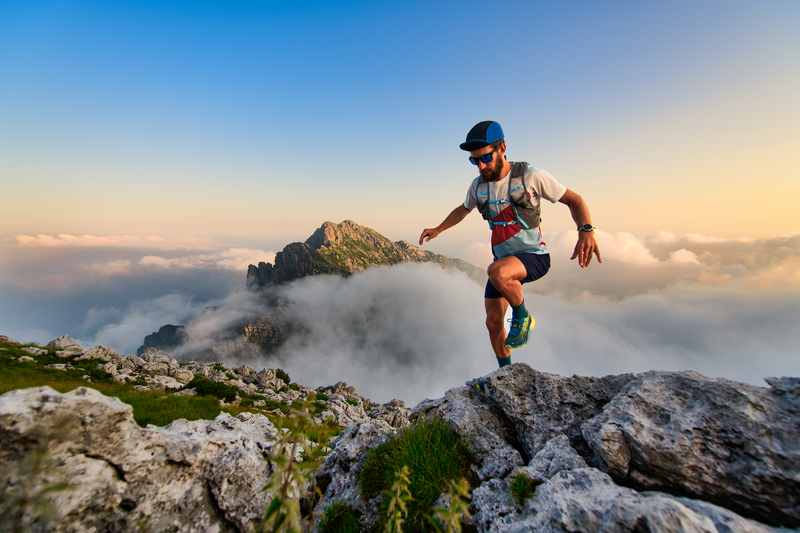
94% of researchers rate our articles as excellent or good
Learn more about the work of our research integrity team to safeguard the quality of each article we publish.
Find out more
REVIEW article
Front. Bioeng. Biotechnol. , 13 January 2023
Sec. Biomaterials
Volume 10 - 2022 | https://doi.org/10.3389/fbioe.2022.1100892
Exosomes are the smallest extracellular vesicles that can be released by practically all cell types, and range in size from 30 nm to 150 nm. As the major marker of liquid biopsies, exosomes have great potential for disease diagnosis, therapy, and prognosis. However, their inherent heterogeneity, the complexity of biological fluids, and the presence of nanoscale contaminants make the isolation of exosomes a great challenge. Traditional isolation methods of exosomes are cumbersome and challenging with complex and time-consuming operations. In recent years, the emergence of microfluidic chips, nanolithography, electro-deposition, and other technologies has promoted the combination and innovation of the isolation methods. The application of these methods has brought very considerable benefits to the isolation of exosomes such as ultra-fast, portable integration, and low loss. There are significant functional improvements in isolation yield, isolation purity, and clinical applications. In this review, a series of methods for the isolation of exosomes are summarized, with emphasis on the emerging methods, and in-depth comparison and analysis of each method are provided, including their principles, merits, and demerits.
Exosomes are membranous vesicles released by intracellular multivesicular bodies that can be involved in intercellular communication, angiogenesis (Geng et al., 2020), and tumor formation (Li et al., 2021). They play an important role in the early diagnosis of cancer (Wu et al., 2021), infectious diseases (Shrivastava et al., 2021), and cardiovascular diseases (Neves et al., 2022). Although biological properties and functional characteristics of exosomes have been intensively studied, the high heterogeneity of exosomes in terms of size, cargo, and origin (Han et al., 2021a), as well as the presence of nanoscale contaminants such as lipoproteins, retroviruses, etc., have limited the development of methods for isolation and purification of exosomes.
So far, the methods for exosome isolation are constantly improving. The traditional methods mainly include differential ultracentrifugation, size-based isolation, and polymer-based precipitation. These methods can be used to handle large volume samples or to obtain high purity samples. However, high yield and purity cannot be guaranteed by most exosome isolation methods. Some isolation methods are not only tedious and costly, but also require specialized instruments. Like ultracentrifugation, exosomes are easily damaged mechanically under the action of centrifugal force, and it is difficult to effectively maintain the bioactivity and morphological integrity of exosomes.
In recent years, emerging methods and technologies, such as microfluidics (Hassanpour Tamrin et al., 2021), nanolithography, electro-deposition, immunomagnetic beads, and covalent chemistry, have significant effects on isolation of exosomes (Figure 1). As an example, field flow isolation (Kim et al., 2022a), label-free magnetic isolation (Zeng et al., 2021), and functional micro/nanostructures (Lim et al., 2022) can be used to extract exosomes efficiently. Meanwhile, microfluidic devices have been developed to integrate the isolation and analysis of exosomes into a platform where exosomes can be directly characterized and analyzed for downstream genomics and proteomics. In addition, the extracellular vesicles on demand (EVOD) chip can be used to isolate and analyze lung cancer derived exosomes (Kang et al., 2020).
FIGURE 1. The emerging isolation methods and technologies and the hallmarkers of exosomes. Exosomes are usually found to express markers like CD63, CD81, CD9, HSP70, HSP90, TSG101, and Alix. Currently, exosomes can be isolated by a variety of methods and technologies such as nanolithography, electro-deposition, immunomagnetic beads, covalent chemistry, DNAzyme probes, and negative magneticelectrophoresis to achieve the isolation efficiency of simple and rapid, high yield and purity, time and cost-saving.
While ensuring high yield and purity, the bioactivity and structural integrity of exosomes can be maintained. Only on the basis of good separation products can exosomes be effectively studied. Therefore, the selection of appropriate separation technique is the prerequisite of exosome research. In this review, the improvements and innovations on the traditional methods, such as dichotomic size-exclusion chromatography (Guo et al., 2021a), ultracentrifugation in combination with size-exclusion chromatography (Vergauwen et al., 2021) are introduced. The emerging methods proposed in the last two years are elaborated, such as label-free separation of nanoparticles based on the principle of particle ferrohydrodynamics (Liu et al., 2020) and separation by DSPE-PEG derivatives in combined with biotin-avidin systems (Wei et al., 2021).
Traditional methods for exosome isolation include ultracentrifugation-based methods, size-based methods (size-exclusion chromatography and ultrafiltration), precipitation, and immunoaffinity capture. The first three methods are mainly based on the size and density of exosomes, and the last method is mainly based on the specific binding between antibodies or aptamers and exosomal signature proteins. The comparison of each method is summarized in Table 1. On this basis, many researchers have integrated and innovated on the existing methods. For example, size-exclusion chromatography combined with iodixanol density gradient centrifugation was used for isolation, aiming to achieve high yield and purity (Alameldin et al., 2021).
Differential ultracentrifugation is considered to be the “gold standard” method for exosome isolation, which can effectively isolate microscopic particles such as bacteria, organelles, and exosomes (Ter-Ovanesyan et al., 2021). To minimize the effect of Tamm-Horsfall protein polymer on the yield and purity of urinary extracellular vesicles, differential ultracentrifugation combines Tamm-Horsfall protein polymer reduction and alkaline washing to reduce common protein contaminations (Correll et al., 2022). Density gradient centrifugation (Langevin et al., 2019) is a time-consuming method due to the high-density medium (sucrose or iodixanol, etc.) is added to the layer which can be used to isolate extracellular components (such as exosomes, apoptotic vesicles, and protein aggregates). One-step sucrose cushion ultracentrifugation can be used to achieve the extraction of MSCs-derived exosomes (Gupta et al., 2018). Li et al. (2018) and Luo et al. (2021) have improved this method to maximize the recovery and purity of exosomes while minimizing the exosome damage. In contrast to sucrose-based gradient centrifugation, the isolation of subpopulations of extracellular vesicles can be achieved by high-resolution iodixanol density gradient centrifugation more efficiently (D'Acunzo et al., 2022).
To improve the isolation purity, researchers have tried to combine ultracentrifugation and ultrafiltration with size-exclusion chromatography for the isolation of exosomes (Guan et al., 2020; Shu et al., 2021; Turner et al., 2022). Studies have shown that ultrafiltration combined with size-exclusion chromatography can reduce the level of impurity cytokine (interleukin-10) in isolated exosomes. The combination of various methods can effectively reduce the presence of nanoscale contaminants, improve the separation purity, while maintaining the natural properties of exosomes. In addition to methods combination, other researchers have proposed dichotomic size-exclusion chromatography for exosome isolation, and found that CL-6B 20 mL column had the best performance with higher isolation yield and tighter EVs peaks (Figure 2) (Guo et al., 2021a). Nowadays, qEV size-exclusion columns exist on the market. Highly active exosomes can be extracted from a wide range of samples within 15 min based on the principle of molecular particle size segmentation (Cardoso et al., 2021). Besides, studies have shown that tangential flow filtration is more suitable for ultrafiltration processes than traditional dead-end filtration (Han et al., 2021b; Kim et al., 2021). In tangential flow filtration, the potential clogging can be effectively reduced, and thorough filtration under the effect of parallel flow dynamics can be ensured. Precipitation-based and immunoaffinity-based kits have been widely used for exosome isolation (Kamei et al., 2021; Mondal and Whiteside, 2021). Furthermore, polysaccharide chitosan could facilitate the isolation of small extracellular vesicles (Kumar et al., 2021). And researchers (Crescitelli et al., 2021) have summarized the process of isolating and characterizing subpopulations of EVs from tissues.
FIGURE 2. Establishment of dichotomic SEC. The dichotomic SEC separation with 2 bulk elution steps was sufficient for the isolation of the extracellular vesicle. When the packing material is CL-6B and the bed volume is 20 mL, it will be the best separation performance. Reproduced with permission from (Guo et al., 2021a).
Although traditional isolation methods of exosomes such as ultracentrifugation and kit extraction are widely used for scientific research, these methods have certain limitations such as low isolation yield and purity, time-consuming. The practice of exosomes in clinical applications (such as point-of-care testing, POCT) is seriously hindered. In recent years, with the development of microfluidic chips (Lu et al., 2022), it has become possible to handle or manipulate small volumes of liquid in microplates and microchannels. Microfluidic devices are used to achieve the integration of isolation and identification on a microchip (Ullah Khan et al., 2021). In addition, the multidirectional reference of the development of exosome isolation techniques can be provided by applications of nanomaterials, immunomagnetic beads, and covalent chemistry. They play an important role in improving the isolation yield and purity and maintaining the bioactivity of exosomes. The principles, advantages, and disadvantages of each method are summarized in Table 2.
The microfluidic technology refers to the manipulation and processing of tiny fluids in micropores. The isolation and characterization of exosomes in a single step process are allowed, with microfabrication technologies to develop chip-based microfluidic systems. The comprehensive microfluidic devices based on immunoaffinity capture (Mondal and Whiteside, 2021), size-exclusion chromatography (μSEC) (Leong et al., 2022), and ultracentrifugation (Kang et al., 2021) have been developed for the efficient isolation of exosomes. The rapid isolation of exosomes from minute volumes of biofluids, real-time characterization, and in situ diagnosis of exosomes can be realized by the combination of microfluidic devices and signal detection platforms. It is important for the non-invasive disease detection. The advantages of microfluidics are convenience, high efficiency, ease of automation, integration, and portability. However, the sample throughput is low because the sample is fed in the fingertip volume. The microfluidic devices can also be combined with exponential isothermal amplification (EXPAR) of miRNAs (Qian et al., 2022) for the isolation and identification of exosomes. Meanwhile, integrated microfluidic platforms consisting of a single-cell capture chip and a spatially coded antibody barcode chip can also be used to explore single-cell heterogeneity (Song et al., 2022).
Field flow fractionation can be used to isolate macromolecules (Manning et al., 2021). The molecules passing through a flat channel are subjected to both horizontal and vertical flow fields. When the flat channel is closed above and only open below is called Asymmetric Flow Field-Flow Fractionation (AF4). AF4 is mainly based on the size to isolate nanoscale soluble particles (Wu et al., 2020). There is a high correlation between the particle size and diffusion coefficient (Zhang and Lyden, 2019). The isolation of particles can be affected by diffusion coefficient, so that the key of the isolation of AF4 is the particle size. The bottom of AF4 is a semi-permeable membrane with the specific size. The molecules with sizes smaller than the cut-off size can pass through the semi-permeable membrane, and components larger than the cut-off size are retained. Studies have shown that AF4 can identify particles from the nanometer to the micrometer scale, and has a large dynamic range and high resolution, with a resolution of up to 1 nm (Marioli et al., 2019).
Compared with other isolation methods, AF4 is no labeling, no magnetic beads, and has a high reproducibility. However, AF4 can only be used to process small volume samples and cannot be used to screen particles with same size and different morphology. The complicated production process is a stumbling block to clinical applications (Berger et al., 2021). Furthermore, asymmetric depth-filtration (DF) can isolate extracellular vesicles by immobilizing extracellular vesicles on the surface and within the depth of porous medium, then recovering them by reversing the carrier flow through the filter. Compared to the optimized three-step isolation procedure, DF is more suitable for the separation of plasma extracellular vesicles (Chernyshev et al., 2022).
Deterministic Lateral Displacement (DLD) is particle size-based isolation, and can be used for the isolation of exosomes (Hochstetter et al., 2020). The system consists of an ordered array of microcolumns, where the gaps and offsets between microcolumns determine the diameter of particles that can pass through. The cut-off size parameter between the serrated and displaced modes in DLD is called DLD critical diameter (Dc) and is mainly determined by the column shape, the column gap, and the array gradient (Ho et al., 2020). Biofluids flow through the array. When the particle size is smaller than the critical size, particles will follow the initial streamline through the array gap. When the particle size is larger than the critical size, particles are laterally displaced by the column impact in a cross-order flow line. Thanks to the continuous flow column array gradient, DLD is widely used for the isolation of bacteria, viruses, and yeast.
However, due to the fluid dynamics involved at the micron or even nanoscale, it is more difficult in fabrication and sorting. Smith et al. (2018) refined on a nanoDLD chip with an elevated fluid flow rate of ∼900 μL/h compared to conventional arrays. As early as 2016, Zeming KK et al. designed a DLD device for the isolation of nanoparticles, mainly by varying the concentration of salt ions to modulate the interaction between nanostructures and particles. To a certain extent, the bioactivity and morphological integrity of exosomes can be maintained. In addition, exosomes can be isolated by DLD with high separation yield and low consumption, and without labeling. However, the chip is more prone to clogging than conventional isolation methods, resulting in lower isolation purity.
Dielectrophoretic isolation (DEP) is based on the translational motion of neutral particles subjected to dielectric polarization in a non-uniform electric field, including positive and negative dielectrophoresis. Smaller particles enter the DEP high field region around the edges of microelectrodes, and larger particles enter the DEP low field region between electrodes. In an inhomogeneous symmetric electric field, particles are subjected to different local electric field strengths on both sides, generating an electrostatic force, i.e., the dielectric electrophoretic force. The dielectric electrophoretic force is determined by the size of suspended particles, dielectric constant, and electric field strength. Since the suspended particles and the medium have different dielectric constants, particles will move in the direction of a stronger or weaker electric field. Compared with other separation methods, DEP is more selective and controllable for the rapid separation of exosomes, and no labeling (Tayebi et al., 2021; Zhang et al., 2022a).
To extend the isolation efficacy of DEP, Ayala-Mar et al. (2019) proposed a DC insulator-based dielectrophoresis (DC-iDEP). iDEP is a variant of DEP that can isolate particles based on the size. Since the insertion of electrodes in the chip is not required, the fabrication process is greatly simplified (Shi et al., 2018). Researchers designed a microdevice consisting of two electrically insulated column fraction channels, and the isolation efficiency of exosomes can be assessed by analyzing the particle size of the recovered fraction in DC-iDEP. Although iDEP can capture exosomes efficiently and differentiate subpopulations of exosomes to a certain extent, it is undeniable that the isolation purity is low and the device is subject to overheating. Zhao et al. (2021) proposed an ExoDEP-chip microfluidic device which contains a large number of microwells in the DEP chamber with forked-finger DEP electrodes, and single polystyrene (PS) microsphere is immobilized in the micropores. Exosome capture is achieved by pre-conjugated antibodies on the PS surface. ExoDEP-chip is more flexible and less complex in terms of the equipment and operation.
Additionally, a label-free magnetic isolation system that can be used to extract small extracellular vesicles (sEVs) from cell culture supernatants based on negative magnetoelectrophoresis was presented (Zeng et al., 2022). The strong magnetic field within the microchannel is generated by Four NdFeB magnets (N52). Particles are concentrated in the isolation channel by sheath flow and are repelled by the magnetic pole array into the center of isolation channel by the strong magnetic force, with particles of different sizes separated in different exits. Higher recovery and purity can be achieved by using biocompatible ferromagnetic fluids with recovery up to 85.80% and separation purity up to 80.45% compared with other isolation methods. Since the isolation of sEVs by negative magnetoelectrophoresis is performed in the magnetic solution, which is likely to affect the bioactivity of sEVs, so the magnetic solution is highly required.
Acoustic Fractionation can isolate particles based on the size and density (Wang et al., 2021). Particles are subjected to acoustic standing waves (SAW) in the micro channel. Acoustic radiation force and Stokes resistance can be generated. For larger particles, the acoustic radiation force dominates, and for smaller particles, the Stokes resistance dominates. Acoustic fractionation is no labeling and simple to operate. Relevant studies have shown that exosomes can be separated from biofluids with a purity of up to 98% and a separation rate of up to 82%. Wu et al. (2017) designed an acoustic flow control platform integrated onto a single chip that can isolate exosomes directly from blood samples. The salivary exosomes can be directly isolated by the device (Figure 3) (Wang et al., 2020). However, its clinical application is limited by the need for specialized instrumentation. With the development of single vesicle technique, researchers proposed that single vesicle technique combined with atomic force microscopy (AFM) and ultrasensitive TiN-NH-localized surface plasmon resonance (LSPR) biosensors could be used to characterize and quantify exosome-associated proteins for liquid biopsy in vivo (Silva et al., 2021; Thakur et al., 2021).
FIGURE 3. Schematic diagram of an acoustic fluid device for salivary exosome isolation. Micrometer and submicrometer waste can be separated by using 20 MHz and 40 MHz surface sound waves. During the separation process, the particles are subjected to the acoustic radiation force and the drag force. Exosomes can be separated in the second separation module. Reproduced with permission from (Wang et al., 2020).
In addition to acoustic fractionation, a FerroChip device combining ferrohydrodynamic and microfluidic systems has been proposed to isolate extracellular vesicles (Liu et al., 2020). Under the action of ferromagnetic fluid sheath flow, premixes of antimagnetic nanoparticles and extracellular vesicles with ferromagnetic fluid enter straight microchannels. Small particles are allowed to migrate at a slower rate by difference of the particle size, while extracellular vesicles migrating at a faster rate, resulting in spatial isolation at the channel exit. The microfluidic chip is no labeling, and extracellular vesicles will be captured by a continuous flow and size-dependent manner. It has been reported to achieve 94.3% recovery and 87.9% isolation purity.
The separation principle of non-contact microfluidics is that the fluid containing the electric vehicle encounters a sheath flow along the wall of the microchannel in a microfluidic system based on viscoelastic media flow (Rodriguez-Quijada and Dahl, 2021). After applying the elastic lifting force generated by the viscoelasticity of fluids, exosomes and other extracellular components are driven towards the centerline of the microchannel according to size. Larger particles eventually reach the centerline, thus achieving the isolation of exosomes. Besides, researchers have pointed out that extracellular vesicles can be rapidly enriched and detected by phospholipids and transmembrane proteins in microfluidic chips (Ren et al., 2022). Li et al. (2022) proposed a 3D-SiO2 porous chip, which applied to prostate cancer (PCa) staging in mice and early detection of clinical PCa patients. Results show that early detection rate of clinical patients can be improved by non-contact microfluidics. Combining nanoscale porous properties with exosome-specific markers, the sensitivity of biosensing can be improved by the 3D-SiO2 porous chip greatly.
Chen et al. (2021) proposed an ultra-fast exosome isolation system (EXODUS) for the efficient isolation and detection of exosomes. The automatic purification of exosomes from different biofluids is allowed by EXODUS. The key part is the isolation of exosomes by membrane vibrations generated by negative pressure oscillations and dual coupled resonators. Dual frequency transverse waves on the membrane are generated. Nanoporous membranes and cartridge oscillations are used to suppress membrane fouling effects to achieve clog-free and ultra-fast purification of exosomes. There is a dual-filter cuvette with one left and right outlet in the system, both of which are connected to nanoporous anodic aluminum oxide membranes (Patel et al., 2020). By switching the direction of negative pressure and air pressure, periodic negative pressure oscillations are generated across anodic aluminum oxide membranes. Periodic switching of negative pressure is used to allow the passage of small particles and fluids while exosomes are retained in the central chamber. With high-frequency harmonic oscillations generated by the nanopore disk resonator and low-frequency harmonic oscillations used throughout the device, dual-frequency transverse waves and acoustic fluid resuspend surface particles, effectively suppressing particle aggregation and scaling effects (Figure 4). They took 113 clinical urine samples for the functional validation of EXODUS.
FIGURE 4. The mechanism of NPO and the schematic diagram of the EXODUS device. Exosomes can be isolated ultra-rapidly using negative pressure oscillations and double-coupled harmonic oscillator membrane vibrations. Reproduced with permission from (Chen et al., 2021).
Compared to the conventional isolation methods such as precipitation and ultracentrifugation, EXODUS has advantages in terms of isolation speed, yield, and purity. Moreover, the processing time of samples is short, narrowing down to 10 min for a 10 mL of urine. The purity of exosomes was verified by the uromodulin (Droste et al., 2021). In comparison with precipitation and membrane affinity, the band intensity of uromodulin was lower, indicating the good purification performance of EXODUS. Purification of 15 mL cell culture medium with EXODUS resulted in removal of approximately 99% of protein impurities and recovery of approximately 90% of exosomes. iTEARS can rapidly isolate exosomes from small volumes of tears (∼10 µL) by using EXODUS (Hu et al., 2022). This system has important implications for the establishment of the precision medicine based on tear exosomes.
The centrifugal microfluidic disc system (Exo-CMDS) is proposed by Zhao et al. (2022) for the isolation of exosomes. The automated centrifugal microfluidic disc system is combined with a functionalized membrane to form Exo-CMDS. The centrifugal microfluidic disc contains two functional membranes, one of which is used to filter blood cells and larger particles, and the other is used to enrich exosomes. The membrane is a quaternized regenerated cellulose membrane with a positive charge that adsorbs the negative charge from the exosome surface. In this system, exosomes can be adsorbed onto the membrane by the loading buffer (ExoL), and exosomes on the membrane can be eluted by the separation buffer (ExoI). The advantages of Exo-CMDS over conventional isolation methods are higher isolation yield and purity. In addition, Exo-CMDS has higher sensitivity, higher specificity, and low cost. It took only 8 min to process blood samples with a volume of less than 300 μL. At the same time, the novel aptamer fluorescence system (Exo-AFS) can be incorporated with Exo-CMDS to detect exosomal surface proteins, which is useful for diagnosing cancer. Furthermore, the quantification of specific subpopulations is allowed by the droplet-based extracellular vesicle analysis (DEVA) (Yang et al., 2022a). In this platform, droplet generation, processing, and analysis can be performed simultaneously, enabling high-throughput digital determination of extracellular vesicles.
Hao et al. (2020) presented a multifunctional 3D nanostructured array by using the acoustofluidic technology. The acoustic sensor was integrated with an acoustofluidic reactor to build a 3D ZnO nanoarray within a capillary microchannel, which can capture nanoscale biomolecules. The ZnO nanoarray has a wide elastic range in length, density, and diameter. At the same time, Ag nanoparticles were deposited onto the ZnO nanoarrays to obtain ZnO-Ag capillary elements for the detection of exosomes, DNA, oligonucleotides. The functional validation of ZnO-Ag capillaries was performed by the finite-difference time-domain (FDTD) simulations. The ideas of the development of microanalytical devices can be provided by the enhanced optical sensing of ZnO-Ag. Zhang et al. (2019) developed an integrated platform for the analysis of exosomes (ExoProfile chip). The 3D porous serpentine nanostructures were innovatively constructed, and multiplexed detection of exosomes was allowed by this chip. In addition, it has shown that the dual-selective fluorescent nanosensor (Feng et al., 2022), 3D nanomachine based on Janus wireframe DNA cube (Xu et al., 2022), and 3D molecular beacon based on cubic DNA nanospaces (Mao et al., 2022) can be used for the characterization and analysis of exosomes (Guo et al., 2021b).
The immunological methods for exosome isolation are mainly to capture the characteristic proteins on the surface of exosomes (such as CD63, CD9, and heat shock proteins) by the modified antibodies or aptamers. The biotinylated antibodies or aptamers and immunomagnetic beads can be used for exosome isolation. The functionalized magnetic beads have been widely used for the exosome isolation by combining microfluidic devices with immunological methods. Meanwhile, the integration of the exosome isolation and detection can be realized by the combination of methods. For example, Zhang et al. (2022b) performed label-free detection of PD-L1 in exosomes based on the surface plasmon resonance biochip (SPR-ExoPD-L1) to provide effective information for the diagnosis and treatment of cancer. Either a fluorescent biosensor (Chen et al., 2022) or a ROX-labeled aptamer (ROX-Apt) assembled on the surface of MoS2-AuNSs specifically bound to CD63 can be used to detect exosomes (Pan et al., 2022).
Lipid microarray is a novel strategy for exosome capture. Microarrays can carry the specific antibodies that can recognize the characteristic proteins on the surface of exosomes. In combination with dip-pen nanolithography, highly selective capture of extracellular vesicles (EVs) is allowed. Liu et al. (2021) used lipid dip-pen nanolithography (L-DPN) to form the micron or nanoscale patterns of lipid patches on various substrates. Lipid membranes of DOPC (Fox et al., 2021) with a 5 mol% admixing of the biotinylated phospholipid cap biotinyl are obtained via L-DPN, which is then incubated with streptavidin solution to provide the binding sites for biotinylated antibodies. The target EVs can be captured as EVs pass through the array. EV cargos are eventually captured in the membrane patch through the membrane fusion (Figure 5). Highly sensitive recovery with only a small volume of liquid can be achieved by lipid microarrays. At the same time, the microarray has inherent anti-fouling properties, providing a basis for downstream genomic and proteomic analysis. To visualize EVs, EVs can be clearly observed under the fluorescence microscopy by using the lipophilic dye staining (e.g., PKH26). The lipid microarray platform can effectively capture EVs from MCF7 cell conditioned medium verified by various controls/experiments. Besides, functional EVs can be screened by the anion-exchange method (Seo et al., 2022). However, this microarray is suitable for large scale isolation of biologically active exosomes.
FIGURE 5. Scheme of extracellular vesicles capture by supporting lipid membranes (SLM). (A) Fabrication of biotinylated SLM arrays can be achieved by L-DPN. (B) Biotinylated SLM arrays are encapsulated with streptavidin. (C) Biotinylated antibodies can bind to the SLM arrays to capture specific EVs. (D) Cancer-associated EVs are captured on the SLM arrays. (E) Fusion of EV with SLM. (F) Trapping of EV biomaterials (such as RNA, proteins). Reproduced with permission from (Liu et al., 2021).
Liquid biopsy chips are gradually becoming the mainstream for the integration of the isolation and identification of exosomes. Bathini S et al. proposed a magnetic particle-based chip to isolate EVs by using the synthetic peptide Vn96 (Bathini et al., 2021). Vn96 can bind to the heat shock protein (HSP) expressed on the surface of EVs (Taylor et al., 2020; Roy et al., 2021). A 3D mixer and a sedimentation unit are included in the chip. The streptavidin-coated magnetic particles were co-incubated with Biotin-PEG-Vn96 in a centrifuge tube for 30 min to become the Vn96-bound magnetic particles, followed by collected into syringes. Equal amounts of Vn96-bound magnetic particles were injected into the liquid biopsy chip simultaneously with MCF7 CCM at the same flow rate. The particles fully bound within the chamber start settling within 0.5 mm from the beginning of the chamber. The precipitate is finally collected (Figure 6). Then EVs are eluted and characterized, combined with the droplet digital PCR for quantification of the housekeeping gene (Pan et al., 2020). The bioactivity and morphological structure of EVs can be maintained by the chip. Similar to lipid microarray, it can be used for early diagnosis of diseases. In addition, Park et al. (2021) proposed a high-throughput integrated magneto-electrochemical device (HiMEX) which is a 96-well assay that can be used for clinical high-throughput EVs analysis. This assay was able to enrich EVs by antibody-coated magnetic beads and quantitate the proteins bound to EVs by electrochemical detection after enzyme amplification.
FIGURE 6. Scheme of the EV-isolation from MCF7 CCM. Vn96-bound magnetic particles are injected into the liquid biopsy chip simultaneously with MCF7 CCM at the same flow rate for EVs isolation. EVs are captured by the magnetic particles in the sedimentation unit. Reproduced with permission from (Bathini et al., 2021).
The highly integrated Exosome Separation and Detection (ExoSD) chip proposed by Yu et al. (2021) is used to extract exosomes from cell culture supernatants in a continuous flow manner, followed by the detection of exosomes by immunofluorescence. ExoSD chip contains a separation zone and a detection zone. Exosomes in the cell culture supernatant were captured by immunomagnetic nanoparticles (IMNPs). The mixture was injected into the ExoSD chip. The Exosomes@IMNPs will be separated in the separation zone from the mixture. The separated Exosomes@IMNPs were flowed into the detection zone and were captured on the Ni cylinder array. The nickel (Ni) comb-like structure was used to enhance the magnetic force on IMNPs. Finally, the cancer cell-derived exosomes will be labeled with fluorescent antibodies (Figure 7). ExoSD chip has gradually become a multifunctional platform for exosome isolation and detection. The recovery of exosomes was more than 80%, and the purity was more than 83% at the injection frequency of 4.8 mL/h. Exosomes can be detected by the plasma-coupled electrochemiluminescence immunosensors (Xiong et al., 2022).
FIGURE 7. ExoSD chip for exosome separation by IMNPs. The separated exosomes@IMNPs were captured on the Ni cylinder array and labeled with fluorescent antibodies for detection. (A) Scheme of the Ni comb-like structure for local magnetic field and magnetic field gradient enhancement. (B) SEM image of the microfilter. (C) Schematic diagram of capturing exosomes@IMNPs by Ni cylindrical array. (D) Three-dimensional diagram of the ExoSD chip. Reproduced with permission from (Yu et al., 2021).
Cheng et al. (2022) proposed a system for the isolation and release of exosomes, called immunomagnetic hedgehog particles (IMHPs). TiO2 bundles were assembled into hedgehog-type TiO2 particles (TiO2HPs) with specific spikes by using a hydrothermal method. Then magnetic responsive nanoparticles Fe3O4 with redox responsive disulfide bonds and CD63 antibodies were immobilized on the TiO2HPs to obtain TiO2@Fe3O4-anti-CD63HPs (IMHPs). The capture efficiency of exosomes isolated from MCF-7 cells by IMHPs was up to 91.70%. Exosomes were released by reducing the disulfide bonds on IMHPs by Tris(2-carboxyethyl)phosphine (TCEP) with the release efficiency of up to 82.45%. In addition to high isolation efficiency, the bioactivity and structural integrity of exosomes can be maintained by IMHPs compared with traditional isolation methods. To reduce the pretreatment steps for the exosome isolation, Boriachek K et al. used CD63 antibodies functionalized gold-loaded iron oxide nanocubes (Au-NPFe2O3NC) to directly isolate exosomes (Boriachek et al., 2019).
3D Paper-Based sEV isolation system (sEV-IsoPD) is used for the rapid isolation, and the advantages of high yield and purity can be provided (Zhang et al., 2022c). sEV-IsoPD consists of a peristaltic pump, a filter holder, and four cell containers. The filter holder is composed of a polycarbonate porous membrane for size exclusion and metal-organic framework (MOF)/CD63 antibody-modified paper (Ab@MOF@paper). EVs can be isolated from serum and cell culture medium by Ab@MOF@paper. After being captured by Ab@MOF@paper, sEVs can be released by catabolizing glutathione from ZIF-90 (Figure 8). Compared to ultracentrifugation and filtration, higher yield exosomes can be recovered in a short time. In addition to this, microfluidic chips consist of polydimethylsiloxane and glass to specifically capture EVs by using CD63 (Song et al., 2020) and PTK7 aptamers in the microchannel. CLIKKPF (the higher affinity peptide) was immobilized on SiO2 microspheres to capture exosomes by the high affinity between phosphatidylserine and CLIKKPF (Yang et al., 2022b). Kim et al. (2022b) pointed out that EVs can be captured by adsorption of antibodies (e.g., CD63 antibody) onto a citrate-covered plasma gold surface by physical adsorption, which showed higher efficiency and specificity.
FIGURE 8. Schematic of the sEV-IsoPD. (A) The device consists of a peristaltic pump, four-cell container, valves, and filter holder. (B) Schematic diagram of the flow system of sEV-IsoPD. (C) Scheme of the PC membrane and the fabrication of Ab@MOF@paper. (D) The process of capturing, releasing, and detecting sEVs by sEV-IsoPD. Reproduced with permission from (Seo et al., 2022).
Zheng et al. (2022) designed a microfluidic chip with a teardrop-shaped micropillar array. Exosomes were captured by Tim4-modified magnetic beads (Tim4 beads). The teardrop-shaped microcolumn arrays play an important role in the trapping process. The capture of exosomes by Tim4 beads is dependent on Ca2+. The intact exosomes can be released by using the chelators. Therefore, the bioactivity and morphological integrity of exosomes can be maintained with high efficiency and sensitivity. Moreover, the DNAzyme-triggered system was proposed to aggregate individual sEVs into clusters (Yu et al., 2022a). In this system, DNAzyme probes with cholesterol tails was hybridized to the CD63 aptamer-modified substrate probes to enrich exosomes. Exosomes can be rapidly isolated without ultracentrifugation. The reproducible temperature-sensitive electrochemical biosensor was constructed to detect exosomes (Liu et al., 2022a).
The specific purification of Ewing sarcoma (ES)-derived EVs can be achieved by using the ES-EV Click Chip (Dong et al., 2020). Si nanowires are embedded in the microchip, and then combined with covalent chemistry-mediated capture of EVs. The chip consists of a Tz-grafted SiNWS and a PDMS-based chaotic mixer with a serpentine microchannel. Tz was immobilized on Si nanowires by the reaction of the Tz-sulfo-NHS ester with the NHS ester on the SiNWS, yielding the Tz-grafted SiNWS. The chaotic mixer is combined with Tz-grafted SiNWS by microfluidic scaffolding to make a complete ES-EV Click chip. LINGO-1 is a specific marker of ES which was found to be expressed on the surface of ES EVs by immunogold-TEM. ES EVs can be specifically recognized and conjugated by anti-LINGO-1. The primary amine group on anti-LINGO-1 was reacted with TCO-PEG4-NHS ester to form TCO-anti-LINGO-1 conjugate. The inverse electron demand Diels–Alder (IEDDA) reaction between the Tz and TCO moieties was used to capture EVs on the Tz-grafted SiNWS. EVs were released via the disulfide bond cleavage agents (such as 1,4-dithiothreitol, DTT). Specific purification of ES-derived EVs is allowed by EVs ES-EV Click Chip, while the integrity of EVs is maintained. The chip is suitable for the study of downstream functions of ES EVs.
Liu X et al. proposed a stimulus-mediated exosome enrichment and purification system for efficient extraction of exosomes from plasma samples (Liu et al., 2022b). Targeted enrichment and purification of exosomes can be achieved by mounting specific stimulus corresponding copolymers on lipid bilayers of exosomes. N-isopropylacrylamide (NIPAM) and N-acryloyloxysuccinimide (NAS) were polymerized via reversible addition-fragmentation chain transfer (RAFT) to form PNN. PNN-SA was formed by PNN and streptavidin (SA) via a carbodiimide cross-linker (Figure 9). DSPE-SS-Biotin inserted into the lipid bilayer of exosomes, with the biotin tail exposed. PNN-covered exosomes (PNN-Exos) was produced by the interaction between the anchored biotin tag and PNN-SA. The lower critical solution temperature (LCST) is 31°C. PNN dissolves in aqueous solution at room temperature 25°C (T < LCST). With the increasing temperature (T > LCST), PNN undergoes reversible hydrophilic-hydrophobic collapse, leading to phase separation and precipitation, and the solution changes from clear to turbid. Compared with traditional isolation methods (e.g., ultracentrifugation, ExoQuick), the bioactivity of exosomes can be effectively maintained by this system with higher yield and purity. In addition, the system can be combined with specific analytical tools to characterize relevant exosome biomarkers such as microRNAs. However, this system cannot be applied to larger EVs, and the validation in larger clinical cohorts for prospective studies is required.
FIGURE 9. The molecular structures of PNN-SA and DSPE-SS-Biotin. DSPE-SS-Biotin can be inserted into the lipid bilayer of exosomes, and bare biotin interacts with PNN-SA to capture exosomes. The process is temperature-dependent. Reproduced with permission from (Zhang et al., 2022c).
In order to achieve rapid capture of EVs, Sun et al. (2022) proposed the concept of Click Beads. TCO-PEG-NHS was co-incubated with DSPE-PEG1000-NH2 to form DSPE-PEG1000-TCO conjugates. EVs were labeled by inserting the lipid motif of DSPE-PEG1000-TCO into the EVs membrane. TCO-labeled EVs were captured on Click Beads by a bioorthogonal click chemistry reaction between TCO and Tz motifs. Isolated exosomes on Click Beads were collected in tubes by the centrifugation. Compared to traditional isolation methods, Click Beads are time-saving and efficient, allowing for the capture of multiple cancer-derived exosomes and downstream analysis by RT-dPCR. Moreover, further analysis of exosomes can be attempted in combination with the one-step thermophoretic AND gate operation (Tango) assay (Deng et al., 2022).
It is difficult to efficiently isolate exosomes from biofluids, in order to solve the problem, a new microvortex chip has been constructed. EVs can be efficiently separated by integrating lipid nanoprobe modified Morpho Menelaus (M. Menelaus) wings into microfluidic chips (Han et al., 2020). DSPE-PEG-biotin lipid nanoprobe solution was co-incubated with affin-coated M. Menelaus wings, and biotin-avidin reaction was used to obtain lipid nanoprobe modified M. Menelaus wings. The lipid tail can be inserted into the EVs membrane for the exosome capture. Also, the downstream analysis is allowed by the chip (Figure 10). GPC-1 mRNA from breast cancer cell-derived EVs is used for the functional validation. This novel microvortex chip was reported to achieve the high throughput enrichment of EVs with an efficiency of up to 70%. The natural M. Menelaus wing has a series of intersecting point-connected 3D microgroove structures arranged in parallel on the surface, generating microvortexes for EVs isolation.
FIGURE 10. Modification of M. Menelaus wing by lipid nanoprobes and SEM images of M. Menelaus wings at different stages of the process. (A) Schematic diagram of the modification process of lipid nanoprobes on the surface of M. Menelaus wing. (B, C) SEM image of wing structure. (D) TEM image of the EVs. (E) SEM image of EVs captured by M. Menelaus wing without lipid nanoprobes modification. (F) SEM image of EVs captured by lipid nanoprobes modified M. Menelaus wing (as shown by the white arrow). (G) SEM image of EVs captured by M. Menelaus wing after treatment with triethylamine. Reproduced with permission from (Han et al., 2020).
Tulkens et al. (2020) combined ultrafiltration, size-exclusion chromatography, and density gradient centrifugation in sequence to isolate bacterial-derived EVs, ensuring the integrity of EVs and eliminating the need for labeling and facilitating the subsequent characterization of isolated EVs. In addition, exosomes can also be isolated by CD9-HPLC-IAC (Zhu et al., 2021) and untouched isolation (Yu et al., 2022b). Recombinant EVs are gradually developed for disease diagnosis and treatment (Geeurickx et al., 2019). The process of exosome uptake and content transport is becoming clear (Bonsergent et al., 2021). To solve the problem of natural exosomes being cleared from blood circulation in three hours, Lathwal et al. (2021) designed exosome-polymer hybrid to make exosomes more stable in vitro. Extracellular vesicle mimetics prepared by top-down or bottom-up strategies have great potential for clinical applications (Liu et al., 2022c).These approaches shown above all focused attempts to better isolate exosomes.
Exosomes have attracted extensive attention over the past few decades due to their potential clinical applications. Nowadays, many methods and technologies have been developed for the separation of exosomes. In this section, we discuss the challenges and prospects of current exosome separation methods. Due to the heterogeneity of exosomes and the complexity of the matrix, exosome separation at high purity, yield, and recovery remains a challenge. The current methods for separating exosomes are mainly dependent on their size and surface-specific proteins. Size-based methods are label-free, but they suffer from low purity. Immunoaffinity methods can isolate exosomes within a short time, but they depend on the specificity of the markers on the surface of exosomes. However, the commonly used markers are not entirely specific to exosomes. The selection of isolation method is very important for the follow-up research, for example, related studies on exosome genomics and proteomics, as well as the role of exosomes in the treatment of diseases.
The low purity of exosome separation is mainly caused by the large number of co-isolated contaminants. In particular, co-isolated non-exosomal functional vesicles will affect the subsequent experiments. There are also a large number of co-isolated proteins that overlap characteristics with exosomes in terms of density and size. Therefore, it is difficult to isolate exosomes from biological fluids without contaminations, which may affect the purity of the isolated exosomes.
In order to improve the purity of isolation, the first problem to be solved is to identify the specific differences in size, density, surface protein, and other physicochemical properties between exosomes and other non-vesicle components. For example, immunoaffinity capture can obtain higher purity by selecting appropriate antibodies or aptamers to bind exosomes according to the surface-specific markers on exosomes. Currently, combinations of multiple methods are being applied for the isolation and recovery of high-purity exosomes, such as ultracentrifugation combined with size-exclusion chromatography. The comprehensive method has the excellent performance to improve the label-free recovery of exosomes with high purity. However, there are few studies on the isolation of exosomes by comprehensive methods. A lot of work needs to be done to promote the development of this method.
Due to changes in external forces or microenvironment, some existing separation methods inevitably destroy the bioactivity and morphological integrity of exosomes. For example, high rotating external force and extrusion of filtration membrane will cause mechanical damage to exosomes. The bioactivity and structural integrity of exosomes can be effectively maintained by avoiding external damage reasonably, such as the use of size-exclusion chromatography which depends on natural gravity-based isolation. In addition, the bioactivity and integrity of exosomes may be affected by the physicochemical properties in different biological fluids or by substances that bind to exosomes specifically. Capture by covalent chemistry can also effectively preserve the bioactivity and integrity of exosomes, or by using easily isolated substances such as Ca2+-dependent Tim4 proteins. It is crucial to efficiently recover intact exosomes for the disease treatment and biology studies of exosomes.
The microfluidic technology is considered to be highly promising because of low consumption, automation, and portability. However, the technology is high cost and low throughput. For example, due to inherent label-free features, the deterministic lateral displacement, dielectrophoretic, etc., show great potential for the recovery of exosomes. However, these methods suffer from relatively low purity. It is essential to improve the throughput and separation velocity. Although many microfluidic methods have been developed for exosome isolation, none are widely used in clinical practice. One of the main reasons is that there are limited samples available for clinical validation in microfluidic platforms. Some microfluidic chips can achieve good separation performance in culture medium. However, due to the complexity of the sample, a large number of clinical samples are needed to verify its reliability, sensitivity and specificity. However, clinical validation with a large number of samples is not easy and need us to make more efforts. We believe that the microfluidic device suitable for clinical exosome separation will be developed.
Despite the remarkable application prospects, there are still some problems worth discussing. The heterogeneity of exosomes is not well understood, which may derivatively influence the selection of different separation methods. With so many metrics to consider, such as purity, recovery, yield, etc., it is hard to say which method is the best option. At present, choice of the most appropriate methods must be based on each specific research requirements and application scenarios. The comprehensive method is also recommended by using multiple separation methods to improve purity and yield. It should be emphasized that the separation method chosen must be based on factors that may differ from studies. Therefore, there is no uniform method for exosome separation.
Appropriate isolation method/means is the premise of exosome research. Overall, more and more methods used for the isolation of exosomes have made great progress in maintaining the bioactivity and morphological integrity of exosomes. So far, many isolation methods can be used not only to guarantee high isolation purity, but also to ensure high isolation yield, which have a great functional improvement. However, each method has its own merits and demerits. The microfluidic systems also have the disadvantage of low sample capacity. The combination of methods can be used to solve certain problems through complementary advantages, but there are still limitations. Therefore, it is challenging to develop an integrated isolation device with the advantages of simplicity, convenience, high yield, high purity, and low cost. The road to standardization, integration, and high throughput of isolation equipment is obstacle-packed and long. The development of comprehensive microarrays is a promising method for exosome isolation, while the study of exosome subgroups and heterogeneity will be expanded in depth. It is believed that in the near future, researchers will make efforts on cutting-edge exosome isolation method for clinical application.
ZS put forward the conception and design of the manuscript. LL provided guidance and revised the manuscript. JG wrote the first draft of the manuscript. AL summarized and analyzed the methods. JH contributed to the charting. LF revised the manuscript carefully.
This work was supported by the Fundamental Research Funds for the Central Universities (WK9110000057); the Key Programs for Research and Development of Anhui Province (No. 1704a0802153); and the Project of the Science and Technology Innovation of Anhui province (2017070802D146).
The authors declare that the research was conducted in the absence of any commercial or financial relationships that could be construed as a potential conflict of interest.
All claims expressed in this article are solely those of the authors and do not necessarily represent those of their affiliated organizations, or those of the publisher, the editors and the reviewers. Any product that may be evaluated in this article, or claim that may be made by its manufacturer, is not guaranteed or endorsed by the publisher.
Alameldin, S., Costina, V., Abdel-Baset, H. A., Nitschke, K., Nuhn, P., Neumaier, M., et al. (2021). Coupling size exclusion chromatography to ultracentrifugation improves detection of exosomal proteins from human plasma by LC-MS. Pract. Lab. Med. 26, e00241. doi:10.1016/j.plabm.2021.e00241
Ayala-Mar, S., Perez-Gonzalez, V. H., Mata-Gomez, M. A., Gallo-Villanueva, R. C., and Gonzalez-Valdez, J. (2019). Electrokinetically driven exosome separation and concentration using dielectrophoretic-enhanced PDMS-based microfluidics. Anal. Chem. 91, 14975–14982. doi:10.1021/acs.analchem.9b03448
Bathini, S., Pakkiriswami, S., Ouellette, R. J., Ghosh, A., and Packirisamy, M. (2021). Magnetic particle based liquid biopsy chip for isolation of extracellular vesicles and characterization by gene amplification. Biosens. Bioelectron. 194, 113585. doi:10.1016/j.bios.2021.113585
Berger, M., Scherer, C., Noskov, S., Bantz, C., Nickel, C., Schupp, W., et al. (2021). Influence of oscillating main flow on separation efficiency in asymmetrical flow field-flow fractionation. J. Chromatogr. A 1640, 461941. doi:10.1016/j.chroma.2021.461941
Bonsergent, E., Grisard, E., Buchrieser, J., Schwartz, O., Thery, C., and Lavieu, G. (2021). Quantitative characterization of extracellular vesicle uptake and content delivery within mammalian cells. Nat. Commun. 12, 1864. doi:10.1038/s41467-021-22126-y
Boriachek, K., Masud, M. K., Palma, C., Phan, H. P., Yamauchi, Y., Hossain, M. S. A., et al. (2019). Avoiding pre-isolation step in exosome analysis: Direct isolation and sensitive detection of exosomes using gold-loaded nanoporous ferric oxide nanozymes. Anal. Chem. 91, 3827–3834. doi:10.1021/acs.analchem.8b03619
Cardoso, R. M. S., Rodrigues, S. C., Gomes, C. F., Duarte, F. V., Romao, M., Leal, E. C., et al. (2021). Development of an optimized and scalable method for isolation of umbilical cord blood-derived small extracellular vesicles for future clinical use. Stem Cells Transl. Med. 10, 910–921. doi:10.1002/sctm.20-0376
Chen, W., Zhang, Y., Di, K., Liu, C., Xia, Y., Ding, S., et al. (2022). A washing-free and easy-to-operate fluorescent biosensor for highly efficient detection of breast cancer-derived exosomes. Front. Bioeng. Biotechnol. 10, 945858. doi:10.3389/fbioe.2022.945858
Chen, Y., Zhu, Q., Cheng, L., Wang, Y., Li, M., Yang, Q., et al. (2021). Exosome detection via the ultrafast-isolation system: Exodus. Nat. Methods 18, 212–218. doi:10.1038/s41592-020-01034-x
Cheng, J., Zhu, N., Zhang, Y., Yu, Y., Kang, K., Yi, Q., et al. (2022). Hedgehog-inspired immunomagnetic beads for high-efficient capture and release of exosomes. J. Mater Chem. B 10, 4059–4069. doi:10.1039/d2tb00226d
Chernyshev, V. S., Chuprov-Netochin, R. N., Tsydenzhapova, E., Svirshchevskaya, E. V., Poltavtseva, R. A., Merdalimova, A., et al. (2022). Asymmetric depth-filtration: A versatile and scalable method for high-yield isolation of extracellular vesicles with low contamination. J. Extracell. Vesicles 11, e12256. doi:10.1002/jev2.12256
Correll, V. L., Otto, J. J., Risi, C. M., Main, B. P., Boutros, P. C., Kislinger, T., et al. (2022). Optimization of small extracellular vesicle isolation from expressed prostatic secretions in urine for in-depth proteomic analysis. J. Extracell. Vesicles 11, e12184. doi:10.1002/jev2.12184
Crescitelli, R., Lasser, C., and Lotvall, J. (2021). Isolation and characterization of extracellular vesicle subpopulations from tissues. Nat. Protoc. 16, 1548–1580. doi:10.1038/s41596-020-00466-1
D'Acunzo, P., Kim, Y., Ungania, J. M., Perez-Gonzalez, R., Goulbourne, C. N., and Levy, E. (2022). Isolation of mitochondria-derived mitovesicles and subpopulations of microvesicles and exosomes from brain tissues. Nat. Protoc. 17, 2517–2549. doi:10.1038/s41596-022-00719-1
Deng, J., Zhao, S., Li, J., Cheng, Y., Liu, C., Liu, Z., et al. (2022). One-step thermophoretic and gate operation on extracellular vesicles improves diagnosis of prostate cancer. Angew. Chem. Int. Ed. Engl. 61, e202207037. doi:10.1002/anie.202207037
Dong, J., Zhang, R. Y., Sun, N., Hu, J., Smalley, M. D., Zhou, A., et al. (2020). Coupling nanostructured microchips with covalent chemistry enables purification of sarcoma-derived extracellular vesicles for downstream functional studies. Adv. Funct. Mater 30, 2003237. doi:10.1002/adfm.202003237
Droste, M., Tertel, T., Jeruschke, S., Dittrich, R., Kontopoulou, E., Walkenfort, B., et al. (2021). Single extracellular vesicle analysis performed by imaging flow cytometry and nanoparticle tracking analysis evaluate the accuracy of urinary extracellular vesicle preparation techniques differently. Int. J. Mol. Sci. 22, 12436. doi:10.3390/ijms222212436
Feng, D., Ren, M., Miao, Y., Liao, Z., Zhang, T., Chen, S., et al. (2022). Dual selective sensor for exosomes in serum using magnetic imprinted polymer isolation sandwiched with aptamer/graphene oxide based FRET fluorescent ignition. Biosens. Bioelectron. 207, 114112. doi:10.1016/j.bios.2022.114112
Fox, L. J., Slastanova, A., Taylor, N., Wlodek, M., Bikondoa, O., Richardson, R. M., et al. (2021). Interactions between PAMAM dendrimers and DOPC lipid multilayers: Membrane thinning and structural disorder. Biochim. Biophys. Acta Gen. Subj. 1865, 129542. doi:10.1016/j.bbagen.2020.129542
Geeurickx, E., Tulkens, J., Dhondt, B., Van Deun, J., Lippens, L., Vergauwen, G., et al. (2019). The generation and use of recombinant extracellular vesicles as biological reference material. Nat. Commun. 10, 3288. doi:10.1038/s41467-019-11182-0
Geng, T., Song, Z. Y., Xing, J. X., Wang, B. X., Dai, S. P., and Xu, Z. S. (2020). <p>Exosome derived from coronary serum of patients with myocardial infarction promotes angiogenesis through the miRNA-143/IGF-IR pathway</p>. Int. J. Nanomedicine 15, 2647–2658. doi:10.2147/IJN.S242908
Guan, S., Yu, H., Yan, G., Gao, M., Sun, W., and Zhang, X. (2020). Characterization of urinary exosomes purified with size exclusion chromatography and ultracentrifugation. J. Proteome Res. 19, 2217–2225. doi:10.1021/acs.jproteome.9b00693
Guo, J., Wu, C., Lin, X., Zhou, J., Zhang, J., Zheng, W., et al. (2021). Establishment of a simplified dichotomic size-exclusion chromatography for isolating extracellular vesicles toward clinical applications. J. Extracell. Vesicles 10, e12145. doi:10.1002/jev2.12145
Guo, K., Li, Z., Win, A., Coreas, R., Adkins, G. B., Cui, X., et al. (2021). Calibration-free analysis of surface proteins on single extracellular vesicles enabled by DNA nanostructure. Biosens. Bioelectron. 192, 113502. doi:10.1016/j.bios.2021.113502
Gupta, S., Rawat, S., Arora, V., Kottarath, S. K., Dinda, A. K., Vaishnav, P. K., et al. (2018). An improvised one-step sucrose cushion ultracentrifugation method for exosome isolation from culture supernatants of mesenchymal stem cells. Stem Cell. Res. Ther. 9, 180. doi:10.1186/s13287-018-0923-0
Han, C., Kang, H., Yi, J., Kang, M., Lee, H., Kwon, Y., et al. (2021). Single-vesicle imaging and co-localization analysis for tetraspanin profiling of individual extracellular vesicles. J. Extracell. Vesicles 10, e12047. doi:10.1002/jev2.12047
Han, S., Xu, Y., Sun, J., Liu, Y., Zhao, Y., Tao, W., et al. (2020). Isolation and analysis of extracellular vesicles in a Morpho butterfly wing-integrated microvortex biochip. Biosens. Bioelectron. 154, 112073. doi:10.1016/j.bios.2020.112073
Han, Z., Peng, C., Yi, J., Zhang, D., Xiang, X., Peng, X., et al. (2021). Highly efficient exosome purification from human plasma by tangential flow filtration based microfluidic chip. Sensors Actuators B Chem. 333, 129563. doi:10.1016/j.snb.2021.129563
Hao, N., Liu, P., Bachman, H., Pei, Z., Zhang, P., Rufo, J., et al. (2020). Acoustofluidics-assisted engineering of multifunctional three-dimensional zinc oxide nanoarrays. ACS Nano 14, 6150–6163. doi:10.1021/acsnano.0c02145
Hassanpour Tamrin, S., Sanati Nezhad, A., and Sen, A. (2021). Label-free isolation of exosomes using microfluidic technologies. ACS Nano 15, 17047–17079. doi:10.1021/acsnano.1c03469
Ho, B. D., Beech, J. P., and Tegenfeldt, J. O. (2020). Cell sorting using electrokinetic deterministic lateral displacement. Micromachines (Basel) 12, 30. doi:10.3390/mi12010030
Hochstetter, A., Vernekar, R., Austin, R. H., Becker, H., Beech, J. P., Fedosov, D. A., et al. (2020). Deterministic lateral displacement: Challenges and perspectives. ACS Nano 14, 10784–10795. doi:10.1021/acsnano.0c05186
Hu, L., Zhang, T., Ma, H., Pan, Y., Wang, S., Liu, X., et al. (2022). Discovering the secret of diseases by incorporated tear exosomes analysis via rapid-isolation system: iTEARS. ACS Nano 16, 11720–11732. doi:10.1021/acsnano.2c02531
Kamei, N., Nishimura, H., Matsumoto, A., Asano, R., Muranaka, K., Fujita, M., et al. (2021). Comparative study of commercial protocols for high recovery of high-purity mesenchymal stem cell-derived extracellular vesicle isolation and their efficient labeling with fluorescent dyes. Nanomedicine 35, 102396. doi:10.1016/j.nano.2021.102396
Kang, Y. T., Hadlock, T., Jolly, S., and Nagrath, S. (2020). Extracellular vesicles on demand (EVOD) chip for screening and quantification of cancer-associated extracellular vesicles. Biosens. Bioelectron. 168, 112535. doi:10.1016/j.bios.2020.112535
Kang, Y. T., Niu, Z., Hadlock, T., Purcell, E., Lo, T. W., Zeinali, M., et al. (2021). On-chip biogenesis of circulating NK cell-derived exosomes in non-small cell lung cancer exhibits antitumoral activity. Adv. Sci. (Weinh) 8, 2003747. doi:10.1002/advs.202003747
Kim, K., Park, J., Jung, J. H., Lee, R., Park, J. H., Yuk, J. M., et al. (2021). Cyclic tangential flow filtration system for isolation of extracellular vesicles. Apl. Bioeng. 5, 016103. doi:10.1063/5.0037768
Kim, K., Son, T., Hong, J. S., Kwak, T. J., Jeong, M. H., Weissleder, R., et al. (2022). Physisorption of affinity ligands facilitates extracellular vesicle detection with low non-specific binding to plasmonic gold substrates. ACS Appl. Mater Interfaces 14, 26548–26556. doi:10.1021/acsami.2c07317
Kim, Y. B., Lee, G. B., and Moon, M. H. (2022). Size separation of exosomes and microvesicles using flow field-flow fractionation/multiangle light scattering and lipidomic comparison. Anal. Chem. 94, 8958–8965. doi:10.1021/acs.analchem.2c00806
Kumar, A., Dhadi, S. R., Mai, N. N., Taylor, C., Roy, J. W., Barnett, D. A., et al. (2021). The polysaccharide chitosan facilitates the isolation of small extracellular vesicles from multiple biofluids. J. Extracell. Vesicles 10, e12138. doi:10.1002/jev2.12138
Langevin, S. M., Kuhnell, D., Orr-Asman, M. A., Biesiada, J., Zhang, X., Medvedovic, M., et al. (2019). Balancing yield, purity and practicality: A modified differential ultracentrifugation protocol for efficient isolation of small extracellular vesicles from human serum. RNA Biol. 16, 5–12. doi:10.1080/15476286.2018.1564465
Lathwal, S., Yerneni, S. S., Boye, S., Muza, U. L., Takahashi, S., Sugimoto, N., et al. (2021). Engineering exosome polymer hybrids by atom transfer radical polymerization. Proc. Natl. Acad. Sci. U. S. A. 118, e2020241118. doi:10.1073/pnas.2020241118
Leong, S. Y., Ong, H. B., Tay, H. M., Kong, F., Upadya, M., Gong, L., et al. (2022). Microfluidic size exclusion chromatography (μSEC) for extracellular vesicles and plasma protein separation. Small 18, e2104470. doi:10.1002/smll.202104470
Li, K., Wong, D. K., Hong, K. Y., and Raffai, R. L. (2018). Cushioned-density gradient ultracentrifugation (C-dguc): A refined and high performance method for the isolation, characterization, and use of exosomes. Methods Mol. Biol. 1740, 69–83. doi:10.1007/978-1-4939-7652-2_7
Li, L., Zhao, J., Zhang, Q., Tao, Y., Shen, C., Li, R., et al. (2021). Cancer cell-derived exosomes promote HCC tumorigenesis through hedgehog pathway. Front. Oncol. 11, 756205. doi:10.3389/fonc.2021.756205
Li, Q., Wang, Y., Xue, Y., Qiao, L., Yu, G., Liu, Y., et al. (2022). Ultrasensitive analysis of exosomes using a 3D self-assembled nanostructured SiO2 microfluidic chip. ACS Appl. Mater Interfaces 14, 14693–14702. doi:10.1021/acsami.1c22569
Lim, J., Kang, B., Son, H. Y., Mun, B., Huh, Y. M., Rho, H. W., et al. (2022). Microfluidic device for one-step detection of breast cancer-derived exosomal mRNA in blood using signal-amplifiable 3D nanostructure. Biosens. Bioelectron. 197, 113753. doi:10.1016/j.bios.2021.113753
Liu, C., Wang, Y., Li, L., He, D., Chi, J., Li, Q., et al. (2022). Engineered extracellular vesicles and their mimetics for cancer immunotherapy. J. Control Release 349, 679–698. doi:10.1016/j.jconrel.2022.05.062
Liu, D., Tang, J., Xu, H., Yuan, K., Aryee, A. A., Zhang, C., et al. (2022). Split-aptamer mediated regenerable temperature-sensitive electrochemical biosensor for the detection of tumour exosomes. Anal. Chim. Acta 1219, 340027. doi:10.1016/j.aca.2022.340027
Liu, H. Y., Kumar, R., Zhong, C., Gorji, S., Paniushkina, L., Masood, R., et al. (2021). Rapid capture of cancer extracellular vesicles by lipid patch microarrays. Adv. Mater 33, e2008493. doi:10.1002/adma.202008493
Liu, X., Zong, Z., Liu, X., Li, Q., Li, A., Xu, C., et al. (2022). Stimuli-mediated specific isolation of exosomes from blood plasma for high-throughput profiling of cancer biomarkers. Small Methods 6, e2101234. doi:10.1002/smtd.202101234
Liu, Y., Zhao, W., Cheng, R., Logun, M., Zayas-Viera, M. D. M., Karumbaiah, L., et al. (2020). Label-free ferrohydrodynamic separation of exosome-like nanoparticles. Lab. Chip 20, 3187–3201. doi:10.1039/d0lc00609b
Lu, Y., Ye, L., Jian, X., Yang, D., Zhang, H., Tong, Z., et al. (2022). Integrated microfluidic system for isolating exosome and analyzing protein marker PD-L1. Biosens. Bioelectron. 204, 113879. doi:10.1016/j.bios.2021.113879
Luo, Y., Gao, D., Wang, P., Lou, C., Li, T., Niu, W., et al. (2021). Optimized culture methods for isolating small extracellular vesicles derived from human induced pluripotent stem cells. J. Extracell. Vesicles 10, e12065. doi:10.1002/jev2.12065
Manning, R. R., Holcomb, R. E., Katayama, D. S., Payne, R. W., Stillahn, J. M., Henry, C. S., et al. (2021). Analysis of peptides using asymmetrical flow field-flow fractionation (AF4). J. Pharm. Sci. 110, 3969–3972. doi:10.1016/j.xphs.2021.09.036
Mao, D., Zheng, M., Li, W., Xu, Y., Wang, C., Qian, Q., et al. (2022). Cubic DNA nanocage-based three-dimensional molecular beacon for accurate detection of exosomal miRNAs in confined spaces. Biosens. Bioelectron. 204, 114077. doi:10.1016/j.bios.2022.114077
Marioli, M., Kavurt, U. B., Stamatialis, D., and Kok, W. T. (2019). Application of microstructured membranes for increasing retention, selectivity and resolution in asymmetrical flow field-flow fractionation. J. Chromatogr. A 1605, 360347. doi:10.1016/j.chroma.2019.07.001
Mondal, S. K., and Whiteside, T. L. (2021). Immunoaffinity-based isolation of melanoma cell-derived and T cell-derived exosomes from plasma of melanoma patients. Methods Mol. Biol. 2265, 305–321. doi:10.1007/978-1-0716-1205-7_23
Neves, K. B., Rios, F. J., Sevilla-Montero, J., Montezano, A. C., and Touyz, R. M. (2022). Exosomes and the cardiovascular system: Role in cardiovascular health and disease. J. Physiol. doi:10.1113/JP282054
Pan, H., Dong, Y., Gong, L., Zhai, J., Song, C., Ge, Z., et al. (2022). Sensing gastric cancer exosomes with MoS2-based SERS aptasensor. Biosens. Bioelectron. 215, 114553. doi:10.1016/j.bios.2022.114553
Pan, Y., Ma, T., Meng, Q., Mao, Y., Chu, K., Men, Y., et al. (2020). Droplet digital PCR enabled by microfluidic impact printing for absolute gene quantification. Talanta 211, 120680. doi:10.1016/j.talanta.2019.120680
Park, J., Park, J. S., Huang, C. H., Jo, A., Cook, K., Wang, R., et al. (2021). An integrated magneto-electrochemical device for the rapid profiling of tumour extracellular vesicles from blood plasma. Nat. Biomed. Eng. 5, 678–689. doi:10.1038/s41551-021-00752-7
Patel, Y., Janusas, G., Palevicius, A., and Vilkauskas, A. (2020). Development of nanoporous AAO membrane for nano filtration using the acoustophoresis method. Sensors (Basel) 20, 3833. doi:10.3390/s20143833
Qian, J., Zhang, Q., Liu, M., Wang, Y., and Lu, M. (2022). A portable system for isothermal amplification and detection of exosomal microRNAs. Biosens. Bioelectron. 196, 113707. doi:10.1016/j.bios.2021.113707
Ren, Y., Ge, K., Sun, D., Hong, Z., Jia, C., Hu, H., et al. (2022). Rapid enrichment and sensitive detection of extracellular vesicles through measuring the phospholipids and transmembrane protein in a microfluidic chip. Biosens. Bioelectron. 199, 113870. doi:10.1016/j.bios.2021.113870
Rodriguez-Quijada, C., and Dahl, J. B. (2021). Non-contact microfluidic mechanical property measurements of single apoptotic bodies. Biochim. Biophys. Acta Gen. Subj. 1865, 129657. doi:10.1016/j.bbagen.2020.129657
Roy, J. W., Taylor, C. A., Beauregard, A. P., Dhadi, S. R., Ayre, D. C., Fry, S., et al. (2021). A multiparametric extraction method for Vn96-isolated plasma extracellular vesicles and cell-free DNA that enables multi-omic profiling. Sci. Rep. 11, 8085. doi:10.1038/s41598-021-87526-y
Seo, N., Nakamura, J., Kaneda, T., Tateno, H., Shimoda, A., Ichiki, T., et al. (2022). Distinguishing functional exosomes and other extracellular vesicles as a nucleic acid cargo by the anion-exchange method. J. Extracell. Vesicles 11, e12205. doi:10.1002/jev2.12205
Shi, L., Rana, A., and Esfandiari, L. (2018). A low voltage nanopipette dielectrophoretic device for rapid entrapment of nanoparticles and exosomes extracted from plasma of healthy donors. Sci. Rep. 8, 6751. doi:10.1038/s41598-018-25026-2
Shrivastava, S., Ray, R. M., Holguin, L., Echavarria, L., Grepo, N., Scott, T. A., et al. (2021). Exosome-mediated stable epigenetic repression of HIV-1. Nat. Commun. 12, 5541. doi:10.1038/s41467-021-25839-2
Shu, S., Allen, C. L., Benjamin-Davalos, S., Koroleva, M., MacFarland, D., Minderman, H., et al. (2021). A rapid exosome isolation using ultrafiltration and size exclusion chromatography (REIUS) method for exosome isolation from melanoma cell lines. Methods Mol. Biol. 2265, 289–304. doi:10.1007/978-1-0716-1205-7_22
Silva, A. M., Lazaro-Ibanez, E., Gunnarsson, A., Dhande, A., Daaboul, G., Peacock, B., et al. (2021). Quantification of protein cargo loading into engineered extracellular vesicles at single-vesicle and single-molecule resolution. J. Extracell. Vesicles 10, e12130. doi:10.1002/jev2.12130
Smith, J. T., Wunsch, B. H., Dogra, N., Ahsen, M. E., Lee, K., Yadav, K. K., et al. (2018). Integrated nanoscale deterministic lateral displacement arrays for separation of extracellular vesicles from clinically-relevant volumes of biological samples. Lab. Chip 18, 3913–3925. doi:10.1039/c8lc01017j
Song, F., Wang, C., Wang, C., Wang, J., Wu, Y., Wang, Y., et al. (2022). Multi-phenotypic exosome secretion profiling microfluidic platform for exploring single-cell heterogeneity. Small Methods 6, e2200717. doi:10.1002/smtd.202200717
Song, Z., Mao, J., Barrero, R. A., Wang, P., Zhang, F., and Wang, T. (2020). Development of a CD63 aptamer for efficient cancer immunochemistry and immunoaffinity-based exosome isolation. Molecules 25, 5585. doi:10.3390/molecules25235585
Sun, N., Tran, B. V., Peng, Z., Wang, J., Zhang, C., Yang, P., et al. (2022). Coupling lipid labeling and click chemistry enables isolation of extracellular vesicles for noninvasive detection of oncogenic gene alterations. Adv. Sci. (Weinh) 9, e2105853. doi:10.1002/advs.202105853
Tayebi, M., Yang, D., Collins, D. J., and Ai, Y. (2021). Deterministic sorting of submicrometer particles and extracellular vesicles using a combined electric and acoustic field. Nano Lett. 21, 6835–6842. doi:10.1021/acs.nanolett.1c01827
Taylor, C., Chacko, S., Davey, M., Lacroix, J., MacPherson, A., Finn, N., et al. (2020). Peptide-affinity precipitation of extracellular vesicles and cell-free DNA improves sequencing performance for the detection of pathogenic mutations in lung cancer patient plasma. Int. J. Mol. Sci. 21, 9083. doi:10.3390/ijms21239083
Ter-Ovanesyan, D., Norman, M., Lazarovits, R., Trieu, W., Lee, J-H., Church, G. M., et al. (2021). Framework for rapid comparison of extracellular vesicle isolation methods. eLife 10, e70725. doi:10.7554/eLife.70725
Thakur, A., Xu, C., Li, W. K., Qiu, G., He, B., Ng, S. P., et al. (2021). In vivo liquid biopsy for glioblastoma malignancy by the AFM and LSPR based sensing of exosomal CD44 and CD133 in a mouse model. Biosens. Bioelectron. 191, 113476. doi:10.1016/j.bios.2021.113476
Tulkens, J., De Wever, O., and Hendrix, A. (2020). Analyzing bacterial extracellular vesicles in human body fluids by orthogonal biophysical separation and biochemical characterization. Nat. Protoc. 15, 40–67. doi:10.1038/s41596-019-0236-5
Turner, N. P., Abeysinghe, P., Kwan Cheung, K. A., Vaswani, K., Logan, J., Sadowski, P., et al. (2022). A comparison of blood plasma small extracellular vesicle enrichment strategies for proteomic analysis. Proteomes 10, 19. doi:10.3390/proteomes10020019
Ullah Khan, N., Muhammad, Z., Liu, X., Lin, J., Zheng, Q., Zhang, H., et al. (2021). Ultrasensitive detection of exosome using biofunctionalized gold nanorods on a silver-island film. Nano Lett. 21, 5532–5539. doi:10.1021/acs.nanolett.1c00830
Vergauwen, G., Tulkens, J., Pinheiro, C., Avila Cobos, F., Dedeyne, S., De Scheerder, M. A., et al. (2021). Robust sequential biophysical fractionation of blood plasma to study variations in the biomolecular landscape of systemically circulating extracellular vesicles across clinical conditions. J. Extracell. Vesicles 10, e12122. doi:10.1002/jev2.12122
Wang, Z., Li, F., Rufo, J., Chen, C., Yang, S., Li, L., et al. (2020). Acoustofluidic salivary exosome isolation: A liquid biopsy compatible approach for human papillomavirus-associated oropharyngeal cancer detection. J. Mol. Diagn 22, 50–59. doi:10.1016/j.jmoldx.2019.08.004
Wang, Z., Wang, H., Becker, R., Rufo, J., Yang, S., Mace, B. E., et al. (2021). Acoustofluidic separation enables early diagnosis of traumatic brain injury based on circulating exosomes. Microsyst. Nanoeng. 7, 20. doi:10.1038/s41378-021-00244-3
Wei, Z., Zhao, Y., Hsu, P., Guo, S., Zhang, C., and Zhong, B. (2021). Exosomes for gene therapy effectively inhibit the endothelial-mesenchymal transition in mouse aortic endothelial cells. BMC Musculoskelet. Disord. 22, 1000. doi:10.1186/s12891-021-04896-0
Wu, B., Chen, X., Wang, J., Qing, X., Wang, Z., Ding, X., et al. (2020). Separation and characterization of extracellular vesicles from human plasma by asymmetrical flow field-flow fractionation. Anal. Chim. Acta 1127, 234–245. doi:10.1016/j.aca.2020.06.071
Wu, J., Zeng, D., Zhi, S., Ye, Z., Qiu, W., Huang, N., et al. (2021). Single-cell analysis of a tumor-derived exosome signature correlates with prognosis and immunotherapy response. J. Transl. Med. 19, 381. doi:10.1186/s12967-021-03053-4
Wu, M., Ouyang, Y., Wang, Z., Zhang, R., Huang, P. H., Chen, C., et al. (2017). Isolation of exosomes from whole blood by integrating acoustics and microfluidics. Proc. Natl. Acad. Sci. U. S. A. 114, 10584–10589. doi:10.1073/pnas.1709210114
Xiong, H., Huang, Z., Lin, Q., Yang, B., Yan, F., Liu, B., et al. (2022). Surface plasmon coupling electrochemiluminescence immunosensor based on polymer dots and AuNPs for ultrasensitive detection of pancreatic cancer exosomes. Anal. Chem. 94, 837–846. doi:10.1021/acs.analchem.1c03535
Xu, Y., Li, X., Niu, C., Wu, H., Yong, Y., Qi, C., et al. (2022). Janus wireframe DNA cube-based 3D nanomachine for rapid and stable fluorescence detection of exosomal microRNA. Biosens. Bioelectron. 212, 114405. doi:10.1016/j.bios.2022.114405
Yang, K., Jia, M., Cheddah, S., Zhang, Z., Wang, W., Li, X., et al. (2022). Peptide ligand-SiO2 microspheres with specific affinity for phosphatidylserine as a new strategy to isolate exosomes and application in proteomics to differentiate hepatic cancer. Bioact. Mater 15, 343–354. doi:10.1016/j.bioactmat.2021.12.017
Yang, Z., Atiyas, Y., Shen, H., Siedlik, M. J., Wu, J., Beard, K., et al. (2022). Ultrasensitive single extracellular vesicle detection using high throughput droplet digital enzyme-linked immunosorbent assay. Nano Lett. 22, 4315–4324. doi:10.1021/acs.nanolett.2c00274
Yu, X., Chen, X., Sun, Z., Niu, R., Deng, Y., Wang, L., et al. (2022). Ultracentrifugation-free enrichment and quantification of small extracellular vesicles. Anal. Chem. 94, 10337–10345. doi:10.1021/acs.analchem.1c05491
Yu, Z., Lin, S., Xia, F., Liu, Y., Zhang, D., Wang, F., et al. (2021). ExoSD chips for high-purity immunomagnetic separation and high-sensitivity detection of gastric cancer cell-derived exosomes. Biosens. Bioelectron. 194, 113594. doi:10.1016/j.bios.2021.113594
Yu, Z. L., Liu, X. C., Wu, M., Shi, S., Fu, Q. Y., Jia, J., et al. (2022). Untouched isolation enables targeted functional analysis of tumour-cell-derived extracellular vesicles from tumour tissues. J. Extracell. Vesicles 11, e12214. doi:10.1002/jev2.12214
Zeng, L., Chen, X., Du, J., Yu, Z., Zhang, R., Zhang, Y., et al. (2021). Label-free separation of nanoscale particles by an ultrahigh gradient magnetic field in a microfluidic device. Nanoscale 13, 4029–4037. doi:10.1039/d0nr08383f
Zeng, L., Hu, S., Chen, X., Zhang, P., Gu, G., Wang, Y., et al. (2022). Extraction of small extracellular vesicles by label-free and biocompatible on-chip magnetic separation. Lab. Chip 22, 2476–2488. doi:10.1039/d2lc00217e
Zhang, H., and Lyden, D. (2019). Asymmetric-flow field-flow fractionation technology for exomere and small extracellular vesicle separation and characterization. Nat. Protoc. 14, 1027–1053. doi:10.1038/s41596-019-0126-x
Zhang, J., Zhu, Y., Guan, M., Liu, Y., Lv, M., Zhang, C., et al. (2022). Isolation of circulating exosomes and identification of exosomal PD-L1 for predicting immunotherapy response. Nanoscale 14, 8995–9003. doi:10.1039/d2nr00829g
Zhang, L., Yin, W., Tong, Y., Zhang, Y., Xu, Y., Liu, S. Y., et al. (2022). Highly efficient isolation and sensitive detection of small extracellular vesicles using a paper-based device. Anal. Chem. 94, 10991–10999. doi:10.1021/acs.analchem.2c01378
Zhang, P., Zhou, X., and Zeng, Y. (2019). Multiplexed immunophenotyping of circulating exosomes on nano-engineered ExoProfile chip towards early diagnosis of cancer. Chem. Sci. 10, 5495–5504. doi:10.1039/c9sc00961b
Zhang, Y., Murakami, K., Borra, V. J., Ozen, M. O., Demirci, U., Nakamura, T., et al. (2022). A label-free electrical impedance spectroscopy for detection of clusters of extracellular vesicles based on their unique dielectric properties. Biosens. (Basel) 12, 104. doi:10.3390/bios12020104
Zhao, L., Wang, H., Fu, J., Wu, X., Liang, X. Y., Liu, X. Y., et al. (2022). Microfluidic-based exosome isolation and highly sensitive aptamer exosome membrane protein detection for lung cancer diagnosis. Biosens. Bioelectron. 214, 114487. doi:10.1016/j.bios.2022.114487
Zhao, W., Zhang, L., Ye, Y., Li, Y., Luan, X., Liu, J., et al. (2021). Microsphere mediated exosome isolation and ultra-sensitive detection on a dielectrophoresis integrated microfluidic device. Analyst 146, 5962–5972. doi:10.1039/d1an01061a
Zheng, L., Wang, H., Zuo, P., Liu, Y., Xu, H., and Ye, B. C. (2022). Rapid on-chip isolation of cancer-associated exosomes and combined analysis of exosomes and exosomal proteins. Anal. Chem. 94, 7703–7712. doi:10.1021/acs.analchem.2c01187
Keywords: exosomes, extracellular vesicles, isolation methods, isolation technologies, review
Citation: Gao J, Li A, Hu J, Feng L, Liu L and Shen Z (2023) Recent developments in isolating methods for exosomes. Front. Bioeng. Biotechnol. 10:1100892. doi: 10.3389/fbioe.2022.1100892
Received: 17 November 2022; Accepted: 22 December 2022;
Published: 13 January 2023.
Edited by:
Gang Wu, VU Amsterdam, NetherlandsReviewed by:
Chengjun Huang, Institute of Microelectronics (CAS), ChinaCopyright © 2023 Gao, Li, Hu, Feng, Liu and Shen. This is an open-access article distributed under the terms of the Creative Commons Attribution License (CC BY). The use, distribution or reproduction in other forums is permitted, provided the original author(s) and the copyright owner(s) are credited and that the original publication in this journal is cited, in accordance with accepted academic practice. No use, distribution or reproduction is permitted which does not comply with these terms.
*Correspondence: Zuojun Shen, enVvanVuc2hlbkB1c3RjLmVkdS5jbg==; Liu Liu, YWxpY2VsbEB1c3RjLmVkdS5jbg==
Disclaimer: All claims expressed in this article are solely those of the authors and do not necessarily represent those of their affiliated organizations, or those of the publisher, the editors and the reviewers. Any product that may be evaluated in this article or claim that may be made by its manufacturer is not guaranteed or endorsed by the publisher.
Research integrity at Frontiers
Learn more about the work of our research integrity team to safeguard the quality of each article we publish.