACS Appl. Mat. Interfaces 10, 43923–43935. doi:10.1021/acsami.8b17041
Ma, Z., Ming, H., Huang, H., Liu, Y., and Kang, Z. (2012). One-step Ultrasonic Synthesis of Fluorescent N-Doped Carbon Dots from Glucose and Their Visible-Light Sensitive Photocatalytic Ability. New J. Chem. 36, 861–864. doi:10.1039/c2nj20942j
Mahmoud, M. E., Fekry, N. A., and Abdelfattah, A. M. (2020). Removal of Uranium (VI) from Water by the Action of Microwave-Rapid Green Synthesized Carbon Quantum Dots from Starch-Water System and Supported onto Polymeric Matrix. J. Hazard. Mater. 397, 122770. doi:10.1016/j.jhazmat.2020.122770
Malavika, J. P., Shobana, C., Ragupathi, M., Kumar, P., Lee, Y. S., Govarthanan, M., et al. (2021). A Sustainable Green Synthesis of Functionalized Biocompatible Carbon Quantum Dots from Aloe Barbadensis Miller and its Multifunctional Applications. Environ. Res. 200, 111414. doi:10.1016/j.envres.2021.111414
Meena, R., Singh, R., Marappan, G., Kushwaha, G., Gupta, N., Meena, R., et al. (2019). Fluorescent Carbon Dots Driven from Ayurvedic Medicinal Plants for Cancer Cell Imaging and Phototherapy. Heliyon 5, e02483. doi:10.1016/j.heliyon.2019.e02483
Meirow, D., and Nugent, D. (2001). The Effects of Radiotherapy and Chemotherapy on Female Reproduction. Hum. Reprod. Update 7, 535–543. doi:10.1093/humupd/7.6.535
Mohajeri, N., Mostafavi, E., and Zarghami, N. (2020). The Feasibility and Usability of DNA-Dot Bioconjugation to Antibody for Targeted In Vitro Cancer Cell Fluorescence Imaging. J. Photochem. Photobiol. B Biol. 209, 111944. doi:10.1016/j.jphotobiol.2020.111944
Naik, G. G., Alam, M. B., Pandey, V., Mohapatra, D., Dubey, P. K., Parmar, A. S., et al. (2020). Multi-Functional Carbon Dots from an Ayurvedic Medicinal Plant for Cancer Cell Bioimaging Applications. J. Fluoresc. 30, 407–418. doi:10.1007/s10895-020-02515-0
Naik, G. G., Shah, J., Balasubramaniam, A. K., and Sahu, A. N. (2021). Applications of Natural Product-Derived Carbon Dots in Cancer Biology. Nanomedicine 16, 587–608. doi:10.2217/nnm-2020-0424
Nasrin, A., Hassan, M., Mann, G., and Gomes, V. G. (2020). Conjugated Ternary Doped Carbon Dots from Vitamin B Derivative: Multispectral Nanoprobes for Targeted Melanoma Bioimaging and Photosensitization. J. Luminescence 217, 116811. doi:10.1016/j.jlumin.2019.116811
Nawaz, F., Wang, L., Zhu, L.-f., Meng, X.-j., and Xiao, F.-S. (2013). Ascorbic Acid Assisted Green Route for Synthesis of Water Dispersible Carbon Dots. Chem. Res. Chin. Univ. 29, 401–403. doi:10.1007/s40242-013-2339-9
Ndlovu, G., Fouche, G., Tselanyane, M., Cordier, W., and Steenkamp, V. (2013). In Vitro determination of the Anti-aging Potential of Four Southern African Medicinal Plants. BMC Complement. Altern. Med. 13, 304. doi:10.1186/1472-6882-13-304
Niino, S., Takeshita, S., Iso, Y., and Isobe, T. (2016). Influence of Chemical States of Doped Nitrogen on Photoluminescence Intensity of Hydrothermally Synthesized Carbon Dots. J. Luminescence 180, 123–131. doi:10.1016/j.jlumin.2016.08.021
Otzen, D. E. (2002). Protein Unfolding in Detergents: Effect of Micelle Structure, Ionic Strength, pH, and Temperature. Biophysical J. 83, 2219–2230. doi:10.1016/S0006-3495(02)73982-9
Park, H.-R., Park, S. B., Hong, H.-D., Suh, H. J., and Shin, K.-S. (2017). Structural Elucidation of Anti-metastatic Rhamnogalacturonan II from the Pectinase Digest of Citrus Peels (Citrus Unshiu). Int. J. Biol. Macromol. 94, 161–169. doi:10.1016/j.ijbiomac.2016.09.100
Park, S. Y., Lee, H. U., Park, E. S., Lee, S. C., Lee, J.-W., Jeong, S. W., et al. (2014). Photoluminescent Green Carbon Nanodots from Food-Waste-Derived Sources: Large-Scale Synthesis, Properties, and Biomedical Applications. ACS Appl. Mat. Interfaces 6, 3365–3370. doi:10.1021/am500159p
Pearce, A., Haas, M., Viney, R., Pearson, S., Haywood, P., Brown, C., et al. (2017). Mức Độ Nghiêm Trọng Của Tác Dụng Phụ.Pdf, 1–12.
Pei, S., Zhang, J., Gao, M., Wu, D., Yang, Y., and Liu, R. (2015). A Facile Hydrothermal Approach towards Photoluminescent Carbon Dots from Amino Acids. J. Colloid Interface Sci. 439, 129–133. doi:10.1016/j.jcis.2014.10.030
Peng, H., and Travas-Sejdic, J. (2009). Simple Aqueous Solution Route to Luminescent Carbogenic Dots from Carbohydrates. Chem. Mat. 21, 5563–5565. doi:10.1021/cm901593y
Peng, Z., Han, X., Li, S., Al-youbi, A. O., Bashammakh, A. S., El-shahawi, M. S., et al. (2017). Carbon Dots: Biomacromolecule Interaction, Bioimaging and Nanomedicine. Coord. Chem. Rev. 343, 256–277. doi:10.1016/j.ccr.2017.06.001
Pramanik, A., Begum, S., Rightsell, C., Gates, K., Zhang, Q., Jones, S., et al. (2019). Designing Highly Crystalline Multifunctional Multicolor-Luminescence Nanosystem for Tracking Breast Cancer Heterogeneity. Nanoscale Adv. 1, 1021–1034. doi:10.1039/c8na00089a
Qiao, L., Sun, T., Zheng, X., Zheng, M., and Xie, Z. (2018). Exploring the Optimal Ratio of D-glucose/L-Aspartic Acid for Targeting Carbon Dots toward Brain Tumor Cells. Mater. Sci. Eng. C 85, 1–6. doi:10.1016/j.msec.2017.12.011
Qu, Z., Liu, L., Sun, T., Hou, J., Sun, Y., Yu, M., et al. (2020). Synthesis of Bifunctional Carbon Quantum Dots for Bioimaging and Anti-inflammation. Nanotechnology 31, 175102. doi:10.1088/1361-6528/ab6b9d
Radad, K., Gille, G., Liu, L., and Rausch, W.-D. (2006). Use of Ginseng in Medicine with Emphasis on Neurodegenerative Disorders. J. Pharmacol. Sci. 100, 175–186. doi:10.1254/jphs.CRJ05010X
Raksha, B. (2014). Bioactive Compounds and Medicinal Properties of Aloe Vera L.: An Update. J. Plant Sci. 2, 102. doi:10.11648/j.jps.20140203.11
Ramezani, Z., Qorbanpour, M., and Rahbar, N. (2018). Green Synthesis of Carbon Quantum Dots Using Quince Fruit (Cydonia Oblonga) Powder as Carbon Precursor: Application in Cell Imaging and As3+ Determination. Colloids Surfaces A Physicochem. Eng. Aspects 549, 58–66. doi:10.1016/j.colsurfa.2018.04.006
Ravipati, A. S., Zhang, L., Koyyalamudi, S. R., Jeong, S. C., Reddy, N., Bartlett, J., et al. (2012). Antioxidant and Anti-inflammatory Activities of Selected Chinese Medicinal Plants and Their Relation with Antioxidant Content. BMC Complement. Altern. Med. 12, 5–10. doi:10.1186/1472-6882-12-173
Ren, X., Zhang, F., Guo, B., Gao, N., and Zhang, X. (2019). Synthesis of N-Doped Micropore Carbon Quantum Dots with High Quantum Yield and Dual-Wavelength Photoluminescence Emission from Biomass for Cellular Imaging. Nanomaterials 9, 22–25. doi:10.3390/nano9040495
Rojas-valencia, O. G., Regules-carrasco, M., Hernández-Fuentes, J., Germán, C. M. R.-S., Estrada-Flores, M., and Villagarcía-Chávez, E. (2021). Synthesis of Blue Emissive Carbon Quantum Dots from Hibiscus Sabdariffa Flower: Surface Functionalization Analysis by FT-IR Spectroscopy. Materialia 19, 101182. doi:10.1016/j.mtla.2021.101182
Sachdev, A., and Gopinath, P. (2015). Green Synthesis of Multifunctional Carbon Dots from Coriander Leaves and Their Potential Application as Antioxidants, Sensors and Bioimaging Agents. Analyst 140, 4260–4269. doi:10.1039/c5an00454c
Sahu, S., Behera, B., Maiti, T. K., and Mohapatra, S. (2012). Simple One-step Synthesis of Highly Luminescent Carbon Dots from Orange Juice: Application as Excellent Bio-Imaging Agents. Chem. Commun. 48, 8835–8837. doi:10.1039/c2cc33796g
Sai, L., Chen, J., Chang, Q., Shi, W., Chen, Q., and Huang, L. (2017). Protein-derived Carbon Nanodots with an Ethylenediamine-Modulated Structure as Sensitive Fluorescent Probes for Cu2+detection. RSC Adv. 7, 16608–16615. doi:10.1039/c7ra01441d
Samimi, S., Ardestani, M. S., and Dorkoosh, F. A. (2021). Preparation of Carbon Quantum Dots- Quinic Acid for Drug Delivery of Gemcitabine to Breast Cancer Cells. J. Drug Deliv. Sci. Technol. 61, 102287. doi:10.1016/j.jddst.2020.102287
Schneider, J., Reckmeier, C. J., Xiong, Y., von Seckendorff, M., Susha, A. S., Kasák, P., et al. (2017). Molecular Fluorescence in Citric Acid-Based Carbon Dots. J. Phys. Chem. C 121, 2014–2022. doi:10.1021/acs.jpcc.6b12519
Shahshahanipour, M., Rezaei, B., Ensafi, A. A., and Etemadifar, Z. (2019). An Ancient Plant for the Synthesis of a Novel Carbon Dot and its Applications as an Antibacterial Agent and Probe for Sensing of an Anti-cancer Drug. Mater. Sci. Eng. C 98, 826–833. doi:10.1016/j.msec.2019.01.041
Shi, C., Qi, H., Ma, R., Sun, Z., Xiao, L., Wei, G., et al. (2019). N,S-self-doped Carbon Quantum Dots from Fungus Fibers for Sensing Tetracyclines and for Bioimaging Cancer Cells. Mater. Sci. Eng. C 105, 110132. doi:10.1016/j.msec.2019.110132
Shi, L., Yang, J. H., Zeng, H. B., Chen, Y. M., Yang, S. C., Wu, C., et al. (2016). Carbon Dots with High Fluorescence Quantum Yield: The Fluorescence Originates from Organic Fluorophores. Nanoscale 8, 14374–14378. doi:10.1039/c6nr00451b
Shukla, D., Das, M., Kasade, D., Pandey, M., Dubey, A. K., Yadav, S. K., et al. (2020). Sandalwood-derived Carbon Quantum Dots as Bioimaging Tools to Investigate the Toxicological Effects of Malachite Green in Model Organisms. Chemosphere 248, 125998. doi:10.1016/j.chemosphere.2020.125998
Şimşek, S., Şüküroğlu, A. A., Yetkin, D., Özbek, B., Battal, D., and Genç, R. (2020). DNA-damage and Cell Cycle Arrest Initiated Anti-cancer Potency of Super Tiny Carbon Dots on MCF7 Cell Line. Sci. Rep. 10, 1–14. doi:10.1038/s41598-020-70796-3
Singh, A., Mallika, T. N., Gorain, B., Yadav, A. K., Tiwari, S., Flora, S. J. S., et al. (2021). Quantum Dot: Heralding a Brighter Future in Neurodegenerative Disorders. J. Drug Deliv. Sci. Technol. 65, 102700. doi:10.1016/j.jddst.2021.102700
Song, T., Zhu, X., Zhou, S., Yang, G., Gan, W., and Yuan, Q. (2015). DNA Derived Fluorescent Bio-Dots for Sensitive Detection of Mercury and Silver Ions in Aqueous Solution. Appl. Surf. Sci. 347, 505–513. doi:10.1016/j.apsusc.2015.04.143
Song, Y., Li, X., Cong, S., Zhao, H., and Tan, M. (2019). Nuclear-targeted of TAT Peptide-Conjugated Carbon Dots for Both One-And Two-Photon Fluorescence Imaging. Colloids Surfaces B Biointerfaces 180, 449–456. doi:10.1016/j.colsurfb.2019.05.015
Srinivas Naik, L., Shyam, P., Paul Marx, K., Baskari, S., and Devi, C. V. R. (2015). Antimicrobial Activity and Phytochemical Analysis of Ocimum Tenuiflorum Leaf Extract. Int. J. PharmTech Res. 8, 88–95.
Su, W., Guo, R., Yuan, F., Li, Y., Li, X., Zhang, Y., et al. (2020). Red-Emissive Carbon Quantum Dots for Nuclear Drug Delivery in Cancer Stem Cells. J. Phys. Chem. Lett. 11, 1357–1363. doi:10.1021/acs.jpclett.9b03891
Sun, S., Klebaner, F., Zhang, X., and Tian, T. (2018). Instantaneous Mutation Rate in Cancer Initiation and Progression. BMC Syst. Biol. 12, 110. doi:10.1186/s12918-018-0629-z
Sung, H., Ferlay, J., Siegel, R. L., Laversanne, M., Soerjomataram, I., Jemal, A., et al. (2021). Global Cancer Statistics 2020: GLOBOCAN Estimates of Incidence and Mortality Worldwide for 36 Cancers in 185 Countries. CA A Cancer J. Clin. 71, 209–249. doi:10.3322/caac.21660
Tadesse, A., Hagos, M., Ramadevi, D., Basavaiah, K., and Belachew, N. (2020). Fluorescent-Nitrogen-Doped Carbon Quantum Dots Derived from Citrus Lemon Juice: Green Synthesis, Mercury(II) Ion Sensing, and Live Cell Imaging. ACS Omega 5, 3889–3898. doi:10.1021/acsomega.9b03175
Tan, M., Li, X., Wu, H., Wang, B., and Wu, J. (2015). N-doped Carbon Dots Derived from Bovine Serum Albumin and Formic Acid with One- and Two-Photon Fluorescence for Live Cell Nuclear Imaging. Colloids Surfaces B Biointerfaces 136, 141–149. doi:10.1016/j.colsurfb.2015.09.008
Teradal, N. L., and Jelinek, R. (2017). Carbon Nanomaterials in Biological Studies and Biomedicine. Adv. Healthc. Mat. 6, 1–36. doi:10.1002/adhm.201700574
Tian, L., Li, Z., Wang, P., Zhai, X., Wang, X., and Li, T. (2021). Carbon Quantum Dots for Advanced Electrocatalysis. J. Energy Chem. 55, 279–294. doi:10.1016/j.jechem.2020.06.057
Vandarkuzhali, S. A. A., Jeyalakshmi, V., Sivaraman, G., Singaravadivel, S., Krishnamurthy, K. R., and Viswanathan, B. (2017). Highly Fluorescent Carbon Dots from Pseudo-stem of Banana Plant: Applications as Nanosensor and Bio-Imaging Agents. Sensors Actuators B Chem. 252, 894–900. doi:10.1016/j.snb.2017.06.088
Vemuri, S. K., Banala, R. R., Subbaiah, G. P. V., Srivastava, S. K., Reddy, A. V. G., and Malarvili, T. (2017b). Anti-cancer Potential of a Mix of Natural Extracts of Turmeric, Ginger and Garlic: A Cell-Based Study. Egypt. J. Basic Appl. Sci. 4, 332–344. doi:10.1016/j.ejbas.2017.07.005
Wang, C., Shi, H., Yang, M., Yan, Y., Liu, E., Ji, Z., et al. (2020a). Facile Synthesis of Novel Carbon Quantum Dots from Biomass Waste for Highly Sensitive Detection of Iron Ions. Mater. Res. Bull. 124, 110730. doi:10.1016/j.materresbull.2019.110730
Wang, C., and Wallace, G. G. (2015). Flexible Electrodes and Electrolytes for Energy Storage. Electrochimica Acta 175, 87–95. doi:10.1016/j.electacta.2015.04.067
Wang, D., Zhu, L., Mccleese, C., Burda, C., Chen, J.-F., and Dai, L. (2016a). Fluorescent Carbon Dots from Milk by Microwave Cooking. RSC Adv. 6, 41516–41521. doi:10.1039/c6ra06120f
Wang, M., Tsukamoto, M., Sergeyev, V. G., and Zinchenko, A. (2021). Metal Ions Sensing by Biodots Prepared from DNA, RNA, and Nucleotides. Biosensors 11, 333. doi:10.3390/bios11090333
Wang, Y., Guo, G., Gao, J., Li, Z., Yin, X., Zhu, C., et al. (2020b). Multicenter-Emitting Carbon Dots: Color Tunable Fluorescence and Dynamics Monitoring Oxidative Stress In Vivo. Chem. Mat. 32, 8146–8157. doi:10.1021/acs.chemmater.0c01391
Wang, Y., and Hu, A. (2014). Carbon Quantum Dots: Synthesis, Properties and Applications. J. Mat. Chem. C 2, 6921–6939. doi:10.1039/c4tc00988f
Wang, Y., Li, Y., Yan, Y., Xu, J., Guan, B., Wang, Q., et al. (2013). Luminescent Carbon Dots in a New Magnesium Aluminophosphate Zeolite. Chem. Commun. 49, 9006–9008. doi:10.1039/c3cc43375g
Wang, Y., Shi, Z., and Yin, J. (2011). Facile Synthesis of Soluble Graphene via a Green Reduction of Graphene Oxide in Tea Solution and its Biocomposites. ACS Appl. Mat. Interfaces 3, 1127–1133. doi:10.1021/am1012613
Wang, Z., Fu, B., Zou, S., Duan, B., Chang, C., Yang, B., et al. (2016b). Facile Construction of Carbon Dots via Acid Catalytic Hydrothermal Method and Their Application for Target Imaging of Cancer Cells. Nano Res. 9, 214–223. doi:10.1007/s12274-016-0992-2
Wei, X., Li, L., Liu, J., Yu, L., Li, H., Cheng, F., et al. (2019). Green Synthesis of Fluorescent Carbon Dots from Gynostemma for Bioimaging and Antioxidant in Zebrafish. ACS Appl. Mat. Interfaces 11, 9832–9840. doi:10.1021/acsami.9b00074
Wu, X., Tian, F., Wang, W., Chen, J., Wu, M., and Zhao, J. X. (2013). Fabrication of Highly Fluorescent Graphene Quantum Dots Using L-Glutamic Acid for In Vitro/In Vivo Imaging and Sensing. J. Mat. Chem. C 1, 4676–4684. doi:10.1039/c3tc30820k
Xu, H. V., Zheng, X. T., Zhao, Y., and Tan, Y. N. (2018). Uncovering the Design Principle of Amino Acid-Derived Photoluminescent Biodots with Tailor-Made Structure-Properties and Applications for Cellular Bioimaging. ACS Appl. Mat. Interfaces 10, 19881–19888. doi:10.1021/acsami.8b04864
Xue, M., Zou, M., Zhao, J., Zhan, Z., and Zhao, S. (2015). Green Preparation of Fluorescent Carbon Dots from Lychee Seeds and Their Application for the Selective Detection of Methylene Blue and Imaging in Living Cells. J. Mat. Chem. B 3, 6783–6789. doi:10.1039/c5tb01073j
Yadav, K., Das, M., Hassan, N., Mishra, A., Lahiri, J., Dubey, A. K., et al. (2021). Synthesis and Characterization of Novel Protein Nanodots as Drug Delivery Carriers with an Enhanced Biological Efficacy of Melatonin in Breast Cancer Cells. RSC Adv. 11, 9076–9085. doi:10.1039/d0ra08959a
Yang, N., Xiao, W., Song, X., Wang, W., and Dong, X. (2020). Recent Advances in Tumor Microenvironment Hydrogen Peroxide-Responsive Materials for Cancer Photodynamic Therapy. Nano-Micro Lett. 12, 1–27. doi:10.1007/s40820-019-0347-0
Yao, H., Li, J., Song, Y., Zhao, H., Wei, Z., Li, X., et al. (2018). Synthesis of Ginsenoside Re-based Carbon Dots Applied for Bioimaging and Effective Inhibition of Cancer Cells. Int. J. Nanomedicine 13, 6249–6264. doi:10.2147/IJN.S176176
Yao, H., Su, L., Zeng, M., Cao, L., Zhao, W., Chen, C., et al. (2016). Construction of Magnetic-Carbon-Quantum-Dots-Probe-Labeled Apoferritin Nanocages for Bioimaging and Targeted Therapy. Int. J. Nanomedicine 11, 4423–4438. doi:10.2147/IJN.S108039
Yoon, I.-S., Park, D.-H., Kim, J.-E., Yoo, J.-C., Bae, M.-S., Oh, D.-S., et al. (2017). Identification of the Biologically Active Constituents of Camellia Japonica Leaf and Anti-hyperuricemic Effect In Vitro and In Vivo. Int. J. Mol. Med. 39, 1613–1620. doi:10.3892/ijmm.2017.2973
Yoshinaga, T., Iso, Y., and Isobe, T. (2018). Particulate, Structural, and Optical Properties of D-Glucose-Derived Carbon Dots Synthesized by Microwave-Assisted Hydrothermal Treatment. ECS J. Solid State Sci. Technol. 7, R3034–R3039. doi:10.1149/2.0091801jss
Yunus, U., Zulfiqar, M. A., Ajmal, M., Bhatti, M. H., Chaudhry, G. E., Muhammad, T. S. T., et al. (2020). Targeted Drug Delivery Systems: Synthesis and In Vitro Bioactivity and Apoptosis Studies of Gemcitabine-Carbon Dot Conjugates. Biomed. Mater 15, 065004. doi:10.1088/1748-605X/ab95e1
Zeng, Q., Shao, D., He, X., Ren, Z., Ji, W., Shan, C., et al. (2016). Carbon Dots as a Trackable Drug Delivery Carrier for Localized Cancer Therapy In Vivo. J. Mat. Chem. B 4, 5119–5126. doi:10.1039/c6tb01259k
Zeng, X., Zhang, L., Yang, J., Guo, Y., Huang, Y., Yuan, H., et al. (2017). A Novel Carbon Dots Derived from Reduced L-Glutathione as Fluorescent Probe for the Detection of the L-/d-Arginine. New J. Chem. 41, 15216–15228. doi:10.1039/c7nj03320f
Zhang, M., Wang, W., Zhou, N., Yuan, P., Su, Y., Shao, M., et al. (2017). Near-infrared Light Triggered Photo-Therapy, in Combination with Chemotherapy Using Magnetofluorescent Carbon Quantum Dots for Effective Cancer Treating. Carbon 118, 752–764. doi:10.1016/j.carbon.2017.03.085
Zhang, R., and Chen, W. (2014). Nitrogen-doped Carbon Quantum Dots: Facile Synthesis and Application as a "Turn-Off" Fluorescent Probe for Detection of Hg2+ Ions. Biosens. Bioelectron. 55, 83–90. doi:10.1016/j.bios.2013.11.074
Zhao, Q.-L., Zhang, Z.-L., Huang, B.-H., Peng, J., Zhang, M., and Pang, D.-W. (2008). Facile Preparation of Low Cytotoxicity Fluorescent Carbon Nanocrystals by Electrooxidation of Graphite. Chem. Commun. 41, 5116–5118. doi:10.1039/b812420e
Zhao, S., Huang, L., Xie, Y., Wang, B., Wang, F., and Lan, M. (2021). Green Synthesis of Multifunctional Carbon Dots for Anti-cancer and Anti-fungal Applications. Chin. J. Chem. Eng. 37, 97–104. doi:10.1016/j.cjche.2021.03.008
Zheng, M., Ruan, S., Liu, S., Sun, T., Qu, D., Zhao, H., et al. (2015). Self-Targeting Fluorescent Carbon Dots for Diagnosis of Brain Cancer Cells. ACS Nano 9, 11455–11461. doi:10.1021/acsnano.5b05575
Zheng, X. T., Lai, Y. C., and Tan, Y. N. (2019). Nucleotide-derived Theranostic Nanodots with Intrinsic Fluorescence and Singlet Oxygen Generation for Bioimaging and Photodynamic Therapy. Nanoscale Adv. 1, 2250–2257. doi:10.1039/c9na00058e
Zhou, J., Sheng, Z., Han, H., Zou, M., and Li, C. (2012). Facile Synthesis of Fluorescent Carbon Dots Using Watermelon Peel as a Carbon Source. Mater. Lett. 66, 222–224. doi:10.1016/j.matlet.2011.08.081
Zhu, B., Sun, S., Wang, Y., Deng, S., Qian, G., Wang, M., et al. (2013). Preparation of Carbon Nanodots from Single Chain Polymeric Nanoparticles and Theoretical Investigation of the Photoluminescence Mechanism. J. Mat. Chem. C 1, 580–586. doi:10.1039/c2tc00140c
Zhu, H., Wang, X., Li, Y., Wang, Z., Yang, F., and Yang, X. (2009). Microwave Synthesis of Fluorescent Carbon Nanoparticles with Electrochemiluminescence Properties. Chem. Commun. 34, 5118–5120. doi:10.1039/b907612c
Zhu, L., Yin, Y., Wang, C.-F., and Chen, S. (2013). Plant Leaf-Derived Fluorescent Carbon Dots for Sensing, Patterning and Coding. J. Mat. Chem. C 1, 4925–4932. doi:10.1039/c3tc30701h
Keywords: cancer, carbon quantum dots, bioimaging, drug delivery, photoinduced absorption
Citation: Naik K, Chaudhary S, Ye L and Parmar AS (2022) A Strategic Review on Carbon Quantum Dots for Cancer-Diagnostics and Treatment. Front. Bioeng. Biotechnol. 10:882100. doi: 10.3389/fbioe.2022.882100
Received: 23 February 2022; Accepted: 12 April 2022;
Published: 18 May 2022.
Edited by:
Gopinath Packirisamy, Indian Institute of Technology Roorkee, IndiaReviewed by:
Sudip Mukherjee, Rice University, United StatesCopyright © 2022 Naik, Chaudhary, Ye and Parmar. This is an open-access article distributed under the terms of the Creative Commons Attribution License (CC BY). The use, distribution or reproduction in other forums is permitted, provided the original author(s) and the copyright owner(s) are credited and that the original publication in this journal is cited, in accordance with accepted academic practice. No use, distribution or reproduction is permitted which does not comply with these terms.
*Correspondence: Shilpi Chaudhary, c2hpbHBpY2hhdWRoYXJ5QHBlYy5lZHUuaW4=; Avanish Singh Parmar, YXNwYXJtYXIucGh5QGl0Ymh1LmFjLmlu
Disclaimer: All claims expressed in this article are solely those of the authors and do not necessarily represent those of their affiliated organizations, or those of the publisher, the editors and the reviewers. Any product that may be evaluated in this article or claim that may be made by its manufacturer is not guaranteed or endorsed by the publisher.
Research integrity at Frontiers
Learn more about the work of our research integrity team to safeguard the quality of each article we publish.