- Water-Energy Nexus Laboratory, Department of Environmental Engineering, University of Seoul, Seoul, South Korea
Biogas produced from anaerobic digestion consists of 55–65% methane and 35–45% carbon dioxide, with an additional 1–2% of other impurities. To utilize biogas as renewable energy, a process called biogas upgrading is required. Biogas upgrading is the separation of methane from carbon dioxide and other impurities, and is performed to increase CH4 content to more than 95%, allowing heat to be secured at the natural gas level. The profitability of existing biogas technologies strongly depends on operation and maintenance costs. Conventional biogas upgrading technologies have many issues, such as unstable high-purity methane generation and high energy consumption. However, hydrogenotrophs-based biological biogas upgrading offers an advantage of converting CO2 in biogas directly into CH4 without additional processes. Thus, biological upgrading through applying hydrogenotrophic methanogens for the biological conversion of CO2 and H2 to CH4 receives growing attention due to its simplicity and high technological potential. This review analyzes the recent advance of hydrogenotrophs-based biomethanation processes, addressing their potential impact on public acceptance of biogas plants for the promotion of biogas production.
1 Introduction
Over the last 2 decades, the bioenergy sector has received increasing attention, especially in the usage and production of biogas. The number of facilities producing biogas via anaerobic digestion (AD) processes has increased steadily. Germany is a leader in terms of biogas plants. Currently, about 9,000 farm-scale digesters are operating in the country (Vasco-Correa et al., 2018). In the US (U.S. EPA, 2017 & 2018), there are a total of 209 anaerobic digesters fed with food-waste and 1,250 anaerobic digesters fed with wastewater sludge. In 2017, Australia had 242 biogas plants, half of which were on landfill sites (Carlu et al., 2019). In Denmark, 150 biogas-producing plants were operating in 2015 (EBA, 2015). According to Kalyuzhnyi (2008), there were around 100 anaerobic digesters in Russia in 2008. From 2012 to 2020, the number of biogas plants in the Republic of Korea increased from 49 to 110 (Kim et al., 2012; Korean Ministry of Environment, 2020).
In China, the amount of wastes treated by the AD process increased from 21,600 tons per day in 2015 to 36,400 tons per day in 2020 (Khalid et al., 2020). Nonetheless, actual biogas production is only about 6% of the potential for China (35 Mtoe vs. 570 Mtoe), according to the International Energy Agency (IEA) (2018). In India, approximately five million small-size family biogas plants have been installed, but only 56 biogas-powered plants are operating (Mittal et al., 2018). It appears that the biogas production and usage has a great potential for development and application. Although the number of biogas plants has increased, the produced biogas has been limitedly utilized to produce electricity or heat for homes or towns in the vicinity. It is mainly because the amount of biogas produced by a plant is not large enough to supply to industrial plants and the biogas is not pure enough to directly supply to a gas grid or automobiles without further purification. Therefore, biogas upgrading to biomethane, i.e., biogas mainly consisting of methane, has recently received particular attention from biogas producers.
The composition of biogas produced during AD is around 55–65% methane and 35–45% carbon dioxide, similar to landfill gas (Oslaj et al., 2010; Nasir et al., 2012; Ounnar et al., 2012). To meet the requirements to be used as biofuel (e.g., for gas-powered vehicles), biogas must be purified to increase the methane gas content (ISO 13686:1998(en) Natural gas—Quality designation, 1998). Thus, biomethane is supplied to natural gas facilities and used directly as a raw material for energy production and the chemical industry.
Biogas upgrading aims to remove or separate the carbon dioxide and other impurities from the biogas to achieve a methane content of up to 95%, thereby securing heat at the natural gas level and further utilization as a fuel (Sun et al., 2015). One of the purposes of biogas upgrading is to make biogas a stable energy source and an alternative to fossil fuels (Lecker et al., 2017). Additionally, the upgraded biogas can be injected directly into existing gas pipelines with no extra processes required. However, issues such as unstable production of high-purity methane gas, high operation costs, large facility size, and high energy consumption during the upgrading process are still a challenge in biogas upgrading that must be resolved (Ahern et al., 2015; Adnan et al., 2019).
The application of conventional biogas upgrading includes many scrubber processes that utilize water or amine as an absorbent or use pressure swing adsorption and membrane separation (Angelidaki et al., 2018; Struk et al., 2020; Nguyen et al., 2021). Although the membrane-based upgrading process has high energy efficiency and is easy to operate and maintain, additional capital investment is required for the installation of compressors, membrane modules, heat exchangers, and off-gas treatment devices (Angelidaki et al., 2018; Nguyen et al., 2021). Furthermore, a large amount of energy is required to achieve a high level of purity of methane, which is the issue to maintain operating costs in an acceptable range (Angelidaki et al., 2018; Nguyen et al., 2021).
In addition, physical condensation and chemical adsorption or absorption methods are applied mostly to remove moisture, H2S, ammonia, and other trace elements. To remove CO2 from biogas, additional technologies (e.g., chemical absorption, water scrubbing, cryogenic separation, membrane separation, or pressure separation) are necessary (Ryckebosch et al., 2011; Muñoz et al., 2015; Awe et al., 2017). The use of physical and chemical methods has many disadvantages, including, but not limited to, high energy consumption, difficult operation, CH4 loss during purification, and a high cost of investment and operation (Awe et al., 2017). Compared to those technologies, biological upgrading technologies overcome these problems (Khan et al., 2021).
With specific microorganisms known as hydrogenotrophic methanogens, conversion of CO2 into CH4 is possible, allowing an increase in CH4 content of up to 95% and meeting natural gas standards (ISO 13686:1998(en) Natural gas—Quality designation, 1998). Recent research has demonstrated that hydrogenotrophs-based biological methanation (HBM) could be a promising technology for biogas upgrading (Singhal et al., 2017; Adnan et al., 2019). In fact, HBM has been demonstrated to be the most effective way of converting excess electricity into natural gas to avoid energy losses (Lecker et al., 2017). Based on the findings, Luo and Angelidaki (2012) proposed excessive hydrogen utilization via biological biogas upgrading. The study by Adnan et al. (2019) has reviewed different biogas upgrading techniques and found HBM as a good potential for sustainability, cost-effectiveness, and environmental impact, although the development of biological upgrading is still in its early stage.
However, due to its novelty, there are just a few case studies concerning biological methane upgrading in large-scale systems (IEA Bioenergy, 2018; Jensen et al., 2018; Lebranchu et al., 2019). Additionally, since HBM is a developing technology, there are only a few studies focusing on review of the biological upgrading processes (Lecker et al., 2017; Zabranska and Pokorna, 2018; Voelklein et al., 2019; Fu et al., 2020).
This review examines biogas upgrading systems utilizing hydrogenotrophic methanogens. For the first time, this review explores the microbial pathways of hydrogenotrophic methanogens involved in the biogas upgrading to biomethane. The pros and cons of the different biogas-upgrading system configurations are analyzed, along with methods to improve H2 transfer and the operational conditions. Perspectives for the improvement of public acceptance of biogas production are discussed, and directions for future research are suggested.
2 Biogas Upgrading via Hydrogenotrophic Methanogens
Biogas upgrading, as a way of increasing methane content in biogas, is performed by 1) removing CO2 and other trace gas components (water vapor, siloxane, hydrogen sulfide, ammonia, oxygen, nitrogen) from biogas through the additional physical/chemical processes attached to the AD process (Muñoz et al., 2015; Awe et al., 2017; Adnan et al., 2019); 2) by converting CO2 from biogas to methane (Lecker et al., 2017; Adnan et al., 2019; Fu et al., 2020). Hydrogenotrophs-based biological biogas upgrading technologies are performed by converting CO2 in biogas to methane and utilizing specific microorganisms called hydrogenotrophic methanogens [e.g., Methanosarcina barkeri, Methanogenium frittonii, Methanomicrobium mobile (Dworkin et al., 2006); Methanothermus fervidus; Methanobacterium bryantii; Methanothermobacter thermautotrophicus (McGenity et al., 2010)]. Due to the technical simplicity, the technologies have been widely utilized. Biological upgrading is attractive in terms of 1) biogas purification; 2) an environment-friendly technology by capturing CO2 and converting it to CH4, a source for electricity production; 3) simplicity; and 4) easy operation (Fu et al., 2020). Nonetheless, there are a number of issues to be resolved for wide applications of the technology. In this section, technical aspects of the hydrogenotrophs-based biological biogas upgrading are reviewed and discussed.
2.1 Microbial Pathways for Biogas Upgrading and HBM System Configurations
There are two main methanogens groups in the anaerobic digester—acetoclastic methanogens (AMs) (using acetic acid as a substrate), and hydrogenotrophic methanogens (HMs) (using hydrogen and carbon dioxide as substrates). Both types convert the substrates into methane (Jones et al., 1987; Tian et al., 2019; Conrad, 2020). Biological biogas upgrading primarily applies to microbial communities of HMs. Because additional processes are not necessarily required, relatively little energy is consumed compared to other technologies (Angelidaki et al., 2018). This type of methanogens can also influence the operation efficacy of an AD reactor.
HBM upgrading provides additional methane production through combining H2 and CO2 via the metabolic pathway under either mesophilic or thermophilic conditions (Ahern et al., 2015; Guneratnam et al., 2017; Zabranska and Pokorna, 2018). The principal schematic of biological pathways of the process is presented in Figure 1. When using microorganisms, usually both AMs and HMs are applied to produce high-purity biomethane. The primary reaction pathways of HBM include 1) conversion of CO2 and H2 to CH4 by HMs (Eqs 1, 2) methanation of acetic acid by AM activity (Eqs 2, 3) generation of acetic acid through a homoacetogenesis reaction due to the high substrate activity (the Wood-Ljungdahl pathway) (Eq. 3), as shown in the following equations (Thauer et al., 1977; Ragsdale and Pierce, 2008).
Hydrogenotrophs-based biological methanation is classified into in-situ, ex-situ, or hybrid biogas upgrading, depending on the reactor configuration and the injection of H2 and CO2 (Lecker et al., 2017). Figure 2 demonstrates the in-situ, ex-situ, and hybrid processes of HBM. For in-situ biogas upgrading, H2 gas is injected directly into an AD reactor and converted to methane by HMs, along with CO2. Together, AMs convert volatile fatty acids (VFAs) into methane in the same reactor (Lecker et al., 2017; Angelidaki et al., 2018). On the other hand, in ex-situ biogas upgrading, H2, CO2, and CH4 gases are injected at a separate or stand-alone reactor with a single culture of HMs (Lecker et al., 2017; Angelidaki et al., 2018). Although the ex-situ process overcomes various biological and mechanical challenges, separate reactor construction is required, which might be undesirable for plants with limited space.
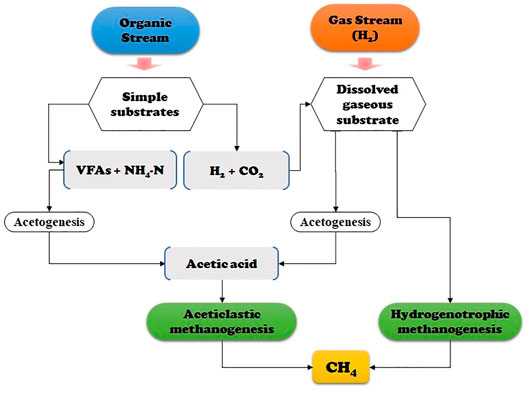
FIGURE 1. Schematic diagram of the acetate-oxidizing bacteria assisted metabolic pathway via H2-substrate and hydrogenotrophic methanogenesis.
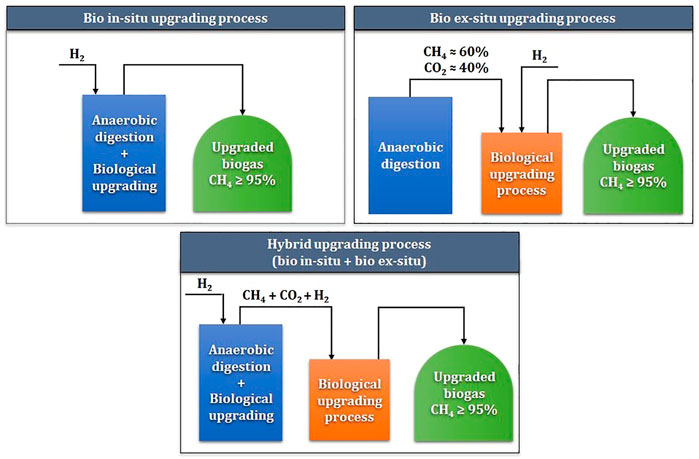
FIGURE 2. Conceptual diagram of in-situ, ex-situ, and hybrid biological hydrogen methanation processes.
Hybrid systems combining both in-situ and ex-situ technologies can be achieved for HBM. These biological biogas upgrading technologies combine technological advantages and avoid the disadvantages of a purely in-situ or ex-situ process. The hybrid systems can be performed as a combination of biological processes only or as a combination along with physical-chemical processes (Khan et al., 2021; Nguyen et al., 2021). Whereas hybrid systems appear the most efficient for biomethane production, high investment and complexity make the technology less attractive compared to solely in-situ or ex-situ processes.
HBM systems have the potential to produce up to 95% CH4 content in continuous hydrogen injection systems (Corbellini et al., 2018; Voelklein et al., 2019), yet the reaction between cells and gases in the biomethanation process may not occur sufficiently due to the solubility difference between H2 and CO2; the solubility of H2 in water (1.6 mg L−1; Kaye and Laby, 1986) is extremely lower, compared to that of CO2 (1.7 g L−1). In addition, the accumulation of VFAs in the system may decrease pH and cause the reactor to be disturbed (Corbellini et al., 2018). Thus, H2 supply to the system needs to be adjusted optimally to maintain the metabolic pathways of biological upgrading. Advantages and disadvantages of HBM system configurations are analyzed in the following sections.
2.2 Pros and Cons of In-Situ Biogas Upgrading Technologies
In in-situ HBM upgrading process H2 is supplied directly into the AD reactor. Depending on operational conditions, reactor configurations, and the substrates supplied, the AD reactors vary widely, creating a great variety of upgrading technologies. Furthermore, additional system constructions are not required for the in-situ process and essential CO2 and H2 are generated during the AD process. Despite various benefits to in-situ upgrading, the main drawback is difficulties in supplying the additional H2 to maintain the proper H2/CO2 ratio and finding the best way to supply H2 to increase gas-liquid transfer. Other issues with in-situ biogas upgrading include 1) the low solubility of H2; 2) changes in the microbial pathway from the addition of H2, causing operational issues or even reactor failure if not properly controlled; 3) CO2 depletion and pH increase through excessive H2 input (Corbellini et al., 2019).
An HBM method of biological binding of H2 is presented in a study by Luo et al. (2012), specifically a process of converting CO2 into CH4 through the supply of H2 as an electron donor by HMs. A biological methanation study with an in-situ lab-scale reactor performed by Luo and Angelidaki (2012) demonstrated that the CH4 generation rate during the stabilization period of 10 days was 1.5–5.3 L Lreac−1 d−1, rising to 6–24 L Lreac−1 d−1 as the amount of H2 injection was increased. Nonetheless, the CH4 content was maintained at 90–95% all the time. A CH4/H2 yield of 0.23, which is slightly lower than the theoretical CH4/H2 yield of 0.25, was reported, suggesting that most of the supplied H2 was used to produce CH4. Since the operation system was thermophilic, the reactor was maintained at 55°C, with pH maintained at around 7.8, while mixing at 500–800 rpm was supplied to the reactor (Luo and Angelidaki, 2012). Methanobacteriales was found the dominant order in the microbial community, with 90% CH4 content. The study also demonstrated that the gas-liquid mass conversion of H2 would limit the performance of HBM, thereby obtaining the conversion rate of 130 ml H2 min−1, which corresponds to the H2 injection rate of up to 24 L Lreactor−1 d−1.
2.2.1 Advance of H2 Transfer
As aforementioned, the low solubility of H2 limits its availability for microbial reactions in biological upgrading. One of the ways to overcome this issue is to improve the gas-liquid transfer through changing reactor configurations such as increase of gas pressure and by the installation of proper H2 diffusion systems. Different studies investigated different diffusers to improve the H2 transfer. For example, Bassani et al. (2016) applied upgrading to the up-flow anaerobic sludge blanket (UASB) thermophilic AD reactor treating potato-starch wastewater. Results indicated that the CO2 content in the biogas decreased from 42 to 10%, and that the final biogas was upgraded from 58 to 82% CH4 content. This was achieved by distributing H2 through a metallic diffuser, followed by a ceramic sponge in a separate chamber, and by having a volume of 25% of the reactor, and by applying a mild gas recirculation. Lebranchu et al. (2019) carried out a study on the HBM process, in which they used a 100 L pilot-scale digester with a dense membrane for H2 injection. In their study, residual H2 from high H2 injection flow rates was found at the digester outlet, indicating a transport limit caused by dissolved CO2 rather than by H2 mass transfer. In addition, their study regarding the effects of agitation rate on gas-flow rates revealed that, although hydrogen transfer was improved by its injection into the membrane, it was still highly affected by the agitation rate.
Recently, membrane diffusers have been applied to supply H2 gas to an HBM process. Especially, a hollow fiber membrane (HFM) has been applied for AD processes for the purpose of dissolving more H2 in the mixed liquor and producing more biomethane (Luo and Angelidaki, 2013; Wang et al., 2013; Alfaro et al., 2019). The newly developed system consisting of simultaneous coke oven gas (COG) biomethanation and in-situ biogas upgrading, showed high final CH4 content levels, up to 98–99% (Wang et al., 2013). The direct injection of COG, consisting of 92% H2 and 8% CO, into the anaerobic reactor through an HFM appears to be a highly efficient way of treating sewage sludge at a controlled pH of 8, with no apparent negative effects. However, the addition of COG could influence the structures of both the bacteria and archaea communities in the liquid.
Several studies revealed that the biofilm formed on the membrane contributed to the biological conversion of H2 and CO2 to CH4 (Luo and Angelidaki, 2013; Wang et al., 2013; Alfaro et al., 2019). Notably, their studies found that the majority of H2 was still utilized by microorganisms in the liquid. A study by Luo and Angelidaki (2013) demonstrated an increase in the generation of CH4, up to 96% in the upgraded biogas, and another study showed an increase of CH4 production by 42% compared to conventional digestion (Alfaro et al., 2019). The effect of biofilm on CH4 production through the biological conversion of H2 and CO2 needs further evaluation in terms of mechanisms, operational conditions, and environmental factors.
Table 1 summarizes the efficiency of in-situ systems with different operating conditions. In-situ HBM systems are based on the type of substrate and diffuser, operational temperature, pH, and H2 supply rate. Among different systems, the final CH4 content in the biogas was highest from the use of an HFM diffuser, taking it to 98.8% CH4 content (Luo and Angelidaki, 2013; Wang et al., 2013), indicating that H2 transfer can be successfully improved by the installation of an HFM diffuser. However, given that the in-situ reactor is operated with a mixed culture of AMs and HMs, a negative impact on gas transfer to the HMs is predicted, caused by the presence of highly concentrated organic waste and their derivatives (e.g., VFAs).
There have been inconsistent results with regard to glucose stability. A final methane content of 94.5% appeared in a batch study with glucose stability, while much lower methane contents were reported in other studies (Wahid et al., 2019). Such an issue could be overcome by moving the HM culture to a separate reactor to use ex-situ bio-upgrading. The lowest CH4 generation was shown when using grass as a substrate and a fish stone diffuser at pH 8 (Voelklein et al., 2019). Notably, the methane evolution rate was highest at 2.52 L L−1 d−1 with a ceramic diffuser and a grass substrate, compared to other types of diffusers used with the same type of reactor.
2.2.2 Operational Considerations
Other issues with in-situ biological upgrading include the effects of H2 supply on microbial pathways and changes in reactor performance. Because the AD process requires anaerobic microorganisms for organics conversion to methane, the efficacy of the AD process essentially relies on the structure of the microbial communities in the reactor (Mulat et al., 2017; Martínez et al., 2019). Excessive H2 supply results in CO2 depletion, which increases the pH to 8.5 or higher. As such, it could negatively influence the operation of an AD reactor because strongly alkaline conditions are not suitable for methanogens, especially AMs (Luo et al., 2012; Bassani et al., 2015; Mulat et al., 2017; Wahid et al., 2019; Van et al., 2020).
Due to the complex nature of microbial composition in AD reactors, there is no single ideal microbial community used for an effective AD process and biological upgrading. In general, however, there are several dominating groups of microorganisms in an AD reactor, and their composition and ratio define the reaction pathways. HBM mainly relies on HMs, which belong to the orders Methanobacteriales, Methanococcales, Methanomicrobiales, and some representatives from Methanosarcinales, which are facultative hydrogenotrophs (Karakashev, et al., 2005; Sarmiento, et al., 2011). A more detailed taxonomic structure of HMs with some growth conditions (pH, temperature, G+C content) is summarized in Supplementary Table S1 in the Supplementary Information. Changes in dominating orders and their inner families, genera, and species ratio are caused by operational conditions, substrates, or other parameters (Luo and Angelidaki, 2012; Mulat et al., 2017; Martínez et al., 2019; Khan et al., 2022).
As a co-substrate, H2 itself has the potential to change microbial communities and influence the reactor performance, therefore, an optimum H2 supply must be achieved (Mulat et al., 2017). Thus, Luo and Angelidaki (2012) reported that the number of HMs increased with H2 addition, and the structure of the archaeal community altered. For example, in the reactor with H2 addition, a species M. thermautotrophicus, which can grow on H2, was detected. Interestingly, M. thermautotrophicus not only utilizes H2 and CO2 for methane production but also requires H2S for its growth (Strevett et al., 1995). Thus, this species can remove two contaminants from the biogas.
Wang et al. (2013) reported that H2 addition could lead to an increase of both homoacetogens and HMs. Thus, homoacetogenic bacterial genus Treponema and archaeal genus Methanosaeta dominated after H2 addition, together with HM genus Methanoculleus. In the study by Kim et al. (2013), AM genus Methanosaeta and HM genus Methanospirillum dominated the archaeal community after H2 supply.
Similar results were also reported in several studies. Martínez et al. (2019) expected HMs’ domination after H2 supply, but family Methanosaetaceae (AMs) remained the major methanogens in the reactor. However, after H2 supply, some HMs (such as genus Methanospirillum) increased in abundance. With additional H2 supply, the number of homoacetogens (such as bacterial families Clostridiaceae and Eubacteriaceae) has been shown to increase with an insignificant change in the ratio between AMs and HMs (Martínez et al., 2019).
Wu et al. (2021) reported that Methanosaeta (AMs) and Methanobacterium (HMs) were the two dominant archaeal genera. Among Methanosaeta, the major species was M. harundinacea; among Methanobacterium, the major species were M. beijingense, M. petrolearium, and M. formicicum. According to the study by Khan et al. (2022), even if hydrogenotrophic methanation was performed in the in-situ HBM, mostly by Methanomicrobiales, Methanobacteriales, and Methanosarcinales orders, the dominating species inside those groups varied according to the volume of supplied H2.
Thus, the HBM requires strict control of H2 supply to the system. When H2 supply is maintained properly, high efficiency is achieved in the conversion of CO2 to methane, and high system stability is performed. Thus, continuous monitoring of biogas composition is required, with installing an automatic gas analyzer and additional labor (Lecker et al., 2017).
2.3 Pros and Cons of Ex-Situ and Hybrid Biogas Upgrading Technologies
Both in-situ and ex-situ technologies have a few similar operational issues, such as H2 transfer and pH control. However, the ex-situ biogas upgrading is carried out in a separate system, where these issues could be resolved by applying a wider range of operational strategies for HMs. The most common way to improve the gas-liquid transfer of H2 (in almost 30 times), is the installation of a proper H2 gas diffuser (Bassani et al., 2017; Kougias et al., 2017; Voelklein et al., 2019; Ghofrani-Isfahani et al., 2021; Tang et al., 2021). Other strategies for improving the mass transfer rate are as follows. Burkhard et al. (2015) and Baransi-Karkaby et al. (2020) immobilized the microorganisms to avoid issues with gas-liquid transfer and to provide direct contact between the gas and microorganisms. Their studies resulted in 89–98% of CH4 content, respectively. Burkhard et al. (2015) found a correlation between increasing methane content by decreasing liquid recirculation rate. A recent study by Miehle et al. (2021) revealed that using a lab-scale bioreactor composed of 19 tubular dead-end membranes connected in series with a membrane pore size of 0.2 μM allowed a generation of gas with CH4 content of 99%. However, further study is needed to assess the potential for future applications.
Recent studies on ex-situ biological biogas upgrading are summarized in Table 2. In general, ex-situ processes showed higher efficiency and stability, rendering it a good option for new sites or sites with insufficient space for conventional systems construction. However, ex-situ reactor setups require a longer time for the adaptation and stabilization of microorganisms. While almost all studies were conducted on a small scale, no reviews are given on the maintenance of either the pure HM culture in an ex-situ reactor or the mixed culture of AMs and HMs in an in-situ reactor, meaning further investigation is needed.
Hybrid system combines in-situ and ex-situ upgrading methods in one system. According to the study by Corbellini et al. (2018), a hybrid biogas upgrading system with both in-situ and ex-situ methods, along with thermophilic digestion was proposed. The system was composed of CSTR for the in-situ stage and UASB reactor for the ex-situ stage. The in-situ upgrading reactor was assisted with three stainless steel diffusers (2 µM pore size), while the reactor for the ex-situ process was assisted with a ceramic membrane. Hydrogen was directly injected into the first reactor providing in-situ upgrading, and the produced gas was subsequently moved for the ex-situ upgrading process. Although increasing CH4 content of up to 95% was found, with a pH maintained at 8.3–8.5, the accumulation of VFAs was confirmed in the in-situ process upon H2 injection, requiring the periodical control of VFAs. In contrast, a stable low level of VFAs was maintained in the ex-situ upgrading reactor with the high CH4 content. Improvement of H2 transfer efficiency was shown when using a ceramic membrane in the ex-situ process (Corbellini et al., 2018). When applying the hybrid process, high hydrogen utilization was achieved (up to 98%), indicating a possible application of the hybrid process to field plants.
2.4 Full-Scale Projects for Biological Biogas Upgrading
Conventional methods for biogas upgrading, which are mainly based on physical and chemical processes, are widely investigated and applied in practice. Multiple reviews on chemical/physical biogas upgrading projects and demonstration plants are available (Vartiainen, 2016; Ahmadi, 2017; Bailera et al., 2017; Thema et al., 2019). However, biological upgrading technology is still in the early stage of development and there is scarce information concerning large-scale field studies.
Although a number of studies have explored HBM, only a few pilot or full-scale applications have been tried. Some of the full-scale investigations are summarized. First, the MicrobEnergy Company conducted research on the in-situ biological biogas upgrading by adding H2 to an AD reactor in Schwandorf, Germany (Benjaminsson et al., 2013), increasing the methane content from 50 to 75%.
The BioCat project in Avedøre, Denmark, achieved 97% of methane content via biological upgrading technology in their demonstration unit of 4.8 m3 reactor volume (Power-to-Gas via Biological Catalysis, 2017). An ex-situ demonstration plant operated in Germany (IEA Bioenergy, 2018) showed 30 m3 of raw biogas per hour with 98% of methane content. In the study, a CSTR methanation tank with a working volume of 5 m3 was operating at a working temperature of 50–80°C and an internal pressure of 5–15 bar.
Jensen et al. (2018) investigated a special way of improving liquid-gas transfer. In their study, they applied a venturi-type injection system to a full-scale thermophilic digester, treating manure with in-situ HBM. The consumption rate of H2 in the study varied from 10 to 26%, indicating an incomplete reaction for CO2 conversion. This resulted in CH4 upgrading to 0.17–1.34% only, while potential upgrading could be 7%. Recirculation of the gas in the headspace of the reactor increased the consumption of H2. However, further investigation is necessary to evaluate the H2 injection method.
Store&Go project revealed three biogas upgrading operation sites. One of them, located in Solothurn, Switzerland, used HBM technology (Schlautmann et al., 2021). H2 was provided by proton exchange membrane electrolysis and CO2 was transported from a nearby wastewater treatment plant via pipeline. CO2 and H2 were converted to CH4 in a bubble-stirred column bioreactor with a temperature at 62°C and a pressure at 11 bar. The produced biomethane (more than 99% CH4 content) was injected into the urban gas distribution grid.
Despite its potential, further investigations of the biological upgrading technology are required, particularly for full-scale plants. Despite its long history and environmental and economic benefits, biogas/biomethane usage is still not widely considered as a sustainable energy source. Given the positive results of existing studies and the high technological potential of the HBM method, promoting biogas and biomethane production and usage is still encouraged.
3 HBM Upgrading as a Method to Promote Biogas Plants and Public Acceptance
Globally, there is growing awareness of greenhouse gas emissions, sustainable energy production, and the environmental impacts of fuel combustion among the public. However, it appears most countries do not consider biogas and biomethane as a sustainable energy source. The public acceptance of biogas varies among different countries and sometimes among neighborhoods, revealing different attitudes to biogas production and usage (Liu et al., 2013; Kemausuor et al., 2018; Xue et al., 2020).
The EU has led the use of upgraded biogas (IEA, 2018), and almost 500 conventional upgrading plants are operating in the EU as of 2019 (IEA, 2019; Schmid et al., 2019). There are conventional biogas upgrading plants in the United States, China, Canada, Brazil, South Korea, and Japan (Moon et al., 2019a; Moon et al., 2019b; IEA, 2019; Schmid et al., 2019; CIBiogás, 2021). On another hand, in areas with not developed or developing biogas markets, such as Eastern Europe, Balkan region, Central Asia, India, Australia, Latin America, Sub-Saharan Africa, the attitude to biogas usage usually varies from neutral to negative. More information about the status of biogas acceptance in selected countries and regions is summarized in Supplementary Table S2.
As shown from this maldistribution of the plants, still biogas plants are not widely applied, despite the apparent environmental and economic benefits of using biogas and upgraded biogas, which can be attributed to still lower public acceptance. Such lower public acceptance appears due to multiple reasons (e.g., improper standardization for biogas/biomethane quality, poor infrastructure, improper management), but the main limitation for biogas promotion is poor quality of produced biogas compared to natural gas (Pollmann et al., 2014; Garfí et al., 2016; Moreda, 2016; Kemausuor et al., 2018; Carlu et al., 2019; Mittal et al., 2019; Singh et al., 2019; Martinov et al., 2020; Abilmazhinov et al., 2021; Kuzhel et al., 2021; Zainutdinova et al., 2021). Thus, improved performance of biogas plants with higher methane gas output via applying HBM technology can significantly increase biogas plants promotion.
In areas with negative experience in biogas production in the past, HBM can be a good option to improve public and authorities’ attitude to biogas. For example, due to the energy crisis, there was the intensive implementation of AD reactors in the Latin America region in the 1970s (Garfí et al., 2016; Díaz-Vázquez et al., 2020). However, poor performance due to financial and technical issues, resulted in low support and most of those digesters stopped operating (Pérez et al., 2014; Garfí et al., 2016). Since Latin governments plan to promote small-size anaerobic digesters (Garfí et al., 2016), conventional upgrading systems are infeasible. Thus, by applying HBM upgrading it is possible to improve the performance of planned biogas plants, possibly increasing the public acceptance and supporting biogas plants.
In addition, HBM can be directly beneficial for the biogas market in developing green energy markets. Nagel and von Blottnitz (2021) stated that the construction and operation of large-scale (>1 MW) biomethane plants is more feasible in immature biogas markets due to higher energy values produced, followed by higher profits. In this case, due to lower capital investment, easier operation, and smaller size, the HBM becomes more attractive upgrading technology for large-scale sites compared to conventional systems.
Additionally, HBM biogas upgrading is considered a way to promote the developed biogas markets. For example, in Germany, where a significant amount of the biogas is produced from small- or medium-scale AD plants in rural areas, around 87% of upgrading facilities are performed on a large-scale (Daniel-Gromke et al., 2018). Even though farmers show positive attitude to biogas and biomethane plants (Emmann et al., 2013; Liu et al., 2013; Zemo et al., 2019), they tend to support only small and medium-scale plants (Zemo and Termansen, 2018; Zemo et al., 2019). Thus, due to its compactness HBM is considered the most optimal way to upgrade biogas on those sites (Daniel-Gromke et al., 2018; Liebetrau et al., 2021). Additionally, since HBM can be emphasized as a carbon-neutral process, additional economic and environmental benefits are foreseen, especially for regions with strict environmental policies.
Moreover, by the development and application of HBM upgrading technology, it is possible to make biogas/biomethane the most sustainable green energy source. Since biogas is produced from organic wastes, and upgrading can replace natural gas, it is considered to be the perfect green and sustainable technology. Nonetheless, government policies together with public support are critical for the development of the biogas and biomethane plants (Kapoor et al., 2019; Nevzorova and Karakaya, 2020; Xue et al., 2020). A potential solution would be for governments and businesses to develop a business model for biogas utilization, which focuses on generating profits from gas and electricity and the sale of organic waste. It is possible to upgrade biogas to biomethane to inject in existing gas lines or to sell it as CNG for transportation and cooperation with city gas. As a proposed utilization business model, a fuel cell (Solid Oxide Fuel Cell, SOFC) profit model can be constructed for the supply of fuel for transportation by electricity and hydrogen reforming, as shown in Figure 3.
HBM biogas upgrading appears to be successfully applied in mature and immature biogas markets, in small, medium, and large-scale systems, in developed and developing regions. With the profits received from HBM technology application and the resultant support of the biogas and biomethane plants, it is possible to establish biomethane as a sustainable energy source.
4 Future Research Outlook
Biomethane is an attractive energy source when considering reducing climate change, developing zero-carbon policies and increasing economic profits from bioenergy. In most regions there is a positive attitude from locals towards biogas and biomethane usage. Nevertheless, several issues, including a lack of clear governmental policies and regulations; a limited number of technical experts; insufficient public education, and local construction issues, create hurdles for technology applications. More research is directed towards investigating biological biogas upgrading technologies, and positive propaganda of green energy such as biomethane is being used to convince residents and businesses of its benefits. Based on the literature, several future research directions have been identified, including 1) the effect of biofilm on CH4 generation in biogas upgrading systems; 2) performance evaluation of hybrid biogas upgrading; and 3) life cycle assessments of biogas upgrading.
Few studies have investigated if biofilm influences the performance of CH4 generation in biogas upgrading systems, especially the pathways and operating factors responsible for increasing CH4 production in biogas upgrading. One of the issues found in AD is low mass transfer efficiency (Matsumoto et al., 2012). To overcome the low mass transfer efficiency between the substrate and microorganisms, different types of fibrous biofilm carriers were tested in a study by Liu et al. (2017).
Among four types of fibrous biofilm carriers—polypropylene, polyester, polyamide, polyurethane fiber material—the polypropylene biofilm carrier system produced more biogas (∼45%) and methane (∼50%), compared to the control system (Liu et al., 2017). Similarly, polypropylene was seen to influence the start-up of methanogenic biofilm reactors, producing the highest biofilm concentrations with the highest removal of chemical oxygen demand and organic loading rate in anaerobic biofilm reactors (Habouzit et al., 2014). Despite ongoing research on biogas upgrading and the effects of biofilm on the AD performance, limited information has been shared about the effects of biofilm on CH4 generation in biogas upgrading.
While this review focused mainly on in-situ and ex-situ biological hydrogen methanation, especially recent updates on developments and prospects, a hybrid biogas upgrading system has not been studied enough and future research is needed to develop the technology, especially that which takes advantage of both in-situ and ex-situ biogas upgrading. While experimental data encourage further development of a hybrid system (95% CH4 content in the study of Corbellini et al., 2018), further pilot-scale studies are recommended to demonstrate applicability to the field. Analysis of the technology and its costs is also required as studies on a hybrid upgrade are limited to lab-based experimental or concept stages.
Lastly, there is limited information assessing in-situ vs. ex-situ biogas upgrading systems from case studies. Life cycle assessment (LCA) is a useful tool for assessing environmental impact. In a study by Starr et al. (2012), three biogas upgrading technologies (high-pressure water scrubbing (HPWS); alkaline with regeneration (AwR); and bottom ash upgrading (BABIU) were assessed using LCA. It was observed that, compared to water scrubbing, a higher impact on all LCA categories (global warming potential (GWP); eutrophication potential (EP); photochemical ozone creation potential (POCP) was found with AwR, whereas low GWP was found from AwR and BABIU through capturing and storing CO2 emissions (Starr et al., 2012). More studies on LCA as a tool for assessing biogas upgrading via HBM could assist in developing cost-effective and highly efficient biogas upgrading technologies for producing CH4.
5 Conclusion
Recently, the biogas conversion to a high-quality biomethane has been a strategic target in many countries. Although physical/chemical upgrading methods are at a high level of technological readiness, their wide application is limited. Biological upgrading via the HBM process is a new technology that creates new prospects for integrating different forms of renewable energy, including upgrading advances in energy storage and decoupling bioenergy production from biomass availability.
As far as the physical/chemical upgrade process is concerned, refining and upgrading processes in biogas production account for 60–70% of the total costs. As such, stabilizing the process for a long time and solving issues (e.g., methane concentration and efficacy of impurity removal) are essential. Further technological development is necessary to solve issues such as CH4 loss, environmental impact, maintenance costs, energy consumption in the separation process, CO2 separation conditions for solidification, and optimization to maintain appropriate partial pressure.
Recent research has been directed towards biogas upgrading using the HBM process. Upgrading via the HBM process is considered to be a low-cost, highly efficient way to upgrade biogas, and because CH4 content has the potential to reach up to 95% concentration through this process, it is possible to reduce CH4 purification costs by replacing existing technologies with biological biogas upgrading. The method, which uses HMs, consumes CO2 and H2 from the AD process and from outsourcing. As such, it consumes relatively little energy and has low costs.
However, an issue with an inefficient conversion rate of the gas to liquid during the H2 injection should be addressed. As long as the hydrogen economy is revitalized in the future and the H2 supply is stabilized through water electrolysis using renewable energy, the application of biogas upgrading via HBM process to field plants will be possible. With the HBM process, it is possible to create a sustainable energy source and promote biogas plants development. Despite the long history and high potential, in many regions the biogas market is undeveloped. Thus, the growing concern about renewable energy is a great opportunity to promote biogas production by biological biogas upgrading applications and to develop the green energy sector.
Author Contributions
Conceptualization, TA and SJ; methodology, TA and IL; investigation, TA and IL; writing—original draft, TA and IL; supervision, SJ and HK.
Funding
This work was supported by Korea Environment Industry and; Technology Institute (KEITI) through Post Plastic, a specialized program of the Graduate School funded by Korea Ministry of Environment (MOE) and the Korea Institute of Energy Technology Evaluation and Planning (KETEP) grant funded by the Korea government (MOTIE) (No. 20173010092510).
Conflict of Interest
The authors declare that the research was conducted in the absence of any commercial or financial relationships that could be construed as a potential conflict of interest.
Publisher’s Note
All claims expressed in this article are solely those of the authors and do not necessarily represent those of their affiliated organizations, or those of the publisher, the editors and the reviewers. Any product that may be evaluated in this article, or claim that may be made by its manufacturer, is not guaranteed or endorsed by the publisher.
Supplementary Material
The Supplementary Material for this article can be found online at: https://www.frontiersin.org/articles/10.3389/fbioe.2022.833482/full#supplementary-material
Abbreviations
AMs, Acetoclastic Methanogens; AD, Anaerobic digestion; HBM, hydrogenotrophs-based biological methanation; COG, coke oven gas; CNG, compressed natural gas; CSTR, continuous stirred-tank reactor; EU, European Union; HFM, hollow fiber membrane; HMs, hydrogenotrophic methanogens; IBBR, immobilized biomethanation bioreactor; LCA, life cycle assessment; MBR, membrane bioreactor; MBfR, membrane biofilm reactor; Mtoe, million tonnes of oil equivalent; SAOB, syntrophic acetate-oxidizing bacteria; UASB, up-flow anaerobic sludge blanket; VFAs, volatile fatty acids.
References
Abilmazhinov, E. T., Shaiakhmetov, E. Y., Anibaev, S. N., Nurgaliyev, N. N., Shakerkhan, K. O., and Sailauov, D. M. (2021). Development of the Biogas Industry and Prospects for the Implementation of Biogas Plants in Kazakhstan. Eurasian Phys. Tech. J. 18 (337), 76–82. doi:10.31489/2021No3/76-82
Adnan, A. I., Ong, M. Y., Nomanbhay, S., Chew, K. W., and Show, P. L. (2019). Technologies for Biogas Upgrading to Biomethane: A Review. Bioengineering 6 (4), 92. doi:10.3390/bioengineering6040092
Ahern, E. P., Deane, P., Persson, T., Ó Gallachóir, B., and Murphy, J. D. (2015). A Perspective on the Potential Role of Renewable Gas in a Smart Energy Island System. Renew. Energ. 78, 648–656. doi:10.1016/j.renene.2015.01.048
Ahmadi, R. (2017). Review of CO2/H2-methanation within Power-To-Gas Processes (Doctoral Dissertation, Wien). Available at: https://repositum.tuwien.at/bitstream/20.500.12708/5712/2/Ahmadi%20Rouhollah%20-%202017%20-%20Review%20of%20CO2H2-methanation%20within%20power-to-gaspdf (Accessed on February 15, 2022).
Alfaro, N., Fdz-Polanco, M., Fdz-Polanco, F., and Díaz, I. (2019). H2 Addition through a Submerged Membrane for Iin-Ssitu Biogas Upgrading in the Anaerobic Digestion of Sewage Sludge. Bioresour. Technol. 280, 1–8. doi:10.1016/j.biortech.2019.01.135
Angelidaki, I., Treu, L., Tsapekos, P., Luo, G., Campanaro, S., Wenzel, H., et al. (2018). Biogas Upgrading and Utilization: Current Status and Perspectives. Biotechnol. Adv. 36 (2), 452–466. doi:10.1016/j.biotechadv.2018.01.011
Awe, O. W., Zhao, Y., Nzihou, A., Minh, D. P., and Lyczko, N. (2017). A Review of Biogas Utilisation, Purification and Upgrading Technologies. Waste Biomass Valor. 8 (2), 267–283. doi:10.1007/s12649-016-9826-4
Bailera, M., Lisbona, P., Romeo, L. M., and Espatolero, S. (2017). Power to Gas Projects Review: Lab, Pilot and Demo Plants for Storing Renewable Energy and CO2. Renew. Sustain. Energ. Rev. 69, 292–312. doi:10.1016/j.rser.2016.11.130
Baransi-Karkaby, K., Hassanin, M., Muhsein, S., Massalha, N., and Sabbah, I. (2020). Innovative Ex-Situ Biological Biogas Upgrading Using Immobilized Biomethanation Bioreactor (IBBR). Water Sci. Tech. 81 (6), 1319–1328. doi:10.2166/wst.2020.234
Bassani, I., Kougias, P. G., and Angelidaki, I. (2016). In-situ Biogas Upgrading in Thermophilic Granular UASB Reactor: Key Factors Affecting the Hydrogen Mass Transfer Rate. Bioresour. Technol. 221, 485–491. doi:10.1016/j.biortech.2016.09.083
Bassani, I., Kougias, P. G., Treu, L., and Angelidaki, I. (2015). Biogas Upgrading via Hydrogenotrophic Methanogenesis in Two-Stage Continuous Stirred Tank Reactors at Mesophilic and Thermophilic Conditions. Environ. Sci. Technol. 49 (20), 12585–12593. doi:10.1021/acs.est.5b03451
Bassani, I., Kougias, P. G., Treu, L., Porté, H., Campanaro, S., and Angelidaki, I. (2017). Optimization of Hydrogen Dispersion in Thermophilic Up-Flow Reactors for Ex Situ Biogas Upgrading. Bioresour. Technol. 234, 310–319. doi:10.1016/j.biortech.2017.03.055
Benjaminsson, G., Benjaminsson, J., and Rudberg, R. B. (2013). Power-to-gas: A Technical Review (P. 284). Svenskt Gastekniskt center. Available at: http://www.sgc.se/ckfinder/userfiles/files/SGC284_eng.pdf (Accessed on February 13, 2022).
Burkhardt, M., Koschack, T., and Busch, G. (2015). Biocatalytic Methanation of Hydrogen and Carbon Dioxide in an Anaerobic Three-phase System. Bioresour. Technol. 178, 330–333. doi:10.1016/j.biortech.2014.08.023
Carlu, E., Truong, T., and Kundevsk, M. (2019). Biogas Opportunities for Australia. Available at: https://apo.org.au/node/243296 (Accessed on February 7, 2022).
Chasnyk, O., Sołowski, G., and Shkarupa, O. (2015). Historical, Technical and Economic Aspects of Biogas Development: Case of Poland and Ukraine. Renew. Sustain. Energ. Rev. 52, 227–239. doi:10.1016/j.rser.2015.07.122
Chen, L., Du, S., and Xie, L. (2021). Effects of pH on Ex-Situ Biomethanation with Hydrogenotrophic Methanogens under Thermophilic and Extreme-Thermophilic Conditions. J. Biosci. Bioeng. 131 (2), 168–175. doi:10.1016/j.jbiosc.2020.09.018
CIBiogás (2021). Outlook of Biogas in Brazil 2020. (Panorama do biogás do Brasil 2020) (No. 001/2021)Available at: https://abiogas.org.br/wp-content/uploads/2021/06/PANORAMA-DO-BIOGAS-NO-BRASIL-2020-v.8.0-1_1.pdf ((In Portuguese) [Accessed on March 15, 2022).
Conrad, R. (2020). Importance of Hydrogenotrophic, Aceticlastic and Methylotrophic Methanogenesis for Methane Production in Terrestrial, Aquatic and Other Anoxic Environments: a Mini Review. Pedosphere 30 (1), 25–39. doi:10.1016/S1002-0160(18)60052-9
Corbellini, V., Catenacci, A., and Malpei, F. (2019). Hydrogenotrophic Biogas Upgrading Integrated into WWTPs: Enrichment Strategy. Water Sci. Tech. 79 (4), 759–770. doi:10.2166/wst.2019.096
Corbellini, V., Kougias, P. G., Treu, L., Bassani, I., Malpei, F., and Angelidaki, I. (2018). Hybrid Biogas Upgrading in a Two-Stage Thermophilic Reactor. Energ. Convers. Manage. 168, 1–10. doi:10.1016/j.enconman.2018.04.074
Daniel-Gromke, J., Rensberg, N., Denysenko, V., Stinner, W., Schmalfuß, T., Scheftelowitz, M., Nelles, M., and Liebetrau, J. (2018). Current Developments in Production and Utilization of Biogas and Biomethane in Germany. Chem. Ingenieur Technik 90 (1-2), 17–35. doi:10.1002/cite.201700077
Díaz-Vázquez, D., Alvarado-Cummings, S. C., Meza-Rodríguez, D., Senés-Guerrero, C., de Anda, J., and Gradilla-Hernández, M. S. (2020). Evaluation of Biogas Potential from Livestock Manures and Multicriteria Site Selection for Centralized Anaerobic Digester Systems: The Case of Jalisco, México. Sustainability 12 (9), 3527. doi:10.3390/su12093527
Dworkin, M., Falkow, S., Rosenberg, E., Schleifer, K. H., and Stackebrandt, E. (2006). The Prokaryotes, A Handbook on the Biology of Bacteria, 3. Archaea. Bacteria: Firmicutes, ActinomycetesNew York: Springer.
EBA (2015). Biomethane and Biogas Report 2015. Brussels, Belgium: European Biogas Association. Available at: https://www.europeanbiogas.eu/biogasreport2015/(Accessed May 11, 2021).
Emmann, C. H., Arens, L., and Theuvsen, L. (2013). Individual Acceptance of the Biogas Innovation: A Structural Equation Model. Energy Policy 62, 372–378. doi:10.1016/j.enpol.2013.07.083
EPA (2017). Anaerobic Digestion Facilities Processing Food Waste in the United States. & 2018Available at: https://www.epa.gov/sites/production/files/2021-02/documents/2021_final_ad_report_feb_2_with_links.pdf (Accessed May 11, 2021).
Fu, S., Angelidaki, I., and Zhang, Y. (2021). In Situ Biogas Upgrading by CO2-to-CH4 Bioconversion. Trends Biotechnol. 39, 336–347. doi:10.1016/j.tibtech.2020.08.006
Garfí, M., Martí-Herrero, J., Garwood, A., and Ferrer, I. (2016). Household Anaerobic Digesters for Biogas Production in Latin America: A Review. Renew. Sustain. Energ. Rev. 60, 599–614. doi:10.1016/j.rser.2016.01.071
Ghofrani-Isfahani, P., Tsapekos, P., Peprah, M., Kougias, P., Zhu, X., Kovalovszki, A., Zervas, A., Zha, X., Jacobsen, C. S., and Angelidaki, I. (2021). Ex-situ Biogas Upgrading in Thermophilic Up-Flow Reactors: The Effect of Different Gas Diffusers and Gas Retention Times. Bioresour. Tech. 340, 125694. doi:10.1016/j.biortech.2021.125694
Guneratnam, A. J., Ahern, E., FitzGerald, J. A., Jackson, S. A., Xia, A., Dobson, A. D. W., et al. (2017). Study of the Performance of a Thermophilic Biological Methanation System. Bioresour. Technol. 225, 308–315. doi:10.1016/j.biortech.2016.11.066
Habouzit, F., Hamelin, J., Santa‐Catalina, G., Steyer, J. P., and Bernet, N. (2014). Biofilm Development during the Start‐up Period of Anaerobic Biofilm Reactors: the Biofilm Archaea Community Is Highly Dependent on the Support Material. Microb. Biotechnol. 7 (3), 257–264. doi:10.1111/1751-7915.12115
IEA Bioenergy (2018). Biological Methanation Demonstration Plant in Allendorf, Germany - an Upgrading Facility for Biogas. IEA Bioenergy Task; 37 Oct 2018. Available at: https://www.ieabioenergy.com/wp-content/uploads/2018/11/Germany-P2G_Case-Story_LAY2.pdf (Accessed February 5, 2022).
IEA (2019). Bioenergy Task 37. Upgrading Plant List. Available at: http://task37.ieabioenergy.com/plant-list.html (Accessed on February 3, 2022).
IEA (2018). Outlook for Biogas and Biomethane. Prospects for Organic Growth. World Energy Outlook Special Report. Available at: https://iea.blob.core.windows.net/assets/03aeb10c-c38c-4d10-bcec-de92e9ab815f/Outlook_for_biogas_and_biomethane.pdf (Accessed November 9, 2021).
ISO 13686:1998(en) Natural gas ‒ Quality designation (1998). ISO 13686:1998(en) Natural gas ‒ Quality designation. Available at: https://www.iso.org/standard/22614.html (Accessed November 27, 2021).
Jensen, M. B., Kofoed, M. V. W., Fischer, K., Voigt, N. V., Agneessens, L. M., Batstone, D. J., et al. (2018). Venturi-type Injection System as a Potential H2 Mass Transfer Technology for Full-Scale In Situ Biomethanation. Appl. Energ. 222, 840–846. doi:10.1016/j.apenergy.2018.04.034
Jones, W. J., Nagle, D. P., and Whitman, W. B. (1987). Methanogens and the Diversity of Archaebacteria. Microbiol. Rev. 51 (1), 135–177. doi:10.1128/mr.51.1.135-177.1987
Kalyuzhnyi, S. (2008). Energy Potential of Anaerobic Digestion of Wastes Produced in Russia via Biogas and Microbial Fuel Cell Technologies. Pure Appl. Chem. 80 (10), 2115–2124. doi:10.1351/pac200880102115
Kapoor, R., Ghosh, P., Kumar, M., and Vijay, V. K. (2019). Evaluation of Biogas Upgrading Technologies and Future Perspectives: a Review. Environ. Sci. Pollut. Res. 26 (12), 11631–11661. doi:10.1007/s11356-019-04767-1
Karakashev, D., Batstone, D. J., and Angelidaki, I. (2005). Influence of Environmental Conditions on Methanogenic Compositions in Anaerobic Biogas Reactors. Appl. Environ. Microbiol. 71 (1), 331–338. doi:10.1128/AEM.71.1.331-338.2005
Kaye, G. W. C., and Laby, T. H. (1986). Tables of Physical and Chemical Constants. 15th ed. NY: Longman, 219.
Kemausuor, F., Adaramola, M., and Morken, J. (2018). A Review of Commercial Biogas Systems and Lessons for Africa. Energies 11 (11), 2984. doi:10.3390/en11112984
Khalid, H., Zhang, H., Liu, C., Li, W., Abuzar, M. K., Amin, F. R., et al. (2020). PEST (Political, Environmental, Social & Technical) Analysis of the Development of the Waste-To-Energy Anaerobic Digestion Industry in China as a Representative for Developing Countries. Sustain. Energ. Fuels 4 (3), 1048–1062. doi:10.1039/C9SE00692C
Khan, A., Akbar, S., Okonkwo, V., Smith, C., Khan, S., Ali Shah, A., Adnan, F., Zeeshan Ijaz, U., Ahmed, S., and Badshah, M. (2022). Enrichment of the Hydrogenotrophic Methanogens for, Iin-Ssitu Biogas Up-Gradation by Recirculation of Gases and Supply of Hydrogen in Methanogenic Reactor. Bioresour. Tech. 345, 126219. doi:10.1016/j.biortech.2021.126219
Khan, M. U., Lee, J. T. E., Bashir, M. A., Dissanayake, P. D., Ok, Y. S., Tong, Y. W., Shariati, M. A., Wu, S., and Ahring, B. K. (2021). Current Status of Biogas Upgrading for Direct Biomethane Use: A Review. Renew. Sustain. Energ. Rev. 149, 111343. doi:10.1016/j.rser.2021.111343
Kim, S., Choi, K., and Chung, J. (2013). Reduction in Carbon Dioxide and Production of Methane by Biological Reaction in the Electronics Industry. Int. J. Hydrogen Energ. 38 (8), 3488–3496. doi:10.1016/j.ijhydene.2012.12.007
Kim, Y.-S., Yoon, Y.-M., Kim, C.-H., and Giersdorf, J. (2012). Status of Biogas Technologies and Policies in South Korea. Renew. Sustain. Energ. Rev. 16 (5), 3430–3438. doi:10.1016/j.rser.2012.02.075
Korean Ministry of Environment (2020). Current Status of Biogasification Facilities for Organic Waste Resources in. Available at: http://www.me.go.kr/home/web/policy_data/read.do?menuId=10265&seq=7683(In Korean) (Accessed November 3, 2021).
Kougias, P. G., Treu, L., Benavente, D. P., Boe, K., Campanaro, S., and Angelidaki, I. (2017). Ex-situ Biogas Upgrading and Enhancement in Different Reactor Systems. Bioresour. Technol. 225, 429–437. doi:10.1016/j.biortech.2016.11.124
Kuzhel, E. V., Talakh, L. A., and Shimchuk, O. P. (2021). Biogas - an Unconventional Energy Source and Mor In The XV International Science Conference «Trends in the development of science and practice», December 27–29, Madrid, Spain. 436 p. (p. 403). (In Russian)
Landrigan, P. J., Fuller, R., Acosta, N. J. R., Adeyi, O., Arnold, R., Basu, N. N., Baldé, A. B., Bertollini, R., Bose-O'Reilly, S., Boufford, J. I., Breysse, P. N., Chiles, T., Mahidol, C., Coll-Seck, A. M., Cropper, M. L., Fobil, J., Fuster, V., Greenstone, M., Haines, A., Hanrahan, D., Hunter, D., Khare, M., Krupnick, A., Lanphear, B., Lohani, B., Martin, K., Mathiasen, K. V., McTeer, M. A., Murray, C. J. L., Ndahimananjara, J. D., Perera, F., Potočnik, J., Preker, A. S., Ramesh, J., Rockström, J., Salinas, C., Samson, L. D., Sandilya, K., Sly, P. D., Smith, K. R., Steiner, A., Stewart, R. B., Suk, W. A., van Schayck, O. C. P., Yadama, G. N., Yumkella, K., and Zhong, M. (2018). The Lancet Commission on Pollution and Health. Lancet 391 (10119), 462–512. doi:10.1016/S0140-6736(17)32345-0
Lebranchu, A., Blanchard, F., Fick, M., Pacaud, S., Olmos, E., and Delaunay, S. (2019). Pilot-scale Biomethanation of Cattle Manure Using Dense Membranes. Bioresour. Technol. 284, 430–436. doi:10.1016/j.biortech.2019.03.140
Lecker, B., Illi, L., Lemmer, A., and Oechsner, H. (2017). Biological Hydrogen Methanation - A Review. Bioresour. Tech. 245, 1220–1228. doi:10.1016/j.biortech.2017.08.176
Liebetrau, J., Rensberg, N., Maguire, D., Archer, D., and Wall, D. (2021). Murphy, J. D. (2021). Renewable Gas–Discussion on the State of the Industry and its Future in a Decarbonised World. In IEA Bioenergy Task. 37(11).
Liu, W., Wang, C., and Mol, A. P. J. (2013). Rural Public Acceptance of Renewable Energy Deployment: The Case of Shandong in China. Appl. Energ. 102, 1187–1196. doi:10.1016/j.apenergy.2012.06.057
Liu, Y., Zhu, Y., Jia, H., Yong, X., Zhang, L., Zhou, J., et al. (2017). Effects of Different Biofilm Carriers on Biogas Production during Anaerobic Digestion of Corn Straw. Bioresour. Technol. 244, 445–451. doi:10.1016/j.biortech.2017.07.171
Luo, G., and Angelidaki, I. (2013). Co-digestion of Manure and Whey for In Situ Biogas Upgrading by the Addition of H2: Process Performance and Microbial Insights. Appl. Microbiol. Biotechnol. 97 (3), 1373–1381. doi:10.1007/s00253-012-4547-5
Luo, G., and Angelidaki, I. (2012). Integrated Biogas Upgrading and Hydrogen Utilization in an Anaerobic Reactor Containing Enriched Hydrogenotrophic Methanogenic Culture. Biotechnol. Bioeng. 109 (11), 2729–2736. doi:10.1002/bit.24557
Luo, G., Johansson, S., Boe, K., Xie, L., Zhou, Q., and Angelidaki, I. (2012). Simultaneous Hydrogen Utilization and In Situ Biogas Upgrading in an Anaerobic Reactor. Biotechnol. Bioeng. 109 (4), 1088–1094. doi:10.1002/bit.24360
Martínez, E., Sotres, A., Arenas, C., Blanco, D., Martínez, O., and Gómez, X. (2019). Improving Anaerobic Digestion of Sewage Sludge by Hydrogen Addition: Analysis of Microbial Populations and Process Performance. Energies 12 (7), 1228. doi:10.3390/en12071228
Martinov, M., Scarlat, N., Djatkov, D., Dallemand, J. F., Viskovic, M., and Zezelj, B. (2020). Assessing Sustainable Biogas Potentials-Case Study for Serbia. Biomass Conv. Bioref. 10 (2), 367–381. doi:10.1007/s13399-019-00495-1
Matsumoto, S., Ohtaki, A., and Hori, K. (2012). Carbon Fiber as an Excellent Support Material for Wastewater Treatment Biofilms. Environ. Sci. Technol. 46 (18), 10175–10181. doi:10.1021/es3020502
McGenity, T., van der Meer, J. R., and de Lorenzo, V. (2010). in Handbook of Hydrocarbon and Lipid Microbiology. Editor K. N. Timmis (Berlin: Springer), 4716.
Miehle, M., Hackbarth, M., Gescher, J., Horn, H., and Hille-Reichel, A. (2021). Biological Biogas Upgrading in a Membrane Biofilm Reactor with and without Organic Carbon Source. Bioresour. Tech. 335, 125287. doi:10.1016/j.biortech.2021.125287
Mittal, S., Ahlgren, E. O., and Shukla, P. R. (2018). Barriers to Biogas Dissemination in India: A Review. Energy Policy 112, 361–370. doi:10.1016/j.enpol.2017.10.027
Mittal, S., Ahlgren, E. O., and Shukla, P. R. (2019). Future Biogas Resource Potential in India: a Bottom-Up Analysis. Renew. Energ. 141, 379–389. doi:10.1016/j.renene.2019.03.133
Moon, H., Kwon, J., Park, H., Jeon, T., Shin, S., and Lee, D. (2019a). A Study on Establishment of Technical Guideline of the Installation and Operation for the Biogas Utilization of Transportation and City Gas: Results of the Field Investigation. J. Korea Org. Resour. Recycling Assoc. 27 (1), 77–85. (In Korean). doi:10.17137/korrae.2019.27.1.77
Moon, H., Lee, D., Kwon, J., Park, H., and Jeon, T. (2019b). “A Study on the Status of Biogas Upgrading Facility and Conversion Effciency of Biomethane in Korea,” in Proceedings of the spring Academic Presentation of the Korean Society for Waste Recycling, 108. (In Korean).
Moreda, I. L. (2016). The Potential of Biogas Production in Uruguay. Renew. Sustain. Energ. Rev. 54, 1580–1591. doi:10.1016/j.rser.2015.10.099
Mulat, D. G., Mosbæk, F., Ward, A. J., Polag, D., Greule, M., Keppler, F., et al. (2017). Exogenous Addition of H 2 for an In Situ Biogas Upgrading through Biological Reduction of Carbon Dioxide into Methane. Waste Manag. 68, 146–156. doi:10.1016/j.wasman.2017.05.054
Muñoz, R., Meier, L., Diaz, I., and Jeison, D. (2015). A Review on the State-Of-The-Art of Physical/chemical and Biological Technologies for Biogas Upgrading. Rev. Environ. Sci. Biotechnol. 14 (4), 727–759. doi:10.1007/s11157-015-9379-1
Nagel, B. M., and von Blottnitz, H. (2021). Financial Viability and Risk of Biogas and Biomethane Production in an Immature Market. Available at SSRN 3907925.
Nasir, I. M., Mohd Ghazi, T. I., and Omar, R. (2012). Anaerobic Digestion Technology in Livestock Manure Treatment for Biogas Production: a Review. Eng. Life Sci. 12 (3), 258–269. doi:10.1002/elsc.201100150
Nevzorova, T., and Karakaya, E. (2020). Explaining the Drivers of Technological Innovation Systems: The Case of Biogas Technologies in Mature Markets. J. Clean. Prod. 259, 120819. doi:10.1016/j.jclepro.2020.120819
Nguyen, L. N., Kumar, J., Vu, M. T., Mohammed, J. A. H., Pathak, N., Commault, A. S., Sutherland, D., Zdarta, J., Tyagi, V. K., and Nghiem, L. D. (2021). Biomethane Production from Anaerobic Co-digestion at Wastewater Treatment Plants: A Critical Review on Development and Innovations in Biogas Upgrading Techniques. Sci. Total Environ. 765, 142753. doi:10.1016/j.scitotenv.2020.142753
Oslaj, M., Mursec, B., and Vindis, P. (2010). Biogas Production from maize Hybrids. Biomass and bioenergy 34 (11), 1538–1545. doi:10.1016/j.biombioe.2010.04.016
Ounnar, A., Benhabyles, L., and Igoud, S. (2012). Energetic Valorization of Biomethane Produced from Cow-Dung. Proced. Eng. 33, 330–334. doi:10.1016/j.proeng.2012.01.1211
Pérez, I., Garfí, M., Cadena, E., and Ferrer, I. (2014). Technical, Economic and Environmental Assessment of Household Biogas Digesters for Rural Communities. Renew. Energ. 62, 313–318. doi:10.1016/j.renene.2013.07.017
Pollmann, O., Podruzsik, S., and Fehér, O. (2014). Social Acceptance of Renewable Energy: Some Examples from Europe and Developing Africa. Soc. Economy 36 (2), 217–231. doi:10.1556/socec.36.2014.2.5
Power-to-Gas via Biological Catalysis (P2G-Biocat)(2017). Power-to-Gas via Biological Catalysis (P2G-Biocat). Available at: https://www.grese.ch/wp-content/uploads/2017/02/09_Laurent-Lardon.pdf (Accessed on February 10, 2022).
Ragsdale, S. W., and Pierce, E. (2008). Acetogenesis and the Wood-Ljungdahl Pathway of CO2 Fixation. Biochim. Biophys. Acta (Bba) - Proteins Proteomics 1784 (12), 1873–1898. doi:10.1016/j.bbapap.2008.08.012
Ryckebosch, E., Drouillon, M., and Vervaeren, H. (2011). Techniques for Transformation of Biogas to Biomethane. Biomass and bioenergy 35 (5), 1633–1645. doi:10.1016/j.biombioe.2011.02.033
Sarmiento, F. B., Leigh, J. A., and Whitman, W. B. (2011). Genetic Systems for Hydrogenotrophic Methanogens. Methods Enzymol. 494, 43–73. doi:10.1016/B978-0-12-385112-3.00003-2
Schlautmann, R., Böhm, H., Zauner, A., Mörs, F., Tichler, R., Graf, F., et al. (2021). Renewable Power‐to‐Gas: A Technical and Economic Evaluation of Three Demo Sites within the STORE&GO Project. Chem. Ingenieur Technik 93 (4), 568–579. doi:10.1002/cite.202000187
Schmid, C., Horschig, T., Pfeiffer, A., Szarka, N., and Thrän, D. (2019). Biogas Upgrading: a Review of National Biomethane Strategies and Support Policies in Selected Countries. Energies 12 (19), 3803. doi:10.3390/en12193803
Sekoai, P. T., Engelbrecht, N., du Preez, S. P., and Bessarabov, D. (2020). Thermophilic Biogas Upgrading via Ex Situ Addition of H2 and CO2 Using Codigested Feedstocks of Cow Manure and the Organic Fraction of Solid Municipal Waste. ACS omega 5 (28), 17367–17376. doi:10.1021/acsomega.0c01725
Singh, S., Choudhary, B., Xavier, S., Roy, P., Bhagat, N., and Allen, T. (2019). Biogas Potential in India: Production, Policies, Problems, and Future Prospects. Emerging Energy Alternatives for Sustainable Environment, 1–34. doi:10.1201/9780429058271-1
Singhal, S., Agarwal, S., Arora, S., Sharma, P., and Singhal, N. (2017). Upgrading Techniques for Transformation of Biogas to Bio-CNG: a Review. Int. J. Energ. Res. 41 (12), 1657–1669. doi:10.1002/er.3719
Soland, M., Steimer, N., and Walter, G. (2013). Local Acceptance of Existing Biogas Plants in Switzerland. Energy Policy 61, 802–810. doi:10.1016/j.enpol.2013.06.111
Starr, K., Gabarrell, X., Villalba, G., Talens, L., and Lombardi, L. (2012). Life Cycle Assessment of Biogas Upgrading Technologies. Waste Manage. 32 (5), 991–999. doi:10.1016/j.wasman.2011.12.016
Strevett, K. A., Vieth, R. F., and Grasso, D. (1995). Chemo-autotrophic Biogas Purification for Methane Enrichment: Mechanism and Kinetics. Chem. Eng. J. Biochem. Eng. J. 58 (1), 71–79. doi:10.1016/0923-0467(95)06095-2
Struk, M., Kushkevych, I., and Vítězová, M. (2020). Biogas Upgrading Methods: Recent Advancements and Emerging Technologies. Rev. Environ. Sci. Bio/Technology, 101234567–101234621. doi:10.1007/s11157-020-09539-9(0123456789
Sun, Q., Li, H., Yan, J., Liu, L., Yu, Z., and Yu, X. (2015). Selection of Appropriate Biogas Upgrading Technology-A Review of Biogas Cleaning, Upgrading and Utilisation. Renew. Sustain. Energ. Rev. 51, 521–532. doi:10.1016/j.rser.2015.06.029
Tang, Q., Xu, J., Liu, Z., Huang, Z., Zhao, M., Shi, W., et al. (2021). Optimal the Ex-Situ Biogas Biological Upgrading to Biomethane and its Combined Application with the Anaerobic Digestion Stage. Energy Sourc. A: Recovery, Utilization, Environ. Effects 43 (17), 2147–2159. doi:10.1080/15567036.2019.1639851
Thauer, R. K., Jungermann, K., and Decker, K. (1977). Energy Conservation in Chemotrophic Anaerobic Bacteria. Bacteriol. Rev. 41 (1), 100–180. doi:10.1128/br.41.1.100-180.1977
Thema, M., Bauer, F., and Sterner, M. (2019). Power-to-Gas: Electrolysis and Methanation Status Review. Renew. Sustain. Energ. Rev. 112, 775–787. doi:10.1016/j.rser.2019.06.030
Tian, H., Yan, M., Treu, L., Angelidaki, I., and Fotidis, I. A. (2019). Hydrogenotrophic Methanogens Are the Key for a Successful Bioaugmentation to Alleviate Ammonia Inhibition in Thermophilic Anaerobic Digesters. Bioresour. Technol. 293, 122070. doi:10.1016/j.biortech.2019.122070
Van, D. P., Fujiwara, T., Leu Tho, B., Song Toan, P. P., and Hoang Minh, G. (2020). A Review of Anaerobic Digestion Systems for Biodegradable Waste: Configurations, Operating Parameters, and Current Trends. Environ. Eng. Res. 25 (1), 1–17. doi:10.4491/eer.2018.334
Vartiainen, V. (2016). Screening of Power to Gas Projects. Available at: http://www.neocarbonenergy.fi/wp-content/uploads/2015/03/diplomityo_vartiainen_vesa.pdf (Accessed on February 15, 2022).
Vasco-Correa, J., Khanal, S., Manandhar, A., and Shah, A. (2018). Anaerobic Digestion for Bioenergy Production: Global Status, Environmental and Techno-Economic Implications, and Government Policies. Bioresour. Technol. 247, 1015–1026. doi:10.1016/j.biortech.2017.09.004
Voelklein, M. A., Rusmanis, D., and Murphy, J. D. (2019). Biological Methanation: Strategies for Iin-Ssitu and Ex-Situ Upgrading in Anaerobic Digestion. Appl. Energ. 235, 1061–1071. doi:10.1016/j.apenergy.2018.11.006
Wahid, R., Mulat, D. G., Gaby, J. C., and Horn, S. J. (2019). Effects of H2:CO2 Ratio and H2 Supply Fluctuation on Methane Content and Microbial Community Composition during Iin-Ssitu Biological Biogas Upgrading. Biotechnol. Biofuels 12 (1), 104. doi:10.1186/s13068-019-1443-6
Wang, W., Xie, L., Luo, G., Zhou, Q., and Angelidaki, I. (2013). Performance and Microbial Community Analysis of the Anaerobic Reactor with Coke Oven Gas Biomethanation and In Situ Biogas Upgrading. Bioresour. Technol. 146, 234–239. doi:10.1016/j.biortech.2013.07.049
Wang, X., Lu, X., Yang, G., Feng, Y., Ren, G., and Han, X. (2016). Development Process and Probable Future Transformations of Rural Biogas in China. Renew. Sustain. Energ. Rev. 55, 703–712. doi:10.1016/j.rser.2015.09.097
Wilkinson, P., Smith, K. R., Beevers, S., Tonne, C., and Oreszczyn, T. (2007). Energy, Energy Efficiency, and the Built Environment. The lancet 370 (9593), 1175–1187. doi:10.1016/S0140-6736(07)61255-0
Wu, J., Jiang, B., Kong, Z., Yang, C., Li, L., Feng, B., Luo, Z., Xu, K.-Q., Kobayashi, T., and Li, Y.-Y. (2021). Improved Stability of Up-Flow Anaerobic Sludge Blanket Reactor Treating Starch Wastewater by Pre-acidification: Impact on Microbial Community and Metabolic Dynamics. Bioresour. Tech. 326, 124781. doi:10.1016/j.biortech.2021.124781
Xu, J., Bu, F., Zhu, W., Luo, G., and Xie, L. (2020). Microbial Consortiums of Hydrogenotrophic Methanogenic Mixed Cultures in Lab-Scale Ex-Situ Biogas Upgrading Systems under Different Conditions of Temperature, pH and CO. Microorganisms 8 (5), 772. doi:10.3390/microorganisms8050772
Xue, S., Song, J., Wang, X., Shang, Z., Sheng, C., Li, C., Zhu, Y., and Liu, J. (2020). A Systematic Comparison of Biogas Development and Related Policies between China and Europe and Corresponding Insights. Renew. Sustain. Energ. Rev. 117, 109474. doi:10.1016/j.rser.2019.109474
Zabranska, J., and Pokorna, D. (2018). Bioconversion of Carbon Dioxide to Methane Using Hydrogen and Hydrogenotrophic Methanogens. Biotechnol. Adv. 36 (3), 707–720. doi:10.1016/j.biotechadv.2017.12.003
Zainutdinova, A. F., Sadykova, A. R., Ilgamova, L. F., and Mukhametova, I. V. (2021). Analysis of the Prospects for the Use of Biogas in Russia. Int. J. Humanities Nat. Sci. 5-2 (56). (In Russian). doi:10.24412/2500-1000-2021-5-2-181-183
Zemo, K. H., Panduro, T. E., and Termansen, M. (2019). Impact of Biogas Plants on Rural Residential Property Values and Implications for Local Acceptance. Energy policy 129, 1121–1131. doi:10.1016/j.enpol.2019.03.008
Zemo, K. H., and Termansen, M. (2018). Farmers' Willingness to Participate in Collective Biogas Investment: A Discrete Choice experiment Study. Resource Energ. Econ. 52, 87–101. doi:10.1016/j.reseneeco.2017.12.001
Keywords: biogas upgrading, biological hydrogen methanation, hydrogenotrophic methanogens, renewable energy, biogas acceptance
Citation: Antukh T, Lee I, Joo S and Kim H (2022) Hydrogenotrophs-Based Biological Biogas Upgrading Technologies. Front. Bioeng. Biotechnol. 10:833482. doi: 10.3389/fbioe.2022.833482
Received: 11 December 2021; Accepted: 31 March 2022;
Published: 25 April 2022.
Edited by:
Nsikak U. Benson, Covenant University, NigeriaReviewed by:
Misael Sebastián Gradilla-Hernández, Monterrey Institute of Technology and Higher Education (ITESM), MexicoMerve Atasoy, Royal Institute of Technology, Sweden
Copyright © 2022 Antukh, Lee, Joo and Kim. This is an open-access article distributed under the terms of the Creative Commons Attribution License (CC BY). The use, distribution or reproduction in other forums is permitted, provided the original author(s) and the copyright owner(s) are credited and that the original publication in this journal is cited, in accordance with accepted academic practice. No use, distribution or reproduction is permitted which does not comply with these terms.
*Correspondence: Sunghee Joo, c2hqb283MUB1b3MuYWMua3I=; Hyunook Kim, aF9raW1AdW9zLmFjLmty