- 1Interdepartmental Centre for Regenerative Medicine “Stefano Ferrari”, University of Modena and Reggio Emilia, Modena, Italy
- 2Holostem Terapie Avanzate S.r.l., Modena, Italy
- 3Università Cattolica del Sacro Cuore, Rome, Italy
- 4Thoracic Surgery Unit, Fondazione Policlinico Universitario A. Gemelli IRCCS, Rome, Italy
Breathing, being predominantly an automatic action, is often taken for granted. However, respiratory diseases affect millions of people globally, emerging as one of the major causes of disability and death overall. Among the respiratory dysfunctions, tracheal alterations have always represented a primary challenge for clinicians, biologists, and engineers. Indeed, in the case of wide structural alterations involving more than 50% of the tracheal length in adults or 30% in children, the available medical treatments are ineffective or inapplicable. So far, a plethora of reconstructive approaches have been proposed and clinically applied to face this growing, unmet medical need. Unfortunately, none of them has become a well-established and routinely applied clinical procedure to date. This review summarizes the main clinical reconstructive attempts and classifies them as non-tissue engineering and tissue engineering strategies. The analysis of the achievements and the main difficulties that still hinder this field, together with the evaluation of the forefront preclinical experiences in tracheal repair/replacement, is functional to promote a safer and more effective clinical translation in the near future.
Introduction
Tracheal and main bronchi dysfunctions represent an unmet medical need in respiratory medicine. Indeed, congenital malformations, malignancies, inflammations, infections, or even traumatic events, including postoperative complications, can alter tracheal and main bronchi structure and function, heavily affecting patients’ life. Nowadays, this issue represents an extraordinary leading-edge topic. Indeed, as recently stated by the European Laryngological Society, the heightened number of long-term intubations and the huge tracheostomy rate in critically ill COVID-19 patients might shortly determine an unprecedented increase in laryngotracheal granulomas, stenosis, malacia, tracheal necrosis, tracheo-oesophageal and trachea-innominate fistulae (Alturk et al., 2020; Mattioli et al., 2021; Piazza et al., 2021). The first-choice treatment for managing short tracheal structural alterations is based on tracheal resection and reconstruction by end-to-end anastomosis. However, surgery is inapplicable in longer tracheal injuries exceeding 5 cm in adults and 3 cm in children, and only palliative care can be offered to these patients (Delaere et al., 2019).
To date, a variety of approaches has been proposed and clinically adopted to replace long-segment tracheal defects and reconstruct this vital organ. However, despite the great efforts, the limited results clearly pointed out that a final solution for restoring a functional respiratory system is extremely challenging and far from being addressed (Grillo, 2002; Soriano et al., 2021).
Why is the Trachea so Hardly Replaceable?
Despite its apparently simple shape and structure, the trachea is a complex organ, challenging to be replaced. Indeed, it is a semiflexible tube measuring approximately 5 cm in children under 3 months of age (Lee and Yang, 2001) and reaching 10–13 cm in adults (Cinar et al., 2016). It comprises up to 22 C-shaped hyaline cartilaginous rings posteriorly connected by smooth muscle embedded into a fibro-elastic connective tissue (Brand-Saberi and Schäfer, 2014). This structure gives a peculiar lateral rigidity and longitudinal flexibility, critical for controlling the trachea lumen diameter, preventing its collapse and supporting the trachea during inspiration/expiration (Grillo, 2002; Teng et al., 2012). This architecture confers to the trachea also the ability to resist neck movements and pressure coming from the adjacent oesophagus. Moreover, being in direct contact with the outside world, the innermost part of the trachea is lined by a specialized and pseudostratified respiratory epithelium. This latter plays a central role within tracheal physiology, orchestrating one of the most intricated and sophisticated response mechanisms of the entire human body (Ganesan et al., 2013). Indeed, it not only acts as the primary physical barrier against environmental factors, continuously filtering, warming and humidifying the inhaled air, but it also perceives potential dangers and, in synergy with immune and nervous cells, puts in place the most appropriate response (Davis and Wypych, 2021). The complexity of the respiratory epithelium is reflected by the great heterogeneity encountered at the cellular level, in which each cell type plays a specific role within epithelial physiology (Goldfarbmuren et al., 2020). Thus, every alteration in cell content or selective damage to one or more cell types may contribute to the development of respiratory disorders (Davis and Wypych, 2021). Finally, the trachea is nourished by a multitude of tiny capillaries that branch mainly from the inferior thyroid artery and provide the blood supply to the pseudostratified epithelium and the surrounding tissues (Salassa et al., 1977). It is difficult to recreate or restore this intricated blood supply through anastomosis, therefore it appears as a major challenge in tracheal reconstructive attempts (Delaere et al., 2010).
This review is focused on the main clinical reconstructive strategies for replacing long-circumferential tracheal defects. Procedures relying only on external or internal splint/stent as a mechanical support to the weakened tissue and patch-based reconstructive approaches were not included. According to their manufacturing process, the revised approaches were classified in two macro-categories, namely non-tissue engineering (non-TE) and tissue engineering (TE) attempts (Figure 1). Indeed, the former comprises the use of synthetic prostheses or allogenic/autologous tissues directly transplanted in vivo, while the latter relies on an in vitro step of scaffold manufacturing and/or cell expansion. The clinical outcomes have been analyzed for each strategy to point out the achievements and the weaknesses that still hinder this field. Moreover, non-exhaustive examples of the forefront TE attempts on animal models were described as promising perspectives for the clinical translation. Thanks to this analysis, we identified some critical aspects that must be considered and implemented in designing new tracheal substitutes, thus paving the way towards safer and more effective solutions for treating patients with long-circumferential tracheal defects.
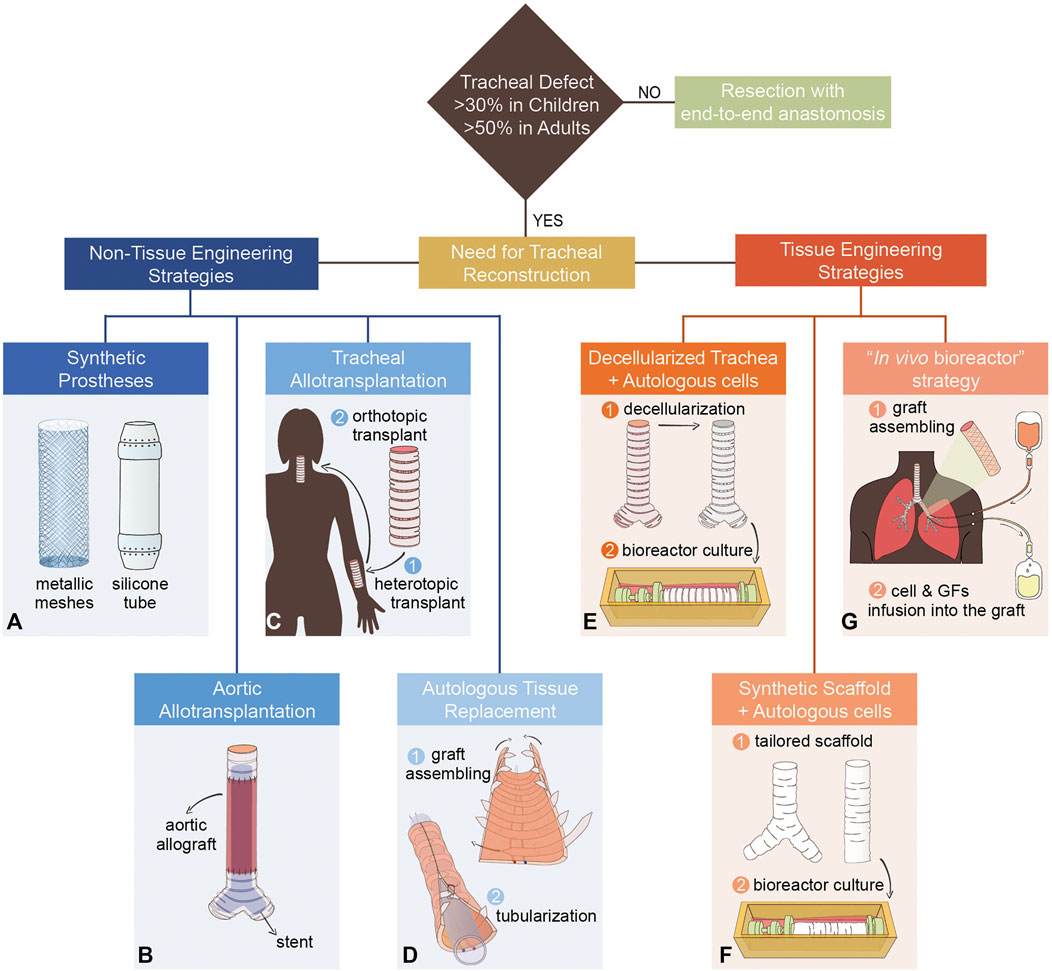
FIGURE 1. Examples of tracheal and main bronchi’s reconstructive strategies. (A) Synthetic prostheses: metallic meshes and silicone tube. (B) Aortic allotransplantation with a supporting stent. (C) Tracheal allotransplantation: two-step procedure with pre-vascularization in heterotopic position (forearm) followed by orthotopic transplantation. (D) Autologous tissue replacement: stripes of rib cartilage are inserted in a skin forearm free flap (graft assembling phase). Then, the graft is tubularized to reproduce the tracheal lumen. (E) An allogenic trachea is decellularized and then recellularized in a rotating bioreactor with autologous cells. (F) Synthetic tailored tracheal grafts seeded with autologous cells in a rotating bioreactor. (G) A graft composed of a nitinol stent inserted between two layers of porcine acellularized dermis matrix is seeded with autologous skin keratinocytes (graft assembling phase). Once transplanted, the graft is alternately perfused, through pumps and cannulas, with antibiotics, autologous cells, and growth factors (GFs).
Non-Tissue Engineering Approaches for Tracheal Restoration
In the last century, a plethora of non-TE approaches has been proposed and applied in small series or even single patients to treat long-circumferential tracheal defects. Based on common methodologies, these attempts can be further clustered into three main categories: synthetic prostheses, allotransplantations, and autologous tissue reconstruction. Table 1 summarizes some of the main non-TE approaches tested so far and still considered as potential tracheal reconstruction strategies.
Synthetic Prostheses
Historically, tracheal reconstructive approaches involving rigid polymer constructs, glass or metal prosthesis, or more flexible materials such as polymeric or metallic wire/meshes, and silicone, have always been unsuccessful in clinical practice (Greaney and Niklason, 2021). Indeed, rigid polymer constructs or prostheses, despite remaining patent, are challenging to be properly sutured and often shifted out. The complication led to airway obstruction with consequent pneumonia or even death (Abbott et al., 1932; Clagett et al., 1948; Longmire, 1948; Cotton et al., 1952; Blades and Beattie, 1986). Moreover, these inert materials do not support the regeneration of the mucosal tissue, essential for a functional tracheal restoration. Similarly, silicone tubes have been used to exploit their durability, low reactivity, and mechanical flexibility. However, the lack of integration with the surrounding tissue, granulation formation and haemorrhages due to graft mobilization strongly limited their clinical diffusion (Neville et al., 1976; Neville et al., 1990; Toomes et al., 1985; Schneider et al., 2001). Finally, polymeric or metallic wire/meshes have also been adopted as tracheal substitutes, displaying unsafe and ineffective outcomes (Greaney and Niklason, 2021). Indeed, besides the usual granulation tissue formation, scaffold migration with erosion of the surrounding vessels and oedema, these prostheses lack adequate mechanical properties, leading to airway narrowing and collapse (Clagett et al., 1948; Kramish and Morfit, 1963). Therefore, to date, prosthetic reconstruction is no longer considered a therapeutic solution for long-circumferential tracheal defects.
Tracheal Replacement With Allogenic Tissues
This strategy relies on the use of human cadaveric allogeneic tissues, among which aortic and tracheal allografts have been widely used.
Aortic Allotransplantation
Aortic allografts have been employed for tracheal repair by different groups, especially in emergencies, due to their wide availability in a variety of sizes (Davidson et al., 2009; Martinod et al., 2017, Martinod et al., 2018; Menna et al., 2021; Wurtz et al., 2006, Wurtz et al., 2010). However, these replacements lack the adequate biomechanical properties of the trachea, as well as the presence of respiratory epithelium. Therefore, to preserve the airway patency, aortic allografts required stenting, often rejected by the patient’s body. Moreover, several frequently fatal surgical-related complications were observed, such as severe bilateral pneumonia, anastomotic dehiscence, fungal infection, spinal cord ischemia and even cardiac arrest (Davidson et al., 2009; Wurtz et al., 2006, Wurtz et al., 2010). Additionally, these reconstructive approaches do not consider the need for an effective blood supply reestablishment, and rely only on host cells migration for graft repopulation.
In 2017, a clinical trial (TRACHEOBRONCART, NCT01331863) evaluated cryopreserved, stented aortic graft for tracheal replacement in patients affected by end-stage airway diseases (Martinod et al., 2017). So far, in the 13 patients reported, Martinod and colleagues showed that the cryopreserved aortic graft promoted the regeneration of new tissue (Martinod et al., 2017, Martinod et al., 2018). However, some stent-related complications such as infection and granuloma were also observed, suggesting that further studies are needed to confirm if this technique is safely applicable for long-circumferential damages or should be restricted to patch repairs only. Recently, a stented cryopreserved aortic allograft has also been used for the first time to treat a post-COVID-19 patient presenting multiple tracheal stenoses, tracheomalacia and ossification (Menna et al., 2021).
Tracheal Allotransplantation
Tracheal segments derived from brain-dead donors have been frequently used as non-vascularized allografts to treat patients with extensive tracheal damages. In 1993, Levashov et al. described a one-stage procedure consisting of direct trachea transplantation from a just passed away donor. Ten days postoperation, despite immunosuppression, signs of rejection appeared, followed by graft stenosis, which required the implantation of a silicone stent (Levashov et al., 1993). The direct tracheal allotransplantations outcomes confirmed these results, coupled with graft’s ischemia and necrosis within 2 months post-implantation. Formalin-fixed tracheas have been allotransplanted to reduce tissue immunogenicity, increase its stiffness and be readily available in case of emergency. However, despite early success, the long-term follow up revealed graft stenosis and the need for stenting, reasons why this procedure was almost completely abandoned (Greaney and Niklason, 2021).
In 2008, after studies in animal models and a first human preliminary report in 1979 (Rose et al., 1979; Delaere et al., 1995; Delaere et al., 1996), Delaere’s group performed a two-step tracheal allotransplantation in a patient with a long history of tracheal stenosis (Delaere et al., 2010). In this procedure, the trachea obtained from a brain-dead individual was first heterotopically implanted into the recipient’s forearm to promote vascularization and then transplanted in its orthotopic position. Additionally, the respiratory mucosa of the donor trachea was replenished by a flap of oral mucosa from the recipient, creating a chimeric graft. The same treatment was applied on six patients leading to several complications, principally related to rejection after withdrawal of immunosuppressant and graft necrosis, which led to a partial loss of the allograft (Delaere et al., 2012; Delaere and Van Raemdonck, 2016). In conclusion, the reconstructed airways were insufficient to sustain respiration, and required tracheostomy in some cases. Recently, the same technology has been applied by Iyer and colleagues to treat a patient affected by tracheal adenoid cystic carcinoma. However, soon after the surgery, a strong haemorrhage arising from the “neotrachea” led to the patient’s death (Iyer et al., 2020).
These poor outcomes point out some significant limitations related to this technology, including 1) dependence on donor availability and related blood matching (Delaere et al., 2010), 2) long time requirement for heterotopic pre-vascularization of the graft (inapplicable in emergencies), 3) extended periods of immunosuppression to limit rejection of the graft, which exposes the subject to opportunistic infections and that is inapplicable in case of cancer patients, and 4) altered mucociliary clearance, colonization with abnormal flora and secondary morbidity in case of heterotopic use of skin/oral mucosa flap to replace the necrotic tracheal epithelium (Udelsman et al., 2018).
Only recently, Genden and colleagues, after preclinical in vivo studies on animal models (Genden et al., 2002; Genden et al., 2003), tried to overturn a longstanding dogma for which the tracheal microvascular anastomoses is not achievable (Genden et al., 2021). Specifically, Genden performed a single-stage tracheal vascular composite allotransplantation (VCA) involving the thyroid arteries and the muscular wall of the donor’s oesophagus that shares blood supply with the donor trachea. The restoration of the blood supply was successfully obtained through microvasculature anastomoses, and a well-perfused continuous airway respiratory epithelium was observed via endoscopy and histological examination. However, even if this approach addresses some of the problems faced in the two-step tracheal allotransplants, the need for immunosuppression still represents a limitation restricting this approach to non-oncologic patients only. Moreover, a longer follow-up and a larger cohort of patients are required to evaluate the safety and the efficacy of this procedure (Randhawa and Patterson, 2021).
Tracheal Replacement With Autologous Tissues
Finally, a different approach involves multiple autologous tissues as a source for tracheal and main bronchi reconstruction, such as aortic autograft (Azorin et al., 2006), pulmonary tissue (Zhang and Liu, 2015), skin (Spaggiari et al., 2005), intercostal artery muscle flap (Bertheuil et al., 2021), forearm flaps and costal cartilage (Beldholm et al., 2003; Olias et al., 2005; Yu et al., 2006; Fabre et al., 2013; Mercier et al., 2018). The common goal of these reconstructive techniques is to generate a tubularized and well-perfused graft to replace the damaged trachea. In many cases, this was combined with a temporary or permanent stent to give structural stability to the transplant (Azorin et al., 2006; Fabre et al., 2013; Zhang and Liu, 2015). In 2013, after preclinical studies on large animal models (Fabre et al., 2009), Fabre and colleagues published the largest series of patients treated with autologous tissue for airway replacement purposes (Fabre et al., 2013, 2015). They used a single-step procedure to generate and transplant a tracheal substitute made by regular intervals of forearm free fasciocutaneous flaps and costal cartilages. Following this procedure, seven out of twelve patients developed acute respiratory distress syndrome, and two became tracheostomy dependent (Udelsman et al., 2018).
Although these innovative approaches allow avoiding immunosuppression, the variable clinical outcomes highlight several issues, such as 1) the need for temporary or permanent stents, 2) the absence of an integer epithelium, which is crucial for a physiologic mucociliary clearance, and 3) the risk of morbidities at the donor site following the withdrawal of autologous tissue.
Tissue Engineering Approaches for Tracheobronchial Reconstruction
Tissue engineering (TE) approaches rely on the combination of cells with an appropriate scaffold for the treatment of significant tissue defects. Indeed, in this interdisciplinary field, principles of biomaterial engineering, genetics, cell biology and clinical science are combined to develop a biological graft to maintain, restore or improve tissues or whole organs (Shafiee and Atala, 2017; Vranckx and Hondt, 2017). This solution can simultaneously address different problems, such as donor shortage (often fatal for patients in critical status, struggling with long waiting lists), immunosuppression therapy and donor site morbidity. In fact, TE strategies take advantage of the body’s regenerative potential and, by in vitro expansion, allow to obtain enough cells to regenerate extensive body areas from a small biopsy (Gallico, 1985; Corradini et al., 2016). Thus, this field represents a promising strategy for expanding the current reconstructive armamentarium to treat severe unmet medical needs (Vranckx and Hondt, 2017).
Between 2008 and 2017, TE attempts for windpipe and main bronchi’s replacement have been tested in compassionate cases, and the trachea has been acclaimed by both the scientific community and mass media as the first tissue-engineered organ (Fountain, 2012). However, this field was impaired by a history of scientific and ethical misconduct (The Lancet, 2018; The Lancet Editors, 2018; Jungebluth et al., 2019), which has led to a general sense of mistrust concerning airway TE potential.
To date, three main strategies have been proposed in tracheal TE, which involves the use of allogenic decellularized human cadaveric donor tissue, synthetic patient-tailored scaffolds, or an “in vivo bioreactor” strategy. Table 1 summarizes some of the main experiences in this field.
Allogenic Decellularized Tissues
Among the different scaffolds used for TE approaches, the extracellular matrix (ECM) obtained through decellularization of allogenic tissues has been used for tracheal replacement. An example of allogenic tissue devoided of the cellular component is the extracellular dermal matrix, recently used as non-vascularized graft for long-segmental tracheal replacement (Bolton et al., 2017). However, this non-resolutive strategy required postoperative refinement due to graft migration. Moreover, this attempt relies only on cell migration from the wound edges, insufficient in case of extensive defects. On the other hand, the ECM obtained from allogenic human cadaveric tracheas has been adopted either with cultured autologous cells or freshly harvested hematopoietic stem cells.
Allogenic Tracheal Extracellular Matrix Plus Cultured Cells
The first patient to receive a TE approach was a 30 year-old woman suffering from end-stage bronchomalacia (Macchiarini et al., 2008). In this compassionate case, a human donor trachea was decellularized with an extensive protocol to obtain a suitable scaffold to be colonized with autologous cells. Specifically, epithelial cells isolated from a bronchus’ mucosal biopsy were cultured under serum-free conditions, while mesenchymal stem cells from bone marrow aspirate were expanded and induced to differentiate in chondrocytes (Macchiarini et al., 2008). Through a perfusion system, a bioreactor with two separate accesses was used to seed epithelial cells onto the internal surface of the decellularized trachea. At the same time, the chondrocytes were injected into the external surface of the matrix. Finally, after surgical removal of the damaged tissue, the avascular graft was shaped and sutured to the remaining native tissue (Macchiarini et al., 2008). In 2014, Gonfiotti et al. reported the patient’s 5-year follow-up, describing this TE approach as safe and promising.
However, the postoperative course was characterized by many complications. The development of granulation tissue and cicatricial scar led to the implantation of several endoluminal stents to maintain the airway patent. Continuous reinterventions were necessary to remove the different stents rejected by the patient’s body and the granulation tissue responsible for stent obstruction (Gonfiotti et al., 2014). Such graft-related postoperative complications strongly affected the patient’s quality of life (Molins, 2019).
Later on, Elliott and colleagues adopted a similar technique to treat a 15 year-old girl born with severe congenital malformations (a single left lung and long-segment congenital tracheal stenosis) after other failed reconstructive approaches (Elliott et al., 2017). In this study, every step of the reconstructive procedure was designed to fulfil good manufacturing practice (GMP) standards. Specifically, a donor trachea was decellularized with GMP-compliant reagents. Meanwhile, autologous bone marrow cells and epithelial cells from the inferior turbinate were expanded in a licensed cell therapy facility and then seeded onto the decellularized matrix in a bioreactor (Elliott et al., 2017). Despite promising early results, an acute tracheal obstruction of the posterior wall occurred 2 weeks post-transplantation and led to the young girl’s death. Given this negative outcome, the authors recommended using stents during the first few months postoperatively. Moreover, they highlighted the difficulties in translating a TE reconstructive approach from the preclinical setting to the clinic, even because the in vivo models cannot mimic complex clinical scenarios (Elliott et al., 2017).
Allogenic Tracheal Extracellular Matrix Plus Freshly Harvested Cells
To reduce the time needed for producing a TE tracheal substitute, a decellularized trachea was saturated with freshly harvested hematopoietic stem cells. Besides, patches of tracheal epithelium were placed as free grafts within its lumen and secured via a bioresorbable stent. This TE attempt aimed to recreate an in vivo microenvironment, recapitulating some of the key stimuli that lead to the physiological post-injury repair. In this respect, several growth factors were systemically administrated both in the preoperative and postoperative periods and locally injected into the avascular TE construct during the implantation. This pharmacological support aimed to mobilize haemopoietic stem cells and endothelial progenitors, improve mesenchymal stromal cells (MSCs) recruitment, induce chondrocyte differentiation, and increase angiogenesis (Elliott et al., 2012). The 4-year follow-up of a 10 year-old child treated with this approach declared the boy’s good health, assessing this procedure as lifesaving (Hamilton et al., 2015). Despite this, many reinterventions (more than 25) were needed, especially during the first year of follow-up, to clear secretion, remove granulation tissue and replace the resorbable stent. Difficulties in the re-epithelialization of the decellularized tracheal matrix have been observed. Indeed, the histological analysis of a biopsy from the TE graft at 1 month post-intervention revealed granulation tissue only. Instead, a biopsy of the proximal transplant collected at 42 months, showed an epithelial layer with a mix of squamous and respiratory epithelium and only a few ciliated cells. Probably, the graft vascularization was insufficient to allow the epithelialization of the decellularized matrix from the free graft’s patches of tracheal mucosa, and the poor epithelialization occurred from the migration of cells from the wound edges. Nonetheless, this mechanism can cover only a few millimetres of the graft, while the central tissue is left as uncovered granular tissue (Delaere et al., 2014).
Patient-Tailored Synthetic Scaffolds
Since 2011, synthetic tailored scaffolds repopulated by autologous bone marrow cells and supported by growth factors have been proposed to revolutionize regenerative medicine. Those constructs were made of nanocomposite polymer POSS-PCU (polyhedral oligomeric silsesquioxane–poly (carbonate-urea) urethane) or electrospun polyblend PET/PU (polyethylene terephthalate (PET) and polyurethane (PU)) (Fux et al., 2020) and were described as able to integrate within the recipient, generating living and functional grafts covered by epithelium (Del Gaudio et al., 2014; Jungebluth et al., 2011, Jungebluth et al., 2013). However, these papers were retracted for scientific misconduct in the following years. Only recently, Fux and colleagues have unequivocally stated the inadequacy of those constructs for TE purposes. In this retrospective study, the authors presented the first long-term follow-up of three patients, who in total received four synthetic tracheal grafts recellularized with bone marrow–derived mononuclear cells (BM-MNCs). During the postoperative period, all patients developed graft-related debilitating complications. Follow-up analysis showed the formation of anastomotic fistulae and obstructive granulation tissue as well as the absence of vascularization, epithelial lining, or integration within the surrounding tissue. All patients died after multiple surgical reinterventions, revealing the failure of TE synthetic tracheal substitutes as living functional grafts (Fux et al., 2020). Indeed, the “bioengineered” constructs behaved only like an inert scaffold, similarly to synthetic tracheal prostheses (Delaere et al., 2019).
“In Vivo Bioreactor” Strategy
To face the challenges related to the delayed revascularization process and infections, Tan et al. proposed the use of the recipient body as a bioreactor for the TE substitute (Tan et al., 2017). This approach aims to combine the commonly separated in vitro 3D cell-scaffold culture with the in vivo regenerative process. With this purpose, a nitinol stent - providing lateral rigidity and longitudinal flexibility to the TE construct - was surrounded by two layers of a biodegradable porcine acellularized dermis matrix (ADM). One hour before transplant, the luminal side of the scaffold was seeded with epidermal keratinocytes obtained from the digestion of a skin flap. Two catheters were inserted among the two ADM layers and associated with peristaltic pumps during the implantation. Through this cannulation system, the avascular TE graft was perfused for 1 month with Ringer’s gentamicin fluid to prevent infection and to keep the epithelial cells alive before final revascularization. Moreover, this system allowed the secondary infusion of total nucleated cells (TNCs) and growth factors to stimulate graft regeneration directly into the transplanted TE construct. Unfortunately, the authors reported the patient’s death 13 months postoperatively. Consequently a longer follow-up is unavailable (Tan et al., 2017). Major doubts remain regarding the durability and functionality of this bioengineered construct. In fact, the gradual biodegradation of the ADM scaffold, observed during the postoperative bronchoscopies, and the use of epidermal keratinocytes instead of airway ciliated epithelial cells could be responsible for long-term severe graft-related complications.
Frontiers of Tracheal Substitutes: Lessons From Preclinical Animal Studies
Failures and controversies raised by the clinical application of several tracheal substitutes have led to a renewed interest in preclinical studies based on animal models. According to Niermeyer et al., 73% of articles focused on tracheal reconstruction and published between 2015 and 2020 involved an in vivo preclinical model. This reveals the need for a more in-depth preliminary analysis of the tracheal substitutes before embarking on new clinical applications (Niermeyer et al., 2020).
This paragraph focuses on the latest in vivo preclinical TE attempts, since they stand as one of the most promising approaches to face hurdles that still hinder this field. Indeed, articles concerning tissue-engineered tracheal grafts (TETGs) aim to improve graft integration within the recipient, mimick complex native trachea biomechanics and prevent host adverse responses (Greaney & Niklason, 2021). To give an idea of the possible future clinical applications, a few non-exhaustive examples belonging to the different categories of the current TE approaches are listed below and summarized in Table 2.
Concerning the use of synthetic material, several methodologies have been investigated to produce tubular scaffold suitable for tracheal replacement. In 2011, a casting-based manufacturing process was used to create a tracheal-shaped substitute starting from mixtures of rat fibroblast/MSCs and collagen hydrogels. The stiffness of the resulting bioartificial trachea was compared to the one of the native trachea showing no differences. However, when the former was transplanted in a rat model, only three of the nine treated animals survived the implantation, dying within 48 h after surgery. The author suggests that these results could be related to the absence of epithelial cells on the inner layer of the transplanted bioartificial trachea (Naito et al., 2011).
Some years later, Pepper et al. employed a polyethylene terephthalate (PET) and polyurethane (PU) scaffold combined with polycarbonate rings. This structure was seeded with BM-MNCs and transplanted in eight sheep to replace a 5 cm long tracheal defect. Despite promising mechanical tests, all animals showed graft stenosis associated with granulation tissue. Overall, this attempt remarked the centrality of the epithelialization and neovascularization, in the absence of which the outcomes are poor (Pepper et al., 2019).
Using a mouse model, another group compared synthetic non-resorbable PET/PU vs. resorbable poly(l-lactide-co-ε-caprolactone)/Polyglycolic acid (PLCL/PGA) scaffolds. Even in this study, graft’s stenosis was revealed in both conditions, with no signs of respiratory epithelization in the central part of the grafts (Dharmadhikari et al., 2019).
Several groups also investigated synthetic scaffolds based on polycaprolactone (PCL), given its easy moldability through 3D printers (Gao et al., 2017; Rehmani et al., 2017). To mimic the tracheal structure and mechanical profile, Lee and colleagues used a bellows-designed PCL scaffold reinforced with collagen, silicon rings and seeded with human turbinate mesenchymal stromal cells (hTMSC) sheets (Lee et al., 2018). After implantation in rabbits, the PCL was successfully incorporated within the adjacent tissue and lined by airway epithelium. However, respiratory distress and mild granulation process were observed in all animals. Additionally, the higher levels of interleukin-2 and interferon gamma detected in treated animals, compared to the baseline values, suggested a possible acute rejection (Lee et al., 2018). Two years later, another group used both electrospinning and 3D printing techniques to generate a PCL synthetic scaffold for tracheal reconstruction in a rabbit model. In this study, human bronchial epithelial cells (hBECs) were used to populate the inner layer of the PCL scaffold, while the outer layer was repopulated by either induced pluripotent stem cells-derived mesenchymal stem cells (iPSC-MSCs) or induced pluripotent stem cells-derived chondrocytes (iPSC-Chds). A regenerated epithelium was observed in both conditions at the study endpoint (4 weeks). However, in the iPSC-MSCs group, the epithelium was fully specialized and better organized than in the iPSC-Chds group, suggesting a paracrine effect of iPSC-MSCs in promoting the re-epithelialization process (Kim et al., 2020). To overcome the aforementioned problems related to synthetic tracheal TE grafts, other groups tried to reduce the immunogenicity of the grafts, increasing their survival prospects through pre-implantation vascularization strategies (Soriano et al., 2021). For example, Zhao et al. seeded smooth muscle cells onto a PGA-nitinol stent scaffold to allow the deposition of a collagenous matrix and increase the scaffold angiogenetic properties. The resulting construct was then decellularized, preserving the new extracellular matrix, and implanted in rabbits and nonhuman primates. No graft collapse was observed, while a columnar respiratory epithelium lined the construct lumen. However, mid-grafts stenosis was present (Zhao et al., 2016). In the preclinical study performed by Sakaguchi and colleagues, 80% of dogs transplanted with a pre-vascularized collagen-coated nitinol scaffold survived for more than 18 months. No signs of stenosis were reported, while a monolayer of ciliated cells covered the graft’s lumen. These promising results may be due to the pre-vascularization obtained through the graft’s heterotopic implantation into the omentum (Sakaguchi et al., 2018). Similar beneficial effects of the pre-vascularization process were observed in a rabbit preclinical study where a 3D printed PLLA (poly L-lactic acid) scaffold seeded with autologous chondrocytes was used to reconstruct a 1.5 cm long tracheal segment. Indeed, the engineered pre-vascularized trachea appeared integrated within the adjacent tissues and covered by respiratory epithelium. Besides, no signs of stenosis were observed with only sporadic granulation tissue formation (Gao et al., 2019).
Besides synthetic scaffolds, decellularized grafts have been employed in preclinical settings. However, when used without the cellular component, they behaved similarly to the polyhedral oligomeric silsesquioxane–poly (carbonate-urea)urethane (POSS-PCU) synthetic scaffolds, mainly developing stenosis (Maughan et al., 2017). Otherwise, when recellularized with epithelial cells and MSC-derived chondrocytes, they become functional and well-tolerated, without signs of rejection or airway collapse (Go et al., 2010). These results remark the need for the cellular component to promote graft re-epithelialization and structural stability essential for preventing host inflammatory response and graft failure.
Finally, new frontiers may arise from scaffold-free constructs. Indeed, through a novel Bio-3D printing system, many groups developed artificial tracheas assembling spheroids composed of various cell types, such as chondrocytes, MSCs and endothelial cells (Taniguchi et al., 2018; Machino et al., 2019). In these studies, to obtain a structure that recapitulates the tracheal shape, the multicellular spheroids were first placed into needle arrays and then underwent a maturation phase inside a bioreactor. Finally, the artificial scaffold-free constructs were implanted in rat models to evaluate the graft performance. At the end of the studies, these artificial tracheas showed signs of chondrogenesis, vasculogenesis, and an epithelium lining the lumen. However, the need for a permanent stent to avoid graft collapse and the presence of granulation tissue represent some limitations of this innovative technique (Taniguchi et al., 2018; Machino et al., 2019).
Discussion
Tracheal and main bronchi dysfunctions represent an unmet and growing medical need, especially in the case of wide circumferential structural alterations where available surgical strategies are ineffective or inapplicable. The long-term clinical outcomes of reconstructive approaches tested so far clearly point out the difficulty of restoring a functional trachea (Grillo, 2002; Soriano et al., 2021).
Since the first challenge of any tracheal replacement’s attempt is to reproduce or mimic the native tracheal structural properties, a suitable tracheal substitute must fulfil specific biomechanical requirements. Indeed, it has to be airtight and possess longitudinal flexibility and lateral rigidity to withstand forces arising from respiration, coughing, neck movements and pressure created by the adjacent oesophagus (Grillo, 2002; Boazak and Auguste, 2018). Former approaches not fulfilling these requirements resulted in airway collapse, strictures, graft migration, or haemorrhages which, altogether, stand as the primary cause (68%) of graft-related mortality (Greaney and Niklason, 2021). Consequently, a preliminary assessment of the tracheal substitute’s suitability needs to be performed to reduce avoidable life-threatening adverse events on the patients. Indeed, several in vivo preclinical studies have been carried out to evaluate biochemical properties of decellularized (Zhao et al., 2016), synthetic (Dharmadhikari et al., 2019) or scaffold-free constructs (Machino et al., 2019). However, since the first requirement for an adequate tracheal substitute is to mimic the tracheal’s native biomechanical properties, the definition of standard approaches and biomechanical tests would be extremely helpful to obtain comparable results among different studies and further improve this field (Martínez-Hernández et al., 2021).
Another limitation for a successful reconstructive approach is the lack of studies on the biocompatibility between the tracheal substitute and the host. As previously described, this problem has been reported to trigger acute rejection, granulation tissue formation and graft necrosis, especially in the case of synthetic prostheses (Neville et al., 1976, Neville et al., 1990; Toomes et al., 1985), bioprosthetic substitutes (Hoffman et al., 2001) and tracheal allograft (Levashov et al., 1993; Delaere et al., 2010; Delaere et al., 2012). Before implantation, specific biocompatibility studies should be performed to ensure that the tracheal substitute is made of safe, non-immunogenic material, well-tolerated by the recipient and able to integrate within the body. In order to avoid the aforementioned rejection problem, particularly marked in the case of allograft, also autologous tissues have been clinically applied (Olias et al., 2005; Spaggiari et al., 2005; Fabre et al., 2013, Fabre et al., 2015; Zhang and Liu, 2015; Mercier et al., 2018). However, even this procedure presented some problems, such as donor-site morbidity (Udelsman et al., 2018). In this scenario, TE represents a unique opportunity to rebuild extensive body surfaces, combining cells extracted from a small autologous biopsy with an appropriate scaffold (Shafiee and Atala, 2017; Vranckx and Hondt, 2017).
Regardless of the reconstructive approach, one of the common hurdles in tracheal replacement is graft epithelialization. Indeed, airway functions are critically dependent on the respiratory epithelium, and its absence triggers several complications, including mucous stagnation, infections, graft stenosis, granulation tissue formation, chondromalacia and fibrogenic reaction (Heijink et al., 2014; Paternoster and Vranckx, 2021). Therefore, any tracheal replacement attempt should be aimed at restoring a functional and well specialized respiratory epithelium (Davis and Wypych, 2021). Several aspects must be considered to ensure the presence of a continuous, self-renewing and specialized respiratory epithelium lining the lumen of the tracheal substitute. First, due to the respiratory tissue’s high complexity and cellular heterogeneity, epithelial cells or sheets derived from other autologous districts (i.e., skin or oral mucosa) cannot be used for airway functional recovery (Tan et al., 2017; Udelsman et al., 2018). Second, spontaneous post-surgery reepithelization of the tracheal substitute cannot be taken for granted. In clinical and preclinical studies, several groups relied on the migration of epithelial cells from the recipient’s wound edges (Martinod et al., 2017, Martinod et al., 2018; Rehmani et al., 2017), with some successful epithelialization only on short-sized scaffolds (Zhao et al., 2016; Lee et al., 2018; Sakaguchi et al., 2018). Indeed, local epithelial cells can only cover a few millimeteres of the graft, while the central part of the tracheal substitute is often left uncovered, leading to the aforementioned adverse consequences of an absent epithelium (Delaere and Van Raemdonck, 2019). Therefore, a precise and rational reepithelization strategy is mandatory, especially for tracheal substitutes of larger dimensions. On this note, in TE approaches, the culture conditions used for the in vitro expansion phase must preserve the proliferative and differentiative potential of the airway epithelial stem cells, preventing their exhaustion. Indeed, the experience gained from regenerative approaches of other human epithelial tissues clearly pointed out that a specific number of stem cells is strictly required to allow the permanent engraftment, renewal, and restoration of the epithelium (Pellegrini et al., 2011; Maurizi et al., 2021). In order to carefully monitor these aspects and have a clear overview of all the process variables, extensive cellular characterization must be performed. This comes through the identification of population-specific molecular markers to be adopted as quality controls during each step of the reconstructive procedure.
To date, there is a huge gap between basic-research knowledge on airway epithelial cells’ biology and the application of these insights to TE translational approaches. Indeed, despite the huge work carried out to understand the airway epithelial cells physiology and heterogeneity (Garcıá et al., 2019; Basil et al., 2020; Goldfarbmuren et al., 2020; Zaragosi et al., 2020; Davis and Wypych, 2021), the few groups that clinically employed airway epithelial cells for tracheal TE approaches did not exploit this knowledge, reporting only limited or inadequate cellular characterization (Macchiarini et al., 2008; Elliott et al., 2017).
In order to bridge this gap, preclinical studies—especially those based on in vitro models—can be extremely useful to precisely evaluate all the interactions among the different components of the bioengineered construct. Indeed, once in contact with the scaffold, colonizing cells should retain their ability to proliferate and differentiate into their respective specialized cell types, a crucial aspect for re-establishing the system’s physiology. Moreover, in the case of TE approaches encompassing cells derived from different tissues (i.e., epithelial cells, chondrocytes, endothelial cells, neural cells etc.), their mutual interaction must be carefully studied to exclude possible acute or chronic cytopathic effects.
Another common issue with all the tracheal reconstructive approaches is the vascularization of the tracheal substitute. Till now, this aspect has deeply hampered the outcomes of treated patients in several clinical studies (Delaere et al., 2012; Delaere et al., 2019). Indeed, efficient and rapid restoration of the blood supply is mandatory to sustain graft survival, allow its integration within the surrounding tissue, avoid necrosis or contamination, and support new cartilage and epithelium regeneration. Some approaches tried to overcome this matter by wrapping tracheal substitutes in a highly vascularized tissue, frequently transposed omentum (Levashov et al., 1993; Elliott et al., 2012; Menna et al., 2021). Although these efforts have been initially interpreted as successful, long-term follow-ups demonstrated that, in most cases, this strategy only temporarily delays the inevitable consequences of wound breakdown at the anastomoses (Delaere and Van Raemdonck, 2014). Alternatively, tracheal substitutes can be in vivo vascularized through heterotopic implantation into the recipient’s forearm, followed by orthotopic repositioning. Still, such strategies’ long-time requirements and invasiveness have strongly limited their application. Once more, a possible solution may arise from in vitro or in vivo preclinical studies investigating the formation of a vascular network through interaction with endothelial cells or from stimulating the revascularization process through the delivery of pro-angiogenic factors (Lovett et al., 2009).
Alongside vascularization, restoring a functional innervation system within the tracheal substitute should be considered. Indeed, the airway epithelium works conjointly with the immune and nervous systems to guarantee respiratory homeostasis (Davis and Wypych, 2021). So far, this aspect has been neglected, as no significant clinical studies have encompassed the inclusion of this component. Thus, it remains a crucial issue to be addressed in the future.
Finally, another critical point to be mentioned regards the legislative side. Since their proposal in 2001 (Directive 2001/83/EC later expanded in REGULATION (EC) No 1394/2007; https://www.ema.europa.eu/en/human-regulatory/overview/advanced-therapies/legal-framework-advanced-therapies), GMP have further complicated the development of novel tissue-engineered advanced therapeutic medicinal products (ATMPs). These regulations have proven themselves critical to achieving safer and standardized advanced therapies (Aiuti et al., 2017; Hirsch et al., 2017; Ram-Liebig et al., 2017; Barbagli et al., 2018; Pellegrini et al., 2018; Kueckelhaus et al., 2021). Nevertheless, the resulting complexity and high expenses in the manufacturing process strongly discouraged researchers from exploring the TE area of study. These obstacles have led to the predilection of non-TE approaches, which instead fall under less stringent legislation.
To conclude, tracheal reconstruction stands as a huge challenge and has yet to be achieved. In this review, we summarized the main lessons learned from the clinical and most recent in vivo preclinical studies, as well as the new frontiers of tracheal TE. We have highlighted the most common issues that still hinders tracheal reconstruction. In our opinion, a strong correlation between basic science and translational medicine is mandatory; careful and extensive preclinical studies are crucial to tackling all the described aspects. Before embarking on new clinical applications, more work on the preclinical side should be done to prevent patients’ exposure to avoidable life-threatening consequences. Only by addressing these points reconstructive approaches can become a turning point in long-circumferential tracheal defects management and affirm themselves as a milestone in regenerative medicine.
Author Contributions
DA, GG, VG, FL, and GP contributed to the analysis of literature, writing, correction and to figures design. DA collected and summarized the whole work. GP also provided the final revision. All authors contributed to the article and approved the submitted version.
Funding
We thank the Award “Lombardia è Ricerca 2018” and “Louis Jeantet Award 2020” for funding the research activities.
Conflict of Interest
Author VG was employed by the company Holostem Terapie Avanzate, author GP is member of the Board of Directors and R & D Director of Holostem Terapie Avanzate.
The remaining authors declare that the research was conducted in the absence of any commercial or financial relationships that could be construed as a potential conflict of interest.
Publisher’s Note
All claims expressed in this article are solely those of the authors and do not necessarily represent those of their affiliated organizations, or those of the publisher, the editors, and the reviewers. Any product that may be evaluated in this article, or claim that may be made by its manufacturer, is not guaranteed or endorsed by the publisher.
Acknowledgments
The authors would like to thank Sara Adamo for her contribution to the Figure 1.
References
Abbott, O. A., Vanfleit, E., and Roberto, A. E. (1932). THE JOURNAL OF THORACIC SURGERY. Med. J. Aust. 1 (4), 134. doi:10.5694/j.1326-5377.1932.tb42299.x
Aiuti, A., Roncarolo, M. G., and Naldini, L. (2017). Gene Therapy for ADA‐SCID, the First Marketing Approval of an Ex Vivo Gene Therapy in Europe: Paving the Road for the Next Generation of Advanced Therapy Medicinal Products. EMBO Mol. Med. 9 (6), 737–740. doi:10.15252/emmm.201707573
Alturk, A., Bara, A., and Darwish, B. (2020). Since January 2020 Elsevier Has Created a COVID-19 Resource centre with Free Information in English and Mandarin on the Novel Coronavirus COVID- 19 . The COVID-19 Resource centre Is Hosted. Elsevier Connect , the company ’ s public news and information . January.
Azorin, J. F., Bertin, F., Martinod, E., and Laskar, M. (2006). Tracheal Replacement with an Aortic Autograft. Eur. J. Cardio-Thoracic Surg. 29 (2), 261–263. doi:10.1016/j.ejcts.2005.11.026
Barbagli, G., Akbarov, I., Heidenreich, A., Zugor, V., Olianas, R., Aragona, M., et al. (2018). Anterior Urethroplasty Using a New Tissue Engineered Oral Mucosa Graft: Surgical Techniques and Outcomes. J. Urol. 200 (2), 448–456. doi:10.1016/j.juro.2018.02.3102
Basil, M. C., Katzen, J., Engler, A. E., Guo, M., Herriges, M. J., Kathiriya, J. J., et al. (2020). The Cellular and Physiological Basis for Lung Repair and Regeneration: Past, Present, and Future. Cell Stem Cell 26 (4), 482–502. doi:10.1016/j.stem.2020.03.009
Beldholm, B. R., Wilson, M. K., Gallagher, R. M., Caminer, D., King, M. J., and Glanville, A. (2003). Reconstruction of the Trachea with a Tubed Radial Forearm Free Flap. J. Thorac. Cardiovasc. Surg. 126 (2), 545–550. doi:10.1016/S0022-5223(03)00357-X
Bertheuil, N., Duisit, J., Isola, N., Lengelé, B., Bergeat, D., and Meunier, B. (2021). Perforator-Based Intercostal Artery Muscle Flap: A Novel Approach for the Treatment of Tracheoesophageal or Bronchoesophageal Fistulas. Plast. Reconstr. Surg. 147, 795E–800e. doi:10.1097/PRS.0000000000007892
Blades, N., and Beattie, J. (1986). The Journal of Thoracic Surgery Original Communications TRACHEAL RECONSTRUCTIOK.
Boazak, E. M., and Auguste, D. T. (2018)., 4. American Chemical Society, 1272–1284. doi:10.1021/acsbiomaterials.7b00738Trachea Mechanics for Tissue Engineering DesignACS Biomater. Sci. Eng.Issue 4
Bolton, W. D., Ben-Or, S., Hale, A. L., and Stephenson, J. E. (2017)., 12. Philadelphia, Pa, 137–139. doi:10.1097/IMI.000000000000034710.1177/155698451701200210Reconstruction of a Long-Segment Tracheal Defect Using an AlloDerm ConduitInnovations2
Brand-Saberi, B. E. M., and Schäfer, T. (2014). Trachea. Thorac. Surg. Clin. 24 (1), 1–5. doi:10.1016/j.thorsurg.2013.09.004
Cinar, U., Halezeroglu, S., Okur, E., Inanici, M. A., and Kayaoglu, S. (2016). Tracheal Length in Adult Human: The Results of 100 Autopsies. Int. J. Morphol. 34 (1), 232–236. doi:10.4067/s0717-95022016000100033
Clagett, O. T., Grindlay, J. H., and Moersch, H. J. (1948). Resection of the Trachea. Arch. Surg. 57 (2), 253–266. doi:10.1001/archsurg.1948.01240020258008
Corradini, F., Zattoni, M., Barbagli, G., Bianchi, G., Giovanardi, M., Serafini, C., et al. (2016). Comparative Assessment of Cultures from Oral and Urethral Stem Cells for Urethral Regeneration. Curr. Stem Cell Res. Ther. 11 (8), 643–651. doi:10.2174/1574888x10666150902094644
Cotton, B. H., Penido, J. R. F., and Penido, J. R. F. (1952). Resection of the Trachea for Carcinoma. J. Thorac. Surg. 24 (3), 231–245. doi:10.1016/S0096-5588(20)31076-X
Davidson, M. B., Mustafa, K., and Girdwood, R. W. (2009). Tracheal Replacement with an Aortic Homograft. Ann. Thorac. Surg. 88 (3), 1006–1008. doi:10.1016/j.athoracsur.2009.01.044
Davis, J. D., and Wypych, T. P. (20212020). Cellular and Functional Heterogeneity of the Airway Epithelium. Mucosal Immunol. 14, 978–990. –13. doi:10.1038/s41385-020-00370-7
Del Gaudio, C., Baiguera, S., Ajalloueian, F., Bianco, A., and Macchiarini, P. (2014). Are Synthetic Scaffolds Suitable for the Development of Clinical Tissue-Engineered Tubular Organs? J. Biomed. Mater. Res. 102 (7), 2427–2447. doi:10.1002/jbm.a.34883
Delaere, P., and Van Raemdonck, D. (2016). Tracheal Replacement. J. Thorac. Dis. 8 (Suppl. 2), S186–S196. doi:10.3978/j.issn.2072-1439.2016.01.85
Delaere, P. R., Liu, Z., Sciot, R., and Welvaart, W. (1996). The Role of Immunosuppression in the Long-Term Survival of Tracheal Allografts. Arch. Otolaryngol. - Head Neck Surg. 122 (11), 1201–1208. doi:10.1001/archotol.1996.01890230047010
Delaere, P. R., Liu, Z. Y., Hermans, R., Sciot, R., and Feenstra, L. (1995). Experimental Tracheal Allograft Revascularization and Transplantation. J. Thorac. Cardiovasc. Surg. 110 (3), 728–737. doi:10.1016/S0022-5223(95)70105-2
Delaere, P. R., and Van Raemdonck, D. (2020). Commentary: The Sobering Truth about Tracheal Regeneration. J. Thorac. Cardiovasc. Surg. 159 (6), 2537–2539. doi:10.1016/j.jtcvs.2019.10.116
Delaere, P. R., and Van Raemdonck, D. (2014). The Trachea: The First Tissue-Engineered Organ? J. Thorac. Cardiovasc. Surg. 147 (4), 1128–1132. doi:10.1016/j.jtcvs.2013.12.024
Delaere, P. R., Vranckx, J. J., and Den Hondt, M. (2014). Tracheal Allograft after Withdrawal of Immunosuppressive Therapy. N. Engl. J. Med. 370 (16), 1568–1570. doi:10.1056/NEJMc1315273
Delaere, P. R., Vranckx, J. J., Meulemans, J., Vander Poorten, V., Segers, K., Van Raemdonck, D., et al. (2012). Learning Curve in Tracheal Allotransplantation. Am. J. Transplant. 12 (9), 2538–2545. doi:10.1111/j.1600-6143.2012.04125.x
Delaere, P., Van Raemdonck, D., and Vranckx, J. (2019). Tracheal Transplantation. Intensive Care Med. 45 (3), 391–393. doi:10.1007/s00134-018-5445-9
Delaere, P., Vranckx, J., Verleden, G., De Leyn, P., Van Raemdonck, D., Vranckx, J., et al. (2010). Tracheal Allotransplantation after Withdrawal of Immunosuppressive Therapy. N. Engl. J. Med. 362 (7), 138–145. doi:10.1056/NEJMoa0810653
Dharmadhikari, S., Best, C. A., King, N., Henderson, M., Johnson, J., Breuer, C. K., et al. (2019). Mouse Model of Tracheal Replacement with Electrospun Nanofiber Scaffolds. Ann. Otol Rhinol Laryngol. 128 (5), 391–400. doi:10.1177/0003489419826134
Elliott, M. J., Butler, C. R., Varanou-Jenkins, A., Partington, L., Carvalho, C., Samuel, E., et al. (2017). Tracheal Replacement Therapy with a Stem Cell-Seeded Graft: Lessons from Compassionate Use Application of a GMP-Compliant Tissue-Engineered Medicine. Stem Cell Translational Med. 6 (6), 1458–1464. doi:10.1002/sctm.16-0443
Elliott, M. J., De Coppi, P., Speggiorin, S., Roebuck, D., Butler, C. R., Samuel, E., et al. (2012). Stem-cell-based, Tissue Engineered Tracheal Replacement in a Child: A 2-year Follow-Up Study. The Lancet 380 (9846), 994–1000. doi:10.1016/S0140-6736(12)60737-5
Fabre, D., Fadel, E., Mussot, S., Kolb, F., Leymarie, N., Mercier, O., et al. (2015). Autologous Tracheal Replacement for cancerChinese Clinical Oncology. Chin. Clin. Oncol. 4 (Issue 4), 46. doi:10.3978/j.issn.2304-3865.2015.12.07
Fabre, D., Kolb, F., Fadel, E., Mercier, O., Mussot, S., Le Chevalier, T., et al. (2013). Successful Tracheal Replacement in Humans Using Autologous Tissues: An 8-year Experience. Ann. Thorac. Surg. 96 (4), 1146–1155. doi:10.1016/j.athoracsur.2013.05.073
Fabre, D., Singhal, S., De Montpreville, V., Decante, B., Mussot, S., Chataigner, O., et al. (2009). Composite Cervical Skin and Cartilage Flap Provides a Novel Large Airway Substitute after Long-Segment Tracheal Resection. J. Thorac. Cardiovasc. Surg. 138 (1), 32–39. doi:10.1016/j.jtcvs.2008.11.071
Fux, T., Österholm, C., Themudo, R., Simonson, O., Grinnemo, K.-H., and Corbascio, M. (2020). Synthetic Tracheal Grafts Seeded with Bone Marrow Cells Fail to Generate Functional Tracheae: First Long-Term Follow-Up Study. J. Thorac. Cardiovasc. Surg. 159 (6), 2525–2537. doi:10.1016/j.jtcvs.2019.09.185
Gallico, G. G. (1985). Permanent Coverage of Large Burn Wounds with Autologous Cultured Human Epithelium. Plast. Reconstr. Surg. 76 (5), 812. doi:10.1097/00006534-198511000-00093
Ganesan, S., Comstock, A. T., and Sajjan, U. S. (2013). Barrier Function of Airway Tract Epithelium. Tissue Barriers 1, e24997. doi:10.4161/tisb.24997
Gao, B., Jing, H., Gao, M., Wang, S., Fu, W., Zhang, X., et al. (2019). Long-segmental Tracheal Reconstruction in Rabbits with Pedicled Tissue-Engineered Trachea Based on a 3D-Printed Scaffold. Acta Biomater. 97, 177–186. doi:10.1016/j.actbio.2019.07.043
Gao, M., Zhang, H., Dong, W., Bai, J., Gao, B., Xia, D., et al. (2017). Tissue-engineered Trachea from a 3D-Printed Scaffold Enhances Whole-Segment Tracheal Repair. Sci. Rep. 7 (1), 1–12. doi:10.1038/s41598-017-05518-3
Genden, E. M., Gannon, P. J., Deftereos, M., Smith, S., and Urken, M. L. (2003). Microvascular Transplantation of Tracheal Allografts in the Canine Model. Ann. Otol Rhinol Laryngol. 112 (4), 307–313. doi:10.1177/000348940311200404
Genden, E. M., Gannon, P. J., Smith, S., Keck, N., Deftereos, M., and Urken, M. L. (2002). Microvascular Transfer of Long Tracheal Autograft Segments in the Canine Model. The Laryngoscope 112 (3), 439–444. doi:10.1097/00005537-200203000-00006
Genden, E. M., Miles, B. A., Harkin, T. J., DeMaria, S., Kaufman, A. J., Mayland, E., et al. (2021). Single‐stage Long‐segment Tracheal Transplantation. Am. J. Transpl. 21 (10), 3421–3427. doi:10.1111/ajt.16752
Go, T., Jungebluth, P., Baiguero, S., Asnaghi, A., Martorell, J., Ostertag, H., et al. (2010). Both epithelial Cells and Mesenchymal Stem Cell-Derived Chondrocytes Contribute to the Survival of Tissue-Engineered Airway Transplants in Pigs. J. Thorac. Cardiovasc. Surg. 139 (2), 437–443. doi:10.1016/j.jtcvs.2009.10.002
Goldfarbmuren, K. C., Jackson, N. D., Sajuthi, S. P., Dyjack, N., Li, K. S., Rios, C. L., et al. (2020). Dissecting the Cellular Specificity of Smoking Effects and Reconstructing Lineages in the Human Airway Epithelium. Nat. Commun. 11 (1). doi:10.1038/s41467-020-16239-z
Gonfiotti, A., Jaus, M. O., Barale, D., Baiguera, S., Comin, C., Lavorini, F., et al. (2014). The First Tissue-Engineered Airway Transplantation: 5-year Follow-Up Results. The Lancet 383 (9913), 238–244. doi:10.1016/S0140-6736(13)62033-4
Greaney, A. M., and Niklason, L. E. (2021). The History of Engineered Tracheal Replacements: Interpreting the Past and Guiding the Future. Tissue Eng. B: Rev. 27 (4), 341–352. doi:10.1089/ten.teb.2020.0238
Grillo, H. C. (2002). Tracheal Replacement: A Critical Review. Ann. Thorac. Surg. 73, 1995–2004. doi:10.1016/s0003-4975(02)03564-6
Hamilton, N. J., Kanani, M., Roebuck, D. J., Hewitt, R. J., Cetto, R., Culme-Seymour, E. J., et al. (2015). Tissue-Engineered Tracheal Replacement in a Child: A 4-Year Follow-Up Study. Am. J. Transplant. 15 (10), 2750–2757. doi:10.1111/ajt.13318
Heijink, I. H., Nawijn, M. C., and Hackett, T. L. (2014). Airway Epithelial Barrier Function Regulates the Pathogenesis of Allergic Asthma. Clin. Exp. Allergy 44 (5), 620–630. doi:10.1111/cea.12296
Hirsch, T., Rothoeft, T., Teig, N., Bauer, J. W., Pellegrini, G., De Rosa, L., et al. (2017). Regeneration of the Entire Human Epidermis Using Transgenic Stem Cells. Nature 551 (7680), 327–332. doi:10.1038/nature24487
Hoffman, T. M., Gaynor, J. W., Bridges, N. D., Paridon, S. M., and Spray, T. L. (2001). Aortic Homograft Interposition for Management of Complete Tracheal Anastomotic Disruption after Heart-Lung Transplantation. J. Thorac. Cardiovasc. Surg. 121 (3), 587–588. doi:10.1067/mtc.2001.110682
Iyer, S., Subramaniam, N., Vidhyadharan, S., Thankappan, K., Balasubramanian, D., Balasubramanian, K. R., et al. (2020). Tracheal Allotransplantation-Lessons Learned. Indian J. Plast. Surg. 53 (2), 306–308. doi:10.1055/s-0040-1716420
Jungebluth, P., Alici, E., Baiguera, S., Blanc, K. Le., Blomberg, P., Bozóky, B., et al. (2011). Tracheobronchial Transplantation with a Stem-Cell-Seeded Bioartificial Nanocomposite: A Proof-Of-Concept Study. The Lancet 378 (9808), 1997–2004. doi:10.1016/S0140-6736(11)61715-7
Jungebluth, P., Haag, J. C., Lim, M. L., Lemon, G., Sjöqvist, S., Gustafsson, Y., et al. (2019). Retraction Notice to:“Verification of Cell Viability in Bioengineered Tissues and Organs Before Clinical Transplantation ” [BIOMATERIALS (2013) 4057–4067]. Biomaterials 199, 88. doi:10.1016/j.biomaterials.2019.02.002
Jungebluth, P., Haag, J. C., Lim, M. L., Lemon, G., Sjöqvist, S., Gustafsson, Y., et al. (2013). Verification of Cell Viability in Bioengineered Tissues and Organs before Clinical Transplantation. Biomaterials 34 (16), 4057–4067. doi:10.1016/j.biomaterials.2013.02.057
Kim, I. G., Park, S. A., Lee, S. H., Choi, J. S., Cho, H., Lee, S. J., et al. (2020). Transplantation of a 3D-Printed Tracheal Graft Combined with iPS Cell-Derived MSCs and Chondrocytes. Scientific Rep. 10 (1), 1–14. doi:10.1038/s41598-020-61405-4
Kramish, D., and Morfit, H. M. (1963). The Use of a Teflon Prosthesis to Bridge Complete Sleeve Defects in the Human Trachea. Am. J. Surg. 106 (5), 704–708. doi:10.1016/0002-9610(63)90388-X
Kueckelhaus, M., Rothoeft, T., De Rosa, L., Yeni, B., Ohmann, T., Maier, C., et al. (2021). Transgenic Epidermal Cultures for Junctional Epidermolysis Bullosa - 5-Year Outcomes. New Engl. J. Med. 385 (24), 2264–2270. doi:10.1056/NEJMoa2108544
Lee, J. Y., Park, J. H., and Cho, D. W. (2018). Comparison of Tracheal Reconstruction with Allograft, Fresh Xenograft and Artificial Trachea Scaffold in a Rabbit Model. J. Artif. Organs 21 (3), 325–331. doi:10.1007/s10047-018-1045-2
Lee, K. S., and Yang, C. C. (2001). Tracheal Length of Infants under Three Months Old. Ann. Otology, Rhinology Laryngol. 110 (3), 268–270. doi:10.1177/000348940111000312
Levashov, Y. U., Yablonsky, P. K., Cherny, S. M., Orlov, S. V., Shafirovsky, B. B., and Kuznetzov, I. M. (1993). One-stage Allotransplantation of Thoracic Segment of the Trachea in a Patient with Idiopathic Fibrosing Mediastinitis and Marked Tracheal Stenosis. Eur. J. Cardio-Thoracic Surg. 7 (7), 383–386. doi:10.1016/1010-7940(93)90071-I
Longmire, W. P. (1948). The Repair of Large Defects of the Trachea. Ann. Otology, Rhinology, Laryngol. 57 (3), 875–883. doi:10.1177/000348944805700322
Lovett, M., Lee, K., Edwards, A., and Kaplan, D. L. (2009). Vascularization Strategies for Tissue Engineering. Tissue Eng. - B: Rev. 15 (3), 353–370. doi:10.1089/ten.teb.2009.0085
Macchiarini, P., Jungebluth, P., Go, T., Asnaghi, M. A., Rees, L. E., Cogan, T. A., et al. (2008). Clinical Transplantation of a Tissue-Engineered Airway. The Lancet 372 (9655), 2023–2030. doi:10.1016/S0140-6736(08)61598-6
Machino, R., Matsumoto, K., Taniguchi, D., Tsuchiya, T., Takeoka, Y., Taura, Y., et al. (2019). Replacement of Rat Tracheas by Layered, Trachea-like, Scaffold-free Structures of Human Cells Using a Bio-3D Printing System. Adv. Healthc. Mater. 8 (7). doi:10.1002/adhm.201800983
Martínez-Hernández, N. J., Mas-Estellés, J., Milián-Medina, L., Martínez-Ramos, C., Cerón-Navarro, J., Galbis-Caravajal, J., et al. (2021). A Standardised Approach to the Biomechanical Evaluation of Tracheal Grafts. Biomolecules 11 (10), 1–12. doi:10.3390/biom11101461
Martinod, E., Chouahnia, K., Radu, D. M., Joudiou, P., Uzunhan, Y., Bensidhoum, M., et al. (2018). Feasibility of Bioengineered Tracheal and Bronchial Reconstruction Using Stented Aortic Matrices. JAMA - J. Am. Med. Assoc. 319 (21), 2212–2222. doi:10.1001/jama.2018.4653
Martinod, E., Paquet, J., Dutau, H., Radu, D. M., Bensidhoum, M., Abad, S., et al. (2017). In Vivo Tissue Engineering of Human Airways. Ann. Thorac. Surg. 103 (5), 1631–1640. doi:10.1016/j.athoracsur.2016.11.027
Mattioli, F., Marchioni, A., Andreani, A., Cappiello, G., Fermi, M., and Presutti, L. (2021). Post-intubation Tracheal Stenosis in COVID-19 patientsEuropean Archives of Oto-Rhino-Laryngology. Eur. Arch. Otorhinolaryngol. 278 (Issue 3), 847. doi:10.1007/s00405-020-06394-w
Maughan, E. F., Butler, C. R., Crowley, C., Teoh, G. Z., Hondt, M. Den., Hamilton, N. J., et al. (2017). A Comparison of Tracheal Scaffold Strategies for Pediatric Transplantation in a Rabbit Model. Laryngoscope 127 (12), E449–E457. doi:10.1002/lary.26611
Maurizi, E., Adamo, D., Magrelli, F. M., Galaverni, G., Attico, E., Merra, A., et al. (2021). Regenerative Medicine of Epithelia: Lessons from the Past and Future Goals. Front. Bioeng. Biotechnol. 9 (March). doi:10.3389/fbioe.2021.652214
Menna, C., Andreetti, C., Ibrahim, M., Ciccone, A. M., D’Andrilli, A., Maurizi, G., et al. (2021). Successful Total Tracheal Replacement by Cryopreserved Aortic Allograft in a Patient Post-COVID-19 Infection. Chest 160 (6), e613–e617. doi:10.1016/j.chest.2021.08.037
Mercier, O., Kolb, F., and Dartevelle, P. G. (2018). Autologous Tracheal Replacement: Surgical Technique and Outcomes. Thorac. Surg. Clin. 28 (3), 347–355. doi:10.1016/j.thorsurg.2018.05.007
Molins, L. (2019). Patient Follow-Up after Tissue-Engineered Airway Transplantation. The Lancet 393 (10176), 1099. doi:10.1016/S0140-6736(19)30485-4
Naito, H., Tojo, T., Kimura, M., Dohi, Y., Zimmermann, W. H., Eschenhagen, T., et al. (2011). Engineering Bioartificial Tracheal Tissue Using Hybrid Fibroblastmesenchymal Stem Cell Cultures in Collagen Hydrogels. Interactive Cardiovasc. Thorac. Surg. 12 (2), 156–161. doi:10.1510/icvts.2010.253559
Neville, W. E., Bolanowski, P. J. P., and Kotia, G. G. (1990). Clinical Experience with the Silicone Tracheal Prosthesis. J. Thorac. Cardiovasc. Surg. 99 (4), 604–613. doi:10.1016/s0022-5223(19)36932-6
Neville, W. E., Bolanowski, P. J., and Soltanzadeh, H. (1976). Prosthetic Reconstruction of the Trachea and Carina. J. Thorac. Cardiovasc. Surg. 72 (4), 525–538. doi:10.1016/s0022-5223(19)40036-6
Niermeyer, W. L., Rodman, C., Li, M. M., and Chiang, T. (2020). Tissue Engineering Applications in Otolaryngology the State of Translation. Laryngoscope Invest. Otolaryngol. 5, 630–648. doi:10.1002/lio2.416
Olias, J., Millán, G., and da Costa, D. (2005). Circumferential Tracheal Reconstruction for the Functional Treatment of Airway Compromise. The Laryngoscope 115 (1), 159–161. doi:10.1097/01.mlg.0000150688.00635.2b
Paternoster, D. J. L., and Vranckx, P. J. J. (2021). State of the Art of Clinical Applications of Tissue Engineering in 2021
Pellegrini, G., Ardigò, D., Milazzo, G., Iotti, G., Guatelli, P., Pelosi, D., et al. (2018). Navigating Market Authorization: The Path Holoclar Took to Become the First Stem Cell Product Approved in the European Union. Stem Cell Translational Med. 7 (1), 146–154. doi:10.1002/sctm.17-0003
Pellegrini, G., Rama, P., and De Luca, M. (2011). Vision from the Right Stem. Trends Mol. Med. 17 (1), 1–7. doi:10.1016/j.molmed.2010.10.003
Pepper, V., Best, C. A., Buckley, K., Schwartz, C., Onwuka, E., King, N., et al. (2019). Factors Influencing Poor Outcomes in Synthetic Tissue-Engineered Tracheal Replacement. Otolaryngol. - Head Neck Surg. (United States) 161 (3), 458–467. doi:10.1177/0194599819844754
Piazza, C., Filauro, M., Dikkers, F. G., Nouraei, S. A. R., Sandu, K., Sittel, C., et al. (2021). Long-term Intubation and High Rate of Tracheostomy in COVID-19 Patients Might Determine an Unprecedented Increase of Airway Stenoses: a Call to Action from the European Laryngological Society. Eur. Arch. Oto-Rhino-Laryngology 278 (1), 1–7. doi:10.1007/s00405-020-06112-6
Ram-Liebig, G., Barbagli, G., Heidenreich, A., Fahlenkamp, D., Romano, G., Rebmann, U., et al. (2017). Results of Use of Tissue-Engineered Autologous Oral Mucosa Graft for Urethral Reconstruction: A Multicenter, Prospective, Observational Trial. EBioMedicine 23, 185–192. doi:10.1016/j.ebiom.2017.08.014
Randhawa, S. K., and Patterson, G. A. (2021). Single-stage Tracheal Transplantation—From Bench to Bedside. Am. J. Transplant. 21 (10), 3223–3224. doi:10.1111/ajt.16776
Rehmani, S. S., Al-Ayoubi, A. M., Ayub, A., Barsky, M., Lewis, E., Flores, R., et al. (2017). Three-Dimensional-Printed Bioengineered Tracheal Grafts: Preclinical Results and Potential for Human Use. Ann. Thorac. Surg. 104 (3), 998–1004. doi:10.1016/j.athoracsur.2017.03.051
Rose, K. G., Sesterhenn, K., and Wustrow, F. (1979). Tracheal Allotransplantation in Man. Lancet 1 (8113), 433. doi:10.1016/s0140-6736(79)90902-4
Ruiz García, S., Deprez, M., Lebrigand, K., Cavard, A., Paquet, A., Arguel, M.-J., et al. (2019)., 146. Cambridge. doi:10.1242/dev.177428Novel Dynamics of Human Mucociliary Differentiation Revealed by Single-Cell RNA Sequencing of Nasal Epithelial CulturesDevelopmentIssue 20
Sakaguchi, Y., Sato, T., Muranishi, Y., Yutaka, Y., Komatsu, T., Omori, K., et al. (2018). Development of a Novel Tissue-Engineered Nitinol Frame Artificial Trachea with Native-like Physical Characteristics. J. Thorac. Cardiovasc. Surg. 156 (3), 1264–1272. doi:10.1016/j.jtcvs.2018.04.073
Salassa, J. R., Pearson, B. W., and Payne, W. S. (1977). Gross and Microscopical Blood Supply of the Trachea. Ann. Thorac. Surg. 24 (2), 100–107. doi:10.1016/S0003-4975(10)63716-2
Schneider, P., Schirren, J., Muley, T., and Vogt-Moykopf, I. (2001). Primary Tracheal Tumors: Experience with 14 Resected Patients. Eur. J. Cardio-Thoracic Surg. 20 (1), 12–18. doi:10.1016/S1010-7940(01)00732-1
Shafiee, A., and Atala, A. (2017). Tissue Engineering: Toward a New Era of Medicine. Annu. Rev. MedicineAnnu Rev Med 68, 29–40. doi:10.1146/annurev-med-102715-092331
Soriano, L., Khalid, T., Whelan, D., O’Huallachain, N., Redmond, K. C., O’Brien, F. J., et al. (2021). Development and Clinical Translation of Tubular Constructs for Tracheal Tissue Engineering: a Review. Eur. Respir. Rev. 30 (162), 210154. doi:10.1183/16000617.0154-2021
Spaggiari, L., Calabrese, L. S., D’Aiuto, M., Veronesi, G., Galetta, D., Venturino, M., et al. (2005). Successful Subtotal Tracheal Replacement (Using a Skin/omental Graft) for Dehiscence after a Resection for Thyroid Cancer. J. Thorac. Cardiovasc. Surg. 129 (6), 1455–1456. doi:10.1016/j.jtcvs.2004.11.010
Tan, Q., Liu, R., Chen, X., Wu, J., Pan, Y., Lu, S., et al. (2017). Clinic Application of Tissue Engineered Bronchus for Lung Cancer Treatment. J. Thorac. Dis. 9 (1), 22–29. doi:10.21037/jtd.2017.01.50
Taniguchi, D., Matsumoto, K., Tsuchiya, T., MacHino, R., Takeoka, Y., Elgalad, A., et al. (2018). Scaffold-free Trachea Regeneration by Tissue Engineering with bio-3D Printing. Interactive Cardiovasc. Thorac. Surg. 26 (5), 745–752. doi:10.1093/icvts/ivx444
Teng, Z., Trabelsi, O., Ochoa, I., He, J., Gillard, J. H., and Doblare, M. (2012). Anisotropic Material Behaviours of Soft Tissues in Human Trachea: An Experimental Study. J. Biomech. 45 (9), 1717–1723. doi:10.1016/j.jbiomech.2012.04.002
The Lancet Editors (2018). Retraction—Tracheobronchial Transplantation with a Stem-Cell-Seeded Bioartificial Nanocomposite: a Proof-Of-Concept Study. The Lancet 392 (10141), 11. doi:10.1016/S0140-6736(18)31558-7
The Lancet, (2018). The Final Verdict on Paolo Macchiarini: Guilty of Misconduct. The Lancet 392 (10141), 2. doi:10.1016/S0140-6736(18)31484-3
Toomes, H., Mickisch, G., and Vogt-Moykopf, I. (1985). Experiences with Prosthetic Reconstruction of the Trachea and Bifurcation. Thorax 40 (1), 32–37. doi:10.1136/thx.40.1.32
Udelsman, B., Mathisen, D. J., and Ott, H. C. (2018). A Reassessment of Tracheal Substitutes-A Systematic Review. Ann. Cardiothorac. Surg. 7 (2), 175–182. doi:10.21037/acs.2018.01.17
Vranckx, J. J., and Hondt, M. Den. (2017). Tissue Engineering and Surgery: From Translational Studies to Human Trials. Innovative Surg. Sci. 2 (4), 189–202. doi:10.1515/iss-2017-0011
Wurtz, A., Porte, H., Conti, M., Desbordes, J., Copin, M. C., Azorin, J., et al. (20061940). Tracheal Replacement with Aortic Allografts. New Engl. J. Med. 355 (18), 1938. doi:10.1056/NEJMc066336
Wurtz, A., Porte, H., Conti, M., Dusson, C., Desbordes, J., Copin, M.-C., et al. (2010). Surgical Technique and Results of Tracheal and Carinal Replacement with Aortic Allografts for Salivary Gland-type Carcinoma. J. Thorac. Cardiovasc. Surg. 140 (2), 387–393. e2. doi:10.1016/j.jtcvs.2010.01.043
Yu, P., Clayman, G. L., and Walsh, G. L. (2006). Human Tracheal Reconstruction with a Composite Radial Forearm Free Flap and Prosthesis. Ann. Thorac. Surg. 81 (2), 714–716. doi:10.1016/j.athoracsur.2004.12.009
Zaragosi, L. E., Deprez, M., and Barbry, P. (2020). Using Single-Cell RNA Sequencing to Unravel Cell Lineage Relationships in the Respiratory Tract. Biochem. Soc. Trans. 48 (1), 327–336. doi:10.1042/BST20191010
Zhang, S., and Liu, Z. (2015). Airway Reconstruction with Autologous Pulmonary Tissue Flap and an Elastic Metallic Stent. World J. Surg. 39 (8), 1981–1985. doi:10.1007/s00268-015-3066-9
Keywords: tracheal replacement, tracheal surgeries, clinical outcomes, preclinical studies, regenerative medicine, tissue engineering, allotransplantation
Citation: Adamo D, Galaverni G, Genna VG, Lococo F and Pellegrini G (2022) The Growing Medical Need for Tracheal Replacement: Reconstructive Strategies Should Overcome Their Limits. Front. Bioeng. Biotechnol. 10:846632. doi: 10.3389/fbioe.2022.846632
Received: 31 December 2021; Accepted: 08 March 2022;
Published: 09 May 2022.
Edited by:
Ranieri Cancedda, Independent researcher, Genova, ItalyReviewed by:
Jerome Richard Duisit, CHU Rennes, University of Rennes, FranceMartin L Tomov, Emory University, United States
Copyright © 2022 Adamo, Galaverni, Genna, Lococo and Pellegrini. This is an open-access article distributed under the terms of the Creative Commons Attribution License (CC BY). The use, distribution or reproduction in other forums is permitted, provided the original author(s) and the copyright owner(s) are credited and that the original publication in this journal is cited, in accordance with accepted academic practice. No use, distribution or reproduction is permitted which does not comply with these terms.
*Correspondence: Graziella Pellegrini, R3JhemllbGxhLnBlbGxlZ3JpbmlAdW5pbW9yZS5pdA==