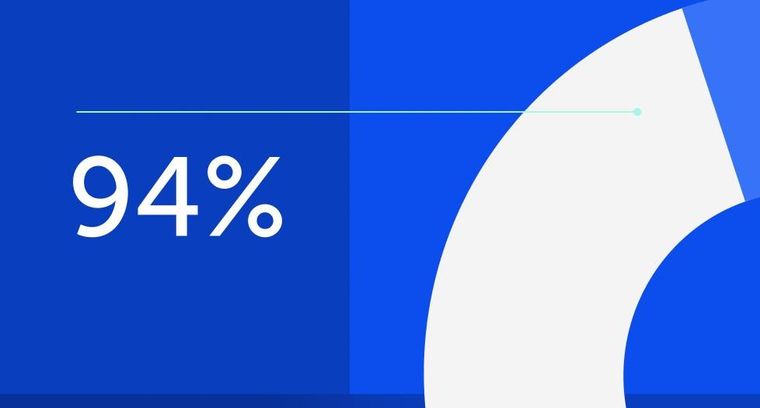
94% of researchers rate our articles as excellent or good
Learn more about the work of our research integrity team to safeguard the quality of each article we publish.
Find out more
REVIEW article
Front. Bioeng. Biotechnol., 28 March 2022
Sec. Nanobiotechnology
Volume 10 - 2022 | https://doi.org/10.3389/fbioe.2022.873369
This article is part of the Research TopicOptical Technologies for Disease Diagnosis and Therapy in Deep TissuesView all 8 articles
Malignant tumors pose a serious threat to human health and have high fatality rates. Conventional clinical anti-tumor treatment is mainly based on traditional surgery, chemotherapy, radiotherapy, and interventional therapy, and even though these treatment methods are constantly updated, a satisfactory efficacy is yet to be obtained. Therefore, research on novel cancer treatments is being actively pursued. We review the classification of gene therapies of malignant tumors and their advantages, as well as the development of gene editing techniques. We further reveal the nano-drug delivery carrier effect in improving the efficiency of gene editing. Finally, we summarize the progress in recent years of gene editing techniques based on nano-drug delivery carriers in the treatment of various malignant tumors, and analyze the prospects of the technique and its restricting factors.
Malignant tumors have specific biological characteristics, including abnormal cell differentiation and proliferation, uncontrolled growth, invasion, and metastasis. Due to their different genotypic changes such as EGFR mutation and ALK gene mutation, different tissues and organs involved, and various stages, the tumors’ responses to various treatments differ accordingly. Traditional treatments include surgery, chemotherapy, radiotherapy, and interventional therapy. Almost all traditional treatment methods face difficulty to completely eradicate the tumor, as their efficacy reaches a plateau that is difficult to break through. Furthermore, the toxicity and side effects caused by traditional radiotherapy and chemotherapy—such as digestive tract reaction, blood system changes, and bone marrow suppression—also severely restrict their wide clinical application. Hence, the search for novel cancer treatments continues.
Gene therapy refers to the introduction of exogenous normal genes to correct or compensate diseases caused by gene defects and abnormal genes, to achieve the purpose of treatment. Gene therapy for malignant tumors includes suicide gene therapy, corrective gene therapy, and toxin/apoptosis-induced gene therapy (Karjoo et al., 2016). The thymine kinase (TK) gene commonly used today is based on this principle. Corrective gene therapy is a method applying therapeutic agents, such as siRNA or, miRNA, or gene-editing tools, into tumor cells to change their gene expression and inhibit their proliferation (Senzer et al., 2013; Tabernero et al., 2013; Chen et al., 2015; Liu et al., 2015). Toxin/apoptosis-induced gene therapy is a more direct approach to inducing tumor cell death through the delivery of genes producing toxic substances, such as TNF-α.
Study has shown that the malignant tumor is a fatal disease that involves multiple genes and changes the epigenetics of the entire genome (Garraway and Lander, 2013). These gene mutations usually promote the occurrence and development of tumors (Sánchez-Rivera and Jacks, 2015). In traditional lesion resection, chemotherapy, and radiation therapy, the treatment effects are limited, and side effects to the patients’ body are significant. Gene therapy, as a novel treatment, has the advantages of high specificity and targeting, leading to less side effects. It is capable of entirely eliminating the tumor, and has therefore attracted the attention of scientists and clinicians. In the past 2 decades, with the help of high-throughput sequencing technology, numerous genes related to the occurrence and development of malignant tumors have been identified (Pon and Marra, 2015). Based on these advances, gene therapy has brought great hope for the treatment of malignant tumors by adjusting gene expression and correcting mutations. More than 2,000 clinical trials have been conducted so far, two-thirds of which were for the treatment of malignant tumors (Bertrand et al., 2014), and many of which yielded encouraging results.
Gene editing is a technique or process that enables precise modification of a specific target gene in an organism’s genome. In the early stage, genetic engineering technology was relatively crude, and only exogenous or endogenous genetic material could be randomly inserted into the host genome. With technological progress, gene editing became more sophisticated, allowing the desired gene to be edited at a specific point. The technology relies on the engineered nuclease, also known as “god’s knife,” at a specific location in the genome to produce a site-specific double-stranded break (DSB), induction of organisms through the homologous end connection, or homologous recombination to repair the DSB, the error-prone repair process, leading to a targeted mutation. This targeted mutation is referred to as gene editing, and has shown great potential in gene research, gene therapy, and genetic improvement owing to its high efficiency in site-directed genome editing.
Homologous recombination was the first technique used to edit the cell genome. It is the exchange (recombination) of genetic information between two similar (homologous) strands of DNA. It involves producing and isolating fragments of DNA with sequences similar to those of the parts of the genome to be edited, injecting these fragments into monocytes, or making them be absorbed by the cell with special chemicals. These fragments, once inside the cell, can be recombined with the cell’s DNA to replace the targeted parts of the genome. The disadvantage of this method is its high error rate and low efficiency.
To overcome this problem and create site-specific double strand breaks, four different types of nucleases have been bioengineered. They are giant nucleases (meganuclease), zinc finger nucleases (ZFNs), transcriptional activating-like effecting-factor nucleases (TALEN), and clustered regularly spaced short palindromic repeats associated systems (CRISPR/Cas). CRISPR-Cas is a prokaryotic immune system that endows prokaryotes with resistance to foreign genetic material, such as those present in plasmids and phages. It is hence an acquired immune system. According to different Cas proteins, the CRISPR/Cas system can be divided into type i, ii, and iii. Cas9 belongs to the type ii CRISPR system and is the currently most widely used gene-editing tool. The Cas9 nuclease consists of two conserved nuclease domains, HNH and RuvC. Under the guidance of crRNA and tracrRNA, specific cleavage of DNA double strands can be performed. The cleavage sites are usually located 3 nt upstream of the protospacer-adjacent motif (PAM) (Sapranauskas et al., 2011). Researchers fused crRNA and tracrRNA to create chimeric single guide RNA (sgRNA) (Jinek et al., 2012). Under the guidance of sgRNA, Cas9 can be directed to a target near the PAM sequence to form DSB at a specific site. Host cells respond to double strand breaks through two different mechanisms, non-homologous end joining (NHEJ) and homology-directed repair (HDR), leading to insertion/deletion and frameshift mutations in the target DNA. When donor DNA is provided as a homologous recombination template, cells repair in the manner of HDR, which can achieve precise insertion, deletion, or replacement of bases at specific sites (Gasiunas et al., 2012). Emmanuelle Charpentier and Jennifer Doudna, who developed the CRISPR/Cas9 gene-editing technology, won the 2020 Nobel Prize in Chemistry.
Currently, gene-editing technology is widely employed in biological and medical research. Because malignant tumors are caused by genomic changes of tumor cells, gene-editing technology can be used in the research field of malignant tumors to explore the potential mechanism of their occurrence and development. In recent years, gene-editing technology based on the CRISPR/Cas9 system has also been applied in clinical trials of a variety of malignant tumors, showing significant potential (Table 1). The CRISPR/Cas9 system can be introduced in three typical forms: plasmid DNA (pDNA), mRNA, and ribonucleoprotein (RNP, a complex of cas9 protein with sgRNA) (Yang et al., 2020; Duan et al., 2021). The pDNA-based CRISPR/Cas9 system generally performed by integrating the both cas9 protein and sgRNA encoding plasmids into a single vector, to avoid multiple transfections. However, the gene fragment size encoding the CRISPR/Cas9 system and the pDNA are often too large (∼4.3 and ∼10 kbp), resulting in a low transfection efficiency. Cas9 mRNA, which can be prepared by in vitro transcription, is another possibility for delivered cargo. The Cas9 mRNA directly translated into protein in the cytoplasm to exert their genome editing function after being transferred into the cells. However, the low stability and limited expression time of mRNA are the main limitation for its application. The RNP-based CRISPR/Cas9 system is considered as the most straightforward strategy, which can quickly start the genome editing without the process of transcription and/or translation following being transferred into cells. However, the activity and the intracellular delivery efficiency of the purified Cas9 protein with large molecular weight become the main challenges. (Zhang S. et al., 2021; Duan et al., 2021). Many nano-drug delivery platforms have been developed for the CRISPR/Cas9 system (Table 2).
Similar to drugs, gene-editing tools must circulate to eventually reach their target cells. The existence of various compounds and enzymes in human blood circulation will remove the foreign substances, which is the first obstacle for gene-editing tools to perform specific functions. The blood–brain barrier, blood–thorax barrier, and other barriers composed of dense capillary endothelial cells and a basal membrane can prevent substances from passing through. These substances must furthermore pass through the cell membrane barrier before entering the nucleus (Xu et al., 2020). Moreover, different tissues have different pH values. Both of these factors have effects and even pose difficulties in the transmission of gene-editing tools. Methods of transmission include physical methods and application carriers (Yin et al., 2017). Common physical transport methods include electroporation, microinjection, osmotic cell proliferation, and iTOP induced channel ions, mechanical cell deformation, and hydraulic jet (Liu et al., 2017). Many of these methods are accompanied by collateral damage, such as cell membrane destruction, and are therefore not suitable for in vivo application (Yan et al., 2021). Thus, an ideal vehicle is needed.
The ideal vector for gene delivery must meet the following criteria: 1) the vector must be able to express transgenes for a duration of time, and the expression must be precisely regulated; 2) the carrier must be easy to produce at higher titers to allow small volume transfer and must be suitable for commercial production and processing; 3) it must have specificity of target cells; 4) immunity is indolent, thus allowing repeated administration; 5) the vector must have no limit on the size of the genetic material it can transfer; 6) the vector must allow site-specific integration into the chromosomes of target cells or exist in the nucleus as epistasis; 7) vectors must be capable of transfecting both mitotic and non-mitotic cells (Somia and Verma, 2000; Mehierhumbert and Guy, 2005; Al-Hendy and Salama, 2006). Commonly used vectors include viral and non-viral vectors. Viral vectors include lentiviruses, and adeno-associated viruses, which are sometimes unstable in nature and may lead to immunogenicity and insertion mutations, or even carcinogenesis (Dai et al., 2020). Nanoparticles (NPs) are ultra-small particles with a diameter below 1000 nm, composed of a variety of materials such as lipids, polymers or metals. The properties of NPs, such as their material composition, particle size, electrical potential, and surface modification can be carefully designed for the delivery of drugs, nucleic acids, and other substances.
NPs can combine nucleic acid therapy based on chemical binding or electrostatic interaction to overcome the treatment challenges of malignant tumors and other diseases (Milling et al., 2017; Riley et al., 2019). First, NPs provide protection against degradation by circulating enzymes and prolongs the circulation half-life (Thomas et al., 2020; Zhang et al., 2022). Second, NPs can reduce the toxicity of inclusion by promoting the accumulation of specific sites and reducing untargeted effects. They can be designed to degrade and release their inclusion in the acidic microenvironment of tumors (Whitehead et al., 2014). Finally, NPs can be modified by targeting ligands and other molecules to promote cellular and nuclear uptake and biological distribution to targeted tissues that overexpress targeted proteins (Large et al., 2019). Thus far, a variety of nanomedicine vectors, led by lipid nanoparticles, achieved the growth inhibition of a variety of tumor cells in vitro and in vivo experiments (Wu et al., 2017; Wu et al., 2018; Mao et al., 2019; Cong et al., 2020; Sun et al., 2020). These vectors can also effectively transfer gene editing tools and carry out gene editing efficiently (Figure 1).
FIGURE 1. Schematic diagram of nanoparticle delivery gene editing tool into tumor cells in vivo. (A) Nanoparticles prevent gene editing tools from degradation by enzymes in circulation. (B) Nanoparticles cross barriers such as the blood-brain barrier with gene editing tools. (C) Nanoparticles accumulate around the tumor cells. (D) Nanoparticles enter the tumor cells and release gene editing tools.
Melanoma is the most deadly skin cancer, and the 5-years survival rate of patients with stage IV melanoma is below 15% (Fleming et al., 2015). The incidence of invasive melanoma is still growing faster than any other cancer (Prado et al., 2019), accounting for about 70% of skin-cancer-related deaths (Leonardi et al., 2018). The main cause of death from melanoma is tumor metastasis (Fidler, 2015). In recent years, studies found that the incidence, prognosis, and treatment of melanoma are closely related to CKIT, NRAS, BRAF, and other gene mutations (Nassar and Tan, 2020; Pham et al., 2020). Patients with gene mutation have a poor prognosis, are prone to relapse, metastasis, and other malignant events (Rastrelli et al., 2014; Yde et al., 2018).
Because melanoma is a malignant tumor of the skin, the in-situ tumor model can be established through a relatively simple subcutaneous seed tumor. Hence, numerous experiments exploited melanoma as the research object. Deng et al. used poly (β-amino ester) copolymer nanoparticles to carry SpCas9/sgRNA plasmids targeting CDK5 to achieve NHEJ-mediated destruction (Deng et al., 2020). Compared with the PEI 25K and HP transfection reagent, these showed superior transfection efficiency in B16F10 cells (Figure 2A). By effectively knocking out Cdk5 target gene, PD-L1 expression was down-regulated in vivo, the CTL mediated immune response was restored, and the immunosuppressive tumor microenvironment was reversed (Figure 2B). Tumor growth was inhibited in B16F10 tumor-bearing mice (Figure 2C). Compared with the anti-PD-L1 antibody, it is capable of maintaining a relatively long-term therapeutic effect. Zhang et al. developed a double-locked nanoparticle called DLNP that can stably exist in blood circulation or normal tissues and release the CRISPR/Cas13a system in the tumor microenvironment with low pH and high H2O2 concentration. It promotes cellular internalization of the CRISPR/Cas13a system and activation of gene editing after entry into the tumor tissue (Zhang et al., 2019). Improved gene editing efficiency at tumor sites and reduced side effects caused by unintended activation of CRISPR/Cas13a in normal tissue were observed. By systemic administration, the tumor growth of B16F10 tumor-bearing mice was significantly inhibited, and the survival rate was improved.
FIGURE 2. Experiments with aPBAE/Cas9-CDK5 nanocomposites. (A) Quantitative analysis of transfection efficiency in B16F10 cells. (B) Nanocomposites-mediated cleavage of Cdk5 gene in B16F10 cells detected by T7EI cleavage assay. (C) Nanocomposites-mediated PD-L1 attenuation suppresses B16F10 tumor growth and triggers T cell-mediated antitumor immune response in the murine melanoma model. Reproduced with permission from Deng et al. (2020).
Plk1 (Polo-like kinase 1) belongs to the Polo-like kinase family, which is a serine/threonine kinase abundant in eukaryotic cells. Overexpression of Plk1 has been found in numerous tumor tissues and model tumor cells (such as A375 cells), and inhibition of Plk1 expression can lead to apoptosis of tumor cells, providing a good strategy for tumor therapy (Lee et al., 2015; Gutteridge et al., 2016; Iliaki et al., 2021). Wang et al. prepared a Cas9 protein/sgPlk1 plasmid carrier with gold nanoclusters as the core and achieved 26.2% Plk1 genome modification in vitro, which is more than 10 times more effective than the traditional plasmid transfection method (Wang et al., 2017). The in vivo antitumor effect of granulosa was evaluated on the A375 nude mouse subcutaneous tumor model. Compared with other control groups, the nanoparticles had lower toxicity and the most significant inhibitory effect on tumor growth.
Photothermal therapy is a treatment method using materials with high photothermal conversion efficiency, implanting them inside the body, gathering them near the tumor tissue with targeted recognition technology, and converting the light energy into heat energy under the irradiation trigger of an external light source (usually near infrared light) to kill cancer cells (Melamed et al., 2015). The advantage of this therapy over traditional cancer therapies is that effective treatments can be performed with precision and few side effects. Numerous studies have addressed this method’s tumor inhibition through photothermal therapy and the interaction of nanoparticles (Hirsch et al., 2003; Dickerson et al., 2008; Chen et al., 2010; Day et al., 2011; Day et al., 2012). Kim et al. constructed a metal-lipid hybrid nanoparticle (MLN) to deliver plasmid DNA of sgRNA and Cas9 proteins encoding TGF-β (Kim et al., 2021). In B16F10 tumor-bearing mice, ifn -γ, cytotoxic T-cells, and mature dendritic cells were increased in the tumor microenvironment by intratumoral injection of the nano-complex plus Near Infra-Red (NIR) irradiation (Figure 3B), which could ablate the primary tumor (Figure 3A) and prevent distant growth of secondary B16F10 cells and lung metastasis (Figure 3C).
FIGURE 3. Related experiments of MLN. (A) Tumor size changes in each group after unilateral tumor inoculation and treatment. (B) Tumor immune microenvironment in each group after unilateral tumor inoculation and treatment. (C) Antitumor efficacy against B16F10, MC38, and EL4 distant tumors and B16F10 lung metastasis. Reproduced with permission from Kim et al. (2021).
Breast cancer is the leading cause of cancer in women, which mostly occurs over 40 years old (Radecka and Litwiniuk, 2016). The incidence of breast cancer is related to genetic and environmental factors (Peairs et al., 2017; Barzaman et al., 2020). According to histological characteristics, breast cancer can be divided into human epidermal growth factor receptor two overexpression (HER2+), hormone receptor positive and three negative breast cancer (TNBC) (Nagini, 2017). Among breast cancers, TNBC is a special breast cancer subtype characterized by deletion of the estrogen receptor, progesterone receptor, and human epidermal growth factor receptor 2 (Foulkes et al., 2010). TNBC lacks effective targeted therapy (Cardoso et al., 2018), is highly invasive and metastatic, and has the highest mortality rate among all breast cancer subtypes (Dent et al., 2007; Foulkes et al., 2010). In recent years, nanomaterials combined with gene therapy has gradually become the focus of TNBC treatment, Chen et al. designed an intelligent nanocomposite that can release CCL25 protein and CD47 siRNA in the tumor tissue of TNBC mouse model. They proved that the transmission of ccl25 in tumor can promote the tumor infiltration of CCR9 + CD8 + T cells by blocking CD47/Sirpα and PD-1/PD-L1 signaling pathways, so as to significantly inhibit tumor growth (Chen et al., 2020). Guo et al. reported a nanolipogel with tumor-targeting, deformable, and non-cationic characteristics, called tNLG, which was used to edit the CRISPR genome in TNBC tumors and successfully inhibited the expression of breast cancer gene Lcn2, mediating a gene editing efficiency of more than 81% (Guo et al., 2019). Deletion of the Lcn2 gene significantly inhibited the migration and mesenchymal phenotype of TNBC cells, thus attenuating the aggressive spread of TNBC. In the TNBC in situ tumor model, administration of MDA-MB-231 tumor-bearing mice through the tail vein inhibited 77% of TNBC tumor growth with minimal systemic toxicity. Deng et al. (Fidler, 2015) reported that poly (β -amino esterification) copolymer nanoparticles coated with CDK5-targeting SpCas9/sgRNA plasmids showed superior transfection efficiency in 4T1 cells. (Deng et al., 2020). Compared with the anti-PD-L1 antibody group, pNDA group and PBS group, the tumor volume and weight were significantly reduced in the TNBC lung metastasis model, and the occurrence of lung metastasis was decreased in the nanoparticle group (Figure 4A). Immunohistochemical results showed that the infiltration of CD8+ T-cells was high, and the expression of Cdk5 was decreased. TUNEL staining also showed enhanced apoptosis of tumor cells after nanoparticle treatment (Figure 4B).
FIGURE 4. Application of aPBAE/Cas9-CDK5 nanoparticles in 4T1 tumor model. (A) Nanoparticles-mediated attenuation of PD-L1 inhibits 4T1 tumor growth and lung metastasis. (B) Immunofluorescence, immunohistochemistry, and TUNEL staining of tumor sections. Reproduced with permission from Deng et al. (2020).
Lung cancer is one of the most threatening malignant tumors threatening human health and survival (Mao et al., 2016; de Sousa and Carvalho, 2018). In the recent 50 years, the incidence and mortality of lung cancer have been reported in numerous countries, and it has been found to rank in the top two cancer types for both men and women (Romaszko and Doboszyńska, 2018). Surgical treatment is suitable for early stage of lung cancer (Hoy et al., 2019). A study on lung cancer patients showed that tumor suppressor gene mutations and the overexpression of oncogenes may be related to the occurrence of lung cancer (Liu et al., 2004). In recent years, gene editing techniques have been used for in vitro experiment of cancer (Jubair and McMillan, 2017). He et al. constructed a natural nanopolymer by combining hyaluronic acid (AHA) functionalization with aptamer (AS1411) and hyaluronic acid (PHA) functionalization with peptide (TAT-NLS) to deliver CRISPR-Cas9 plasmids specifically to tumor cells and achieve effective gene editing. The CTNNB1 gene encoding β -catenin was knocked out, and PD-L1 expression was down-regulated in tumor cells, successfully reversing tumor immunosuppression and immune escape of H1299 (He et al., 2020). Gene-edited tumor cells effectively enhance T cell immunity, including proliferation, cytokine release, and cytolysis activity.
Photothermal therapy (PTT) has attracted increasing attention in the field of tumor therapy owing to its spatio-temporal controlled mode and non-invasive nature (Song et al., 2018). Pan et al. designed a nanocomposite. CRISPR-Cas9 was covalent anchoring of photodegradable 4- (hydroxymethyl) -3-nitrobenzoic acid (ONA) molecules on lanthanum-doped conversion nanoparticles (UCNP), and then coated with polyethylene imine (PEI) to assist the escape of endosomes (Pan et al., 2019). The nano-complex can be effectively internalized by cells via the endocytosis pathway, followed by endosomal escape and cytoplasmic release of ucNPS-Cas9 loaded in the cytoplasm. When exposed to NIR, UCNP emit local ultraviolet light and trigger the rupture of the junction. Consequently, Cas9 can be released from the surface of the UCNP and thus enter the nucleus for gene editing. Tumor growth was successfully inhibited in xenografted nude mouse models of A549 cells by targeting oncogene markers (PLK-1 gene). Although PTT can increase the local temperature of the body to over 50°C and kill tumor cells, it can also cause damage to normal tissues and carry the risk of recurrence or metastasis (Gao et al., 2019). Li et al. applied a low oxygen responsive gold nanorods that can carry sgRNA targeting HSP90α (Li et al., 2021). Due to the hypoxic state of the tumor microenvironment, the azo group of the nano-complex is selectively reduced by overexpression reductase, resulting in the release of Cas9 and subsequent HSP90α gene knockout, which reduces the thermal resistance of cancer cells. Under mild PTT conditions, the nano-complex can achieve efficient tumor ablation in vivo and in vitro. This also reduces thermal damage to normal surrounding tissues.
Hepatocellular carcinoma (HCC) is the third most common cancer worldwide. Surgical resection and liver transplantation are considered to be the only treatments, but are often limited by low liver function and a shortage of liver donors (Waghray et al., 2015; Valverde-López et al., 2018). The epidermal growth factor receptor (EGFR) is a transmembrane receptor that plays an important role in various tumors, especially liver cancer, leading to the growth and proliferation of tumor cells. Sorafenib, a chemotherapy drug, assumes an anti-tumor role by specifically inhibiting multiple molecular targets, such as EGFR and VEGFR2 (Redd Bowman et al., 2020). Zhang et al. designed a polyaminoamine aptamer coated hollow mesoporous silica nanoparticle, which has good stability in blood circulation and a large drug load, and can jointly deliver the Sora and CRISPR/Cas9 system (Zhang et al., 2020). Apt-modified NPs surfaces can effectively enhance the uptake of NPs by HCC cells, thus reducing the side effects of Sora. Moreover, the CRISPR/Cas9 system co-delivered with Sora synergistically inhibits the expression of EGFR and downstream PIK3 Akt pathways, with no detectable off-target effects and a powerful anti-angiogenesis effect. The synergistic efficacy of nanocomposites was studied both in vitro and in vivo. Both model systems demonstrated that NP has strong cytotoxicity to HCC cells by specifically binding EpCAM receptors on tumor cell membranes. In vivo fluorescence imaging shows the accumulation of NPs in the tumor area, while HE staining and blood biochemical analysis showed no significant damage to major organs. Furthermore, effective gene editing of EGFR in vivo was confirmed by sequencing, and inhibition of EGFR expression in tumor tissues was detected by IHC.
The PLK1 gene has also been targeted in related studies of liver cancer. Li et al. constructed a proprietary ionizable lipid nanoparticle named iLP181 (Li et al., 2022). Four plasmids containing PLK1-targeting Cas9 protein and sgRNA were designed. PsgPLK1 with the best activity was selected and loaded with iLP181. Studies have shown that iLP181/psgPLK1 is effectively internalized by hepatocellular carcinoma cells by binding ApoE, resulting in long-term in vivo and in vitro gene editing and significantly inhibiting tumor growth in HepG2-LuC-bearing mice. Compared with Lipo2000 on the market, iLP181 shows strong endogenous escape when delivering nucleic acid.
Glioblastoma (GBM) is the most common and fatal primary brain tumor in adults. The mean survival was only 12–14 months, even after a combination of treatments including surgery, chemotherapy and/or radiation (Davis, 2016). In primary glioblastoma, the molecular changes are mainly the expansion and overexpression of EGFR (Eskilsson et al., 2018), while in secondary glioblastoma, the molecular changes are mainly p53 mutation (Wirsching et al., 2016). BBB block more than 98% of substances from entering the central nervous system and also limit the passage of drugs, including therapeutic drugs. The application of nanocarriers increases the possibility of drugs, nucleic acids, etc. crossing the BBB and further treatment. Yang et al. designed a lipid polymer hybrid nanoparticle (LphNS-CRGD) for efficient and targeted delivery of CRISPR/Cas9 plasmids targeting temozolomide (TMZ) resistance gene O6-methylguanine DNA methyltransferase (MGMT) (Yang et al., 2021). LPHNS-cRGD can target GBM cells and mediate the transfection of pCas9/MGMT to down-regulate the expression of MGMT, resulting in increased sensitivity of GBM cells to TMZ. In vivo, local FUS irradiation can safely increase BBB permeability and allow nanoparticles to accumulate in tumors of in situ tumor-bearing mice, enhancing the therapeutic effect of TMZ on glioblastoma, inhibiting tumor growth, and prolongating survival of tumor-bearing mice with high biosafety. Co-delivery of Cas9 mRNA and sgRNA using delivery system is a promising strategy to efficiently edit the genome in cells. Kataoka et al. developed a PEGylated polyplex micelle (PM) to co-encapsulate the Cas9 mRNA and sgRNA for genome editing, which could prevent the sgRNA release upon dilution and enhance the tolerability of cas9 mRNA and sgRNA against enzymatic degradation (Uchida and Kataoka, 2019). They further achieved effective genome editing in the mouse brain parenchyma in vivo using this PM with co-encapsulated Cas9 mRNA and sgRNA (Abbasi et al., 2021). Rosenblum et al. reported a liposome nanoparticle equipped with PLK1-targeted Cas9 mRNA and sgRNA gene-editing tools, and the gene-editing rate of this system was up to 98% in vitro in a variety of cancer cell types (Figure 5A) (Rosenblum et al., 2020). single-dose administration of nanoparticles on tumors in a mouse GBM model resulted in approximately 70% PLK1 gene editing. The induction of apoptosis in vivo was assessed by activated Caspase three staining, and increased median survival by approximately 50% and overall survival by 30% in GBM tumor-bearing mice (Figure 5B). Liu et al. synthesized a nanoparticle carrying sgRNAs (Liu et al., 2019). PLys and Cas9/sgRNA complexes make up the core, as well as mPEG, and the core and shell are connected by 2,5-dihydro-2,5-dioxofuran-3-acetic acid (CA). Due to the degradation of CA in acidic environment, the complex exhibits an acid response. In the acidic tumor microenvironment, the mPEG shell can be peeled off, and the inclusion can be efficiently accumulated in the tumor, resulting in gene editing. Tumor growth can be inhibited in heterogeneous tumor models with two cell subpopulations by carrying sgRNAs targeting STAT3 and RUNX1.
FIGURE 5. Transfection efficiency of PLK1-targeted CRISPR LNPs (cLNPs) and its application in mouse GBM model. (A) Therapeutic genome editing in HEK 293 cells, 005 (murine GBM) and OV8 (human ovarian carcinoma) cells in vitro. (B) Therapeutic genome editing in 005 GBM bearing mice. Reproduced with permission from Rosenblum et al. (2020).
Every year, there are more than 500,000 new cases of cervical cancer worldwide. Infection with human papillomavirus (HPV) causes 90% of cervical cancer cases (Hu and Ma, 2018; Okunade, 2020). HPV is a spherical DNA virus that causes proliferation of the squamous epithelium, the mucous membrane of the human skin. More than 130 species of HPV have been isolated so far. Among them, HPV-16 and HPV-18 are the main drivers of malignant transformation of cervical epithelial cells. To date, treatment outcomes for cervical cancer remain unsatisfactory, with more than 30% of patients initially treated diagnosed with recurrence and metastasis within 2 years and a 5-years survival rate of less than 10% (Pimple and Mishra, 2019). Therefore, it is urgent to develop new strategies to improve the therapeutic treatments of cervical cancer. Zhen et al. developed a novel liposome nanoparticle containing CRISPR/Cas9 gene-editing tool, which can inhibit the proliferation of HPV16-positive cervical cancer SiHa cells and induce apoptosis by inactivating the hr-HPV16E/E7 oncogene (Zhen et al., 2020). Injection of cationic liposomes targeting HPV16 E6/E7 into subcutaneous tumor models in nude mice significantly inhibited tumor growth without significant toxicity.
Gold nanoclusters have good biocompatibility, chemical inertia, strong fluorescence emission, and tunable surface functionalization, which can efficiently complete the delivery task, and are widely used in tumor and other disease models (Vankayala et al., 2015). Tao et al. designed a nanocarrier composed of protamine with AuNC, a gold nanocluster with high biocompatibility, for transporting Cas9 — sgRNA plasmids for genome editing (Tao et al., 2021). The nanocomplex can effectively knock out the oncogenic E7 gene and inhibit the proliferation of HeLa cancer cells. It also can effectively achieve genome destruction in different cancer cells, which significantly broadens its further applications in cancer treatment. In addition, protamine-AuNCs have excellent fluorescence properties, indicating great potential of our nanocarriers for imaging tracking. Ju et al. also proved that AuNCs could be assembled with purified Streptococcus pyogenes Cas9 (SpCas9) protein under physiological conditions (Ju et al., 2019). The complex is stable at higher pH and decomposes at lower pH. Due to the low pH microenvironment of the tumor, the assembly-decomposition process can promote the entry of SpCas9 into the tumor nucleus and perform its cleavage function. SpCas9—AuNCs combined with HPV18 E6 sgRNA—effectively knocks out the oncogenic E6 gene, triggering the expression of tumor suppressor protein p53, restoring its function, and inducing the apoptosis of cervical cancer cells. Importantly, the process had little effect on other human cells without the HPV E6 gene, demonstrating the high efficiency and specificity of gene therapy for cancer.
Globally, ovarian cancer is the seventh most common cancer in women, with a 5-year survival rate of less than 45%. About 140,000 women die of ovarian cancer every year (Webb and Jordan, 2017; Penny, 2020). Because ovarian cancer lacks specific symptoms in its early stages, and tests do not always yield positive results, most ovarian cancer is not diagnosed until later in life, and many patients have developed malignant peritoneal effusion (Roett and Evans, 2009; Aleksandra Kujawa and Lisowska, 2015). Rosenblum et al. reported a lipid nanoparticle loaded with PLK1 targeted Cas9 mRNA and sgRNA gene-editing tools (Rosenblum et al., 2020). They created a mouse model of peritoneal disseminated ovarian cancer, OV8-Mcherry, and injected nanoparticles through the abdominal cavity. The results showed that the nanoparticles could be selectively ingested into disseminated ovarian tumors (Figures 6A,C), and the gene editing rate was up to 80% in vivo (Figure 6B), and the tumor growth was inhibited (Figure 6D), and the survival rate of tumor bearing mice was increased by about 80% (Figure 6E).
FIGURE 6. Treatment of Plk1-targeted cLNPs in an OV8-Mcherry mouse model with peritoneal disseminated ovarian cancer. (A) Tumor targeting and accumulation of cy5.5-CLNPs in OV8 Tumor-bearing mice. (B) Percentage of gene-editing Events in the LOCUS as determined by NGS analysis of PLK1. (C) in vivo imaging images of OV8-bearing mice. (D) Changes in tumor volume. (E) Survival curve of tumor-bearing mice. Reproduced with permission from Rosenblum et al. (2020).
Head and neck squamous cell carcinoma (HNSCC) is a heterogeneous group of malignant tumors, which is usually caused by drinking and smoking, includes oropharyngeal squamous cell carcinoma (OPSCC), laryngeal squamous cell carcinoma, and oral squamous cell carcinoma (Johnson et al., 2020; von Witzleben et al., 2020). Surgical treatment can lead to visible and functional facial deformities: radiation and chemotherapy are less specific for individual tumors, and have systemic toxic side effects (Murphy et al., 2007). Local or regional metastasis occurs in some patients, which reduces the survival rate to less than 1 year (Schwartz et al., 2000; Forastiere et al., 2001). HuR is an RNA-binding protein encoded by ELAVL1 gene, which plays an important role in regulating the survival, metastasis and drug resistance of HNSCC. Wang et al. designed two different liposome nanocarriers, namely SLN-HPR and LIP-HPR, to deliver CRISPR/Cas9 system and epubicin (Epi), respectively (Wang et al., 2021). These nanoparticles have numerous effects: 1) promoting endosome escape and nuclear localization through the pH response and nucleo-targeted H and R polypeptide sequences; 2) maintaining blood circulation through PEG modified nanoparticles and passively targeting tumors through enhanced permeability and retention effect (EPR); 3) EGFR targeting and cell internalization can be improved by endocytosis mediated by EGFR ligand P; 4) nuclear and/or cytoplasmic release of Epi and/or CRISPR/CAS-9 as a topoisomerase inhibitor HuR knockout system inhibits tumor progression and multiple survival and metastasis pathways, and regulates drug resistance. By establishing a SAS/LUC-BEARING mouse model, HuR CRISPR/SLN-HPR pre-knocked out HuR in SAS cells and co-treated SAS/LUC mice with Epi/LIP-HPR, achieving the most significant antitumor effect.
Nano-drug delivery vectors can efficiently deliver gene-editing tools for a variety of malignancies, and inhibit tumor cell growth in vitro and in vivo experiments. Although most of results have been encouraging, the gene therapy based on nano-drug delivery vectors is currently only confirmed in animal trials, and no clinical trials have been conducted to date. Numerous problems and conditions must be solved and perfected before clinical treatment can be realized. First of all, in most of in vivo experiments, there are few in situ models, but most of them are subcutaneous models. It has little effect on melanoma, breast cancer and other visible tumors, but this is not the real growth pattern of more tumors. We still need better modeling methods and tumor observation methods, and pay attention to the humanization of animal models in order to simulate more realistic tumor growth in humans (Hu et al., 2016; De La Rochere et al., 2018; Ito et al., 2018; Tian et al., 2020). Secondly, in terms of inclusion, although CRISPR/Cas9-based gene-editing technology is stable, efficient, simple, and widely used, some problems remain, such as the off-target effect, targeted mutation, and immune response of human body to bacteria-derived Cas9 protein (Zhang H. et al., 2021). One of the most promising applications of CRISPR/Cas9 in gene therapy is CAR T-cell therapy. However, clinical studies have found that this treatment is neurotoxic in vivo and may lead to cytokine release syndrome (Zhang M. et al., 2021). Nanoparticles as carriers likewise must address some challenges. First, NPs carrying gene-editing tools must successfully edit a sufficient number of cells to achieve the desired therapeutic outcome, which can be met by linking the targeted ligand to the NPs to facilitate its binding and uptake to the targeted cells (Schmid et al., 2017). Second, we need NPs to accurately control the delivery and release time of gene-editing tools (Yin et al., 2016). This challenge can be met by developing NPs using materials with highly adjustable degradation curves to release the inclusion on demand (Kamaly et al., 2016). Finally, materials used in clinical trials and even clinical treatment must have a high level of safety (Kamali Shahri et al., 2021; Martín-Sabroso et al., 2021; Phillips and Mousa, 2022). In addition, the use of nanoparticles to deliver CRISPR/Cas9 to acute myeloid leukemia (AML) and chronic myeloid leukemia (CML) genes in animal models has been shown to be a promising strategy for the treatment of leukemia (Liu et al., 2018; Ho et al., 2021; Ren et al., 2022). However, there are few reports about non-solid tumors. Despite the difficulties, it is expected that in the near future, animal trials, clinical trials, and even clinical treatments for better tumor modeling will begin to yield exciting results.
SX wrote the manuscript. SX and TS contributed to the literaturere search. Y-GY, JS, and TS revised and approved the manuscript. All authors have read and agreed to the published version of the manuscript.
This work was supported by the grants from National Key Research and Development Program of China (2017YFA0208100), NSFC (No’s 81871478, 32171379, 81941008, and 81422026), Jilin Scientific and Technological Development Program (20190201094JC and 20200301007RQ), Interdisciplinary Innovation Project of the First Hospital of Jilin University (JDYYJCHX001), and the Fundamental Research Funds for the Central Universities, JLU.
The authors declare that the research was conducted in the absence of any commercial or financial relationships that could be construed as a potential conflict of interest.
All claims expressed in this article are solely those of the authors and do not necessarily represent those of their affiliated organizations, or those of the publisher, the editors and the reviewers. Any product that may be evaluated in this article, or claim that may be made by its manufacturer, is not guaranteed or endorsed by the publisher.
Abbasi, S., Uchida, S., Toh, K., Tockary, T. A., Dirisala, A., Hayashi, K., et al. (2021). Co-encapsulation of Cas9 mRNA and Guide RNA in Polyplex Micelles Enables Genome Editing in Mouse Brain. J. Controlled Release 332, 260–268. doi:10.1016/j.jconrel.2021.02.026
Al-Hendy, A., and Salama, S. (2006). Gene Therapy and Uterine Leiomyoma: a Review. Hum. Reprod. Update 12, 385–400. doi:10.1093/humupd/dml015
Aleksandra Kujawa, K., and Lisowska, K. M. (2015). Ovarian Cancer - from Biology to Clinic. Postepy Hig Med. Dosw 69, 1275–1290. doi:10.5604/17322693.1184451
Barzaman, K., Karami, J., Zarei, Z., Hosseinzadeh, A., Kazemi, M. H., Moradi-Kalbolandi, S., et al. (2020). Breast Cancer: Biology, Biomarkers, and Treatments. Int. Immunopharmacology 84, 106535. doi:10.1016/j.intimp.2020.106535
Bertrand, N., Wu, J., Xu, X., Kamaly, N., and Farokhzad, O. C. (2014). Cancer Nanotechnology: the Impact of Passive and Active Targeting in the Era of Modern Cancer Biology. Adv. Drug Deliv. Rev. 66, 2–25. doi:10.1016/j.addr.2013.11.009
Cardoso, F., Senkus, E., Costa, A., Papadopoulos, E., Aapro, M., André, F., et al. (2018). 4th ESO-ESMO International Consensus Guidelines for Advanced Breast Cancer (ABC 4)†. Ann. Oncol. 29, 1634–1657. doi:10.1093/annonc/mdy192
Chen, H., Cong, X., Wu, C., Wu, X., Wang, J., Mao, K., et al. (2020). Intratumoral Delivery of CCL25 Enhances Immunotherapy against Triple-Negative Breast Cancer by Recruiting CCR9+ T Cells. Sci. Adv. 6, eaax4690. doi:10.1126/sciadv.aax4690
Chen, J., Glaus, C., Laforest, R., Zhang, Q., Yang, M., Gidding, M., et al. (2010). Gold Nanocages as Photothermal Transducers for Cancer Treatment. Small 6, 811–817. doi:10.1002/smll.200902216
Chen, Y., Gao, D.-Y., and Huang, L. (2015). In Vivo delivery of miRNAs for Cancer Therapy: Challenges and Strategies. Adv. Drug Deliv. Rev. 81, 128–141. doi:10.1016/j.addr.2014.05.009
Cong, X., Tian, H., Liu, S., Mao, K., Chen, H., Xin, Y., et al. (2020). Cationic Liposome/DNA Complexes Mediate Antitumor Immunotherapy by Promoting Immunogenic Tumor Cell Death and Dendritic Cell Activation. ACS Appl. Mater. Inter. 12, 28047–28056. doi:10.1021/acsami.0c08112
Dai, X., Blancafort, P., Wang, P., Sgro, A., Thompson, E. W., and Ostrikov, K. (2020). Innovative Precision Gene‐Editing Tools in Personalized Cancer Medicine. Adv. Sci. 7, 1902552. doi:10.1002/advs.201902552
Davis, M. (2016). Glioblastoma: Overview of Disease and Treatment. Clin. J. Oncol. Nurs. 20, S2–S8. doi:10.1188/16.cjon.s1.2-8
Day, E. S., Thompson, P. A., Zhang, L., Lewinski, N. A., Ahmed, N., Drezek, R. A., et al. (2011). Nanoshell-mediated Photothermal Therapy Improves Survival in a Murine Glioma Model. J. Neurooncol. 104, 55–63. doi:10.1007/s11060-010-0470-8
Day, E. S., Zhang, L., Thompson, P. A., Zawaski, J. A., Kaffes, C. C., Gaber, M. W., et al. (2012). Vascular-targeted Photothermal Therapy of an Orthotopic Murine Glioma Model. Nanomedicine 7, 1133–1148. doi:10.2217/nnm.11.189
De La Rochere, P., Guil-Luna, S., Decaudin, D., Azar, G., Sidhu, S. S., and Piaggio, E. (2018). Humanized Mice for the Study of Immuno-Oncology. Trends Immunol. 39, 748–763. doi:10.1016/j.it.2018.07.001
de Sousa, V. M. L., and Carvalho, L. (2018). Heterogeneity in Lung Cancer. Pathobiology 85, 96–107. doi:10.1159/000487440
Deng, H., Tan, S., Gao, X., Zou, C., Xu, C., Tu, K., et al. (2020). Cdk5 Knocking Out Mediated by CRISPR-Cas9 Genome Editing for PD-L1 Attenuation and Enhanced Antitumor Immunity. Acta Pharmaceutica Sinica B 10, 358–373. doi:10.1016/j.apsb.2019.07.004
Dent, R., Trudeau, M., Pritchard, K. I., Hanna, W. M., Kahn, H. K., Sawka, C. A., et al. (2007). Triple-negative Breast Cancer: Clinical Features and Patterns of Recurrence. Clin. Cancer Res. 13, 4429–4434. doi:10.1158/1078-0432.ccr-06-3045
Dickerson, E. B., Dreaden, E. C., Huang, X., El-Sayed, I. H., Chu, H., Pushpanketh, S., et al. (2008). Gold Nanorod Assisted Near-Infrared Plasmonic Photothermal Therapy (PPTT) of Squamous Cell Carcinoma in Mice. Cancer Lett. 269, 57–66. doi:10.1016/j.canlet.2008.04.026
Duan, L., Ouyang, K., Xu, X., Xu, L., Wen, C., Zhou, X., et al. (2021). Nanoparticle Delivery of CRISPR/Cas9 for Genome Editing. Front. Genet. 12, 673286. doi:10.3389/fgene.2021.673286
Eskilsson, E., Røsland, G. V., Solecki, G., Wang, Q., Harter, P. N., Graziani, G., et al. (2018). EGFR Heterogeneity and Implications for Therapeutic Intervention in Glioblastoma. Neuro Oncol. 20, 743–752. doi:10.1093/neuonc/nox191
Fidler, I. J. (2015). The Biology of Brain Metastasis. Cancer J. 21, 284–293. doi:10.1097/ppo.0000000000000126
Fleming, N. H., Zhong, J., da Silva, I. P., Vega-Saenz de Miera, E., Brady, B., Han, S. W., et al. (2015). Serum-based miRNAs in the Prediction and Detection of Recurrence in Melanoma Patients. Cancer 121, 51–59. doi:10.1002/cncr.28981
Forastiere, A., Koch, W., Trotti, A., and Sidransky, D. (2001). Head and Neck Cancer. N. Engl. J. Med. 345, 1890–1900. doi:10.1056/nejmra001375
Foulkes, W. D., Smith, I. E., and Reis-Filho, J. S. (2010). Triple-negative Breast Cancer. N. Engl. J. Med. 363, 1938–1948. doi:10.1056/nejmra1001389
Gao, G., Jiang, Y. W., Sun, W., Guo, Y., Jia, H. R., Yu, X. W., et al. (2019). Molecular Targeting-Mediated Mild-Temperature Photothermal Therapy with a Smart Albumin-Based Nanodrug. Small 15, e1900501. doi:10.1002/smll.201900501
Garraway, L. A., and Lander, E. S. (2013). Lessons from the Cancer Genome. Cell 153, 17–37. doi:10.1016/j.cell.2013.03.002
Gasiunas, G., Barrangou, R., Horvath, P., and Siksnys, V. (2012). Cas9-crRNA Ribonucleoprotein Complex Mediates Specific DNA Cleavage for Adaptive Immunity in Bacteria. Proc. Natl. Acad. Sci. U S A. 109, E2579–E2586. doi:10.1073/pnas.1208507109
Guo, P., Yang, J., Huang, J., Auguste, D. T., and Moses, M. A. (2019). Therapeutic Genome Editing of Triple-Negative Breast Tumors Using a Noncationic and Deformable Nanolipogel. Proc. Natl. Acad. Sci. U.S.A. 116, 18295–18303. doi:10.1073/pnas.1904697116
Gutteridge, R. E. A., Ndiaye, M. A., Liu, X., and Ahmad, N. (2016). Plk1 Inhibitors in Cancer Therapy: From Laboratory to Clinics. Mol. Cancer Ther. 15, 1427–1435. doi:10.1158/1535-7163.mct-15-0897
He, X. Y., Ren, X. H., Peng, Y., Zhang, J. P., Ai, S. L., Liu, B. Y., et al. (2020). Aptamer/Peptide-Functionalized Genome-Editing System for Effective Immune Restoration through Reversal of PD-L1-Mediated Cancer Immunosuppression. Adv. Mater. 32, e2000208. doi:10.1002/adma.202000208
Hirsch, L. R., Stafford, R. J., Bankson, J. A., Sershen, S. R., Rivera, B., Price, R. E., et al. (2003). Nanoshell-mediated Near-Infrared thermal Therapy of Tumors under Magnetic Resonance Guidance. Proc. Natl. Acad. Sci. U.S.A. 100, 13549–13554. doi:10.1073/pnas.2232479100
Ho, T. C., Kim, H. S., Chen, Y., Li, Y., LaMere, M. W., Chen, C., et al. (2021). Scaffold-mediated CRISPR-Cas9 Delivery System for Acute Myeloid Leukemia Therapy. Sci. Adv. 7, eabg3217. doi:10.1126/sciadv.abg3217
Hoy, H., Lynch, T., and Beck, M. (2019). Surgical Treatment of Lung Cancer. Crit. Care Nurs. Clin. North America 31, 303–313. doi:10.1016/j.cnc.2019.05.002
Hu, Z., and Ma, D. (2018). The Precision Prevention and Therapy of HPV-Related Cervical Cancer: New Concepts and Clinical Implications. Cancer Med. 7, 5217–5236. doi:10.1002/cam4.1501
Hu, Z., Xia, J., Fan, W., Wargo, J., and Yang, Y.-G. (2016). Human Melanoma Immunotherapy Using Tumor Antigen-specific T Cells Generated in Humanized Mice. Oncotarget 7, 6448–6459. doi:10.18632/oncotarget.7044
Iliaki, S., Beyaert, R., and Afonina, I. S. (2021). Polo-like Kinase 1 (PLK1) Signaling in Cancer and beyond. Biochem. Pharmacol. 193, 114747. doi:10.1016/j.bcp.2021.114747
Ito, R., Takahashi, T., and Ito, M. (2018). Humanized Mouse Models: Application to Human Diseases. J. Cel Physiol 233, 3723–3728. doi:10.1002/jcp.26045
Jinek, M., Chylinski, K., Fonfara, I., Hauer, M., Doudna, J. A., and Charpentier, E. (2012). A Programmable Dual-RNA-Guided DNA Endonuclease in Adaptive Bacterial Immunity. Science 337, 816–821. doi:10.1126/science.1225829
Johnson, D. E., Burtness, B., Leemans, C. R., Lui, V. W. Y., Bauman, J. E., and Grandis, J. R. (2020). Head and Neck Squamous Cell Carcinoma. Nat. Rev. Dis. Primers 6, 92. doi:10.1038/s41572-020-00224-3
Ju, E., Li, T., Ramos da Silva, S., and Gao, S.-J. (2019). Gold Nanocluster-Mediated Efficient Delivery of Cas9 Protein through pH-Induced Assembly-Disassembly for Inactivation of Virus Oncogenes. ACS Appl. Mater. Inter. 11, 34717–34724. doi:10.1021/acsami.9b12335
Jubair, L., and McMillan, N. A. J. (2017). The Therapeutic Potential of CRISPR/Cas9 Systems in Oncogene-Addicted Cancer Types: Virally Driven Cancers as a Model System. Mol. Ther. - Nucleic Acids 8, 56–63. doi:10.1016/j.omtn.2017.06.006
Kamali Shahri, S. M., Sharifi, S., and Mahmoudi, M. (2021). Interdependency of Influential Parameters in Therapeutic Nanomedicine. Expert Opin. Drug Deliv. 18, 1379–1394. doi:10.1080/17425247.2021.1921732
Kamaly, N., Yameen, B., Wu, J., and Farokhzad, O. C. (2016). Degradable Controlled-Release Polymers and Polymeric Nanoparticles: Mechanisms of Controlling Drug Release. Chem. Rev. 116, 2602–2663. doi:10.1021/acs.chemrev.5b00346
Karjoo, Z., Chen, X., and Hatefi, A. (2016). Progress and Problems with the Use of Suicide Genes for Targeted Cancer Therapy. Adv. Drug Deliv. Rev. 99, 113–128. doi:10.1016/j.addr.2015.05.009
Kim, D., Wu, Y., Shim, G., and Oh, Y. K. (2021). Genome-Editing-Mediated Restructuring of Tumor Immune Microenvironment for Prevention of Metastasis. ACS Nano 15, 17635–17656. doi:10.1021/acsnano.1c05420
Large, D. E., Soucy, J. R., Hebert, J., and Auguste, D. T. (2019). Advances in Receptor-Mediated, Tumor-Targeted Drug Delivery. Adv. Therap. 2, 1800091. doi:10.1002/adtp.201800091
Lee, K. S., Burke, T. R., Park, J.-E., Bang, J. K., and Lee, E. (2015). Recent Advances and New Strategies in Targeting Plk1 for Anticancer Therapy. Trends Pharmacol. Sci. 36, 858–877. doi:10.1016/j.tips.2015.08.013
Leonardi, G. C., Falzone, L., Salemi, R., Zanghì, A., Spandidos, D. A., McCubrey, J. A., et al. (2018). Cutaneous Melanoma: From Pathogenesis to Therapy (Review). Int. J. Oncol. 52, 1071–1080. doi:10.3892/ijo.2018.4287
Li, C., Yang, T., Weng, Y., Zhang, M., Zhao, D., Guo, S., et al. (2022). Ionizable Lipid-Assisted Efficient Hepatic Delivery of Gene Editing Elements for Oncotherapy. Bioactive Mater. 9, 590–601. doi:10.1016/j.bioactmat.2021.05.051
Li, X., Pan, Y., Chen, C., Gao, Y., Liu, X., Yang, K., et al. (2021). Hypoxia‐Responsive Gene Editing to Reduce Tumor Thermal Tolerance for Mild‐Photothermal Therapy. Angew. Chem. Int. Ed. 60, 21200–21204. doi:10.1002/anie.202107036
Liu, C., Zhang, L., Liu, H., and Cheng, K. (2017). Delivery Strategies of the CRISPR-Cas9 Gene-Editing System for Therapeutic Applications. J. Controlled Release 266, 17–26. doi:10.1016/j.jconrel.2017.09.012
Liu, J., Zhang, C., Hu, W., and Feng, Z. (2015). Tumor Suppressor P53 and its Mutants in Cancer Metabolism. Cancer Lett. 356, 197–203. doi:10.1016/j.canlet.2013.12.025
Liu, Q., Cai, J., Zheng, Y., Tan, Y., Wang, Y., Zhang, Z., et al. (2019). NanoRNP Overcomes Tumor Heterogeneity in Cancer Treatment. Nano Lett. 19, 7662–7672. doi:10.1021/acs.nanolett.9b02501
Liu, Y., Chen, Q., and Zhang, J.-T. (2004). Tumor Suppressor Gene 14-3-3σ Is Down-Regulated whereas the Proto-Oncogene Translation Elongation Factor 1δ Is Up-Regulated in Non-small Cell Lung Cancers as Identified by Proteomic Profiling. J. Proteome Res. 3, 728–735. doi:10.1021/pr034127+
Liu, Y., Zhao, G., Xu, C.-F., Luo, Y.-L., Lu, Z.-D., and Wang, J. (2018). Systemic Delivery of CRISPR/Cas9 with PEG-PLGA Nanoparticles for Chronic Myeloid Leukemia Targeted Therapy. Biomater. Sci. 6, 1592–1603. doi:10.1039/c8bm00263k
Mao, K., Cong, X., Feng, L., Chen, H., Wang, J., Wu, C., et al. (2019). Intratumoral Delivery of M-CSF by Calcium Crosslinked Polymer Micelles Enhances Cancer Immunotherapy. Biomater. Sci. 7, 2769–2776. doi:10.1039/c9bm00226j
Mao, Y., Yang, D., He, J., and Krasna, M. J. (2016). Epidemiology of Lung Cancer. Surg. Oncol. Clin. North America 25, 439–445. doi:10.1016/j.soc.2016.02.001
Martín-Sabroso, C., Fraguas-Sánchez, A. I., Raposo-González, R., and Torres-Suárez, A. I. (2021). Perspectives in Breast and Ovarian Cancer Chemotherapy by Nanomedicine Approach: Nanoformulations in Clinical Research. Curr. Med. Chem. 28, 3271–3286. doi:10.2174/0929867327666200819115403
Mehierhumbert, S., and Guy, R. (2005). Physical Methods for Gene Transfer: Improving the Kinetics of Gene Delivery into Cells. Adv. Drug Deliv. Rev. 57, 733–753. doi:10.1016/j.addr.2004.12.007
Melamed, J. R., Edelstein, R. S., and Day, E. S. (2015). Elucidating the Fundamental Mechanisms of Cell Death Triggered by Photothermal Therapy. ACS Nano 9, 6–11. doi:10.1021/acsnano.5b00021
Milling, L., Zhang, Y., and Irvine, D. J. (2017). Delivering Safer Immunotherapies for Cancer. Adv. Drug Deliv. Rev. 114, 79–101. doi:10.1016/j.addr.2017.05.011
Murphy, B. A., Gilbert, J., and Ridner, S. H. (2007). Systemic and Global Toxicities of Head and Neck Treatment. Expert Rev. Anticancer Ther. 7, 1043–1053. doi:10.1586/14737140.7.7.1043
Nagini, S. (2017). Breast Cancer: Current Molecular Therapeutic Targets and New Players. Anticancer Agents Med. Chem. 17, 152–163. doi:10.2174/1871520616666160502122724
Nassar, K. W., and Tan, A. C. (2020). The Mutational Landscape of Mucosal Melanoma. Semin. Cancer Biol. 61, 139–148. doi:10.1016/j.semcancer.2019.09.013
Okunade, K. S. (2020). Human Papillomavirus and Cervical Cancer. J. Obstet. Gynaecol. 40, 602–608. doi:10.1080/01443615.2019.1634030
Pan, Y., Yang, J., Luan, X., Liu, X., Li, X., Yang, J., et al. (2019). Near-infrared Upconversion-Activated CRISPR-Cas9 System: A Remote-Controlled Gene Editing Platform. Sci. Adv. 5, eaav7199. doi:10.1126/sciadv.aav7199
Peairs, K. S., Choi, Y., Stewart, R. W., and Sateia, H. F. (2017). Screening for Breast Cancer. Semin. Oncol. 44, 60–72. doi:10.1053/j.seminoncol.2017.02.004
Pham, D. D. M., Guhan, S., and Tsao, H. (2020). KIT and Melanoma: Biological Insights and Clinical Implications. Yonsei Med. J. 61, 562–571. doi:10.3349/ymj.2020.61.7.562
Phillips, M. C., and Mousa, S. A. (2022). Clinical Application of Nano-Targeting for Enhancing Chemotherapeutic Efficacy and Safety in Cancer Management. Nanomedicine 17, 405–421. doi:10.2217/nnm-2021-0361
Pimple, S. A., and Mishra, G. A. (2019). Global Strategies for Cervical Cancer Prevention and Screening. Minerva Ginecol 71, 313–320. doi:10.23736/S0026-4784.19.04397-1
Pon, J. R., and Marra, M. A. (2015). Driver and Passenger Mutations in Cancer. Annu. Rev. Pathol. Mech. Dis. 10, 25–50. doi:10.1146/annurev-pathol-012414-040312
Prado, G., Svoboda, R. M., and Rigel, D. S. (2019). What's New in Melanoma. Dermatol. Clin. 37, 159–168. doi:10.1016/j.det.2018.12.005
Radecka, B., and Litwiniuk, M. (2016). Breast Cancer in Young Women. Ginekol Pol. 87, 659–663. doi:10.5603/gp.2016.0062
Rastrelli, M., Tropea, S., Rossi, C. R., and Alaibac, M. (2014). Melanoma: Epidemiology, Risk Factors, Pathogenesis, Diagnosis and Classification. In Vivo 28, 1005–1011.
Redd Bowman, K. E., Lu, P., Vander Mause, E. R., and Lim, C. S. (2020). Advances in Delivery Vectors for Gene Therapy in Liver Cancer. Ther. Deliv. 11, 833–850. doi:10.4155/tde-2019-0076
Ren, X.-H., Xu, C., Li, L.-L., Zuo, Y., Han, D., He, X.-Y., et al. (2022). A Targeting Delivery System for Effective Genome Editing in Leukemia Cells to Reverse Malignancy. J. Controlled Release 343, 645–656. doi:10.1016/j.jconrel.2022.02.012
Riley, R. S., June, C. H., Langer, R., and Mitchell, M. J. (2019). Delivery Technologies for Cancer Immunotherapy. Nat. Rev. Drug Discov. 18, 175–196. doi:10.1038/s41573-018-0006-z
Romaszko, A., and Doboszyńska, A. (2018). Multiple Primary Lung Cancer: A Literature Review. Adv. Clin. Exp. Med. 27, 725–730. doi:10.17219/acem/68631
Rosenblum, D., Gutkin, A., Kedmi, R., Ramishetti, S., Veiga, N., Jacobi, A. M., et al. (2020). CRISPR-Cas9 Genome Editing Using Targeted Lipid Nanoparticles for Cancer Therapy. Sci. Adv. 6, eabc9450. doi:10.1126/sciadv.abc9450
Sánchez-Rivera, F. J., and Jacks, T. (2015). Applications of the CRISPR-Cas9 System in Cancer Biology. Nat. Rev. Cancer 15, 387–393. doi:10.1038/nrc3950
Sapranauskas, R., Gasiunas, G., Fremaux, C., Barrangou, R., Horvath, P., and Siksnys, V. (2011). The Streptococcus Thermophilus CRISPR/Cas System Provides Immunity in Escherichia coli. Nucleic Acids Res. 39, 9275–9282. doi:10.1093/nar/gkr606
Schmid, D., Park, C. G., Hartl, C. A., Subedi, N., Cartwright, A. N., Puerto, R. B., et al. (2017). T Cell-Targeting Nanoparticles Focus Delivery of Immunotherapy to Improve Antitumor Immunity. Nat. Commun. 8, 1747. doi:10.1038/s41467-017-01830-8
Schwartz, G. J., Mehta, R. H., Wenig, B. L., Shaligram, C., and Portugal, L. G. (2000). Salvage Treatment for Recurrent Squamous Cell Carcinoma of the Oral Cavity. Head Neck 22, 34–41. doi:10.1002/(sici)1097-0347(200001)22:1<34::aid-hed6>3.0.co;2-3
Senzer, N., Nemunaitis, J., Nemunaitis, D., Bedell, C., Edelman, G., Barve, M., et al. (2013). Phase I Study of a Systemically Delivered P53 Nanoparticle in Advanced Solid Tumors. Mol. Ther. 21, 1096–1103. doi:10.1038/mt.2013.32
Somia, N., and Verma, I. M. (2000). Gene Therapy: Trials and Tribulations. Nat. Rev. Genet. 1, 91–99. doi:10.1038/35038533
Song, L., Zhou, X., Dai, X., Wang, R., Cheng, G., Zhao, N., et al. (2018). Self-destructible Polysaccharide Nanocomposites with Unlockable Au Nanorods for High-Performance Photothermal Therapy. NPG Asia Mater. 10, 509–521. doi:10.1038/s41427-018-0053-2
Sun, X., Cao, Z., Mao, K., Wu, C., Chen, H., Wang, J., et al. (2020). Photodynamic Therapy Produces Enhanced Efficacy of Antitumor Immunotherapy by Simultaneously Inducing Intratumoral Release of Sorafenib. Biomaterials 240, 119845. doi:10.1016/j.biomaterials.2020.119845
Tabernero, J., Shapiro, G. I., LoRusso, P. M., Cervantes, A., Schwartz, G. K., Weiss, G. J., et al. (2013). First-in-humans Trial of an RNA Interference Therapeutic Targeting VEGF and KSP in Cancer Patients with Liver Involvement. Cancer Discov. 3, 406–417. doi:10.1158/2159-8290.cd-12-0429
Tao, Y., Yi, K., Hu, H., Shao, D., and Li, M. (2021). Coassembly of Nucleus-Targeting Gold Nanoclusters with CRISPR/Cas9 for Simultaneous Bioimaging and Therapeutic Genome Editing. J. Mater. Chem. B 9, 94–100. doi:10.1039/d0tb01925a
Thomas, R. G., Surendran, S. P., and Jeong, Y. Y. (2020). Tumor Microenvironment-Stimuli Responsive Nanoparticles for Anticancer Therapy. Front. Mol. Biosci. 7, 610533. doi:10.3389/fmolb.2020.610533
Tian, H., Lyu, Y., Yang, Y.-G., and Hu, Z. (2020). Humanized Rodent Models for Cancer Research. Front. Oncol. 10, 1696. doi:10.3389/fonc.2020.01696
Uchida, S., and Kataoka, K. (2019). Design Concepts of Polyplex Micelles Forin Vivotherapeutic Delivery of Plasmid DNA and Messenger RNA. J. Biomed. Mater. Res. 107, 978–990. doi:10.1002/jbm.a.36614
Valverde-López, F., Angeles López Garrido, M., Ortega-Suazo, E. J., Vadillo-Calles, F., Muffak-Granero, K., and Nogueras-López, F. (2018). Results of 15-Year Experience in Liver Transplant for Hepatocellular Carcinoma. Transplant. Proc. 50, 617–618. doi:10.1016/j.transproceed.2017.11.050
Vankayala, R., Kuo, C.-L., Nuthalapati, K., Chiang, C.-S., and Hwang, K. C. (2015). Nucleus-Targeting Gold Nanoclusters for Simultaneous In Vivo Fluorescence Imaging, Gene Delivery, and NIR-Light Activated Photodynamic Therapy. Adv. Funct. Mater. 25, 5934–5945. doi:10.1002/adfm.201502650
von Witzleben, A., Wang, C., Laban, S., Savelyeva, N., and Ottensmeier, C. H. (2020). HNSCC: Tumour Antigens and Their Targeting by Immunotherapy. Cells 9, 2103. doi:10.3390/cells9092103
Waghray, A., Murali, A. R., and Menon, K. N. (2015). Hepatocellular Carcinoma: From Diagnosis to Treatment. World J. Hepatol. 7, 1020–1029. doi:10.4254/wjh.v7.i8.1020
Wang, C.-S., Chang, C.-H., Tzeng, T.-Y., Lin, A. M.-Y., and Lo, Y.-L. (2021). Gene-editing by CRISPR-Cas9 in Combination with Anthracycline Therapy via Tumor Microenvironment-Switchable, EGFR-Targeted, and Nucleus-Directed Nanoparticles for Head and Neck Cancer Suppression. Nanoscale Horiz. 6, 729–743. doi:10.1039/d1nh00254f
Wang, P., Zhang, L., Xie, Y., Wang, N., Tang, R., Zheng, W., et al. (2017). Genome Editing for Cancer Therapy: Delivery of Cas9 Protein/sgRNA Plasmid via a Gold Nanocluster/Lipid Core-Shell Nanocarrier. Adv. Sci. 4, 1700175. doi:10.1002/advs.201700175
Webb, P. M., and Jordan, S. J. (2017). Epidemiology of Epithelial Ovarian Cancer. Best Pract. Res. Clin. Obstet. Gynaecol. 41, 3–14. doi:10.1016/j.bpobgyn.2016.08.006
Whitehead, K. A., Dorkin, J. R., Vegas, A. J., Chang, P. H., Veiseh, O., Matthews, J., et al. (2014). Degradable Lipid Nanoparticles with Predictable In Vivo siRNA Delivery Activity. Nat. Commun. 5, 4277. doi:10.1038/ncomms5277
Wirsching, H.-G., Galanis, E., and Weller, M. (2016). Glioblastoma. Handb Clin. Neurol. 134, 381–397. doi:10.1016/b978-0-12-802997-8.00023-2
Wu, C., Chen, H., Wu, X., Cong, X., Wang, L., Wang, Y., et al. (2017). The Influence of Tumor-Induced Immune Dysfunction on the Immune Cell Distribution of Gold Nanoparticles In Vivo. Biomater. Sci. 5, 1531–1536. doi:10.1039/c7bm00335h
Wu, X., Chen, H., Wu, C., Wang, J., Zhang, S., Gao, J., et al. (2018). Inhibition of Intrinsic Coagulation Improves Safety and Tumor-Targeted Drug Delivery of Cationic Solid Lipid Nanoparticles. Biomaterials 156, 77–87. doi:10.1016/j.biomaterials.2017.11.040
Xu, Y., Liu, R., and Dai, Z. (2020). Key Considerations in Designing CRISPR/Cas9-carrying Nanoparticles for Therapeutic Genome Editing. Nanoscale 12, 21001–21014. doi:10.1039/d0nr05452f
Yan, J., Kang, D. D., and Dong, Y. (2021). Harnessing Lipid Nanoparticles for Efficient CRISPR Delivery. Biomater. Sci. 9, 6001–6011. doi:10.1039/d1bm00537e
Yang, P., Chou, S. J., Li, J., Hui, W., Liu, W., Sun, N., et al. (2020). Supramolecular Nanosubstrate-Mediated Delivery System Enables CRISPR-Cas9 Knockin of Hemoglobin Beta Gene for Hemoglobinopathies. Sci. Adv. 6, eabb7107. doi:10.1126/sciadv.abb7107
Yang, Q., Zhou, Y., Chen, J., Huang, N., Wang, Z., and Cheng, Y. (2021). Gene Therapy for Drug-Resistant Glioblastoma via Lipid-Polymer Hybrid Nanoparticles Combined with Focused Ultrasound. Int. J. Nanomedicine 16, 185–199. doi:10.2147/ijn.s286221
Yde, S. S., Sjoegren, P., Heje, M., and Stolle, L. B. (2018). Mucosal Melanoma: a Literature Review. Curr. Oncol. Rep. 20, 28. doi:10.1007/s11912-018-0675-0
Yin, H., Kauffman, K. J., and Anderson, D. G. (2017). Delivery Technologies for Genome Editing. Nat. Rev. Drug Discov. 16, 387–399. doi:10.1038/nrd.2016.280
Yin, H., Song, C.-Q., Dorkin, J. R., Zhu, L. J., Li, Y., Wu, Q., et al. (2016). Therapeutic Genome Editing by Combined Viral and Non-viral Delivery of CRISPR System Components In Vivo. Nat. Biotechnol. 34, 328–333. doi:10.1038/nbt.3471
Zhang, B.-C., Luo, B.-Y., Zou, J.-J., Wu, P.-Y., Jiang, J.-L., Le, J.-Q., et al. (2020). Co-delivery of Sorafenib and CRISPR/Cas9 Based on Targeted Core-Shell Hollow Mesoporous Organosilica Nanoparticles for Synergistic HCC Therapy. ACS Appl. Mater. Inter. 12, 57362–57372. doi:10.1021/acsami.0c17660
Zhang, H., Qin, C., An, C., Zheng, X., Wen, S., Chen, W., et al. (2021). Application of the CRISPR/Cas9-based Gene Editing Technique in Basic Research, Diagnosis, and Therapy of Cancer. Mol. Cancer 20, 126. doi:10.1186/s12943-021-01431-6
Zhang, M., Eshraghian, E. A., Jammal, O. A., Zhang, Z., and Zhu, X. (2021). CRISPR Technology: The Engine that Drives Cancer Therapy. Biomed. Pharmacother. 133, 111007. doi:10.1016/j.biopha.2020.111007
Zhang, P., Chen, D., Li, L., and Sun, K. (2022). Charge Reversal Nano-Systems for Tumor Therapy. J. Nanobiotechnol 20, 31. doi:10.1186/s12951-021-01221-8
Zhang, S., Shen, J., Li, D., and Cheng, Y. (2021). Strategies in the Delivery of Cas9 Ribonucleoprotein for CRISPR/Cas9 Genome Editing. Theranostics 11, 614–648. doi:10.7150/thno.47007
Zhang, Z., Wang, Q., Liu, Q., Zheng, Y., Zheng, C., Yi, K., et al. (2019). Dual-Locking Nanoparticles Disrupt the PD-1/pd-L1 Pathway for Efficient Cancer Immunotherapy. Adv. Mater. 31, e1905751. doi:10.1002/adma.201905751
Keywords: gene editing, tumor, nano-drug delivery vectors, gene therapy, gene-editing technique
Citation: Xi S, Yang Y-G, Suo J and Sun T (2022) Research Progress on Gene Editing Based on Nano-Drug Delivery Vectors for Tumor Therapy. Front. Bioeng. Biotechnol. 10:873369. doi: 10.3389/fbioe.2022.873369
Received: 11 February 2022; Accepted: 11 March 2022;
Published: 28 March 2022.
Edited by:
Junjie Li, Innovation Centre of NanoMedicine (iCONM), JapanReviewed by:
Anjaneyulu Dirisala, Innovation Centre of NanoMedicine (iCONM), JapanCopyright © 2022 Xi, Yang, Suo and Sun. This is an open-access article distributed under the terms of the Creative Commons Attribution License (CC BY). The use, distribution or reproduction in other forums is permitted, provided the original author(s) and the copyright owner(s) are credited and that the original publication in this journal is cited, in accordance with accepted academic practice. No use, distribution or reproduction is permitted which does not comply with these terms.
*Correspondence: Tianmeng Sun, dHN1bjQxQGpsdS5lZHUuY24=; Jian Suo, c3VvamlhbkBqbHUuZWR1LmNu
Disclaimer: All claims expressed in this article are solely those of the authors and do not necessarily represent those of their affiliated organizations, or those of the publisher, the editors and the reviewers. Any product that may be evaluated in this article or claim that may be made by its manufacturer is not guaranteed or endorsed by the publisher.
Research integrity at Frontiers
Learn more about the work of our research integrity team to safeguard the quality of each article we publish.