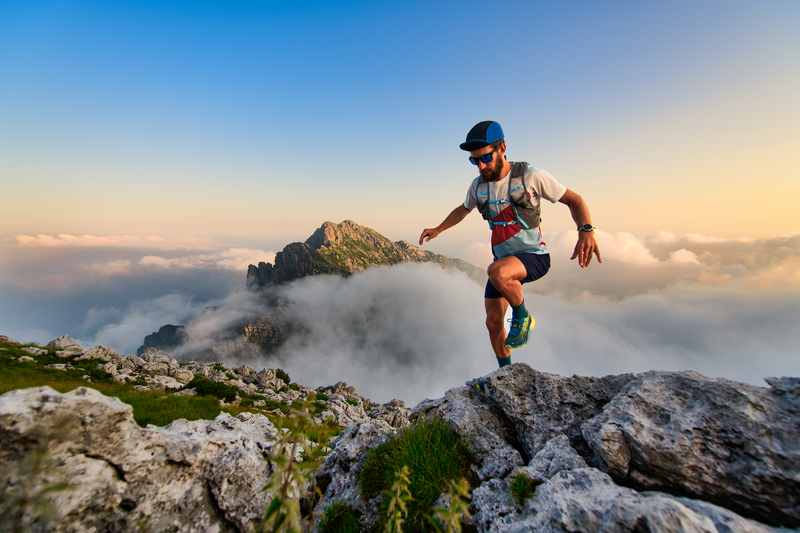
94% of researchers rate our articles as excellent or good
Learn more about the work of our research integrity team to safeguard the quality of each article we publish.
Find out more
ORIGINAL RESEARCH article
Front. Bioeng. Biotechnol. , 11 February 2022
Sec. Bioprocess Engineering
Volume 10 - 2022 | https://doi.org/10.3389/fbioe.2022.849631
This article is part of the Research Topic Wastewater Treatment & Resource Recovery Technologies View all 7 articles
Heavy metal contamination not only causes threat to human health but also raises sustainable development concerns. The use of traditional methods to remediate heavy metal contamination is however time-consuming, and the remediation efficiency may not meet the requirements as expected. The present study conducted a series of test tube experiments to investigate the effect of calcium source on the lead and copper removals. In addition to the test tube experiments, numerical simulations were performed using Visual MINTEQ software package considering different degrees of urea hydrolysis derived from the experiments. The remediation efficiency degrades when NH4+ and OH− concentrations are not sufficient to precipitate the majority of Pb2+ and Cu2+. It also degrades when CaO turns pH into highly alkaline conditions. The numerical simulations do not take the dissolution of precipitation into account and therefore overestimate the remediation efficiency when subjected to lower Pb(NO3)2 or Cu(NO3)2 concentrations. The findings highlight the potential of applying the enzyme-induced carbonate precipitation to lead and copper remediations.
Heavy metal contamination not only raises sustainable concerns but also causes threats to human health and plants (Kumari et al., 2016; Jiang et al., 2019; Rahman et al., 2020; Chen et al., 2021a; Tan et al., 2022). In the past few decades, remediation methods including replacement, soil flushing, electrokinetic remediation, chemical precipitation, ion exchange, and phytoremediation were developed (Mena et al., 2016; Kumari et al., 2017; Kumari et al., 2018; Chen and Achal, 2019; Duarte-Nass et al., 2020; Ahenkorah et al., 2021; Hu L. et al., 2021; Jiang et al., 2021). However, they are usually time-consuming and may cause secondary pollution (Achal et al., 2012; Nancharaiah et al., 2016; Kappaun et al., 2018; Xue et al., 2022; Yan et al., 2021; Duan et al., 2021a; Cheng et al., 2021; Duan et al., 2021b). Furthermore, heavy metal ions may be re-released into surrounding environments when subjected to the change in environmental conditions (Hu et al., 2021b; Bai et al., 2017; Bai et al., 2021; Xue et al., 2021). In light of this, a sustainable countermeasure that is able to immobilize heavy metal ions using an environmental-friendly manner is considered of great necessity. Enzyme-induced carbonate precipitation (EICP) is a novel biogeotechnical technique and has been widely applied to strengthen calcareous sands (Ahenkorah et al., 2021; Wei et al., 2021; Yuan et al., 2021; Chen et al., 2021b). Notwithstanding that, the application of the EICP method to the remediation of heavy metals is rarely reported. Urease enzyme can be derived from animals and plants. The principle of applying EICP technology to heavy metal remediation is to catalyze urea hydrolysis by the urease enzyme toward discharging ammonia and carbonate ions (NH4+ and CO32-). By CO32- and Ca2+, carbonates are formed and precipitated with heavy metal ions (also referred to as immobilization of heavy metal) where Ca2+ ions are derived from the addition of calcium source (Mugwar and Harbottle, 2016). The biochemical reactions that count on urea hydrolysis are shown in Eqs (1–3) (Mobley and Hausinger, 1989; Bai et al., 2019; Bai et al., 2020).
In recent years, EICP technology is also developed as a remediation method for immobilizing inorganic contaminates. Gowthaman et al. (2021) used urease enzyme combined with bone meal to form calcium phosphate biocementation toward decreasing toxic gaseous ammonia by approximately 90%. Furthermore, Moghal et al. (2020) introduced the EICP method that incorporates citric acid (C6H8O7) and ethylene diamine tetra-acetic acid (EDTA) to study the desorption response of heavy metal ions to the soil. Although the literature immobilizes contaminants by EICP technology solely or by a combination of EICP technology with other additions, studies on the mechanisms affecting the remediation efficiency are remarkably limited (Achal and Mukherjee, 2015; Li et al., 2015; Kang and So, 2016). The objectives of this study are 1) to investigate the response of urease activity to high Pb2+ and Cu2+ concentrations by performing a series of test tube experiments, 2) to investigate the effect of calcium source on the speciation of carbonate precipitation, and 3) to reveal the mechanisms affecting the remediation efficiency for Pb and Cu removals.
Canavalia ensiformis is a known plant to be rich in urease enzyme (Yuan et al., 2020). In the present work, urease enzyme was extracted from Canavalia ensiformis. Canavalia ensiformis was first ground using a grinding machine and then sieved using a sieve with 150-μm mesh opening. The solution containing jack bean and 30% ethanol was centrifuged for 30 min and then stored at 4°C for 4 h. After that, the supernatant was second centrifuged for 1 h and then stored at −20°C for future use.
At a temperature of 30°C, 1 international unit (IU) of enzyme activity corresponds to the amount of 1.0 μmol catalyzed transformation in 1 min (Mobley and Hausinger, 1989). In this study, the amount of product (i.e., ammonium ion) catalyzed by urease was measured by Nessler’s reagent colorimetric method. Nessler’s reagent and potassium sodium tartrate were added, and the absorbance was measured by using a spectrophotometer after waiting for 10 min. The calibration line was set up by the same method prior to the measurement, and the measured absorbance was then substituted to the calibration line to get the concentration of ammonium ions. The urease activity being 342.7 U/g was therefore measured in the present work, which is classed as low activity.
To investigate the effect of calcium source on copper and lead remediations, a series of test tube experiments were conducted in this study. Three calcium sources, namely, calcium chloride (CaCl2), calcium acetate (Ca(CH3COO)2), and calcium oxide (CaO) were adopted in these test tube experiments to investigate heavy metal (lead nitrate (Pb(NO3)2) and copper nitrate (Cu(NO3)2)) remediations. A solution applied to the test tube experiments consisted of distilled water, urea, Pb(NO3)2 or Cu(NO3)2, calcium source, and urease enzyme. The concentration of calcium source used in the test tube experiments was 0.25 M, while the urea concentration was 0.5 M. This concentration range was set to produce enough carbonate to precipitate calcium ions. Heavy metal concentrations adopted included both the low and high values of 5 mM, 10 mM, 30 mM, 40 mM, and 50 mM, respectively. The preparation of the solution is demonstrated in Figure 1. Measurements of pH and NH4+ concentration, precipitation mass, and remaining heavy metal ion concentration were performed not only to evaluate the urease activity but also for the final assessment of the remediation efficiency. All of the test tube experiments have three replicates. An error bar has already been added to figures where necessary. Statistical analysis indicates that the coefficient of variance for the test tube experiments is below 10%, which is within the requirement being the coefficient of variance of 15% for usual, accessible experimental measurements.
In addition to the test tube experiments, numerical simulations were performed using Visual MINTEQ software package. The numerical simulations, in fact, refer to the concentration of ions (e.g., NH4+ and CO32-) measured right after the test tube experiments, rather than referring to biochemical processes involved in catalyzing urea hydrolysis. In addition, their stoichiometric ratio sticks to 2:1 (Gat et al., 2017). The concentration of calcium source applied to the simulations was 0.25 M, while NH4+ concentrations (corresponding to the degree of urea hydrolysis) varying in a 10–200 mM range was adopted. The concentration of Pb2+ or Cu2+ fell in a 5–100 mM range. Given that the degree of urea hydrolysis may affect heavy metal removal, the change in NH4+ concentration benefits us to see how the speciation of carbonate precipitation varies with the degree of urea hydrolysis.
Urease has been widely applied to catalyzing urea hydrolysis. Ammonium ions (NH4+) and hydroxide ions (OH−) are known to be discharged after urea hydrolysis (see Eqs 1–3), and therefore, NH4+ and pH are considered as the key indicators of describing the degree of urea hydrolysis (Fisher et al., 2017; Sun et al., 2021). In biomineralization, attending higher degrees of urea hydrolysis is considered of great necessity to improve heavy metal remediation efficiency. The relationships of NH4+ and pH vs heavy metal ion concentration under three calcium sources, CaCl2, Ca(CH3COO)2, and CaO, respectively, are shown in Figure 2. Under CaCl2, NH4+ concentration remaining high (approximately 70 mM) is noted when lower Pb(NO3)2 concentrations (i.e., 5-10 mM) are confronted (see Figure 2A). When subjected to higher Pb(NO3)2 concentrations (i.e., 30-50 mM), there appears a decline in NH4+ concentration. The higher the concentration of Pb2+, the more significant will be the effect on the urease activity and the lower will be the degree of urea hydrolysis. pH remaining in a 7–8 range may be appropriate in securing the urease activity toward elevating the degree of urea hydrolysis (see Figure 2B). The highest NH4+ concentration being about 105 mM is attained under Ca(CH3COO)2, corresponding to the highest degree of urea hydrolysis (see Figure 2C). The urease activity, associated with Ca(CH3COO)2, remains higher than that under CaCl2 when pH is falling in the 6–7 range. NH4+ concentration under CaO approaches 0, indicating the absence of urea hydrolysis. Provided there appears remediation efficiency, its attendance relies upon chemical precipitation that is not relevant to the “biomineralization” but to the reaction between Ca2+ and OH− (Eq. 4).
FIGURE 2. Ammonium ion concentration and pH vs heavy metal concentration: (A) NH4+ concentration, (B) CaCl2, (C) Ca(CH3COO)2, and (D) CaO.
Despite that, the chemical precipitation could depress the urease activity by strongly alkaline pH of surrounding conditions, degrading the degree of urea hydrolysis (Mobley and Hausinger, 1989; Schock et al., 1995; Ferris et al., 2004; Hu et al., 2021c) (see Figure 2D). On the other hand, NH4+ concentration under any of the calcium sources always approaches 0 for Cu remediation (see Figure 2A). This is to say that the effect of Cu2+ on the urease activity is much higher than the effect of Pb2+, thereby reducing the degree of urea hydrolysis. To this end, chemical precipitation is supposed to be present here because of the absence of urea hydrolysis. pH higher than 9 is observed under CaO, while pH lower than 6 is noted under CaCl2 and Ca(CH3COO)2 (see Figures 2B–D). In short, the highest degree of urea hydrolysis presents under Ca(CH3COO)2, followed by CaCl2 and CaO, for the Pb remediation. However, the degree of urea hydrolysis is rather low under any of the calcium sources for the Cu remediation, indicating that there appears remediation efficiency, and chemical precipitation is present here.
This part primarily aims to link the effect of calcium source to the Pb remediation efficiency. The remediation efficiency is defined as follows:
where CI represents the initial heavy metal concentration and CR corresponds to the remaining heavy metal concentration. The lower the remaining heavy metal ion concentration, the higher will be the remediation efficiency. The relationships of the remaining Pb2+ concentration and the remediation efficiency vs Pb(NO3)2 concentration under CaCl2, Ca(CH3COO)2, and CaO, respectively, are shown in Figure 3. Under CaCl2, the remediation efficiency higher than 99% (the highest in the present work), with the remaining Pb2+ concentration way below 2 mM, is observed, although the precipitation mass is not the highest (see Figure 4). It can be inferred that given the second highest degree of urea hydrolysis, biotic precipitation may contribute to the attendance of the highest remediation efficiency. In addition to the biotic precipitation, the highest remediation efficiency could not be achieved without the participation of chemical precipitation. Considering that the chemical precipitation is present earlier than the biotic precipitation, the occupation of Pb2+ by the chemical precipitation first depresses the effect of Pb2+ on the urease activity, forming the biotic precipitation by catalyzing urea hydrolysis to assure the highest remediation efficiency.
FIGURE 3. Relationships of the remaining Pb2+ concentration and the remediation vs Pb(NO3)2 concentration under CaCl2, Ca(CH3COO)2, and CaO, respectively.
FIGURE 4. Relationships of the precipitation mass vs Pb(NO3)2 concentration under CaCl2, Ca(CH3COO)2, and CaO respectively.
Under Ca(CH3COO)2, the degree of urea hydrolysis is the highest, discharging more NH4+ and OH−. The remediation efficiency decreases with the increase in Pb(NO3)2 concentration. These results indicate that the chemical and biotic precipitations inhibit the effect of Pb2+ on the urease activity. However, the depression by the chemical and biotic precipitations turns out to be less significant when subjected to higher Pb(NO3)2 concentrations. It can also be observed from Figure 4 that the precipitation mass under Ca(CH3COO)2 can be compared to that under CaCl2 and is much below that under CaO. On the other hand, under CaO, the degree of urea hydrolysis is the lowest among the three calcium sources; therefore, urea hydrolysis and biotic precipitation that appear are not present here. For this reason, the chemical precipitation, Ca(OH)2, presents. Ca(OH)2 due to its higher solubility product then transfers to Pb(OH)2.
As discussed, strongly alkaline conditions (higher than 10) could depress urease activity and also raise the dissolution potential of chemical precipitation (see Figure 2D). This addresses the degradation in the remediation efficiency when subjected to lower Pb(NO3)2 concentrations. The role of Pb2+ by the chemical precipitations is not only to reduce their effect on the urease activity but also to become more significant when subjected to higher Pb(NO3)2 concentrations. The remediation efficiency increases with the increase in Pb(NO3)2 concentration, with the highest measured at about 95%. On the whole, for the Pb remediation, the highest remediation efficiency is attained using CaCl2, whereas the highest precipitation mass is attained using CaO. Furthermore, the Pb remediation efficiency decreases and increases with the increasing Pb(NO3)2 concentration under Ca(CH3COO)2 and CaO, respectively.
This part primarily targets to enhance our knowledge linkage between the effect of calcium source and Cu remediation. The relationships of the remaining Cu2+ concentration and the remediation efficiency vs Cu(NO3)2 concentration under CaCl2, Ca(CH3COO)2, and CaO, respectively are shown in Figure 5. NH4+ concentration is much below 15 mM under any of the calcium sources, which corresponds to a very low degree of urea hydrolysis (see Figure 2A). Despite that, the highest remediation efficiency is attained using CaO, followed by CaCl2 and Ca(CH3COO)2, most likely because of the formation of chemical precipitation (Shaw and Raval, 1961). The remediation efficiency beyond 90% is attained using CaCl2 when subjected to Cu(NO3)2 concentration at 5 mM, and it decreases with the increase in Cu(NO3)2 concentration. In contrast, under CaO, the remediation efficiency increases with the increasing Cu(NO3)2 concentration, with the maximum when subjected to Cu(NO3)2 concentration varying in a 30–50 mM range. On the other hand, when subjected to Cu(NO3)2 concentration at 5 mM, the remediation efficiency of up to 55% is attained using Ca(CH3COO)2, and it decreases with the increase in Cu(NO3)2 concentration. Similarly, the highest precipitation mass is still attained using CaO, followed by Ca(CH3COO)2 and CaCl2 (see Figure 6). On the whole, the effect of Cu2+ badly causes urease to lose its activity. This leads to the inability of catalyzing urea hydrolysis to form biotic precipitation. The highest remediation efficiency is attained using CaO. Considering the main cause leading to the difference in remediation efficiency under CaCl2, Ca(CH3COO)2, and CaO remains unclear, and it would be discussed later in this article.
FIGURE 5. Relationships of the remaining Cu2+ concentration and the remediation vs Cu(NO3)2 concentration under CaCl2, Ca(CH3COO)2, and CaO, respectively.
FIGURE 6. Relationships of the precipitation mass vs Cu(NO3)2 concentration under CaCl2, Ca(CH3COO)2, and CaO, respectively.
To reveal the speciation of precipitation, a series of simulations were conducted using Visual MINTEQ software package considering different degrees of urea hydrolysis (i.e., NH4+ concentration) derived from the test tube experiments. The three calcium sources applied to the experiments also participated in the simulations. The simulated results would give the speciation of precipitation and also benefit to revealing the mechanisms affecting the remediation efficiency. Considering CaCl2 as the calcium source, the relationships of the remediation efficiency vs NH4+ concentration and Pb(NO3)2 concentration are depicted in Figure 7A. There are five speciations, including cotunnite (PbCl2), laurionite (Pb(OH)Cl), phosgenite (Pb2Cl2CO3), hydrocerussite (Pb3(OH)2(CO3)2), and cerussite (PbCO3). Considering the discharge of CO32- and NH4+ only presents when there appears urea hydrolysis, associated precipitation is also referred to as the “biotic” precipitation. In contrast, precipitation not relevant to CO32- is termed as the “chemical” precipitation. To this end, the later three precipitations are categorized as the biotic precipitations, whereas the first two are classified as the chemical precipitations. PbCO3, Pb3(OH)2(CO3)2, and Pb2Cl2CO3 present when the degree of urea hydrolysis is high enough to precipitate the majority of Pb2+. In total, 1 mM CO32- for PbCO3 is needed to thoroughly precipitate 1 mM Pb2+, whereas for Pb3(OH)2(CO3)2, 2 mM CO32- is required to precipitate 3 mM Pb2+. When the degree of urea hydrolysis is low and cannot discharge enough CO32- and NH4+, Pb(OH)Cl and PbCl2 present. The chemical precipitation in some cases may not be as stable as the biotic precipitation because of its higher solubility product toward causing some difficulty in capsulizing heavy metal ions and raising the concern of their migration. This would eventually degrade the remediation efficiency (Edwards et al., 1992; Baltpurvins et al., 1996).
FIGURE 7. (A) Variations of the Pb remediation efficiency against different Pb2+ concentration ranges considering NH4+ concentrations varying in a 10–200 mM range, (B) variations of the Pb remediation efficiency against different Pb2+ concentration ranges, and (C) variations of pH of surrounding conditions against different Pb2+ concentration ranges (calcium source = CaCl2).
Figures 7B,C respectively show the variations in the remediation efficiency and pH against different Pb2+ concentration ranges considering NH4+ concentrations (i.e., degree of urea hydrolysis) varying in a 10–200 mM range. The remediation efficiency of approximately 100% (corresponding to 100 mM Pb2+) is attained using 200 mM NH4+ (see Figure 7B). In contrast, there appears a substantial reduction in the remediation efficiency to 75% (corresponding to 15 mM Pb2+) when Pb2+ and NH4+ concentrations are 20 and 10 mM, respectively. In total, 10 mM NH4+ corresponds to 5 mM CO32-, and 5 mM CO32- can only precipitate 10 mM Pb2+, in accordance with the speciation of Pb2Cl2CO3 (see Figure 7A). The remaining 5 mM Pb2+ is therefore precipitated by the chemical precipitation (i.e., PbCl2). The degradation in the remediation efficiency is due to the lack of CO32- and NH4+. There appears the other reduction to 90% (corresponding to 36 mM Pb2+) when Pb2+ and NH4+ concentrations are 40 and 30 mM, respectively. In total, 30 mM NH4+ corresponds to 15 mM CO32-. As a result, 15 mM CO32- can only precipitate 30 mM Pb2+, producing the biotic precipitation (i.e., Pb2Cl2CO3) (see Figure 7A). The chemical precipitation (i.e., PbCl2) is to precipitate the remaining 6 mM Pb2+. It is noteworthy that the degradations in the remediation efficiency take place when pH of surrounding conditions become below 6 (see Figure 7C). These results indicate that improving the remediation efficiency relies not only on the degree of urea hydrolysis but also on the pH of surrounding conditions (Krajewska, 2016). pH falling in a 6–9 range causes urease to possess hydrolytic activity (Krajewska, 2016). These also satisfactorily address the reduction in the degree of urea hydrolysis when subjected to Pb2+ concentrations higher than 30 mM and pH below 6 (see Figures 2A,B).
Considering Ca(CH3COO)2 as the calcium source, the relationships of the remediation efficiency vs NH4+ concentration and Pb(NO3)2 concentration are shown in Figure 8A. There are three speciations of precipitation, including cerussite (PbCO3), hydrocerussite (Pb3(OH)2(CO3)2), and lead hydroxide (Pb(OH)2), where the last is classified as the chemical precipitation, and the other two are classified as the biotic precipitation. The remediation efficiency of approximately 100% (corresponding to 100 mM Pb2+) is attained via 200 mM NH4+ (see Figure 8B). Similarly, there appears a reduction in the remediation efficiency when CO32- and NH4+ concentrations are not high enough. The degradations in the remediation efficiency initiate when pH stays away from 7 (see Figure 8C). They develop further when pH drops consistently.
FIGURE 8. (A) Variations of the Pb remediation efficiency against different Pb2+ concentration ranges considering NH4+ concentrations varying in a 10–200 mM range, (B) variations of the Pb remediation efficiency against different Pb2+ concentration ranges, and (C) variations of pH of surrounding conditions against different Pb2+ concentration ranges (calcium source = Ca(CH3COO)2).
When CaO is considered as the calcium source, the relationships of the remediation efficiency vs NH4+ concentration and Pb(NO3)2 concentration are shown in Figure 9A. The precipitation corresponds to one single speciation, namely, Pb(OH)2. Pb(OH)2 is categorized as the chemical precipitation. The remediation efficiency as high as 100% is attained irrespective of NH4+ concentration, indicating the absence of urea hydrolysis (see Figure 9B). As discussed, Ca(OH)2 precipitates earlier than Pb(OH)2, and the former turns pH into strongly alkaline conditions, causing the dissolution of precipitation (see Figure 9C). The dissolution of precipitation, in turn, degrades the remediation efficiency when subjected to lower Pb(NO3)2 concentrations (see Figure 3). pH turns however into weakly alkaline conditions when subjected to higher Pb(NO3)2 concentrations. This tackles difficulty in catalyzing urea hydrolysis under strongly alkaline conditions and therefore raises the remediation efficiency. The experimental results appear to conflict with the simulated ones, most likely due to the neglection of precipitation dissolution by the simulations. The remediation efficiency remaining very high under pH above 12 provides testimony of the aforesaid argument (see Figure 9C).
FIGURE 9. (A) Variations of the Pb remediation efficiency against different Pb2+ concentration ranges considering NH4+ concentrations varying in a 10–200 mM range, (B) variations of the Pb remediation efficiency against different Pb2+ concentration ranges, and (C) variations of pH of surrounding conditions against different Pb2+ concentration ranges (calcium source = CaO).
This part presents the simulated results applied to the Cu removal. Considering CaCl2 as the calcium source, the relationships of the Cu remediation efficiency vs NH4+ concentration and Cu(NO3)2 concentration are shown in Figure 10A. Three speciations of precipitation are identified. Azurite (Cu3(CO3)2(OH)2) and malachite (Cu2(OH)2CO3) are classified as the biotic precipitations, while atacamite (Cu2(OH)3Cl) is classified as the chemical precipitation. Furthermore, under Ca(CH3COO)2, Cu3(CO3)2(OH)2, tenorite (CuO), and Cu2(OH)2CO3 are recognized, as shown in Figure 11A. Moreover, using CaO as calcium source, single one speciation, namely, CuO, is identified, as shown in Figure 12A. It can be seen from Figures 10B,C, 11B,C that under CaCl2 and Ca(CH3COO)2, the lack of CO32- and NH4+ triggers a degradation in the remediation efficiency. In addition to the lack of CO32- and NH4+, the degradation in the remediation efficiency is also counted on the remediation efficiency. Under CaO, it dissolves in water to form Ca(OH)2, and Ca(OH)2 turns pH into highly alkaline conditions toward affecting the urease activity and eventually degrading the remediation efficiency (see Figures 12B,C). The degradation in the remediation efficiency is however absent here as the numerical simulation neglects the precipitation dissolution. To summarize, the effect of Cu2+ can more significantly reduce urease activity. The highest remediation efficiency is attained using CaO. Such high remediation efficiency still presents when subjected to lower Cu(NO3)2 concentrations, which is not in line with the experimental results. The neglection of precipitation dissolution by the simulations is deemed as the main cause leading to the discrepancy. The remediation efficiency degrades when CO32- and NH4+ concentrations are not high enough to precipitate the majority of Cu2+. In addition, the remediation efficiency also degrades when pH turns into highly alkaline conditions, induced by the addition of CaO.
FIGURE 10. (A) Variations of the Cu remediation efficiency against different Cu2+ concentration ranges considering NH4+ concentrations varying in a 10–200 mM range, (B) variations of the Cu remediation efficiency against different Cu2+ concentration ranges, and (C) variations of pH of surrounding conditions against different Cu2+ concentration ranges (calcium source = CaCl2).
FIGURE 11. (A) Variations of the Cu remediation efficiency against different Cu2+ concentration ranges considering NH4+ concentrations varying in a 10–200 mM range, (B) variations of the Cu remediation efficiency against different Cu2+ concentration ranges, and (C) variations of pH of surrounding conditions against different Cu2+ concentration ranges (calcium source = Ca(CH3COO)2).
FIGURE 12. (A) Variations of the Cu remediation efficiency against different Cu2+ concentration ranges considering NH4+ concentrations varying in a 10–200 mM range, (B) variations of the Cu remediation efficiency against different Cu2+ concentration ranges, and (C) variations of pH of surrounding conditions against different Cu2+ concentration ranges (calcium source = CaO).
Heavy metal ions can significantly affect the urease activity and cause some difficulty in catalyzing urea hydrolysis and capsulizing heavy metal ions toward degrading the remediation efficiency. Urease can be capsulized by the addition of calcium sources, preventing loss of their activity. Under CaCl2 and Ca(CH3COO)2, NH4+ concentration for the Pb remediation remains as a high provide testimony of the aforesaid argument. As a result, the biotic precipitation can precipitate the majority of Pb2+ toward securing the remediation efficiency. However, under CaO, NH4+ concentration is approximately 5 mM, which also indicates the absence of urea hydrolysis. In this case, the remediation efficiency relies upon the chemical precipitation. The degradation in the remediation efficiency presents when subjected to lower Pb(NO3)2 concentrations. Ca(OH)2 turning pH into highly alkaline conditions is considered as the leading cause resulting in the degradation of the remediation efficiency. Therefore, the degradation in the remediation efficiency not only relies on the provision of NH4+ and OH− but also on the change in pH, induced by the addition of calcium source. NH4+ concentration for the Cu remediation is measured as low as approximately 5 mM, irrespective of calcium source. As a result, the remediation efficiency is attained not only by the biotic precipitation but also by the chemical precipitation. It is worth noting that under CaO, the reduction as appeared when subjected to lower Cu(NO3)2 concentrations in the test tube experiments is absent in the simulated results. The discrepancy is mainly attributed to the neglection of precipitation dissolution under highly alkaline conditions. To conclude, ensuring the degree of urea hydrolysis is deemed of great necessity when applying the enzyme-induced carbonate precipitation technology to heavy metal remediation. There are two leading causes affecting the remediation efficiency: one is the provision of NH4+ and OH−, and the other is the change in pH, resulting from the addition of calcium source (see Figure 13). Here, NH4+ and OH− concentrations high enough can precipitate the majority of Pb2+ and Cu2+, corresponding to the biotic precipitation. When NH4+ and OH− concentrations are not high enough to precipitate the majority of Pb2+ and Cu2+, the remediation efficiency is attained through a combination of biotic and chemical precipitations. On the other hand, CaO dissolves in water to produce Ca(OH)2 toward turning pH into highly alkaline conditions. Such alkaline conditions could not only depress the urease activity but also cause dissolution of the carbonate precipitation, thereby degrading the remediation efficiency. The degradation is absent in the simulated results because the simulations do not take the dissolution of carbonate precipitation into account.
The test tube experiments were conducted to reproduce catalyzing urea hydrolysis using urease enzyme. The experimental and numerical results were applied to investigate the effect of calcium source on Pb and Cu removals. Based on the results and discussions, some main conclusions can be drawn as follows:
1) The remediation efficiency degrades when NH4+ and OH− concentrations are not sufficient to precipitate the majority of Pb2+ and Cu2+. The remediation efficiency due to the lack of Pb2+ and Cu2+ is attained through a combination of the biotic and chemical precipitations.
2) Highly alkaline conditions could not only affect the urease activity but also cause dissolution of carbonate precipitation. CaO dissolves in water to produce Ca(OH)2, turning pH more alkaline, and degrading the remediation efficiency.
3) The numerical simulations are deemed useful in enhancing our knowledge linkage between the speciation of carbonate precipitation and the remediation efficiency and preventing unfavorable pH of surrounding conditions from affecting the degree of urea hydrolysis. Notwithstanding that, the simulations do not take the dissolution of precipitation into account and therefore overestimate the remediation efficiency when subjected to lower Pb(NO3)2 or Cu(NO3)2 concentrations.
The original contributions presented in the study are included in the article/Supplementary Material, further inquiries can be directed to the corresponding author.
LW: data curation, formal analysis, validation, software, and writing–original draft. W-CC: conceptualization, methodology, writing–review and editing, supervision, and funding acquisition. Z-FX: writing–review and editing.
This paper is based upon work supported by the Shaanxi Educational Department under Grant No. 2020TD-005 through the innovative ability support scheme.
The authors declare that the research was conducted in the absence of any commercial or financial relationships that could be construed as a potential conflict of interest.
All claims expressed in this article are solely those of the authors and do not necessarily represent those of their affiliated organizations, or those of the publisher, the editors, and the reviewers. Any product that may be evaluated in this article, or claim that may be made by its manufacturer, is not guaranteed or endorsed by the publisher.
Achal, V., and Mukherjee, A. (2015). A Review of Microbial Precipitation for Sustainable Construction. Construction Building Mater. 93, 1224–1235. doi:10.1016/j.conbuildmat.2015.04.051
Achal, V., Pan, X., Fu, Q., and Zhang, D. (2012). Biomineralization Based Remediation of As(III) Contaminated Soil by Sporosarcina Ginsengisoli. J. Hazard. Mater. 201-202, 178–184. doi:10.1016/j.jhazmat.2011.11.067
Ahenkorah, I., Rahman, M. M., Karim, M. R., Beecham, S., and Saint, C. (2021). A Review of Enzyme Induced Carbonate Precipitation (EICP): The Role of Enzyme Kinetics. Sustain. Chem. 2 (1), 92–114. doi:10.3390/suschem2010007
Bai, B., Long, F., Rao, D. Y., and Xu, T. (2017). The Effect of Temperature on the Seepage Transport of Suspended Particles in a Porous Medium. Hydrol Process 31 (2), 382–393. doi:10.1002/hyp.11034
Bai, B., Rao, D. Y., Chang, T., Chang, T., and Guo, Z. G. (2019). A Nonlinear Attachment-Detachment Model with Adsorption Hysteresis for Suspension-Colloidal Transport in Porous Media. J. Hydrol. 578, 124080. doi:10.1016/j.jhydrol.2019.124080
Bai, B., Xu, T., Nie, Q. K., and Li, P. P. (2020). Temperature-Driven Migration of Heavy Metal Pb2+ Along with Moisture Movement in Unsaturated Soils. Int. J. Heat Mass Transf. 578, 119573. doi:10.1016/j.ijheatmasstransfer.2020.119573
Bai, B., Nie, Q. K., Zhang, Y. K., Wang, X. L., and Hu, W. (2021). Cotransport of Heavy Metals and SiO2 Particles at Different Temperatures by Seepage. J. Hydrol. 597, 125771. doi:10.1016/j.jhydrol.2020.125771
Baltpurvins, K. A., Burns, R. C., Lawrance, G. A., and Stuart, A. D. (1996). Use of the Solubility Domain Approach for the Modeling of the Hydroxide Precipitation of Heavy Metals from Wastewater. Environ. Sci. Technol. 30 (5), 1493–1499. doi:10.1021/es950421u
Chen, M., Li, Y., Jiang, X., Zhao, D., Liu, X., Zhou, J., et al. (2021a). Study on Soil Physical Structure after the Bioremediation of Pb Pollution Using Microbial-Induced Carbonate Precipitation Methodology. J. Hazard. Mater. 411, 125103. doi:10.1016/j.jhazmat.2021.125103
Chen, W. W., Shao, Z. S., and Wei, W. (2021b). Experimental Study of the Heating Potential of Mortar-Aggregate under Microwave Irradiation. J. Mater. Civ. Eng. 33 (7), 04021153. doi:10.1061/(ASCE)MT.1943-5533.0003782
Chen, X., and Achal, V. (2019). Biostimulation of Carbonate Precipitation Process in Soil for Copper Immobilization. J. Hazard. Mater. 368, 705–713. doi:10.1016/j.jhazmat.2019.01.108
Cheng, W. C., Duan, Z., Xue, Z. F., and Wang, L. (2021). Sandbox Modelling of Interactions of Landslide Deposit with Terrace Sediments Aided by Field Observation. Bull. Eng. Geol. Environ. 80 (21), 3711–3731. doi:10.1007/s10064-021-02144-2
Duan, Z., Yan, X. S., Sun, Q., Tan, X., and Dong, C. X. (2021a). Effects of Water Content and Salt Content on Electrical Resistivity of Loess. Environmental Earth Sciences 80, 469. doi:10.1007/s12665-021-09769-2
Duan, Z., Cheng, W. C., Peng, J. B., and Tang, H. (2021b). Interactions of Landslide Deposit with Terrace Sediments: Perspectives from Velocity of Deposit Movement and Apparent Friction Angle. Engineering Geology 280, 105913. doi:10.1016/j.enggeo.2020.105913
Duarte-Nass, C., Rebolledo, K., Valenzuela, T., Kopp, M., Jeison, D., Rivas, M., et al. (2020). Application of Microbe-Induced Carbonate Precipitation for Copper Removal from Copper-Enriched Waters: Challenges to Future Industrial Application. J. Environ. Manage. 256, 109938. doi:10.1016/j.jenvman.2019.109938
Edwards, R., Gillard, R. D., Williams, P. A., and Pollard, A. M. (1992). Studies of Secondary mineral Formation in the PbO-H2o-HC1 System. Mineral. Mag. 56 (382), 53–65. doi:10.1180/minmag.1992.056.382.07
Ferris, F. G., Phoenix, V., Fujita, Y., and Smith, R. W. (2004). Kinetics of Calcite Precipitation Induced by Ureolytic Bacteria at 10 to 20°C in Artificial Groundwater. Geochimica et Cosmochimica Acta 68 (8), 1701–1710. doi:10.1016/S0016-7037(03)00503-9
Fisher, K. A., Yarwood, S. A., and James, B. R. (2017). Soil Urease Activity and Bacterial ureC Gene Copy Numbers: Effect of pH. Geoderma 285, 1–8. doi:10.1016/j.geoderma.2016.09.012
Gat, D., Ronen, Z., and Tsesarsky, M. (2017). Long-term Sustainability of Microbial-Induced CaCO3 Precipitation in Aqueous media. Chemosphere 184, 524–531. doi:10.1016/j.chemosphere.2017.06.015
Gowthaman, S., Yamamoto, M., Nakashima, K., Ivanov, V., and Kawasaki, S. (2021). Calcium Phosphate Biocement Using Bone Meal and Acid Urease: An Eco-Friendly Approach for Soil Improvement. J. Clean. Prod. 319, 128782. doi:10.1016/j.jclepro.2021.128782
Hu, L., Wang, H., Xu, P., and Zhang, Y. (2021a). Biomineralization of Hypersaline Produced Water Using Microbially Induced Calcite Precipitation. Water Res. 190, 116753. doi:10.1016/j.watres.2020.116753
Hu, W., Cheng, W.-C., Wen, S., and Mizanur Rahman, M. (2021b). Effects of Chemical Contamination on Microscale Structural Characteristics of Intact Loess and Resultant Macroscale Mechanical Properties. Catena 203, 105361. doi:10.1016/j.catena.2021.105361
Hu, W., Cheng, W.-C., Wen, S., and Yuan, K. (2021c). Revealing the Enhancement and Degradation Mechanisms Affecting the Performance of Carbonate Precipitation in EICP Process. Front. Bioeng. Biotechnol. 9, 750258. doi:10.3389/fbioe.2021.750258
Jiang, N.-J., Liu, R., Du, Y.-J., and Bi, Y.-Z. (2019). Microbial Induced Carbonate Precipitation for Immobilizing Pb Contaminants: Toxic Effects on Bacterial Activity and Immobilization Efficiency. Sci. Total Environ. 672, 722–731. doi:10.1016/j.scitotenv.2019.03.294
Jiang, N. J., Wang, Y. J., Chu, J., Kawasaki, S., Tang, C. S., Cheng, L., et al. (2021). Bio‐mediated Soil Improvement: An Introspection into Processes, Materials, Characterization and Applications. Soil Use Manage. 38, 68–93. doi:10.1111/sum.12736
Kang, C.-H., and So, J.-S. (2016). Heavy Metal and Antibiotic Resistance of Ureolytic Bacteria and Their Immobilization of Heavy Metals. Ecol. Eng. 97, 304–312. doi:10.1016/j.ecoleng.2016.10.016
Kappaun, K., Piovesan, A. R., Carlini, C. R., and Ligabue-braun, R. (2018). Ureases: Historical Aspects, Catalytic, and Non-catalytic Properties - a Review. J. Adv. Res. 13, 3–17. doi:10.1016/j.jare.2018.05.010
Krajewska, B. (2016). A Combined Temperature-pH Study of Urease Kinetics. Assigning pKa Values to Ionizable Groups of the Active Site Involved in the Catalytic Reaction. J. Mol. Catal. B: Enzymatic 124, 70–76. doi:10.1016/j.molcatb.2015.11.021
Kumari, D., Qian, X.-Y., Pan, X., Achal, V., Li, Q., and Gadd, G. M. (2016). Microbially-induced Carbonate Precipitation for Immobilization of Toxic Metals. Adv. Appl. Microbiol. 94, 79–108. doi:10.1016/bs.aambs.2015.12.002
Kumari, P., Panda, P. K., Jha, E., Kumari, K., Nisha, K., Mallick, M. A., et al. (2017). Mechanistic Insight to ROS and Apoptosis Regulated Cytotoxicity Inferred by Green Synthesized CuO Nanoparticles from Calotropis Gigantea to Embryonic Zebrafish. Sci. Rep. 7 (1), 16284. doi:10.1038/s41598-017-16581-1
Kumari, P., Panda, P. K., Jha, E., Pramanik, N., Nisha, K., Kumari, K., et al. (2018). Molecular Insight to In Vitro Biocompatibility of Phytofabricated Copper Oxide Nanoparticles with Human Embryonic Kidney Cells. Nanomedicine 13 (19), 2415–2433. doi:10.2217/nnm-2018-0175
Li, M., Fu, Q.-L., Zhang, Q., Achal, V., and Kawasaki, S. (2015). Bio-grout Based on Microbially Induced Sand Solidification by Means of Asparaginase Activity. Sci. Rep. 5, 16128. doi:10.1038/srep16128
Mena, E., Villaseñor, J., Rodrigo, M. A., and Cañizares, P. (2016). Electrokinetic Remediation of Soil Polluted with Insoluble Organics Using Biological Permeable Reactive Barriers: Effect of Periodic Polarity Reversal and Voltage Gradient. Chem. Eng. J. 299, 30–36. doi:10.1016/j.cej.2016.04.049
Mobley, H. L., and Hausinger, R. P. (1989). Microbial Ureases: Significance, Regulation, and Molecular Characterization. Microbiol. Rev. 53 (1), 85–108. doi:10.1128/MR.53.1.85-108.1989
Moghal, A. A. B., Lateef, M. A., Abu Sayeed Mohammed, S., Ahmad, M., Usman, A. R. A., and Almajed, A. (2020). Heavy Metal Immobilization Studies and Enhancement in Geotechnical Properties of Cohesive Soils by EICP Technique. Appl. Sci. 10 (21), 7568. doi:10.3390/app10217568
Mugwar, A. J., and Harbottle, M. J. (2016). Toxicity Effects on Metal Sequestration by Microbially-Induced Carbonate Precipitation. J. Hazard. Mater. 314, 237–248. doi:10.1016/j.jhazmat.2016.04.039
Nancharaiah, Y. V., Mohan, S. V., and Lens, P. N. L. (2016). Biological and Bioelectrochemical Recovery of Critical and Scarce Metals. Trends Biotechnol. 34 (2), 137–155. doi:10.1016/j.tibtech.2015.11.003
Rahman, M. M., Hora, R. N., Ahenkorah, I., Beecham, S., Karim, M. R., and Iqbal, A. (2020). State-of-the-art Review of Microbial-Induced Calcite Precipitation and its Sustainability in Engineering Applications. Sustainability 12 (15), 6281. doi:10.3390/su12156281
Schock, M. R., Lytle, D. A., and Clement, J. A. (1995). Effect of pH, DIC, Orthophosphate and Sulfate on Drinking Water Cuprosolvency. Washington DC: U.S. Environmental Protection Agency.
Shaw, W. H. R., and Raval, D. N. (1961). The Inhibition of Urease by Metal Ions at pH 8.9. J. Am. Chem. Soc. 83 (15), 3184–3187. doi:10.1021/ja01476a004
Sun, X., Miao, L., Wang, H., Yuan, J., and Fan, G. (2021). Enhanced Rainfall Erosion Durability of Enzymatically Induced Carbonate Precipitation for Dust Control. Sci. Total Environ. 791, 148369. doi:10.1016/j.scitotenv.2021.148369
Tan, J., Yi, H., Zhang, Z., Meng, D., Li, Y., Xia, L., et al. (2022). Montmorillonite Facilitated Pb(II) Biomineralization by Chlorella Sorokiniana FK in Soil. J. Hazard. Mater. 423, 127007. doi:10.1016/j.jhazmat.2021.127007
Wei, W., Shao, Z. S., Qiao, R. J., Chen, W. W., Zhang, P. J., and Cheng, J. X. (2021). Workability and Mechanical Properties of Microwave Heating for Recovering High Quality Aggregate from Concrete. Constr. Build. Mater. 276, 122237. doi:10.1016/j.conbuildmat.2020.122237
Xue, Z.-F., Cheng, W.-C., Wang, L., and Hu, W. (2022). Effects of Bacterial Inoculation and Calcium Source on Microbial-Induced Carbonate Precipitation for lead Remediation. J. Hazard. Mater. 426, 128090. doi:10.1016/j.jhazmat.2021.128090
Xue, X. F., Cheng, W. C., Wang, L., and Song, G. Y. (2021). Improvement of the Shearing Behaviour of Loess Using Recycled Straw Fiber Reinforcement. KSCE J. Civ. Eng. 25 (9), 3319–3335. doi:10.1007/s12205-021-2263-3
Yan, X. S., Duan, Z., and Sun, Q. (2021). Influences of Water and Salt Contents on the Thermal Conductivity of Loess. Environmental Earth Sciences 80, 52. doi:10.1007/s12665-020-09335-2
Yuan, H., Ren, G., Liu, K., Zheng, W., and Zhao, Z. (2020). Experimental Study of EICP Combined with Organic Materials for silt Improvement in the Yellow River Flood Area. Appl. Sci. 10 (21), 7678. doi:10.3390/app10217678
Keywords: heavy metal, biomineralization, urease enzyme, urea hydrolysis, remediation efficiency
Citation: Wang L, Cheng W-C and Xue Z-F (2022) The Effect of Calcium Source on Pb and Cu Remediation Using Enzyme-Induced Carbonate Precipitation. Front. Bioeng. Biotechnol. 10:849631. doi: 10.3389/fbioe.2022.849631
Received: 06 January 2022; Accepted: 24 January 2022;
Published: 11 February 2022.
Edited by:
Eduardo Jacob-Lopes, Federal University of Santa Maria, BrazilReviewed by:
Suresh K. Verma, Uppsala University, SwedenCopyright © 2022 Wang, Cheng and Xue. This is an open-access article distributed under the terms of the Creative Commons Attribution License (CC BY). The use, distribution or reproduction in other forums is permitted, provided the original author(s) and the copyright owner(s) are credited and that the original publication in this journal is cited, in accordance with accepted academic practice. No use, distribution or reproduction is permitted which does not comply with these terms.
*Correspondence: Wen-Chieh Cheng, dy1jLmNoZW5nQHhhdWF0LmVkdS5jbg==
Disclaimer: All claims expressed in this article are solely those of the authors and do not necessarily represent those of their affiliated organizations, or those of the publisher, the editors and the reviewers. Any product that may be evaluated in this article or claim that may be made by its manufacturer is not guaranteed or endorsed by the publisher.
Research integrity at Frontiers
Learn more about the work of our research integrity team to safeguard the quality of each article we publish.