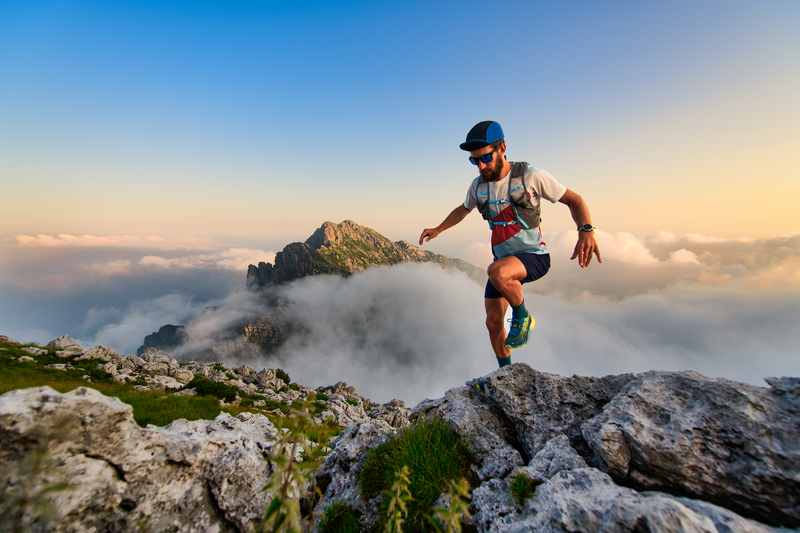
95% of researchers rate our articles as excellent or good
Learn more about the work of our research integrity team to safeguard the quality of each article we publish.
Find out more
REVIEW article
Front. Bioeng. Biotechnol. , 17 February 2022
Sec. Biomaterials
Volume 10 - 2022 | https://doi.org/10.3389/fbioe.2022.830899
This article is part of the Research Topic Tissue and Organ Decellularization Strategies in Regenerative Medicine; Recent Advances, Current Translational Challenges, and Future Directions View all 19 articles
When a tissue or an organ is considered, the attention inevitably falls on the complex and delicate mechanisms regulating the correct interaction of billions of cells that populate it. However, the most critical component for the functionality of specific tissue or organ is not the cell, but the cell-secreted three-dimensional structure known as the extracellular matrix (ECM). Without the presence of an adequate ECM, there would be no optimal support and stimuli for the cellular component to replicate, communicate and interact properly, thus compromising cell dynamics and behaviour and contributing to the loss of tissue-specific cellular phenotype and functions. The limitations of the current bioprosthetic implantable medical devices have led researchers to explore tissue engineering constructs, predominantly using animal tissues as a potentially unlimited source of materials. The high homology of the protein sequences that compose the mammalian ECM, can be exploited to convert a soft animal tissue into a human autologous functional and long-lasting prosthesis ensuring the viability of the cells and maintaining the proper biomechanical function. Decellularization has been shown to be a highly promising technique to generate tissue-specific ECM-derived products for multiple applications, although it might comprise very complex processes that involve the simultaneous use of chemical, biochemical, physical and enzymatic protocols. Several different approaches have been reported in the literature for the treatment of bone, cartilage, adipose, dermal, neural and cardiovascular tissues, as well as skeletal muscle, tendons and gastrointestinal tract matrices. However, most of these reports refer to experimental data. This paper reviews the most common and latest decellularization approaches that have been adopted in cardiovascular tissue engineering. The efficacy of cells removal was specifically reviewed and discussed, together with the parameters that could be used as quality control markers for the evaluation of the effectiveness of decellularization and tissue biocompatibility. The purpose was to provide a panel of parameters that can be shared and taken into consideration by the scientific community to achieve more efficient, comparable, and reliable experimental research results and a faster technology transfer to the market.
Generally, in biological tissue, the main component in terms of volume is not related to the cells, but rather to the cell-secreted three-dimensional extracellular matrix (ECM; Table 1). The ECM provides structural and histoarchitectural integrity and mechanical support in the tissues and organs, working actively in the exchange of ions, nutrients, waters, metabolites, and signals (Gattazzo et al., 2014). The sequencing of the human genome revealed hundreds of proteins that are involved in the constitution of the mature ECM (Naba et al., 2011). The set of these proteins constitutes the matrisome that comprises approximately 1–1.5% of the mammalian proteome (Hynes and Naba, 2011). The ECM acts as a multi-functional environment in which tissue-resident cells attach, communicate and interact, thereby regulating cell dynamics and behaviour and contributing to the maintenance of tissue-specific cell phenotypes and functions. To date, a number of different animal-tissue-derived ECMs have been used to produce bioprosthetic (chemically treated) substitutes for various applications such as for bone, cartilage, muscle, tendon, vascular graft, heart valve, nerve, dermal and gastrointestinal tract tissue repair or replacement (Badylak et al., 2009; Brown and Badylack, 2014; Folli et al., 2018). Many of these biological medical devices have been subjected to treatments that allow preservation of their functionality, but not the viability of their cellular content. Non-viable tissue is not capable of ECM regeneration and remodelling, thus limiting their lifespan and imposing the need for frequent replacement, forcing patients to multiple surgical interventions. To overcome this limitation and develop viable and functional engineered animal-derived ECMs, native tissues have been subjected to controlled removal of their cellular content, generating a decellularized three-dimensional scaffold (Crapo et al., 2011). Reported decellularization protocols have included a combination of physical techniques, detergents, enzymes and chemical compounds that have been shown not to adversely affect the architectural, ultrastructural, mechanical or biological integrity of the ECM (see Table 2). Unfortunately, there has been a lack of standardized assessment of tissue-specific decellularization methods, which compromises the effective comparison of the efficiency of different protocols (Bruyneel and Carr, 2017). Post-decellularization tissue characterization has included in vitro and in vivo studies providing data that are limited to the removal of the endogenous DNA in the ECM scaffolds and the safety of implantable commercial products. However, even when marketed decellularized products have been certified with the ISO standard for biological medical devices, several adverse reactions have been reported when introduced into the clinical setting (Simon et al., 2003; Kasimir et al., 2005; Ruffer et al., 2010; Woo et al., 2016). In particular, clinical application of decellularized porcine pulmonary valves exhibited massive inflammatory reaction associated with necrosis and graft stenosis in adult patients (Breitenbach et al., 2014—; Perri et al., 2012) with a relevant percentage of patients needed reoperation. Similarly, porcine intestinal submucosae (SIS) adopted for heart valve repair and reconstruction exhibited the presence of inflammatory cells, fibrosis and calcification after 4 years from the implant (Padalino et al., 2016—Hofmann et al., 2017—Mosala et al., 2017).
FIGURE 1. Tissue decellularization flow-scheme supported by suggested assessment for post tissue treatment evaluation and implanted graft integration.
In addition, the literature survey has indicated that many different parameters have not been carefully considered in the assessment of decellularised scaffolds, even though they have been shown to be related to adverse events and/or dramatic side effects that compromised the functionality of the prosthesis in the patient and, ultimately, the patient’s health.
Each year, more than 450.000 patients undergo heart valve replacement worldwide, and this number is estimated to reach 1.000.000 by 2050 due to the ageing population and the westernization of the lifestyle in several areas of the world, towards unbalanced diets with fatty foods and, consequently, the increase in cardiovascular disease (Naso and Gandaglia, 2018). Therapies for heart valve disease have most commonly comprised interventional and surgical approaches since pharmacological agents only address symptoms management. Surgical approach to dysfunctional heart valves can be addressed through the use of biological or mechanical prostheses. Bioprosthetic heart valves (BHVs) fabricated from bovine, porcine, or equine pericardium, bovine jugular vein, or porcine heart valve leaflets feature low thrombogenicity with no need for lifelong anti-coagulants (Carpentier et al., 1969). Unfortunately, the longevity of BHVs is limited by structural valve degeneration (SVD), which begins within 5 years after the implantation and typically necessitates BHV replacement after 10–12 years from the surgery (Shinkawa et al., 2015). In particular, BHVs substitutes are subjected to structural degradation, calcification and immune response (Koziarz et al., 2020) as a consequence of incomplete biocompatibility of the animal tissue (frequently bovine pericardium) adopted for their manufacture (Simionescu et al., 2021). In fact, the treatment with glutaraldehyde (GLU, considered as the standard method to ensure tissue biocompatibility, increased mechanical strength, sterilization and safe storage of BHVs) is not able to reach complete masking of the antigenic determinants of animal origin (Naso et al., 2013a). The in vivo interaction between such antigens and the patient’s circulating antibody was shown to exert a powerful pro-calcific effect. Moreover, GLU is responsible be, by itself, a potential calcium-binding site due to the presence of residual aldehydes, acids and Schiff bases.
Evidence suggests that also homograft (allogeneic valves) suffers from a loss of function due to early donor-specific antibody and T cell-mediated responses to human leukocyte antigens (MHC Class 2 HLA-DR) inducing fever and vascular disfunction (Dignan et al., 2000). In particular, concerns have been expressed about the durability of homograft valves in younger pediatric patients after the failure within 8 months in a small clinical retrospective study, due to T- and B-lymphocytic infiltrates (Rajani et al., 1998).
Mechanical valves (tilting disk or bi-leaflets) are made from metal and/or pyrolytic carbon. The main disadvantage of this type of valve is the formation of thrombotic clots in the stagnation points and/or hemolysis which requires lifelong anticoagulation therapy for the patients (Harris et al., 2015).
Notwithstanding the tremendous technological progress in the field of heart valve therapy, an ideal heart valve replacement has yet to be developed, with current replacement options lowering the quality of life and the life expectancy of heart valve replacement patients when compared to age-matched healthy individuals (Huygens et al., 2016). In addition, heart valve replacements are very costly (circa 15 billion Euros annually in the United States and Europe), mainly due to the postoperative care costs and the need for re-operations (Huygens et al., 2018; Etnel et al., 2019). A clear, unmet clinical need remains for a heart valve substitute that does not degenerate, can adjust to functional and somatic changes, and can be implanted via minimally invasive techniques. To overcome these limitations the xenogeneic decellularised heart valve has been considered as an attractive alternative for the development of a viable biological valve, capable of remodelling and regeneration.
A variety of chemicals, including detergents, solvents, acidic and alkaline solutions, and ionic solutions have been used to solubilize cellular membranes along with mechanical agitation or perfusion. Alkaline and acid treatments are very effective in solubilizing the cytoplasmic components; however, they have been rarely used since they are very aggressive towards the ECM proteins. Acetic-, peracetic-, hydrochloric- and sulfuric-acid, as well as ammonium hydroxide, are some examples of chemicals that have been shown to disrupt cellular membranes and intracellular organelles, whilst also dissociating important molecules, such as collagen, protein-protein bonds and GAGs (Gilbert et al., 2005; Gilbert et al., 2006; Reing et al., 2010; Cornelison et al., 2018).
Detergents have been used to solubilize cellular membranes and dissociate the intracellular structure. Nonionic detergents, such as Triton X-100 have been largely used in several decellularization protocols (Malone et al., 1984; Wilson et al., 1995; Bader et al., 1998; Kim et al., 2002; Meyer et al., 2005; Gallo et al., 2012), and has been shown to target the lipid–lipid and lipid-protein chemical bonds, leaving unaltered protein-protein interactions (Cartmell and Dunn, 2000; Woods and Gratzer, 2005). Although Triton X-100 is effective in the decellularization of tissues in which the key ECM component is primarily proteins, it has been generally avoided for the decellularization of tissues particularly rich in glycosaminoglycans (GAGs) (Korossis et al., 2002—; Booth et al., 2002). Moreover, Triton X-100 has often been adopted to remove the remnants of anionic detergents (sodium dodecyl sulfate; SDS) (Naso and Gandaglia, 2018). Anionic detergents including sodium deoxycholate (SDC) and SDS have been also used in many reported studies (Wilson et al., 1995; Korossis et al., 2002; Konertz et al., 2005; Erdbrügger et al., 2006; Gallo et al., 2012). SDS is reported to solubilize both the external and nuclear membranes, but it has also been recognized to denature proteins, altering the native structure of the ECM (Chen et al., 2004; Elder et al., 2010). Owing to this, short treatments with SDS could be a reasonable choice, aiming at minimizing the possible damage to the ECM proteins and overall structure (Kim et al., 2002 -; Tavassoli et al., 2015). Nevertheless, SDS is considered very proficient in removing nuclear and cytoplasmic waste, even though it is more challenging to be rinsed out of the tissue due to its ionic nature (Rajab et al., 2020—; Syed et al., 2014—; Dettin et al., 2017).
Zwitterionic detergents share features of both nonionic and ionic detergents. They are electrically neutral molecules that have both positive and negative charges. The tendency of these detergents to denature proteins has been demonstrated to be lower than ionic detergents, but higher than nonionic ones. CHAPS {3-[(3-cholamidopropyl)dimethylammonio]-1-propanesulfonate} has been a commonly used zwitterionic detergent (Quint et al., 2011). Noteworthy, zwitterionic detergents have been reported not to be effective permeating agents and, therefore, have been mainly used to decellularize thin tissue (Mendibil et al., 2020).
During decellularization, endogenous proteases have been reported to leak in high amounts from the lysed cells, risking irreversible damage to the ECM (Crapo et al., 2011). Phenylmethylsulfonylfluoride (PMSF), aprotinin and leupeptin are common protease inhibitors that have been adopted to prevent proteolysis (Gallo et al., 2012; Luo et al., 2014). Some decellularization protocols have also included antibiotics, such as penicillin, streptomycin, or amphotericin B, to prevent tissue contamination during treatment (Leyh et al., 2003; Lin et al., 2004; Gilpin and Yang, 2017; Rajab et al., 2020).
Biological protocols for tissue decellularization have involved enzymatic reactions, mainly to eliminate cell debris and other undesirable components of the ECM such as nucleic acids residues, fragments of cell membranes and mitochondrial DNA (Londono et al., 2017; Naso and Gandaglia, 2018). The removal of the nucleic acids residues has been of paramount importance in all decellularization processes, due to their tendency to remain stuck to ECM proteins and attract circulating calcium salt. To this end, decellularization protocols have utilized nucleases to catalyze the degradation of nucleic acids. Nucleases have been generally classified into endo- and exo-nucleases that cleave phosphodiester bonds within the nucleic acids and nucleotides from the end of nucleic acids respectively (Haupt et al., 2018).
Proteases have been used to catalyze the degradation of proteins and the effective detachment of the cellular component from its connective support. Among them, trypsin and pepsin have been the most commonly used proteases in decellularization protocols (Naso and Gandaglia, 2018), with the formers targeting the C-side bonds in arginine and lysine, and the latter being a highly aggressive protease commonly found in the stomach and also targeting the bonds between peptides. Noteworthy, the prolonged exposure to proteolytic enzymes has been reported to significantly alter the structure of the ECM, destroying laminin and removing GAGs, thus resulting in severe mechanical weakness of the tissue scaffold (Rieder et al., 2004; Poon et al., 2013; Naso and Gandaglia, 2018).
With a view to removing additional tissue components, different enzymes such as lipase that hydrolyzes lipids and can be useful for fatty tissues, and α-galactosidase that has been shown to be effective in removing the galactose-α-(1,3)-galactose xenoepitope, responsible for xenorejection in humans (Gilbert, 2012) have been used. Due to the aggressiveness of the enzymatic treatments on the ECM proteins, the concentration of the enzymes as well as the duration of the enzymatic treatment, have been shown to be critical parameters for achieving maximal decellularization effectiveness and minimal ECM degradation (Schenke-Layland et al., 2003).
Physical techniques alone have been shown inadequate to produce effective tissue decellularization. As such, they have been used in combination with chemical and/or enzymatic processes. Physical methods that have been reported to facilitate the decellularization process are summarized in Table 2. Briefly, freezing-thawing, direct pressure, agitation, sonication, vascular perfusion, thermal shock, ultrasonic and manual disruption have been shown to facilitate cell disruption, transportation of the decellularization solution to cells and washing out of the cellular debris from tissues (Toumpaniari et al., 2020).
Studies have shown that suitable chemical signals can be coordinated for an intentional stimulation of the endogenous cellular apoptotic pathway. This approach has been reported to completely depend on the distribution of the precise ligands (Tumor Necrosis Factor Receptor TNFR1 (DR-1); Fas/CD95 (DR-2); Apoptosis Antigen Apo-3 (DR-3); TNF-related apoptosis inducible ligand-receptor TRAILR (DR-4 and DR-5); Nerve Growth Factor Receptor (NGFR); Ectodysplasin A Receptor (EDAR) that attach their analogous death receptors to the TNF superfamily (TNF alpha; FasL; TL1A; TRAIL; NGF and EDA respectively) and by the expression level of crucial genes elaborated in the extrinsic or intrinsic (perforin/granzyme) apoptotic pathways (Ashkenazi and Dixit, 1998; Wilson et al., 2009). Throughout the progression of apoptosis, the cellular substance has been shown to be retained within the plasma membrane and the apoptotic bodies. As such, the immunogenic cellular components are not secreted into the nearby environment, thus averting an immune-mediated inflammatory response (Bourgine et al., 2013). The lethal-environmental-conditioning method has been utilised by moderating environmental influences, such as temperature and pH, as well as carbon dioxide/oxygen, nitric oxide and hydrogen peroxide content (Somuncu, 2020).
Since 1989, the year in which the pioneer of heart valve surgery Harken DW sanctioned the “ten commandments” for the ideal heart valve substitute (Harken, 1989), none of the developed heart valve replacements has been shown to possess self-repair, adaptively remodelling and growth whilst being resistant to infection and thrombogenicity. However, decellularized porcine tissues (small intestinal submucosa or pulmonary valves) have been utilized for the manufacturing of bioprosthetic heart valves substitutes (Fioretta et al., 2020). The promising results that were reported from preclinical studies did not match those obtained from the clinical application, many of these medical devices, notwithstanding the approval by regulating bodies have been proven to be ineffectively decellularized, eliciting a massive host-specific inflammatory response (Naso et al., 2014). Incidents of leaflets thickening, fibrosis and calcification have been also described. In particular, a strong immunological reaction was reported just a few hours following the implant of the decellularized valve (O’Brien et al., 1999; Dohmen et al., 2003; Leyh et al., 2003; Simon et al., 2003; Dohmen et al., 2005; Ota et al., 2005; Ruiz et al., 2005; Takagi et al., 2006; Iwai et al., 2007; Ruffer et al., 2010; Dohmen et al., 2012; Hopkins et al., 2013; Fallon et al., 2014; Zafar et al., 2015; Hennessy et al., 2017; Mosala et al., 2017; Goecke et al., 2018; Rijswijk et al., 2020).
Preclinical applications of decellularized xenograft valve replacements have been generally issued with the ISO standard for biological medical devices (see Table 3). More recently, a specific standard for the evaluation of decellularized products has become available (ASTM International, 2021). Generally, “decellularization” is a recognized complex process that allows the use of animal-derived ECM products in medical treatment “with reduced risk of adverse host immune response and immune rejection” by disrupting and removing cells and/or cell contents while aiming to preserve significant features of the ECM structure and/or composition (F3354-19, 2021). Unfortunately, the ISO guidelines currently in place do not adequately account for the immunological and pro-inflammatory aspects of such decellularized devices. Within the tissue engineering community, DNA quantification and qualitative evaluation of cell removal have represented common practices for the characterization of the decellularization effectiveness. Residual DNA is responsible for triggering a strong inflammatory response against the implanted decellularized xenogeneic tissue, resulting in calcification and structural degeneration (Simon et al., 2003). A threshold of 50 ng/mg (DNA/tissue) has been commonly accepted to be non-immunogenic (Crapo et al., 2011). Despite this, various studies have reported decellularized porcine small intestine submucosa with up to 6 μg/mg of nucleic acid debris (Naso et al., 2013a; Rijswijk et al., 2020). It is worth noting that often the evaluation of the residual DNA presence after decellularization is only qualitative, limiting the assessment to fluorescence staining based on the use of DAPI (4′,6-diamidino-2-phenylindole, a fluorescent stain that binds strongly to adenine–thymine-rich regions in DNA). Gilbert and colleagues (Gilbert et al., 2009) have proved the existence of DNA fragments in some commercial products even if, when smaller than 300 bp, they do not seem to be enough to stimulate critically the immune system (Soto-Gutierrez et al., 2012).
TABLE 3. Common reference ISO Standard for the production of class III implantable biological medical devices.
Although quantification of the residual DNA content represents a reasonable way for assessing the effectiveness of decellularization, this approach is limited and not very representative of the immuno-inflammatory risk that can arise following the implantation of decellularized tissues. Homogenized detergent-decellularised scaffolds have been shown to exhibit a high amount of cytosolic and cytoskeleton proteins, such as glyceraldehyde 3-phosphate dehydrogenase (GAPDH) and smooth muscle actin. Interestedly, about 306 proteins of cytosolic, organelle, nuclear and cell membrane origin have been shown to still be identifiable by mass spectrometry (Böer et al., 2011). In addition to the antigenic molecules derived from cell lysis, it is of particular relevance to consider other aspects, such as the remnants of detergents and the presence of pro-inflammatory structures such as hyaluronan fragments.
Xenograft immunogenicity is directly related to the presence of several antigens including the α-Gal epitope (Naso et al., 2013b), the linked N-glycolyl neuraminic sialic acid (Neu5Gc) (Gao et al., 2017) and the Sd(a) (Byrne et al., 2011). These antigenic molecules are expressed in mammals except for Old World monkeys, apes and humans. Following various stimulation pathways, the human body produces antibodies directed against these molecules. Once a xenogeneic tissue is recognized, the complement cascade is activated, triggering endothelial cell dysfunction, platelet aggregation, and vascular thrombosis (Leventhal et al., 1993; Leventhal et al., 1995; Saadi and Platt, 1995; Hedlund et al., 2008; Pham et al., 2009). In particular, the residual presence of the alpha-Gal xenoantigen significantly increases the human anti-galactose titers specifically directed to the Gala1-3Gal residue, starting from day 10 after BHV implantation and reaching a peak at around 3 months for both IgM and IgG isotypes (Mangold et al., 2009—; Konakci et al., 2005). Such antigenic determinants exposed on the cell surface may persist in membrane residues entrapped between the fibers of the ECM or not properly removed by the action of detergents and may still be capable of reacting despite the elimination of all individual cells (Naso and Gandaglia, 2018).
The treatment most widely used to date to ensure the immunogenic tolerance of animal tissues intended for the manufacture of bioprosthetic heart valves is based on chemical crosslinking with glutaraldehyde (GA). Despite being a benchmark, GA treatment is only partially effective in masking such epitopes (Naso et al., 2013b) resulting susceptible to the development of early BHV degeneration (Hawkins et al., 2016) and immune response following BHV implant (Park et al., 2010—; Schussler et al., 2020). The inability of commercially available treatments capable of effectively inactivating this antigen has recently prompted the U.S. Food and Drug Administration (FDA) to officially approve the production of a genetically modified domestic pig line knocked out for the alpha-Gal in order to provide a source of porcine-based materials for the production of safer and more efficient biomedical devices (U.S. Food and Drug Administration, 2020).
Currently, there are only two technologies that have been reported in the literature to produce complete removal of these xeno-epitopes, including the TriCol decellularization protocol (Gallo et al., 2012) and the FACTA® treatment that has been applied to native and GA-treated animal tissues (Naso et al., 2017).
A correct assessment of the presence of these xeno-epitopes requires an initial in vitro evaluation, using suitable antibodies, followed by in vivo testing in an animal model. However, current commercially available animal models cannot be considered suitable for this purpose. Wildtype pigs, sheep or rats, like all mammals, do not develop an immunological reaction against these xeno-epitopes, being inefficient in predicting a possible immune-mediated acute or delayed reaction. Owing to this, it is necessary to use animal models that do not express the epitope in question (Old World primates) or humanized animal models, such as genetically modified (knockout) models in which the gene responsible for expression has been inactivated (Smood et al., 2019; Galili and Stone, 2021).
The decellularization procedures reported in the literature have demonstrated several differences, such as detergent type and concentration, treatment duration, and the number of washes. Most of the studies have indirectly evaluated the amount of detergent residuals in the decellularized scaffolds by determining the amount of the detergents in the washing solutions following cell removal and not directly within the scaffold (Erdbrügger et al., 2006; Caamaño et al., 2009). Effective removal of the detergents is important since the interaction of the ECM with anionic ones has been reported to decrease the tensile strength of elastin fibers (the major component of blood vessels) and to increase the susceptibility of insoluble elastin and collagen to enzymatic degradation (Kagan et al., 1972; Jordan et al., 1974; Guantieri et al., 1983). Elastin degradation products, in turn, can promote the myofibroblastic and osteogenic differentiation of fibroblasts and the in vivo recruitment of inflammatory cells (Senior et al., 1980; Simionescu et al., 2007).
Bile acids and salts may be considered natural detergents as they emulsify fats and modify the permeability of cellular membranes (Naso and Gandaglia, 2018). They have been reported to exist in several states in biological systems (even conjugated with sodium, potassium, glycine, taurine, etc.), including insoluble gels, micelles or vesicles, and insoluble calcium salts (Hofmann, 1989). Precipitation of bile acids in the insoluble protonated acid form is mainly controlled by the critical micellization concentration, pH and temperature, Ca2+ ion activity, and finally the concentration and structure of the monomeric bile acid anion (Hofmann and Mysels, 1992). Variations of physicochemical conditions may cause the bile salts to transit toward the insoluble gel form, remaining trapped within the ECM fibrillar network determining cell cytotoxicity.
The in vitro reseeding of SDS-treated tissues reported controversial results: decellularization of human pericardium and porcine aortic and pulmonary valves using a low concentration of SDS resulted in not cytotoxic drawbacks to in vitro cell seeding and cytotoxicity assessment (Mirsadraee et al., 2006—; Vafaee et al., 2018—; Cebotari et al., 2010—; Iop et al., 2014). In a discordant way, Rieder and colleagues reported cytotoxicity of SDS in porcine aortic valves and leaflets treatment (Rieder et al., 2004). In particular, a reduced attachment of human endothelial cells to the matrix was observed (Knight et al., 2005), as well as cytotoxicity of the PBS used for the storage of the decellularized valves, leading to conclude that the residual SDS in the tissue was a cause of cytotoxicity. Noteworthy, even a relatively low residual amount of SDS was correlated with an in vitro increased fibroblast activation and in vivo foreign body response (Friedrich et al., 2017).
Viability, proliferative capacity and phenotype of human urothelial cells upon urinary bladder matrix scaffolds were maintained on graft treated with Triton X-100, CHAPS and sodium deoxycholate and was similar to cells cultured on the same scaffolds but treated with deionized water (White et al., 2017). SDS treatment resulted in a basement membrane complex with altered ultrastructure and composition, endothelial cells seeded on SDS treated scaffolds reported an atypical morphology, fragmented nuclei and considerably reduced confluence (Faulk et al., 2014).
Reliable information on residual detergents could be used to investigate the relationship, if any, between the actual detergent-based treatment and the observed and/or potential drawbacks in the decellularized scaffold. Additionally, this information could be used for end-product quality control in the manufacturing process of existing decellularised scaffolds currently on the market or under consideration for clinical use. Recently was developed the first cell-based biosensor for residual detergent detection especially in decellularized scaffolds (Ghorbani et al., 2021). This biosensor can be additionally adopted for the evaluation of the cytocompatibility of decellularized tissue giving a comprehensive overview of the general biocompatibility state of the decellularized tissue graft.
Hyaluronic acid also called hyaluronan (HA), is an anionic, non-sulfated glycosaminoglycan distributed widely throughout connective, epithelial, and neural tissues. HA amounts to 18% in porcine aortic leaflets, 25% in porcine pulmonary leaflets, and 15% in the bovine pericardium (Cigliano et al., 2012). It appears usually as a high-molecular-size polymer of up to 2 × 104 kDa, where disaccharides of N-acetyl-glucosamine and glucuronic acid, connected to each other exclusively by β-linkages, are repeated (Prehm, 1984; Lee and Spicer, 2000). The polymer contributes to the maintenance of tissue hydration (Granger et al., 1984), acting also as an anti-apoptotic agent (Forrester and Balazs, 1980; Jing and DeAngelis, 2004). HA has demonstrated excellent anti-inflammatory and immunosuppressive function due to the ability to coat cell membrane thereby preventing access by the ligand to the surface receptors inhibiting phagocytosis by monocytes, macrophages, and polymorphonuclear neutrophils (PMNs) (Forrester and Balazs, 1980).
During the first step of decellularization, cells lysis may release several types of proteases, some of which will be able to degrade HA (either in free forms or being part of proteoglycans PGs). HA fragments, ranging from 500 Da to 1.5 kDa, have shown a marked pro-inflammatory activity able to activate macrophages with the secretion of various pro-inflammatory chemokines (McKee et al., 1996; Horton et al., 1998). Fragments of 1.35 kDa have been reported to result in inducing dendritic cell maturation, which allowed subsequent priming of allogeneic T-cells to initiate the alloimmunity reaction (Termeer et al., 2000). Specifically, when decellularized tissue is implanted in the human host, the functional effects of HA oligomers and their clearance from the jeopardized tissue stimulate the inflammatory response through their interaction with specific host receptors such as CD44, RHAMM and TLR-2,4 (Rayahin et al., 2015). Lysosomes internalize and further degrade such fragments into tetra and hexasaccharides through the intracellular HYAL1 and HYAL2 (Veiseh and Turley, 2011). These small HA pieces, thus obtained, can act as an endogenous danger signal, leading to the activation of both innate and acquired immunity. The separate stimulation of TLR4 and CD44 receptors may prime/amplify the inflammatory response through NF-kB activation (Loniewski et al., 2007—; Hutás et al., 2008).
It is therefore desirable to ensure that no low-molecular-size HA residues will be available in the decellularized scaffold. When HA fragments are entrapped within the insoluble form of bile salt detergent, or not properly washed out from the ECM, they can transform the decellularised graft into a ticking time bomb, ready to trigger acute immune-mediated reaction once perfused with the recipient’s blood (Naso and Gandaglia 2018).
The in-place guidelines contained in the International Organization for Standardization (especially ISO10993 and ISO 5840, Table 3) regulating the manufacturing of decellularized tissue scaffolds appear to need an update. This is evident since the preclinical quantitative assessments of the residual content of xenogeneic reactive molecules, detergents and nucleic acid materials in decellularized scaffolds are insufficient and have led to disastrous results. Decellularized grafts such as the Matrix P prostheses, notwithstanding showed encouraging short-term results, reported unfavourable echocardiographic performances with a relevant dysfunction of the prosthesis in adult patients and a subsequent high rate of reoperation/reintervention for structural pulmonary valve failure (Christ et al., 2019). Histological examination performed by Breitenbach and colleagues exhibited massive inflammatory reaction and necrosis in the Matrix P Plus prostheses adopted as pulmonary homograft in the Ross procedure in adult patients (Breitenbach et al., 2014). Backhoff and colleagues (Backhoff et al., 2014) reported a massive Matrix P® conduit obstruction after 5 years from the implant in 6 years old patients. Conduit dissection allowing blood flow in the thickness of the conduit wall with the formation of pseudoaneurysms was reported, confirming what was already observed also by Rüffer and colleagues (Rüffer et al., 2010). Histological examination showed massive inflammatory reactions and necrosis. Severe inflammation increased fibrous tissue and foreign-body reaction against valve leaflets and fascial tissue was similarly highlighted even by Vogel in young patients (Voges et al., 2013). The reconstruction of aortic heart valves using a decellularized extracellular matrix (CorMatrix®) in pediatric patients reported severe valve dysfunction occurred suddenly during the first three post-operative months. A migration of inflammatory cells into the graft edge bordering the native tissue was identified inducing a significant thickening of the patch. The inflammatory reaction induced structural and functional changes (Hofmann et al., 2017).
A strict requirement for any biomedical device is standardization. The consistency and reproducibility of the treatments made according to predefined quality criteria can be ensured, also by adopting new standards capable of adapting to technological progress and new scientific knowledge, such as in the case of knockout animal models. Researchers and the scientific community, while always looking for technological improvement, should converge in the definition of acceptance criteria related to ensuring the consistent evaluation and use of decellularization in manufacturing medical products. Such criteria should address the adequacy of cellular disruption and removal of cellular remnants, defining acceptable quality levels for the remaining extracellular matrix components (Table 4). It would also be appreciable to define a suitable decellularization reagents list placing selective limits on their persistence on the treated ECM.
TABLE 4. Summary table showing the approaches commonly adopted for evaluating the efficacy of decellularization processes and the methods of investigation for highlighting potentially dangerous tissue changes according to the future performance of the implant.
Similarly, to what happens for the definition and the quality management of any industrial process, a decellularization protocol should also identify according to the step’s objective: 1) the general procedure, 2) materials and equipment utilized, 3) duration or end-point determination, 4) static process parameters, and 5) any variable parameters that may scale with source ECM material characteristics, dimensions, or quantities. Test and inspection procedures should be designed and planned during all the decellularization process, establishing the acceptance criteria in advance. Even if this new standardization approach is unlikely to be applied to products already on the market, it will allow for a more efficient, comparable, and reliable experimental research study to allow a faster technology transfer to the market with an unprecedented level of safety for the patient’s health.
FN and AG contributed to the conception and design of the review. FN wrote the first draft of the manuscript. All authors contributed to manuscript revision, read, and approved the submitted version.
FN and AG were employed by Biocompatibility Innovation Srl.
All claims expressed in this article are solely those of the authors and do not necessarily represent those of their affiliated organizations, or those of the publisher, the editors, and the reviewers. Any product that may be evaluated in this article, or claim that may be made by its manufacturer, is not guaranteed or endorsed by the publisher.
Ashkenazi, A., and Dixit, V. M. (1998). Death Receptors: Signaling and Modulation. Science 281, 1305–1308. doi:10.1126/science.281.5381.1305
ASTM International (2021). F3354-19: Standard Guide for Evaluating Extracellular Matrix Decellularization Processes. Pennsylvania, USA: ASTM International.
Backhoff, D., Steinmetz, M., Sigler, M., and Schneider, H. (2014). Formation of Multiple Conduit Aneurysms Following Matrix P Conduit Implantation in a Boy with Tetralogy of Fallot and Pulmonary Atresia. Eur. J. Cardiothorac. Surg. 46, 500–502. doi:10.1093/ejcts/ezt635
Bader, A., Schilling, T., Teebken, O. E., Brandes, G., Herden, T., Steinhoff, G., et al. (1998). Tissue Engineering of Heart Valves-Hhuman Endothelial Cell Seeding of Detergent Acellularized Porcine Valves. Eur. J. Cardiothorac. Surg. 14, 279–284. doi:10.1016/s1010-7940(98)00171-7
Badylak, S., Freytes, D., and Gilbert, T. (2009). Extracellular Matrix as a Biological Scaffold Material: Structure and Function. Acta Biomater. 5, 1–13. doi:10.1016/j.actbio.2008.09.013
Böer, U., Lohrenz, A., Klingenberg, M., Pich, A., Haverich, A., and Wilhelmi, M. (2011). The Effect of Detergent-Based Decellularization Procedures on Cellular Proteins and Immunogenicity in Equine Carotid Artery Grafts. Biomaterials 32, 9730–9737. doi:10.1016/j.biomaterials.2011.09.015
Booth, C., Korossis, S. A., Wilcox, H. E., Watterson, K. G., Kearney, J. N., Fisher, J., et al. (2002). Tissue Engineering of Cardiac Valve Prostheses I: Development and Histological Characterization of an Acellular Porcine Scaffold. J. Heart Valve Dis. 11, 457–462.
Bourgine, P. E., Pippenger, B. E., Todorov, A., Tchang, L., and Martin, I. (2013). Tissue Decellularization by Activation of Programmed Cell Death. Biomaterials 34, 6099–6108. doi:10.1016/j.biomaterials.2013.04.058
Breitenbach, I., El-Essawi, A., Pahari, D., Kappenberg, T., Harringer, W., and Anssar, M. (2014). Early Failure of Decellularized Xenogenous Pulmonary Valve Conduit (Matrix-P-Plus) for Reconstruction of the Right Ventricular Outflow Tract in the ross Procedure. Thorac. Cardiovasc. Surg. 62, OP123. doi:10.1055/s-0034-1367197
Brown, B. N., and Badylak, S. F. (2014). Extracellular Matrix as an Inductive Scaffold for Functional Tissue Reconstruction. Translational Res. 163, 268–285. doi:10.1016/j.trsl.2013.11.003
Bruyneel, A. A. N., and Carr, C. A. (2017). Ambiguity in the Presentation of Decellularized Tissue Composition: The Need for Standardized Approaches. Artif. Organs 41, 778–784. doi:10.1111/aor.12838
Butler, C. R., Hynds, R. E., Crowley, C., Gowers, K. H. C., Partington, L., Hamilton, N. J., et al. (2017). Vacuum-assisted Decellularization: an Accelerated Protocol to Generate Tissue-Engineered Human Tracheal Scaffolds. Biomaterials 124, 95–105. doi:10.1016/j.biomaterials.2017.02.001
Byrne, G. W., Stalboerger, P. G., Du, Z., Davis, T. R., and McGregor, C. G. A. (2011). Identification of New Carbohydrate and Membrane Protein Antigens in Cardiac Xenotransplantation. Transplantation 91, 287–292. doi:10.1097/TP.0b013e318203c27d
Caamaño, S., Shiori, A., Strauss, S. H., and Orton, E. C. (2009). Does Sodium Dodecyl Sulfate Wash Out of Detergent-Treated Bovine Pericardium at Cytotoxic Concentrations?. J. Heart Valve Dis. 18, 101–105.
Carpentier, A., Lemaigre, G., Robert, L., Carpentier, S., Dubost, C., and Gerbode, F. (1969). Biological Factors Affecting Long-Term Results of Valvular Heterografts. J. Thorac. Cardiovasc. Surg. 58, 467–483. doi:10.1016/s0022-5223(19)42561-0
Cartmell, J. S., and Dunn, M. G. (2000). Effect of Chemical Treatments on Tendon Cellularity and Mechanical Properties. J. Biomed. Mater. Res. 49, 134–140. doi:10.1002/(sici)1097-4636(200001)49:1<134:aid-jbm17>3.0.co;2-d
Cebotari, S., Tudorache, I., Jaekel, T., Hilfiker, A., Dorfman, S., Ternes, W., et al. (2010). Detergent Decellularization of Heart Valves for Tissue Engineering: Toxicological Effects of Residual Detergents on Human Endothelial Cells. Artif. Organs 34, 206–210. doi:10.1111/j.1525-1594.2009.00796.x
Chen, R.-N., Ho, H.-O., Tsai, Y.-T., and Sheu, M.-T. (2004). Process Development of an Acellular Dermal Matrix (ADM) for Biomedical Applications. Biomaterials 25, 2679–2686. doi:10.1016/j.biomaterials.2003.09.070
Christ, T., Paun, A. C., Grubitzsch, H., Holinski, S., Falk, V., and Dushe, S. (2019). Long-term Results after the Ross Procedure with the Decellularized AutoTissue Matrix P Bioprosthesis Used for Pulmonary Valve Replacement. Eur. J. Cardiothorac. Surg. 55, 885–892. doi:10.1093/ejcts/ezy377
Cigliano, A., Gandaglia, A., Lepedda, A. J., Zinellu, E., Naso, F., Gastaldello, A., et al. (2012). Fine Structure of Glycosaminoglycans from Fresh and Decellularized Porcine Cardiac Valves and Pericardium. Biochem. Res. Int. 2012, 1–10. doi:10.1155/2012/979351
Cornelison, R. C., Wellman, S. M., Park, J. H., Porvasnik, S. L., Song, Y. H., Wachs, R. A., et al. (2018). Development of an Apoptosis-Assisted Decellularization Method for Maximal Preservation of Nerve Tissue Structure. Acta Biomater. 77, 116–126. doi:10.1016/j.actbio.2018.07.009
Crapo, P. M., Gilbert, T. W., and Badylak, S. F. (2011). An Overview of Tissue and Whole Organ Decellularization Processes. Biomaterials 32, 3233–3243. doi:10.1016/j.biomaterials.2011.01.057
Curtil, A., Pegg, D. E., and Wilson, A. (1997a). Repopulation of Freeze-Dried Porcine Valves with Human Fibroblasts and Endothelial Cells. J. Heart Valve Dis. 6, 296–306.
Curtil, A., Pegg, D. E., and Wilson, A. (1997b). Freeze Drying of Cardiac Valves in Preparation for Cellular Repopulation. Cryobiology 34, 13–22. doi:10.1006/cryo.1996.1982
Dettin, M., Zamuner, A., Naso, F., Monteleone, A., Spina, M., and Gerosa, G. (2017). Natural Scaffolds for Regenerative Medicine: Direct Determination of Detergents Entrapped in Decellularized Heart Valves. Biomed. Res. Int. 2017, 1–9. doi:10.1155/2017/9274135
Dignan, R., O'Brien, M., Hogan, P., Passage, J., Stephens, F., Thornton, A., et al. (2000). Influence of HLA Matching and Associated Factors on Aortic Valve Homograft Function. J. Heart Valve Dis. 9, 504–511.
Dohmen, P. M., Ozaki, S., Nitsch, R., Yperman, J., Flameng, W., and Konertz, W. (2003). A Tissue Engineered Heart Valve Implanted in a Juvenile Sheep Model. Med. Sci. Monit. 9, BR97–BR104.
Dohmen, P. M., Costa, F. d., Lopes, S. V., Yoshi, S., Souza, F. P. d., Vilani, R., et al. (2005). Results of a Decellularized Porcine Heart Valve Implanted into the Juvenile Sheep Model. Hsf 8, 100–104. doi:10.1532/HSF98.20041140
Dohmen, P. M., Costa, F. d., Yoshi, S., Lopes, S. V., Souza, F. P., Vilani, R., et al. (2012). An Experimental Study of Decellularized Xenografts Implanted into the Aortic Position with 4 Months of Follow up. J. Clin. Exp. Cardiolog S4. doi:10.4172/2155-9880.S4-004
Elder, B. D., Kim, D. H., and Athanasiou, K. A. (2010). Developing an Articular Cartilage Decellularization Process toward Facet Joint Cartilage Replacement. Neurosurgery 66, 722–727. doi:10.1227/01.NEU.0000367616.49291.9F
Erdbrügger, W., Konertz, W., Dohmen, P. M., Posner, S., Ellerbrok, H., Brodde, O.-E., et al. (2006). Decellularized Xenogenic Heart Valves Reveal Remodeling and Growth Potentialin Vivo. Tissue Eng. 12, 2059–2068. doi:10.1089/ten.2006.12.2059
Etnel, J. R. G., Huygens, S. A., Grashuis, P., Pekbay, B., Papageorgiou, G., Roos Hesselink, J. W., et al. (2019). Bioprosthetic Aortic Valve Replacement in Nonelderly Adults. Circ. Cardiovasc. Qual. Outcomes 12, e005481. doi:10.1161/CIRCOUTCOMES.118.005481
Fallon, A. M., Goodchild, T. T., Cox, J. L., and Matheny, R. G. (2014). In Vivo remodeling Potential of a Novel Bioprosthetic Tricuspid Valve in an Ovine Model. J. Thorac. Cardiovasc. Surg. 148, 333–340. doi:10.1016/j.jtcvs.2013.10.048
Faulk, D. M., Carruthers, C. A., Warner, H. J., Kramer, C. R., Reing, J. E., Zhang, L., et al. (2014). The Effect of Detergents on the Basement Membrane Complex of a Biologic Scaffold Material. Acta Biomater. 10, 183–193. doi:10.1016/j.actbio.2013.09.006
Fioretta, E. S., Motta, S. E., Lintas, V., Loerakker, S., Parker, K. K., Baaijens, F. P. T., et al. (2020). Next-generation Tissue-Engineered Heart Valves with Repair, Remodelling and Regeneration Capacity. Nat. Rev. Cardiol. 18, 92–116. doi:10.1038/s41569-020-0422-8
Folli, S., Curcio, A., Melandri, D., Bondioli, E., Rocco, N., Catanuto, G., et al. (2018). A New Human-Derived Acellular Dermal Matrix for Breast Reconstruction Available for the European Market: Preliminary Results. Aesth Plast. Surg. 42, 434–441. doi:10.1007/s00266-017-1069-7
Forrester, J. V., and Balazs, E. A. (1980). Inhibition of Phagocytosis by High Molecular Weight Hyaluronate. Immunology 40, 435–446.
Friedrich, E. E., Lanier, S. T., Niknam‐Bienia, S., Arenas, G. A., Rajendran, D., Wertheim, J. A., et al. (2017). Residual Sodium Dodecyl Sulfate in Decellularized Muscle Matrices Leads to Fibroblast Activation In Vitro and Foreign Body Response In Vivo. J. Tissue Eng. Regen. Med. 12, e1704–e1715. doi:10.1002/term.2604
Galili, U., and Stone, K. R. (2021). In Situ "Humanization" of Porcine Bioprostheses: Demonstration of Tendon Bioprostheses Conversion into Human ACL and Possible Implications for Heart Valve Bioprostheses. Bioengineering 8, 10. doi:10.3390/bioengineering8010010
Gallo, M., Naso, F., Poser, H., Rossi, A., Franci, P., Bianco, R., et al. (2012). Physiological Performance of a Detergent Decellularized Heart Valve Implanted for 15 Months in Vietnamese Pigs: Surgical Procedure, Follow-Up, and Explant Inspection. Artif. Organs 36, E138–E150. doi:10.1111/j.1525-1594.2012.01447.x
Gao, B., Long, C., Lee, W., Zhang, Z., Gao, X., Landsittel, D., et al. (2017). Anti-Neu5Gc and Anti-non-Neu5Gc Antibodies in Healthy Humans. PLoS One 12, e0180768. doi:10.1371/journal.pone.0180768
Gattazzo, F., Urciuolo, A., and Bonaldo, P. (2014). Extracellular Matrix: a Dynamic Microenvironment for Stem Cell Niche. Biochim. Biophys. Acta (Bba) - Gen. Subjects 1840, 2506–2519. doi:10.1016/j.bbagen.2014.01.010
Ghorbani, F., Abdihaji, M., Roudkenar, M. H., and Ebrahimi, A. (2021). Development of a Cell-Based Biosensor for Residual Detergent Detection in Decellularized Scaffolds. ACS Synth. Biol. 10, 2715–2724. doi:10.1021/acssynbio.1c00321
Gilbert, T., Sellaro, T., and Badylak, S. (2006). Decellularization of Tissues and Organs. Biomaterials 27, 3675–3683. doi:10.1016/j.biomaterials.2006.02.014
Gilbert, T. W., Freund, J. M., and Badylak, S. F. (2009). Quantification of DNA in Biologic Scaffold Materials. J. Surg. Res. 152, 135–139. doi:10.1016/j.jss.2008.02.013
Gilbert, T. W., Stolz, D. B., Biancaniello, F., Simmons-Byrd, A., and Badylak, S. F. (2005). Production and Characterization of ECM Powder: Implications for Tissue Engineering Applications. Biomaterials 26, 1431–1435. doi:10.1016/j.biomaterials.2004.04.042
Gilbert, T. W. (2012). Strategies for Tissue and Organ Decellularization. J. Cel. Biochem. 113, 2217–2222. doi:10.1002/jcb.24130
Gilpin, A., and Yang, Y. (2017). Decellularization Strategies for Regenerative Medicine: From Processing Techniques to Applications. Biomed. Res. Int. 2017, 1–13. doi:10.1155/2017/9831534
Goecke, T., Theodoridis, K., Tudorache, I., Ciubotaru, A., Cebotari, S., Ramm, R., et al. (2018). In Vivo performance of Freeze-Dried Decellularized Pulmonary Heart Valve Allo- and Xenografts Orthotopically Implanted into Juvenile Sheep. Acta Biomater. 68, 41–52. doi:10.1016/j.actbio.2017.11.041
Granger, H. J., Laine, G. A., Barnes, G. E., and Lewis, R. E. (1984). Dynamics and Control of Transmicrovascular Fluid Exchange. Edema 8, 189–224.
Guantieri, V., Tamburro, A. M., and Gordini, D. D. (1983). Interactions of Human and Bovine Elastins with Lipids: Their Proteolysis by Elastase. Connect. Tissue Res. 12, 79–83. doi:10.3109/03008208309005614
Harken, D. E. (1989). Heart Valves: Ten Commandments and Still Counting. Ann. Thorac. Surg. 48, S18–S19. doi:10.1016/0003-4975(89)90623-1
Harris, C., Croce, B., and Cao, C. (2015). Tissue and Mechanical Heart Valves. Ann. Cardiothorac. Surg. 4, 399. doi:10.3978/j.issn.2225-319X.2015.07.01
Haupt, J., Lutter, G., Gorb, S. N., Simionescu, D. T., Frank, D., Seiler, J., et al. (2018). Detergent-based Decellularization Strategy Preserves Macro- and Microstructure of Heart Valves. Interact. Cardiovasc. Thorac. Surg. 26, 230–236. doi:10.1093/icvts/ivx316
Hawkins, R. B., Frischtak, H. L., Kron, I. L., and Ghanta, R. K. (2016). Premature Bioprosthetic Aortic Valve Degeneration Associated with Allergy to Galactose‐Alpha‐1,3‐Galactose. J. Card. Surg. 31, 446–448. doi:10.1111/jocs.12764
Hedlund, M., Padler-Karavani, V., Varki, N. M., and Varki, A. (2008). Evidence for a Human-specific Mechanism for Diet and Antibody-Mediated Inflammation in Carcinoma Progression. Proc. Natl. Acad. Sci. 105, 18936–18941. doi:10.1073/pnas.0803943105
Hennessy, R. S., Go, J. L., Hennessy, R. R., Tefft, B. J., Jana, S., Stoyles, N. J., et al. (2017). Recellularization of a Novel Off-The-Shelf Valve Following Xenogenic Implantation into the Right Ventricular Outflow Tract. PLoS ONE 12, e0181614. doi:10.1371/journal.pone.0181614
Hofmann, A. F. (1989). “Enterohepatic Circulation of Bile Acids,” in Handbook of Physiology, the Gastrointestinal System. Editor S G Schultz (Bethesda, MD: American Physiological Society), 567–596. doi:10.1002/cphy.cp060329
Hofmann, A. F., and Mysels, K. J. (1992). Bile Acid Solubility and Precipitation In Vitro and In Vivo: the Role of Conjugation, pH, and Ca2+ Ions. J. Lipid Res. 33, 617–626. doi:10.1016/s0022-2275(20)41426-9
Hofmann, M., Schmiady, M. O., Burkhardt, B. E., Dave, H. H., Hübler, M., Kretschmar, O., et al. (2017). Congenital Aortic Valve Repair Using CorMatrix : A Histologic Evaluation. Xenotransplantation 24, e12341. doi:10.1111/xen.12341
Hopkins, R. A., Bert, A. A., Hilbert, S. L., Quinn, R. W., Brasky, K. M., Drake, W. B., et al. (2013). Bioengineered Human and Allogeneic Pulmonary Valve Conduits Chronically Implanted Orthotopically in Baboons: Hemodynamic Performance and Immunologic Consequences. J. Thorac. Cardiovasc. Surg. 145, 1098–1107. doi:10.1016/j.jtcvs.2012.06.024
Horton, M. R., McKee, C. M., Bao, C., Liao, F., Farber, J. M., Hodge-DuFour, J., et al. (1998). Hyaluronan Fragments Synergize with Interferon-γ to Induce the C-X-C Chemokines Mig and Interferon-Inducible Protein-10 in Mouse Macrophages. J. Biol. Chem. 273, 35088. doi:10.1074/jbc.273.52.35088
Hung, S.-H., Su, C.-H., Lee, F.-P., and Tseng, H. (2013). Larynx Decellularization: Combining Freeze-Drying and Sonication as an Effective Method. J. Voice 27, 289–294. doi:10.1016/j.jvoice.2013.01.018
Hutás, G., Bajnok, É., Gál, I., Finnegan, A., Glant, T. T., and Mikecz, K. (2008). CD44-specific Antibody Treatment and CD44 Deficiency Exert Distinct Effects on Leukocyte Recruitment in Experimental Arthritis. Blood 112, 4999–5006. doi:10.1182/blood-2008-04-150383
Huygens, S. A., Goossens, L. M. A., van Erkelens, J. A., Takkenberg, J. J. M., and Rutten-van Mölken, M. P. M. H. (2018). How Much Does a Heart Valve Implantation Cost and what Are the Health Care Costs Afterwards?. Open Heart 5, e000672. doi:10.1136/openhrt-2017-000672
Huygens, S. A., Mokhles, M. M., Hanif, M., Bekkers, J. A., Bogers, A. J. J. C., Rutten-van Mölken, M. P. M. H., et al. (2016). Contemporary Outcomes after Surgical Aortic Valve Replacement with Bioprostheses and Allografts: a Systematic Review and Meta-Analysis. Eur. J. Cardiothorac. Surg. 50, 605–616. doi:10.1093/ejcts/ezw101
Hynes, R. O., and Naba, A. (2011). Overview of the Matrisome--An Inventory of Extracellular Matrix Constituents and Functions. Cold Spring Harbor Perspect. Biol. 4, a004903. doi:10.1101/cshperspect.a004903
Iop, L., Bonetti, A., Naso, F., Rizzo, S., Cagnin, S., Bianco, R., et al. (2014). Decellularized Allogeneic Heart Valves Demonstrate Self-Regeneration Potential after a Long-Term Preclinical Evaluation. PLoS One 9, e99593. doi:10.1371/journal.pone.0099593
Iwai, S., Torikai, K., Coppin, C. M., and Sawa, Y. (2007). Minimally Immunogenic Decellularized Porcine Valve Provides In Situ Recellularization as a Stentless Bioprosthetic Valve. J. Artif. Organs 10, 29–35. doi:10.1007/s10047-006-0360-1
Jing, W., and DeAngelis, P. L. (2004). Synchronized Chemoenzymatic Synthesis of Monodisperse Hyaluronan Polymers. J. Biol. Chem. 279, 42345–42349. doi:10.1074/jbc.M402744200
Jordan, R. E., Hewitt, N., Lewis, W., Kagan, H., and Franzblau, C. (1974). Regulation of Elastase-Catalyzed Hydrolysis of Insoluble Elastin by Synthetic and Naturally Occurring Hydrophobic Ligands. Biochemistry 13, 3497–3503. doi:10.1021/bi00714a013
Kagan, H. M., Crombie, G. D., Jordan, R. E., Lewis, W., and Franzblau, C. (1972). Proteolysis of Elastin-Ligand Complexes. Stimulation of Elastase Digestion of Insoluble Elastin by Sodium Dodecyl Sulfate. Biochemistry 11, 3412–3418. doi:10.1021/bi00768a014
Kasimir, M.-T., Weigel, G., Sharma, J., Rieder, E., Seebacher, G., Wolner, E., et al. (2005). The Decellularized Porcine Heart Valve Matrix in Tissue Engineering. Thromb. Haemost. 94, 562–567. doi:10.1160/TH05-01-0025
Kim, W. G., Park, J. K., and Lee, W. Y. (2002). Tissue-engineered Heart Valve Leaflets: an Effective Method of Obtaining Acellularized Valve Xenografts. Int. J. Artif. Organs 25, 791–797. doi:10.1177/039139880202500807
Knight, R. L., Booth, C., Wilcox, H. E., Fisher, J., and Ingham, E. (2005). Tissue Engineering of Cardiac Valves: Re-seeding of Acellular Porcine Aortic Valve Matrices with Human Mesenchymal Progenitor Cells. J. Heart Valve Dis. 14, 806–813.
Konakci, K., Bohle, B., Blumer, R., Hoetzenecker, W., Roth, G., Moser, B., et al. (2005). Alpha-Gal on Bioprostheses: Xenograft Immune Response in Cardiac Surgery. Eur. J. Clin. Invest. 35, 17–23. doi:10.1111/j.1365-2362.2005.01441.x
Konertz, W., Dohmen, P. M., Liu, J., Beholz, S., Dushe, S., Posner, S., et al. (2005). Hemodynamic Characteristics of the Matrix P Decellularized Xenograft for Pulmonary Valve Replacement during the Ross Operation. J. Heart Valve Dis. 14, 78–81.
Korossis, S. A., Booth, C., Wilcox, H. E., Watterson, K. G., Kearney, J. N., Fisher, J., et al. (2002). Tissue Engineering of Cardiac Valve Prostheses II: Biomechanical Characterization of Decellularized Porcine Aortic Heart Valves. J. Heart Valve Dis. 11, 463–471.
Koziarz, A., Makhdoum, A., Butany, J., Ouzounian, M., and Chung, J. (2020). Modes of Bioprosthetic Valve Failure: a Narrative Review. Curr. Opin. Cardiol. 35, 123–132. doi:10.1097/HCO.0000000000000711
Lee, J. Y., and Spicer, A. P. (2000). Hyaluronan: a Multifunctional, megaDalton, Stealth Molecule. Curr. Opin. Cel Biol. 12, 581–586. doi:10.1016/s0955-0674(00)00135-6
Leventhal, J. R., John, R., Fryer, J. P., Witson, J. C., Derlich, J. M., Remiszewski, J., et al. (1995). Removal of Baboon and Human Antiporcine IgG and IgM Natural Antibodies by Immunoadsorption. Results of In Vitro and In Vivo Studies. Transplantation 59, 294–300. doi:10.1097/00007890-199501000-00024
Leventhal, J. R., Dalmasso, A. P., Cromwell, J. W., Platt, J. L., Manivel, C. J., Bolman, R. M., et al. (1993). Prolongation of Cardiac Xenograft Survival by Depletion of Complement. Transplantation 55, 857–865. doi:10.1097/00007890-199304000-00033
Leyh, R. G., Wilhelmi, M., Walles, T., Kallenbach, K., Rebe, P., Oberbeck, A., et al. (2003). Acellularized Porcine Heart Valve Scaffolds for Heart Valve Tissue Engineering and the Risk of Cross-Species Transmission of Porcine Endogenous Retrovirus. J. Thorac. Cardiovasc. Surg. 126, 1000–1004. doi:10.1016/s0022-5223(03)00353-2
Lin, P., Chan, W. C. W., Badylak, S. F., and Bhatia, S. N. (2004). Assessing Porcine Liver-Derived Biomatrix for Hepatic Tissue Engineering. Tissue Eng. 10, 1046–1053. doi:10.1089/ten.2004.10.1046
Londono, R., Dziki, J. L., Haljasmaa, E., Turner, N. J., Leifer, C. A., and Badylak, S. F. (2017). The Effect of Cell Debris within Biologic Scaffolds upon the Macrophage Response. J. Biomed. Mater. Res. 105, 2109–2118. doi:10.1002/jbm.a.36055
Loniewski, K. J., Patial, S., and Parameswaran, N. (2007). Sensitivity of TLR4- and -7-induced NFκB1 P105-TPL2-ERK Pathway to TNF-Receptor-Associated-Factor-6 Revealed by RNAi in Mouse Macrophages. Mol. Immunol. 44, 3715–3723. doi:10.1016/j.molimm.2007.04.002
Luo, J., Korossis, S. A., Wilshaw, S.-P., Jennings, L. M., Fisher, J., and Ingham, E. (2014). Development and Characterization of Acellular Porcine Pulmonary Valve Scaffolds for Tissue Engineering. Tissue Eng. A 20, 2963–2974. doi:10.1089/ten.tea.2013.0573
Malone, J. M., Brendel, K., Duhamel, R. C., and Reinert, R. L. (1984). Detergent-extracted Small-Diameter Vascular Prostheses. J. Vasc. Surg. 1, 181–191. doi:10.1067/mva.1984.avs0010181
Mangold, A., Szerafin, T., Hoetzenecker, K., Hacker, S., Lichtenauer, M., Niederpold, T., et al. (2009). Alpha-Gal Specific IgG Immune Response after Implantation of Bioprostheses. Thorac. Cardiovasc. Surg. 57, 191–195. doi:10.1055/s-0029-1185395
McKee, C. M., Penno, M. B., Cowman, M., Burdick, M. D., Strieter, R. M., Bao, C., et al. (1996). Hyaluronan (HA) Fragments Induce Chemokine Gene Expression in Alveolar Macrophages. The Role of HA Size and CD44. J. Clin. Invest. 98, 2403–2413. doi:10.1172/JCI119054
Mendibil, U., Ruiz-Hernandez, R., Retegi-Carrion, S., Garcia-Urquia, N., Olalde-Graells, B., and Abarrategi, A. (2020). Tissue-Specific Decellularization Methods: Rationale and Strategies to Achieve Regenerative Compounds. Ijms 21, 5447. doi:10.3390/ijms21155447
Meyer, S. R., Nagendran, J., Desai, L. S., Rayat, G. R., Churchill, T. A., Anderson, C. C., et al. (2005). Decellularization Reduces the Immune Response to Aortic Valve Allografts in the Rat. J. Thorac. Cardiovasc. Surg. 130, 469–476. doi:10.1016/j.jtcvs.2005.03.021
Mirsadraee, S., Wilcox, H. E., Korossis, S. A., Kearney, J. N., Watterson, K. G., Fisher, J., et al. (2006). Development and Characterization of an Acellular Human Pericardial Matrix for Tissue Engineering. Tissue Eng. 12, 763–773. doi:10.1089/ten.2006.12.763
Naba, A., Clauser, K. R., Hoersch, S., Liu, H., Carr, S. A., and Hynes, R. O. (2011). The Matrisome: In Silico Definition and In Vivo Characterization by Proteomics of normal and Tumor Extracellular Matrices. Mol. Cel. Proteomics 11, M111014647. doi:10.1074/mcp.M111.014647
Naso, F., Aguiari, P., and Iop, L. (2013a). Biocompatibility Evaluation Criteria for Novel Xenograft Materials: Distribution and Quantification of Remnant Nucleic Acid and Alpha-Gal Epitope. J. Stem Cel Res. Ther.S6.. doi:10.4172/2157-7633.S6-009
Naso, F., Gandaglia, A., Bottio, T., Tarzia, V., Nottle, M. B., d'Apice, A. J. F., et al. (2013b). First Quantification of Alpha-Gal Epitope in Current Glutaraldehyde-Fixed Heart Valve Bioprostheses. Xenotransplantation 20, 252–261. doi:10.1111/xen.12044
Naso, F., and Gandaglia, A. (2018). Different Approaches to Heart Valve Decellularization: A Comprehensive Overview of the Past 30 Years. Xenotransplantation 25, e12354. doi:10.1111/xen.12354
Naso, F., Iop, L., Spina, M., and Gerosa, G. (2014). Are FDA and CE Sacrificing Safety for a Faster Commercialization of Xenogeneic Tissue Devices? Unavoidable Need for Legislation in Decellularized Tissue Manufacturing. Tissue Antigens 83, 193–194. doi:10.1111/tan.12275
Naso, F., Stefanelli, U., Buratto, E., Lazzari, G., Perota, A., Galli, C., et al. (2017). Alpha-Gal Inactivated Heart Valve Bioprostheses Exhibit an Anti-calcification Propensity Similar to Knockout Tissues. Tissue Eng. Part A 23, 1181–1195. doi:10.1089/ten.tea.2016.0474
Nonaka, P. N., Campillo, N., Uriarte, J. J., Garreta, E., Melo, E., de Oliveira, L. V. F., et al. (2013). Effects of Freezing/thawing on the Mechanical Properties of Decellularized Lungs. J. Biomed. Mater. Res. 102, 413–419. doi:10.1002/jbm.a.34708
O'Brien, M. F., Goldstein, S., Walsh, S., Black, K. S., Elkins, R., and Clarke, D. (1999). The SynerGraft Valve: a New Acellular (Nonglutaraldehyde-fixed) Tissue Heart Valve for Autologous Recellularization First Experimental Studies before Clinical Implantation. Semin. Thorac. Cardiovasc. Surg. 11, 194–200.
Ota, T., Sawa, Y., Iwai, S., Kitajima, T., Ueda, Y., Coppin, C., et al. (2005). Fibronectin-hepatocyte Growth Factor Enhances Reendothelialization in Tissue-Engineered Heart Valve. Ann. Thorac. Surg. 80, 1794–1801. doi:10.1016/j.athoracsur.2005.05.002
Padalino, M. A., Castaldi, B., Fedrigo, M., Gallo, M., Zucchetta, F., Vida, V. L., et al. (2016). Porcine Intestinal Submucosa (CorMatrix) for Semilunar Valve Repair in Children: A Word of Caution after Midterm Results. Semin. Thorac. Cardiovasc. Surg. 28, 436–445. doi:10.1053/j.semtcvs.2016.04.015
Park, C. S., Park, S. S., Choi, S. Y., Yoon, S. H., Kim, W. H., and Kim, Y. J. (2010). Anti Alpha-Gal Immune Response Following Porcine Bioprosthesis Implantation in Children. J. Heart Valve Dis. 19, 124–130.
Perri, G., Polito, A., Esposito, C., Albanese, S. B., Francalanci, P., Pongiglione, G., et al. (2012). Early and Late Failure of Tissue-Engineered Pulmonary Valve Conduits Used for Right Ventricular Outflow Tract Reconstruction in Patients with Congenital Heart Disease. Eur. J. Cardio-Thoracic Surg. 41, 1320–1325. doi:10.1093/ejcts/ezr221
Pham, T., Gregg, C. J., Karp, F., Chow, R., Padler-Karavani, V., Cao, H., et al. (2009). Evidence for a Novel Human-specific Xeno-Auto-Antibody Response against Vascular Endothelium. Blood 114, 5225–5235. doi:10.1182/blood-2009-05-220400
Poncelet, A., Fervaille, C., de Kerchove, L., Gianello, P., and Mosala Nezhad, Z. (2017). Experimental Aortic Valve Cusp Extension with Cormatrix in a Porcine Model. Thorac. Cardiovasc. Surg. 65, 206–210. doi:10.1055/s-0036-1586756
Poon, C. J., Pereira E. Cotta, M. V., Sinha, S., Palmer, J. A., Woods, A. A., Morrison, W. A., et al. (2013). Preparation of an Adipogenic Hydrogel from Subcutaneous Adipose Tissue. Acta Biomater. 9, 5609–5620. doi:10.1016/j.actbio.2012.11.003
Prehm, P. (1984). Hyaluronate Is Synthesized at Plasma Membranes. Biochem. J. 220, 597–600. doi:10.1042/bj2200597
Quint, C., Kondo, Y., Manson, R. J., Lawson, J. H., Dardik, A., and Niklason, L. E. (2011). Decellularized Tissue-Engineered Blood Vessel as an Arterial Conduit. Proc. Natl. Acad. Sci. 108, 9214–9219. doi:10.1073/pnas.1019506108
Rajab, T. K., O’Malley, T. J., and Tchantchaleishvili, V. (2020). Decellularized Scaffolds for Tissue Engineering: Current Status and Future Perspective. Artif. Organs. 44, 1031–1043. doi:10.1111/aor.13701
Rajani, B., Mee, R. B., and Ratliff, N. B. (1998). Evidence for Rejection of Homograft Cardiac Valves in Infants. J. Thorac. Cardiovasc. Surg. 115, 111–117. doi:10.1016/s0022-5223(98)70449-0
Rayahin, J. E., Buhrman, J. S., Zhang, Y., Koh, T. J., and Gemeinhart, R. A. (2015). High and Low Molecular Weight Hyaluronic Acid Differentially Influence Macrophage Activation. ACS Biomater. Sci. Eng. 1, 481–493. doi:10.1021/acsbiomaterials.5b00181
Reing, J. E., Brown, B. N., Daly, K. A., Freund, J. M., Gilbert, T. W., Hsiong, S. X., et al. (2010). The Effects of Processing Methods upon Mechanical and Biologic Properties of Porcine Dermal Extracellular Matrix Scaffolds. Biomaterials 31, 8626–8633. doi:10.1016/j.biomaterials.2010.07.083
Rieder, E., Kasimir, M.-T., Silberhumer, G., Seebacher, G., Wolner, E., Simon, P., et al. (2004). Decellularization Protocols of Porcine Heart Valves Differ Importantly in Efficiency of Cell Removal and Susceptibility of the Matrix to Recellularization with Human Vascular Cells. J. Thorac. Cardiovasc. Surg. 127, 399–405. doi:10.1016/j.jtcvs.2003.06.017
Rüffer, A., Purbojo, A., Cicha, I., Glöckler, M., Potapov, S., Dittrich, S., et al. (2010). Early Failure of Xenogenous De-cellularised Pulmonary Valve Conduits - a Word of Caution!☆. Eur. J. Cardio-Thoracic Surg. 38, 78–85. doi:10.1016/j.ejcts.2010.01.044
Ruiz, C. E., Iemura, M., Medie, S., Varga, P., Van Alstine, W. G., Mack, S., et al. (2005). Transcatheter Placement of a Low-Profile Biodegradable Pulmonary Valve Made of Small Intestinal Submucosa: a Long-Term Study in a Swine Model. J. Thorac. Cardiovasc. Surg. 130, e1–477. doi:10.1016/j.jtcvs.2005.04.008
Saadi, S., and Platt, J. L. (1995). Transient Perturbation of Endothelial Integrity Induced by Natural Antibodies and Complement. J. Exp. Med. 181, 21–31. doi:10.1084/jem.181.1.21
Schenke-Layland, K., Vasilevski, O., Opitz, F., König, K., Riemann, I., Halbhuber, K. J., et al. (2003). Impact of Decellularization of Xenogeneic Tissue on Extracellular Matrix Integrity for Tissue Engineering of Heart Valves. J. Struct. Biol. 143, 201–208. doi:10.1016/j.jsb.2003.08.002
Schussler, O., Lila, N., Grau, J., Ruel, M., Lecarpentier, Y., and Carpentier, A. (2020). Possible Link between the ABO Blood Group of Bioprosthesis Recipients and Specific Types of Structural Degeneration. J. Am. Heart Assoc. 9, e015909. doi:10.1161/JAHA.119.015909
Senior, R. M., Griffin, G. L., and Mecham, R. P. (1980). Chemotactic Activity of Elastin-Derived Peptides. J. Clin. Invest. 66, 859–862. doi:10.1172/JCI109926
Shinkawa, T., Lu, C. K., Chipman, C., Tang, X., Gossett, J. M., and Imamura, M. (2015). The Midterm Outcomes of Bioprosthetic Pulmonary Valve Replacement in Children. Semin. Thorac. Cardiovasc. Surg. 27, 310–318. doi:10.1053/j.semtcvs.2015.07.010
Simionescu, A., Simionescu, D. T., and Vyavahare, N. R. (2007). Osteogenic Responses in Fibroblasts Activated by Elastin Degradation Products and Transforming Growth Factor-Β1. Am. J. Pathol. 171, 116–123. doi:10.2353/ajpath.2007.060930
Simionescu, D., Harpa, M. M., Harpa, M. M., Oprita, C., Movileanu, I., and Simionescu, A. (2021). Tissue Engineering Heart Valves - a Review of More Than Two Decades into Preclinical and Clinical Testing for Obtaining the Next Generation of Heart Valve Substitutes. Rom. J. Cardio 31, 501–510. doi:10.47803/rjc.2021.31.3.501
Simon, P., Kasimir, M. T., Seebacher, G., Weigel, G., Ullrich, R., Muhar, U. S., et al. (2003). Early Failure of the Tissue Engineered Porcine Heart Valve SYNERGRAFT in Pediatric Patients. Eur. J. Cardio-Thoracic Surg. 23, 1002–1006. doi:10.1016/s1010-7940(03)00094-0
Smood, B., Hara, H., Cleveland, D. C., and Cooper, D. K. C. (2019). In Search of the Ideal Valve: Optimizing Genetic Modifications to Prevent Bioprosthetic Degeneration. Ann. Thorac. Surg. 108, 624–635. doi:10.1016/j.athoracsur.2019.01.054
Somuncu, Ö. S. (2019). Decellularization Concept in Regenerative Medicine. Adv. Exp. Med. Biol. 1212, 71–85. doi:10.1007/5584_2019_338
Soto-Gutierrez, A., Wertheim, J. A., Ott, H. C., and Gilbert, T. W. (2012). Perspectives on Whole-Organ Assembly: Moving toward Transplantation on Demand. J. Clin. Invest. 122, 3817–3823. doi:10.1172/JCI61974
Syed, O., Walters, N. J., Day, R. M., Kim, H.-W., and Knowles, J. C. (2014). Evaluation of Decellularization Protocols for Production of Tubular Small Intestine Submucosa Scaffolds for Use in Oesophageal Tissue Engineering. Acta Biomater. 10, 5043–5054. doi:10.1016/j.actbio.2014.08.024
Takagi, K., Fukunaga, S., Nishi, A., Shojima, T., Yoshikawa, K., Hori, H., et al. (2006). In Vivo recellularization of plain Decellularized Xenografts with Specific Cell Characterization in the Systemic Circulation: Histological and Immunohistochemical Study. Artif. Organs 30, 233–241. doi:10.1111/j.1525-1594.2006.00210.x
Tavassoli, A., Matin, M. M., Niaki, M. A., Mahdavi-Shahri, N., and Shahabipour, F. (2015). Mesenchymal Stem Cells Can Survive on the Extracellular Matrix-Derived Decellularized Bovine Articular Cartilage Scaffold. Iran J. Basic Med. Sci. 18, 1221–1227.
Termeer, C. C., Hennies, J., Voith, U., Ahrens, T., M. Weiss, J., Prehm, P., et al. (2000). Oligosaccharides of Hyaluronan Are Potent Activators of Dendritic Cells. J. Immunol. 165, 1863–1870. doi:10.4049/jimmunol.165.4.1863
Toumpaniari, S., Hilfiker, A., Haverich, A., and Korossis, S. (2020). Tissue-Engineered Vascular Grafts. Springer International Publishing.
U.S. Food & Drug Administration (2020). FDA Approves First-Of-Its-Kind Intentional Genomic Alteration in Line of Domestic Pigs for Both Human Food, Potential Therapeutic Uses. Available at: : https://www.fda.gov/news-events/press-announcements/fda-approves-first-its-kind-intentional-genomic-alteration-line-domestic-pigs-both-human-food (Accessed December 14, 2020).
Vafaee, T., Thomas, D., Desai, A., Jennings, L. M., Berry, H., Rooney, P., et al. (2018). Decellularization of Human Donor Aortic and Pulmonary Valved Conduits Using Low Concentration Sodium Dodecyl Sulfate. J. Tissue Eng. Regen. Med. 12, e841–e853. doi:10.1002/term.2391
van Rijswijk, J. W., Talacua, H., Mulder, K., van Hout, G. P. J., Bouten, C. V. C., Gründeman, P. F., et al. (2020). Failure of Decellularized Porcine Small Intestinal Submucosa as a Heart Valved Conduit. J. Thorac. Cardiovasc. Surg. 160, e201–e215. doi:10.1016/j.jtcvs.2019.09.164
Veiseh, M., and Turley, E. A. (2011). Hyaluronan Metabolism in Remodeling Extracellular Matrix: Probes for Imaging and Therapy of Breast Cancer. Integr. Biol. (Camb) 3, 304–315. doi:10.1039/c0ib00096e
Voges, I., Bräsen, J. H., Entenmann, A., Scheid, M., Scheewe, J., Fischer, G., et al. (2013). Adverse Results of a Decellularized Tissue-Engineered Pulmonary Valve in Humans Assessed with Magnetic Resonance Imaging. Eur. J. Cardiothorac. Surg. 44, e272–e279. doi:10.1093/ejcts/ezt328
Waletzko, J., Dau, M., Seyfarth, A., Springer, A., Frank, M., Bader, R., et al. (2020). Devitalizing Effect of High Hydrostatic Pressure on Human Cells-Influence on Cell Death in Osteoblasts and Chondrocytes. Ijms 21, 3836. doi:10.3390/ijms21113836
White, L. J., Taylor, A. J., Faulk, D. M., Keane, T. J., Saldin, L. T., Reing, J. E., et al. (2017). The Impact of Detergents on the Tissue Decellularization Process: A ToF-SIMS Study. Acta Biomater. 50, 207–219. doi:10.1016/j.actbio.2016.12.033
Wilson, G. J., Courtman, D. W., Klement, P., Lee, J. M., and Yeger, H. (1995). Acellular Matrix: a Biomaterials Approach for Coronary Artery Bypass and Heart Valve Replacement. Ann. Thorac. Surg. 60, S353–S358. doi:10.1016/0003-4975(95)98967-y
Wilson, N. S., Dixit, V., and Ashkenazi, A. (2009). Death Receptor Signal Transducers: Nodes of Coordination in Immune Signaling Networks. Nat. Immunol. 10, 348–355. doi:10.1038/ni.1714
Woo, J. S., Fishbein, M. C., and Reemtsen, B. (2016). Histologic Examination of Decellularized Porcine Intestinal Submucosa Extracellular Matrix (CorMatrix) in Pediatric Congenital Heart Surgery. Cardiovasc. Pathol. 25, 12–17. doi:10.1016/j.carpath.2015.08.007
Woods, T., and Gratzer, P. F. (2005). Effectiveness of Three Extraction Techniques in the Development of a Decellularized Bone-Anterior Cruciate Ligament-Bone Graft. Biomaterials 26, 7339–7349. doi:10.1016/j.biomaterials.2005.05.066
Keywords: biocompatibility, heart valve decellularization, protocols standardization, xenoantigens removal, regenerative medicine
Citation: Naso F and Gandaglia A (2022) Can Heart Valve Decellularization Be Standardized? A Review of the Parameters Used for the Quality Control of Decellularization Processes. Front. Bioeng. Biotechnol. 10:830899. doi: 10.3389/fbioe.2022.830899
Received: 07 December 2021; Accepted: 31 January 2022;
Published: 17 February 2022.
Edited by:
Sotirios Korossis, Loughborough University, United KingdomReviewed by:
Katsuhiro Hosoyama, Iwate Prefectural Central Hospital, JapanCopyright © 2022 Naso and Gandaglia. This is an open-access article distributed under the terms of the Creative Commons Attribution License (CC BY). The use, distribution or reproduction in other forums is permitted, provided the original author(s) and the copyright owner(s) are credited and that the original publication in this journal is cited, in accordance with accepted academic practice. No use, distribution or reproduction is permitted which does not comply with these terms.
*Correspondence: F. Naso, ZmlsaXBwby5uYXNvQGJpb2NvbXBhdGliaWxpdHkuYmlv
†These authors have contributed equally to this work and share first authorship
Disclaimer: All claims expressed in this article are solely those of the authors and do not necessarily represent those of their affiliated organizations, or those of the publisher, the editors and the reviewers. Any product that may be evaluated in this article or claim that may be made by its manufacturer is not guaranteed or endorsed by the publisher.
Research integrity at Frontiers
Learn more about the work of our research integrity team to safeguard the quality of each article we publish.