- 1School of Dental Medicine, University of Pennsylvania, Philadelphia, PA, United States
- 2Department of Periodontics, School of Dental Medicine, University of Pennsylvania, Philadelphia, PA, United States
- 3Department of Orthodontics, School of Dental Medicine, University of Pennsylvania, Philadelphia, PA, United States
- 4Key Laboratory of Shannxi Province for Craniofacial Precision Medicine Research, College of Stomatology, Xi’an Jiaotong University, Xi’an, China
- 5Clinical Research Center of Shannxi Province for Dental and Maxillofacial Diseases, College of Stomatology, Xi’an Jiaotong University, Xi’an, China
- 6Department of Orthodontics, College of Stomatology, Xi’an Jiaotong University, Xi’an, China
- 7David Geffen School of Medicine, University of California, Los Angeles, Los Angeles, CA, United States
- 8School of Dentistry, University of California, Los Angeles, Los Angeles, CA, United States
Sufficient alveolar bone is a safeguard for achieving desired outcomes in orthodontic treatment. Moving a tooth into an alveolar bony defect may result in a periodontal defect or worse–tooth loss. Therefore, when facing a pathologic situation such as periodontal bone loss, alveolar clefts, long-term tooth loss, trauma, and thin phenotype, bone grafting is often necessary to augment bone for orthodontic treatment purposes. Currently, diverse bone grafts are used in clinical practice, but no single grafting material shows absolutely superior results over the others. All available materials demonstrate pros and cons, most notably donor morbidity and adverse effects on orthodontic treatment. Here, we review newly developed graft materials that are still in the pre-clinical stage, as well as new combinations of existing materials, by highlighting their effects on alveolar bone regeneration and orthodontic tooth movement. In addition, novel manufacturing techniques, such as bioprinting, will be discussed. This mini-review article will provide state-of-the-art information to assist clinicians in selecting grafting material(s) that enhance alveolar bone augmentation while avoiding unfavorable side effects during orthodontic treatment.
Introduction
To avoid fenestrations or dehiscences during orthodontic tooth movement, it is critical for alveolar bone to possess adequate contour, thickness, and quality (Atwood and Coy, 1971; Abrams et al., 1987; Seifi and Ghoraishian, 2012). Orthodontically moving teeth into a region with reduced alveolar bone can worsen the periodontal status, slow down tooth movement, and cause root resorption or even tooth loss (Reichert et al., 2010). Clinical scenarios such as severe periodontitis, congenital alveolar clefts, long-term tooth loss, and trauma can induce alveolar bone loss (McAllister and Haghighat, 2007). Thus, augmentation of insufficient bone volume is often indicated prior to the initiation of orthodontic treatment.
In addition, patients with a thin phenotype have narrow alveolar bone support, which significantly limits the range of orthodontic tooth movement. To address this issue, the periodontally accelerated osteogenic orthodontics (PAOO) technique has been developed to broaden the biological range of orthodontic treatment by adding bone grafting material to the alveolar cortical surface (Wilcko et al., 2008). Pre-orthodontic bone grafting can also promote easier and less detrimental tooth movement through primary woven bone (Diedrich, 1996). Ideally, bone graft materials for orthodontic treatment should protect the teeth from complications and enhance the alveolar bone phenotype.
Based on where bone grafts are sourced, they may be categorized as autografts, allografts, xenografts, or synthetics. Autografts prevail amongst these categories in the maxillofacial region and are the current gold standard as they [1] consist of an abundance of spongy bone that is close to the alveolar bone structure, [2] display osteoconductive and osteoinductive potential (Boyne and Sands, 1972; Enemark et al., 1987; Ozaki and Buchman, 1998), and [3] promote periodontal regeneration (Ivanovic et al., 2014) without significantly unfavorable sequelae when teeth are orthodontically moved into grafted areas (Lu et al., 2021). However, the drawbacks of autografts are substantial, including but not limited to inadequate availability, expensive cost, mismatched size, and inevitable additional surgery for autograft harvest (Sharif et al., 2016). These limitations lend support to the use of substitute graft materials.
Allografts, such as decalcified freeze-dried bone allogeneic grafts (DFDBA) and freeze-dried bone allogeneic grafts (FDBA), are orthodontic-friendly (Lu et al., 2021); however, their osteoinductive potency is not conclusive (Schwartz et al., 1998). Xenografts, such as Bio-Oss® and Gen-Tech®, are the most common alveolar grafting materials for clinical use. They are successful when used for alveolar bone augmentation (da Silva et al., 2020), but can severely impair orthodontic treatment and cause substantial root resorption when teeth are moved into the grafted region (Lu et al., 2021). Although synthetic bone grafts, such as NanoBone® and BoneCeramic®, also promote bone augmentation, major adverse effects (namely root resorption and gingival invagination) make them an unfavorable choice for pre-orthodontic alveolar bone grafting (Lu et al., 2021). Therefore, there is an emerging need for new grafting materials to be not only osteoinductive and osteoconductive but also supportive of highly active bone metabolism during orthodontic tooth movement without adverse effects.
In this review, we highlight recent research advances in novel alveolar graft materials, as well as new combinations of previously developed materials, with a focus on orthodontic applications supported by pre-clinical and clinical evidence (Table 1).
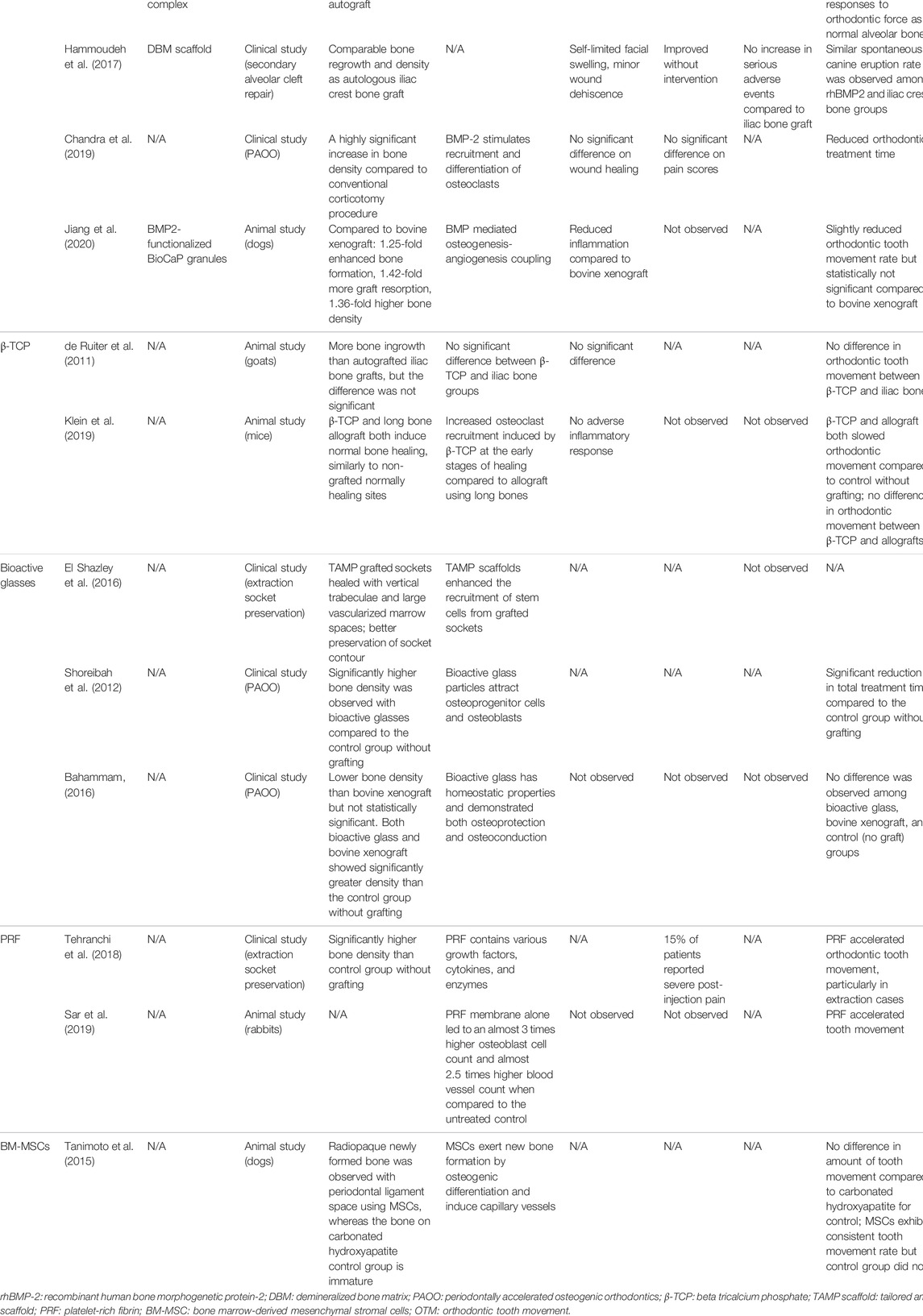
TABLE 1. The alveolar bone regeneration efficiency and the orthodontic impactions of the alveolar bone grafting materials.
Osteoinductive Growth Factor Bone Morphogenetic Protein 2 (BMP2)
Growth factors, cytokines, and chemokines that potentially enhance osteoblast proliferation and function as well as facilitate orthodontic tooth movement have been investigated for use as bone graft materials. For example, recombinant human BMP2 (rhBMP2), a potent osteogenic growth factor, is currently the only Food and Drug Administration (FDA)-approved osteoinductive growth factor for bone graft substitutes (James et al., 2016). In the alveolar region, animal studies show that rhBMP2 with a poly [D,L-(lactide-co-glycolide)]/gelatin sponge complex has superior osteoinductive activity compared to spongiosa from the tibia, and the newly generated bone in both groups shows a similar histological response to orthodontic force as that of normal alveolar bone (Kawamoto et al., 2002). However, root resorption was observed over the 6-months course of tooth movement when the rhBMP2-based graft was used, while no significant resorption was observed in the autograft and control groups (Kawamoto et al., 2002). Moreover, Hammoudeh’s group showed comparable bone regrowth and density values following secondary alveolar cleft repair in humans using a rhBMP2/DBM scaffold with an autologous iliac bone graft (Hammoudeh et al., 2017; Liang et al., 2017). The spontaneous canine eruption rate was similar among different grafting groups (Hammoudeh et al., 2017). In addition, applying rhBMP2 during PAOO procedures increased bone density around corticotomy sites and shortened orthodontic treatment time compared to conventional corticotomy alone (Chandra et al., 2019).
It is worth noting that although the osteoinductive activity of rhBMP2 increases with dose (El Bialy et al., 2017), high-dose rhBMP2 may not be favorable for orthodontic tooth movement. Kawamoto et al. found that high-dose rhBMP2 delays bone remodeling compared to low-dose rhBMP2 (Kawamoto et al., 2003). Moreover, high-dose rhBMP2 induces root resorption, while low-dose rhBMP2 causes only partial cementum resorption on the pressure side (Kawamoto et al., 2003).
To minimize the adverse effects of high-dose rhBMP2 while reducing the cost of this expensive material, rhBMP2-functionalized biomimetic calcium phosphate (BioCap) granules have been developed to achieve controlled and sustained rhBMP2 release. BioCap granules robustly enhanced bone regeneration and graft degradation over deproteinized bovine bone in an animal study (Jiang et al., 2020). In addition, due to its low immunogenicity and high angiogenic potency, BioCap graft reduces inflammation and periodontal probing depth during orthodontic treatment, while only slightly reducing the rate of orthodontic tooth movement (Jiang et al., 2020).
A synergistic effect was observed when rhBMP2 and vascular endothelial growth factor (VEGF) were used together to enhance bone generation around implant sites via an insoluble collagenous bone matrix (Schorn et al., 2017). In this combination, VEGF promotes angiogenesis and enhances osteoblastic differentiation, thereby facilitating craniofacial ossification (Duan et al., 2016), while the matrix acts as a scaffold for migrating osteoblasts. This combination product can reduce surgery time and minimize donor site morbidity while maintaining bone stability, as little resorption was observed over time (Schorn et al., 2017).
Despite its advantages, clinical complications such as significant postoperative facial swelling were observed in patients grafted with rhBMP2 (Hammoudeh et al., 2017). Along with increasing clinical use of rhBMP2 in orthopedics, a growing side-effect profile has emerged, including postoperative inflammation, ectopic bone formation, osteoclast-mediated bone resorption, and inappropriate adipogenesis (James et al., 2016). BMP2 has also been associated with osteosarcoma growth (Tian et al., 2019); this complication has cast doubt on its application after tumor resection. Safe application of rhBMP2 therefore remains an inherent issue to conquer.
Synthetic Inorganic Materials
Unlike autografts, allografts, and xenografts, synthetic materials are free from cross-infection and disease transmission and are not associated with donor site sacrifice. However, synthetic materials, particularly inorganic ones, are often osteoconductive without any osteoinductive or osteogenic potential. β-tricalcium phosphate (β-TCP), hydroxyapatite, and bioactive glasses are the most commonly used inorganic graft materials in periodontal regeneration (Sheikh et al., 2017).
β-tricalcium Phosphate (β-TCP)
TCPs were the first generation of calcium compounds used as bone grafts (Bohner et al., 2020). They are osteoconductive and have a similar composition to bone minerals. TCP has two crystallographic forms, α-TCP and β-TCP (Bohner et al., 2020), with the latter exhibiting good biocompatibility and osteoconductivity. As a graft material for alveolar cleft repair in animals, β-TCP promotes bone regeneration as effectively as autologous iliac crest bone (de Ruiter et al., 2011) and allograft from long bones (Klein et al., 2019). Moreover, no difference in orthodontic movement is observed between β-TCP and autograft (de Ruiter et al., 2011) or allograft (Klein et al., 2019). Since β-TCP shows no significant adverse effects on tooth movement in grafted sites, it is a promising material for further clinical investigation.
Bioactive Glasses
First introduced as a bone graft in early 1970, biocompatible tissue-bonding bioactive glasses are another synthesized inorganic graft material that has received clinical attention. After implantation, a hydroxycarbonate apatite layer and silicon-rich gel layer form on the surface of the bioactive glass. The roles of these layers are to attach to the surrounding bone and attract osteoprogenitor cells and osteoblasts, respectively (Hench, 1991). The composition of a particular bioactive glass (i.e. a combination of silicon dioxide, calcium oxide, sodium oxide, and phosphorus pentoxide) will determine its bioactivity (Shue et al., 2012). For instance, increasing silicon dioxide, decreasing alkali, and supplementing aluminum oxide modulates the durability and water resistance of bioactive glass, thereby altering its reliability and success (Pereira et al., 1994).
Different types of bioactive glass have been tested for alveolar bone grafting and novel modifications have been developed to improve biocompatibility of the material. For example, a novel bioactive glass scaffold, tailored amorphous multiparous (TAMP), was introduced in 2016 for extraction socket preservation (El Shazley et al., 2016). Distinct from non-grafted sockets that showed corticalization after healing, the TAMP-grafted sockets healed with vertical trabeculae and large vascularized marrow spaces (El Shazley et al., 2016). Better preservation of socket contour was also observed with TAMP grafts (El Shazley et al., 2016). In addition, GlassBONE™ (Noraker, France), a synthetic resorbable bioactive glass 45S5 ceramic, has been successfully used for alveolar cleft reconstruction, with satisfactory healing found in two-thirds of tested patients (Graillon et al., 2018).
When bioactive glass is grafted, a significant increase in bone density is noted 6 months after the cessation of tooth movement; this finding may be attributed to the beneficial effects of alkalization on collagen synthesis and hydroxyapatite formation (Shoreibah et al., 2012). In addition, a marked reduction in orthodontic treatment duration was associated with bioactive glass grafting. Periodontal health was also enhanced with negligible apical root resorption and improved probing depth (Shoreibah et al., 2012). Although bioactive glass does not provide the same level of bone density as bovine-derived xenograft, both materials decrease the duration of orthodontic treatment and reduce the risk of root resorption (Bahammam, 2016).
Bioactive glass has also been applied with other grafting materials. For example, a case report from 2000 described how grafting a DFDBA-granular bioactive glass (1:1) mixture in the buccal aspect of the edentulous cleft region of a patient with cleft lip and palate resulted in good bone regeneration and successful orthodontic tooth movement into the grafted site (Yilmaz et al., 2000). However, these results should be interpreted with caution as they are derived from a single case report.
Platelet-Rich Fibrin (PRF)
Endogenous biomaterials have been developed to overcome the limitations associated with current clinical approaches for autografting. PRF is a cost-effective material (Miron and Choukroun, 2017) that is increasingly being used for regenerative dentistry, specifically next-generation autologous platelet therapy (Liu et al., 2019). PRF contains stem cells, growth factors, and cytokines and is obtained through a minimally invasive procedure that centrifuges whole blood without additives (Choukroun et al., 2006). It can modulate inflammation and enhance the healing process, thereby promoting the regenerative capacity of the periosteum (Miron and Choukroun, 2017). In addition, its dense, protein-rich fibrin mesh functions as a three-dimensional fibrous scaffold for cell migration and a retainer for sustained growth factor release (Karimi and Rockwell, 2019).
Both animal (Sar et al., 2019) and clinical studies (Tehranchi et al., 2018) show that PRF significantly accelerates alveolar bone turnover and orthodontic tooth movement, especially at the beginning of orthodontic treatment (Tehranchi et al., 2018). However, 15% of grafted patients experience severe pain attributable to PRF application (Tehranchi et al., 2018), highlighting the need for further investigation. Although the clinical applications of PRF in regenerative dentistry have grown in recent years (Miron and Choukroun, 2017), its application in orthodontics is limited. It is largely unknown if the content variation of PRF from different patients or the same patient at different health statuses will impact its outcome as a graft in orthodontic treatment. Additionally, since PRF contains donor cells, it is not suitable to be used as an allograft. Its usage as an autograft material is also limited by availability when extracted from the patient’s blood (Choukroun et al., 2006).
Pluri and Multipotent Cells
Over the last few decades, multiple pluri- and multi-potent cells have been explored for use in bone augmentation (Li C. et al., 2021; Holly et al., 2021). Bone marrow is the main source of MSCs for clinical applications; in fact, bone marrow-derived mesenchymal stromal cells (BM-MSCs) were the first MSCs to be discovered (Strioga et al., 2012). Compared to iliac crest bone grafts, resorbable collagen sponges combined with BM-MSCs provide similar bone healing results in the closure of alveolar cleft defects with reduced donor site morbidity and decreased donor site pain intensity and frequency (Gimbel et al., 2007).
Recently, successful bone regeneration has been reported using autogenous BM-MSCs in a dog model of an artificial alveolar cleft. In this study, new bone formation was achieved, thereby allowing orthodontic tooth movement beyond the anatomical limit (Tanimoto et al., 2015). Furthermore, a consistent rate of orthodontic tooth movement was observed in the experimental group compared to varied rates in the control group (Tanimoto et al., 2015), suggesting that MSCs in bone graft materials may have a modulatory effect on the bone remodeling process during orthodontic treatment. In alignment with this observation, the expression of RANKL, a molecule that regulates osteoclastic differentiation, was significantly increased in BM-MSCs under compressive stress (Wang et al., 2021). This finding suggests that BM-MSCs may accelerate tooth movement by expressing cytokines that promote osteoclastogenesis.
Due to ease of accessibility, dental-derived MSCs have gained attention in the past few years and have entered clinical trials (Paz et al., 2018). First isolated from the dental pulp of extracted third molars, dental-derived MSCs have now been purified from various dental tissues, including pulp tissue of permanent teeth and exfoliated deciduous teeth, apical papilla, periodontal ligament, gingiva, dental follicle, tooth germ, and alveolar bone (Gan et al., 2020). Dental-derived MSCs not only display the same characteristics as BM-MSCs but also possess immunomodulatory and anti-inflammatory advantages in the local dental tissue environment (Spagnuolo et al., 2018). Tanikawa et al. utilized autologous deciduous dental pulp stem cells for maxillary alveolar reconstruction and achieved progressive alveolar bone union without grafting site complications in cleft lip and palate patients (Tanikawa et al., 2020). Previous studies have also suggested that gingival-derived MSCs have great potential for repairing alveolar bone defects (Gao and Cao, 2020; Kandalam et al., 2021). However, the impact of dental-derived MSCs on orthodontic tooth movement is not yet well understood.
Materials With 3D Printed Scaffolds
Conventional bone grafts, such as allografts and xenografts, often fail to provide the support necessary to maintain the desired generated tissue volume, especially under the mechanical forces in the oral cavity (Seciu et al., 2019). This is particularly challenging for vertical bone augmentation or personalized esthetic bone reconstruction, where highly tailored bone contours and structural stability are required. To overcome this obstacle, materials with three-dimensional architecture mimicking the anatomical and histological arrangement of natural bone have been developed (Kim et al., 2010).
Recent advances in microfabrication, particularly 3D bio-printing, support the construction of complex structures from bioactive/biodegradable materials, including polymers, bioceramics, and composites [as reviewed in (Asa’ad et al., 2016)]. In a recent study, a 3D-printed calcium phosphate scaffold was fabricated according to the geometry of artificial alveolar clefts in rats and showed promising scaffolding and osteoconductive properties (Korn et al., 2020). A 3D-printed custom hydroxyapatite/TCP graft supplied with rhBMP2 also achieved bone regeneration to the same level of rhBMP2-coupled deproteinized bovine bone material (Bio-Oss®) (Ryu et al., 2021). Although the exact mechanism of how 3D-printed scaffolds benefit orthodontic tooth movement remains unmapped, evidence suggests that grafting with 3D-printed scaffolds may offer enhanced orthodontic outcomes.
Conclusion and Future Directions
Optimizing esthetics, providing functional and comfortable occlusion, and improving overall health are all goals of successful orthodontic treatment, for which preservation of the alveolar bone is a crucial limiting factor. Most materials reviewed in this article mediate accelerated orthodontic tooth movement and thus can reduce treatment duration and cost. These features are particularly attractive to patients facing extended treatment times, such as those in need of tooth extractions and additional periodontal support. Although a quantitative report is not currently realistic due to the limited available research to date, qualitatively analyzing pre-clinical novel materials will provide insight for their future usage in regenerative orthodontics. High-quality randomized controlled trials with larger sample sizes and longer follow-up periods are nevertheless warranted for translating these novel biological concepts into clinical practice. In our opinion, future exploration should also aim to reveal the potential long-term complications of these materials, as well as their impacts on growth and development in adolescents.
A rising number of reports suggest that adjunct treatments can support grafting and have the potential to improve orthodontic treatment. For example, the possibility of vibration accelerating orthodontic tooth movement has been a hot study topic over the last decade (Telatar and Gungor, 2021; Mayama et al., 2022). At the same time, studies have shown that high-frequency vibration treatment increases osteogenic differentiation of human BM-MSCs in vitro (Pre et al., 2013) and low-level mechanical vibration stimulates osteogenesis and osteointegration of porous titanium implants in the repair of long bone defects (Jing et al., 2015). In addition, low-intensity pulsed ultrasound (LIPUS) has been proven to accelerate new alveolar bone formation in a periodontal injury animal model (Wang et al., 2018) and enhance BM-MSCs-based periodontal regenerative therapies (Wang et al., 2022). Moreover, LIPUS can shorten the overall duration of orthodontic treatment (Kaur and El-Bialy, 2020) and minimize orthodontically-induced tooth root resorption (El-Bialy et al., 2020). Last but not least, laser photobiomodulation in combination with PRF demonstrated better bone healing than PRF alone in an iliac crest critical-sized bone defect sheep model (Surmeli Baran et al., 2021). Photobiomodulation was also found to enhance bone formation of hydroxyapatite biomaterial in the dental alveolus in an experimental extraction rat model (Dalapria et al., 2022). On the other hand, the effects of photobiomodulation on orthodontic treatment have started to attract attention (Li J. et al., 2021; Yavagal et al., 2021). In all, a detailed assessment of the influence of adjunct treatments with different grafting materials on orthodontic tooth movement is warranted to further optimize treatment outcomes.
Author Contributions
YM, writing—original draft preparation, review and editing. Y-CC, NT, C-HC, NA, MZ, and ZZ, writing—review and editing. CL, conceptualization, writing—review and editing, funding acquisition. All authors have read and agreed to the published version of the manuscript.
Funding
This study was supported by the American Academy of Periodontology Foundation Teaching Fellowship and Schoenleber Pilot Grant, University of Pennsylvania for Y-CC; and the American Association of Orthodontists Foundation (AAOF) Orthodontic Faculty Development Fellowship Award, American Association of Orthodontists (AAO) Full-Time Faculty Fellowship Award, University of Pennsylvania School of Dental Medicine Joseph and Josephine Rabinowitz Award for Excellence in Research, and J. Henry O’Hern Jr Pilot Grant from the Department of Orthodontics, University of Pennsylvania School of Dental Medicine for CL.
Conflict of Interest
The authors declare that the research was conducted in the absence of any commercial or financial relationships that could be construed as a potential conflict of interest.
Publisher’s Note
All claims expressed in this article are solely those of the authors and do not necessarily represent those of their affiliated organizations, or those of the publisher, the editors and the reviewers. Any product that may be evaluated in this article, or claim that may be made by its manufacturer, is not guaranteed or endorsed by the publisher.
References
Abrams, H., Kopczyk, R. A., and Kaplan, A. L. (1987). Incidence of Anterior Ridge Deformities in Partially Edentulous Patients. J. Prosthet. Dent. 57 (2), 191–194. doi:10.1016/0022-3913(87)90145-4
Asaad, F., Pagni, G., Pilipchuk, S. P., Giannì, A. B., Giannobile, W. V., and Rasperini, G. (2016). 3D-Printed Scaffolds and Biomaterials: Review of Alveolar Bone Augmentation and Periodontal Regeneration Applications. Int. J. Dent. 2016, 1–15. doi:10.1155/2016/1239842
Atwood, D. A., and Coy, W. A. (1971). Clinical, Cephalometric, and Densitometric Study of Reduction of Residual Ridges. J. Prosthet. Dent. 26 (3), 280–295. doi:10.1016/0022-3913(71)90070-9
Bahammam, M. A. (2016). Effectiveness of Bovine-Derived Xenograft versus Bioactive Glass with Periodontally Accelerated Osteogenic Orthodontics in Adults: a Randomized, Controlled Clinical Trial. BMC Oral Health 16 (1), 126. doi:10.1186/s12903-016-0321-x
Bohner, M., Santoni, B. L. G., and Döbelin, N. (2020). β-Tricalcium Phosphate for Bone Substitution: Synthesis and Properties. Acta Biomater. 113, 23–41. doi:10.1016/j.actbio.2020.06.022
Boyne, P. J., and Sands, N. R. (1972). Secondary Bone Grafting of Residual Alveolar and Palatal Clefts. J. Oral Surg. 30 (2), 87–92.
Chandra, R., Rachala, M., Madhavi, K., Kambalyal, P., Reddy, A., and Ali, M. (2019). Periodontally Accelerated Osteogenic Orthodontics Combined with Recombinant Human Bone Morphogenetic Protein-2: An Outcome Assessment. J. Indian Soc. Periodontol. 23 (3), 257–263. doi:10.4103/jisp.jisp_612_18
Choukroun, J., Diss, A., Simonpieri, A., Girard, M.-O., Schoeffler, C., Dohan, S. L., et al. (2006). Platelet-rich Fibrin (PRF): a Second-Generation Platelet Concentrate. Part IV: Clinical Effects on Tissue Healing. Oral Surg. Oral Med. Oral Pathology, Oral Radiology, Endodontology 101 (3), e56–e60. doi:10.1016/j.tripleo.2005.07.011
de Ruiter, R., Meijer, G., Dormaar, T., Janssen, N., van der Bilt, A., Slootweg, P., et al. (2011). β-TCP versus Autologous Bone for Repair of Alveolar Clefts in a Goat Model. Cleft Palate-Craniofacial J. 48 (6), 654–662. doi:10.1597/09-219
da Silva, H. F., Goulart, D. R., Sverzut, A. T., Olate, S., and de Moraes, M. (2020). Comparison of Two Anorganic Bovine Bone in Maxillary Sinus Lift: a Split-Mouth Study with Clinical, Radiographical, and Histomorphometrical Analysis. Int. J. Implant Dent. 6 (1), 17. doi:10.1186/s40729-020-00214-w
Dalapria, V., Marcos, R. L., Bussadori, S. K., Anselmo, G., Benetti, C., da Silva Santana, A. C. A., et al. (2022). LED Photobiomodulation Therapy Combined with Biomaterial as a Scaffold Promotes Better Bone Quality in the Dental Alveolus in an Experimental Extraction Model. Lasers Med. Sci. 37 (3), 1583–1592. doi:10.1007/s10103-021-03407-w
Diedrich, P. R. (1996). Guided Tissue Regeneration Associated with Orthodontic Therapy. Seminars Orthod. 2 (1), 39–45. doi:10.1016/s1073-8746(96)80038-7
Duan, X., Bradbury, S. R., Olsen, B. R., and Berendsen, A. D. (2016). VEGF Stimulates Intramembranous Bone Formation during Craniofacial Skeletal Development. Matrix Biol. 52-54, 127–140. doi:10.1016/j.matbio.2016.02.005
El Bialy, I., Jiskoot, W., and Reza Nejadnik, M. (2017). Formulation, Delivery and Stability of Bone Morphogenetic Proteins for Effective Bone Regeneration. Pharm. Res. 34 (6), 1152–1170. doi:10.1007/s11095-017-2147-x
El Shazley, N., Hamdy, A., El-Eneen, H. A., El Backly, R. M., Saad, M. M., Essam, W., et al. (2016). Bioglass in Alveolar Bone Regeneration in Orthodontic Patients. JDR Clin. Transl. Res. 1 (3), 244–255. doi:10.1177/2380084416660672
El-Bialy, T., Farouk, K., Carlyle, T. D., Wiltshire, W., Drummond, R., Dumore, T., et al. (2020). Effect of Low Intensity Pulsed Ultrasound (LIPUS) on Tooth Movement and Root Resorption: A Prospective Multi-Center Randomized Controlled Trial. Jcm 9 (3), 804. doi:10.3390/jcm9030804
Enemark, H., Sindet-Pedersen, S., and Bundgaard, M. (1987). Long-term Results after Secondary Bone Grafting of Alveolar Clefts. J. Oral Maxillofac. Surg. 45 (11), 913–918. doi:10.1016/0278-2391(87)90439-3
Gan, L., Liu, Y., Cui, D., Pan, Y., Zheng, L., and Wan, M. (2020). Dental Tissue-Derived Human Mesenchymal Stem Cells and Their Potential in Therapeutic Application. Stem Cells Int. 2020, 1–17. doi:10.1155/2020/8864572
Gao, X., and Cao, Z. (2020). Gingiva-derived Mesenchymal Stem Cells and Their Potential Applications in Oral and Maxillofacial Diseases. Cscr 15 (1), 43–53. doi:10.2174/1574888X14666191107100311
Gimbel, M., Ashley, R. K., Sisodia, M., Gabbay, J. S., Wasson, K. L., Heller, J., et al. (2007). Repair of Alveolar Cleft Defects. J. Craniofac Surg. 18 (4), 895–901. doi:10.1097/scs.0b013e3180a771af
Graillon, N., Degardin, N., Foletti, J. M., Seiler, M., Alessandrini, M., and Gallucci, A. (2018). Bioactive Glass 45S5 Ceramic for Alveolar Cleft Reconstruction, about 58 Cases. J. Cranio-Maxillofacial Surg. 46 (10), 1772–1776. doi:10.1016/j.jcms.2018.07.016
Hammoudeh, J. A., Fahradyan, A., Gould, D. J., Liang, F., Imahiyerobo, T., Urbinelli, L., et al. (2017). A Comparative Analysis of Recombinant Human Bone Morphogenetic Protein-2 with a Demineralized Bone Matrix versus Iliac Crest Bone Graft for Secondary Alveolar Bone Grafts in Patients with Cleft Lip and Palate. Plastic Reconstr. Surg. 140 (2), 318e–325e. doi:10.1097/PRS.0000000000003519
Hench, L. L. (1991). Bioceramics: from Concept to Clinic. J Am. Ceram. Soc. 74 (7), 1487–1510. doi:10.1111/j.1151-2916.1991.tb07132.x
Hollý, D., Klein, M., Mazreku, M., Zamborský, R., Polák, Š., Danišovič, Ľ., et al. (2021). Stem Cells and Their Derivatives-Implications for Alveolar Bone Regeneration: A Comprehensive Review. Ijms 22 (21), 11746. doi:10.3390/ijms222111746
Ivanovic, A., Nikou, G., Miron, R. J., Nikolidakis, D., and Sculean, A. (2014). Which Biomaterials May Promote Periodontal Regeneration in Intrabony Periodontal Defects? A Systematic Review of Preclinical Studies. Quintessence Int. 45 (5), 385–395. doi:10.3290/j.qi.a31538
James, A. W., LaChaud, G., Shen, J., Asatrian, G., Nguyen, V., Zhang, X., et al. (2016). A Review of the Clinical Side Effects of Bone Morphogenetic Protein-2. Tissue Eng. Part B Rev. 22 (4), 284–297. doi:10.1089/ten.TEB.2015.0357
Jiang, S., Liu, T., Wu, G., Li, W., Feng, X., Pathak, J. L., et al. (2020). BMP2-Functionalized Biomimetic Calcium Phosphate Graft Promotes Alveolar Defect Healing during Orthodontic Tooth Movement in Beagle Dogs. Front. Bioeng. Biotechnol. 8, 517. doi:10.3389/fbioe.2020.00517
Jing, D., Tong, S., Zhai, M., Li, X., Cai, J., Wu, Y., et al. (2015). Effect of Low-Level Mechanical Vibration on Osteogenesis and Osseointegration of Porous Titanium Implants in the Repair of Long Bone Defects. Sci. Rep. 5, 17134. doi:10.1038/srep17134
Kandalam, U., Kawai, T., Ravindran, G., Brockman, R., Romero, J., Munro, M., et al. (2021). Predifferentiated Gingival Stem Cell-Induced Bone Regeneration in Rat Alveolar Bone Defect Model. Tissue Eng. Part A 27 (5-6), 424–436. doi:10.1089/ten.TEA.2020.0052
Karimi, K., and Rockwell, H. (2019). The Benefits of Platelet-Rich Fibrin. Facial Plastic Surg. Clin. N. Am. 27 (3), 331–340. doi:10.1016/j.fsc.2019.03.005
Kaur, H., and El-Bialy, T. (2020). Shortening of Overall Orthodontic Treatment Duration with Low-Intensity Pulsed Ultrasound (LIPUS). Jcm 9 (5), 1303. doi:10.3390/jcm9051303
Kawamoto, T., Motohashi, N., Kitamura, A., Baba, Y., Suzuki, S., and Kuroda, T. (2003). Experimental Tooth Movement into Bone Induced by Recombinant Human Bone Morphogenetic Protein-2. Cleft Palate-Craniofacial J. 40 (5), 538–543. doi:10.1597/1545-1569_2003_040_0538_etmibi_2.0.co_210.1597/1545-1569(2003)040<0538:etmibi>2.0.co;2
Kawamoto, T., Motohashi, N., Kitamura, A., Baba, Y., Takahashi, K., Suzuki, S., et al. (2002). A Histological Study on Experimental Tooth Movement into Bone Induced by Recombinant Human Bone Morphogenetic Protein-2 in Beagle Dogs. Cleft Palate-Craniofacial J. 39 (4), 439–448. doi:10.1597/1545-1569_2002_039_0439_ahsoet_2.0.co_2
Kim, K., Lee, C. H., Kim, B. K., and Mao, J. J. (2010). Anatomically Shaped Tooth and Periodontal Regeneration by Cell Homing. J. Dent. Res. 89 (8), 842–847. doi:10.1177/0022034510370803
Klein, Y., Kunthawong, N., Fleissig, O., Casap, N., Polak, D., and Chaushu, S. (2020). The Impact of Alloplast and Allograft on Bone Homeostasis: Orthodontic Tooth Movement into Regenerated Bone. J. Periodontol. 91, 1067–1075. doi:10.1002/JPER.19-0145
Korn, P., Ahlfeld, T., Lahmeyer, F., Kilian, D., Sembdner, P., Stelzer, R., et al. (2020). 3D Printing of Bone Grafts for Cleft Alveolar Osteoplasty - In Vivo Evaluation in a Preclinical Model. Front. Bioeng. Biotechnol. 8, 217. doi:10.3389/fbioe.2020.00217
Li, C., Mills, Z., and Zheng, Z. (2021a). Novel Cell Sources for Bone Regeneration. MedComm 2 (2), 145–174. doi:10.1002/mco2.51
Li, J., Ge, X., Guan, H., Jia, L., Chang, W., and Ma, W. (2021b). The Effectiveness of Photobiomodulation on Accelerating Tooth Movement in Orthodontics: A Systematic Review and Meta-Analysis. Photobiomodulation, Photomed. Laser Surg. 39 (4), 232–244. doi:10.1089/photob.2020.4954
Liang, F., Yen, S. L.-K., Imahiyerobo, T., Sanborn, L., Yen, L., Yen, D., et al. (2017). Three-Dimensional Cone Beam Computed Tomography Volumetric Outcomes of rhBMP-2/Demineralized Bone Matrix versus Iliac Crest Bone Graft for Alveolar Cleft Reconstruction. Plastic Reconstr. Surg. 140 (4), 767–774. doi:10.1097/PRS.0000000000003686
Liu, Y., Sun, X., Yu, J., Wang, J., Zhai, P., Chen, S., et al. (2019). Platelet-rich Fibrin as a Bone Graft Material in Oral and Maxillofacial Bone Regeneration: Classification and Summary for Better Application. BioMed Res. Int. 2019, 3295756. doi:10.1155/2019/3295756
Lu, J., Wang, Z., Zhang, H., Xu, W., Zhang, C., Yang, Y., et al. (2022). Bone Graft Materials for Alveolar Bone Defects in Orthodontic Tooth Movement. Tissue Eng. Part B Rev. 28, 35–51. doi:10.1089/ten.TEB.2020.0212
Mayama, A., Seiryu, M., and Takano-Yamamoto, T. (2022). Effect of Vibration on Orthodontic Tooth Movement in a Double Blind Prospective Randomized Controlled Trial. Sci. Rep. 12 (1), 1288. doi:10.1038/s41598-022-05395-5
McAllister, B. S., and Haghighat, K. (2007). Bone Augmentation Techniques. J. Periodontology 78 (3), 377–396. doi:10.1902/jop.2007.060048
Miron, R. J., and Choukroun, J. (2017). Platelet Rich Fibrin in Regenerative Dentistry: Biological Background and Clinical Indications. Hoboken, NJ: John Wiley & Sons, Inc.
Ozaki, W., and Buchman, S. R. (1998). Volume Maintenance of Onlay Bone Grafts in the Craniofacial Skeleton: Micro-architecture versus Embryologic Origin. Plastic Reconstr. Surg. 102 (2), 291–299. doi:10.1097/00006534-199808000-00001
Paz, A. G., Maghaireh, H., and Mangano, F. G. (2018). Stem Cells in Dentistry: Types of Intra- and Extraoral Tissue-Derived Stem Cells and Clinical Applications. Stem Cells Int. 2018, 1–14. doi:10.1155/2018/4313610
Pereira, M. M., Clark, A. E., and Hench, L. L. (1994). Calcium Phosphate Formation on Sol-Gel-Derived Bioactive Glassesin Vitro. J. Biomed. Mat. Res. 28 (6), 693–698. doi:10.1002/jbm.820280606
Prè, D., Ceccarelli, G., Visai, L., Benedetti, L., Imbriani, M., Cusella De Angelis, M. G., et al. (2013). High-Frequency Vibration Treatment of Human Bone Marrow Stromal Cells Increases Differentiation toward Bone Tissue. Bone Marrow Res. 2013, 1–13. doi:10.1155/2013/803450
Reichert, C., Götz, W., Smeets, R., Wenghöfer, M., and Jäger, A. (2010). The Impact of Nonautogenous Bone Graft on Orthodontic Treatment. Quintessence Int. 41 (8), 665–672.
Ryu, J.-I., Yang, B.-E., Yi, S.-M., Choi, H.-G., On, S.-W., Hong, S.-J., et al. (2021). Bone Regeneration of a 3D-Printed Alloplastic and Particulate Xenogenic Graft with rhBMP-2. Ijms 22 (22), 12518. doi:10.3390/ijms222212518
Sar, C., Akdeniz, S. S., Arman Ozcirpici, A., Helvacioglu, F., and Bacanlı, D. (2019). Histological Evaluation of Combined Platelet-Rich Fibrin Membrane and Piezo-Incision Application in Orthodontic Tooth Movement. Int. J. Oral Maxillofac. Surg. 48 (10), 1380–1385. doi:10.1016/j.ijom.2019.04.001
Schorn, L., Sproll, C., Ommerborn, M., Naujoks, C., Kübler, N. R., and Depprich, R. (2017). Vertical Bone Regeneration Using rhBMP-2 and VEGF. Head. Face Med. 13 (1), 11. doi:10.1186/s13005-017-0146-0
Schwartz, Z., Somers, A., Mellonig, J. T., Carnes, D. L., Dean, D. D., Cochran, D. L., et al. (1998). Ability of Commercial Demineralized Freeze-Dried Bone Allograft to Induce New Bone Formation Is Dependent on Donor Age but Not Gender. J. Periodontology 69 (4), 470–478. doi:10.1902/jop.1998.69.4.470
Seciu, A.-M., Craciunescu, O., Stanciuc, A.-M., and Zarnescu, O. (2019). Tailored Biomaterials for Therapeutic Strategies Applied in Periodontal Tissue Engineering. Stem cells Dev. 28 (15), 963–973. doi:10.1089/scd.2019.0016
Seifi, M., and Ghoraishian, S. (2012). Determination of Orthodontic Tooth Movement and Tissue Reaction Following Demineralized Freeze-Dried Bone Allograft Grafting Intervention. Dent. Res. J. 9 (2), 203–208. doi:10.4103/1735-3327.95237
Sharif, F., Ur Rehman, I., Muhammad, N., and MacNeil, S. (2016). Dental Materials for Cleft Palate Repair. Mater. Sci. Eng. C 61, 1018–1028. doi:10.1016/j.msec.2015.12.019
Sheikh, Z., Hamdan, N., Ikeda, Y., Grynpas, M., Ganss, B., and Glogauer, M. (2017). Natural Graft Tissues and Synthetic Biomaterials for Periodontal and Alveolar Bone Reconstructive Applications: a Review. Biomater. Res. 21, 9. doi:10.1186/s40824-017-0095-5
Shoreibah, E. A., Ibrahim, S. A., Attia, M. S., and Diab, M. M. (2012). Clinical and Radiographic Evaluation of Bone Grafting in Corticotomy-Facilitated Orthodontics in Adults. J. Int. Acad. Periodontol. 14 (4), 105–113.
Shue, L., Yufeng, Z., and Mony, U. (2012). Biomaterials for Periodontal Regeneration. Biomatter 2 (4), 271–277. doi:10.4161/biom.22948
Spagnuolo, G., Codispoti, B., Marrelli, M., Rengo, C., Rengo, S., and Tatullo, M. (2018). Commitment of Oral-Derived Stem Cells in Dental and Maxillofacial Applications. Dent. J. 6 (4), 72. doi:10.3390/dj6040072
Strioga, M., Viswanathan, S., Darinskas, A., Slaby, O., and Michalek, J. (2012). Same or Not the Same? Comparison of Adipose Tissue-Derived versus Bone Marrow-Derived Mesenchymal Stem and Stromal Cells. Stem Cells Dev. 21 (14), 2724–2752. doi:10.1089/scd.2011.0722
Surmeli Baran, S., Temmerman, A., Salimov, F., Ucak Turer, O., Sapmaz, T., Haytac, M. C., et al. (2021). The Effects of Photobiomodulation on Leukocyte and Platelet-Rich Fibrin as Barrier Membrane on Bone Regeneration: An Experimental Animal Study. Photobiomodulation, Photomed. Laser Surg. 39 (4), 245–253. doi:10.1089/photob.2020.4943
Tanikawa, D. Y. S., Pinheiro, C. C. G., Almeida, M. C. A., Oliveira, C. R. G. C. M., Coudry, R. d. A., Rocha, D. L., et al. (2020). Deciduous Dental Pulp Stem Cells for Maxillary Alveolar Reconstruction in Cleft Lip and Palate Patients. Stem Cells Int. 2020, 1–9. doi:10.1155/2020/6234167
Tanimoto, K., Sumi, K., Yoshioka, M., Oki, N., Tanne, Y., Awada, T., et al. (2015). Experimental Tooth Movement into New Bone Area Regenerated by Use of Bone Marrow-Derived Mesenchymal Stem Cells. Cleft Palate-Craniofacial J. 52 (4), 386–394. doi:10.1597/12-232
Tehranchi, A., Behnia, H., Pourdanesh, F., Behnia, P., Pinto, N., and Younessian, F. (2018). The Effect of Autologous Leukocyte Platelet Rich Fibrin on the Rate of Orthodontic Tooth Movement: A Prospective Randomized Clinical Trial. Eur. J. Dent. 12 (3), 350–357. doi:10.4103/ejd.ejd_424_17
Telatar, B. C., and Gungor, A. Y. (2021). Effectiveness of Vibrational Forces on Orthodontic Treatment. J. Orofac. Orthop. 82 (5), 288–294. doi:10.1007/s00056-020-00257-z
Tian, H., Zhou, T., Chen, H., Li, C., Jiang, Z., Lao, L., et al. (2019). Bone Morphogenetic Protein‐2 Promotes Osteosarcoma Growth by Promoting Epithelial‐mesenchymal Transition (EMT) through the Wnt/β‐catenin Signaling Pathway. J. Orthop. Res. 37 (7), 1638–1648. doi:10.1002/jor.24244
Wang, J., Jiao, D., Huang, X., and Bai, Y. (2021). Osteoclastic Effects of mBMMSCs under Compressive Pressure during Orthodontic Tooth Movement. Stem Cell. Res. Ther. 12 (1), 148. doi:10.1186/s13287-021-02220-0
Wang, Y., Li, J., Zhou, J., Qiu, Y., and Song, J. (2022). Low-intensity Pulsed Ultrasound Enhances Bone Marrow-Derived Stem Cells-Based Periodontal Regenerative Therapies. Ultrasonics 121, 106678. doi:10.1016/j.ultras.2021.106678
Wang, Y., Qiu, Y., Li, J., Zhao, C., and Song, J. (2018). Low-intensity Pulsed Ultrasound Promotes Alveolar Bone Regeneration in a Periodontal Injury Model. Ultrasonics 90, 166–172. doi:10.1016/j.ultras.2018.06.015
Wilcko, M. T., Wilcko, W. M., and Bissada, N. F. (2008). An Evidence-Based Analysis of Periodontally Accelerated Orthodontic and Osteogenic Techniques: A Synthesis of Scientific Perspectives. Seminars Orthod. 14 (4), 305–316. doi:10.1053/j.sodo.2008.07.007
Yavagal, C. M., Matondkar, S. P., and Yavagal, P. C. (2021). Efficacy of Laser Photobiomodulation in Accelerating Orthodontic Tooth Movement in Children: A Systematic Review with Meta-Analysis. Int. J. Clin. Pediatr. Dent. 14 (Suppl. 1), S91–S97. doi:10.5005/jp-journals-10005-1964
Keywords: orthodontic tooth movement, alveolar bone graft, novel material, BMP-2, platelet-rich fibrin (PRF), bioactive glass, stem cell
Citation: Miao Y, Chang Y-C, Tanna N, Almer N, Chung C-H, Zou M, Zheng Z and Li C (2022) Impact of Frontier Development of Alveolar Bone Grafting on Orthodontic Tooth Movement. Front. Bioeng. Biotechnol. 10:869191. doi: 10.3389/fbioe.2022.869191
Received: 04 February 2022; Accepted: 13 June 2022;
Published: 30 June 2022.
Edited by:
Mohammad Khursheed Alam, Al Jouf University, Saudi ArabiaReviewed by:
Tarek El-Bialy, University of Alberta, CanadaCopyright © 2022 Miao, Chang, Tanna, Almer, Chung, Zou, Zheng and Li. This is an open-access article distributed under the terms of the Creative Commons Attribution License (CC BY). The use, distribution or reproduction in other forums is permitted, provided the original author(s) and the copyright owner(s) are credited and that the original publication in this journal is cited, in accordance with accepted academic practice. No use, distribution or reproduction is permitted which does not comply with these terms.
*Correspondence: Zhong Zheng, enpoZW5nQGRlbnRpc3RyeS51Y2xhLmVkdQ==; Chenshuang Li, bGljaGVuc0B1cGVubi5lZHU=
†These two authors have contributed equally to this work and share the first authorship