- 1State Key Laboratory of Oral Diseases & National Clinical Research Center for Oral Diseases, Sichuan University, Chengdu, Sichuan, China
- 2Department of Preventive Dentistry, Guanghua School of Stomatology, Sun Yat-sen University, Guangzhou, Guangdong, China
- 3Department of Operative Dentistry and Endodontics, West China School of Stomatology, Sichuan University, Chengdu, Sichuan, China
Untreated dental caries, tooth trauma and dental anatomical variations such as dens invaginatus can result in pulpitis. However, standard root canal therapy cannot treat immature permanent teeth due to an open apical foramen and thin dentinal walls. Thus, regenerative endodontics treatment (RET) following a disinfection step with pulp regeneration has been developed. Pulp connective-tissue, dentin formation, revascularization and reinnervation can occur in this procedure which should be supplemented with intelligent biomaterials to improve repeatability and support well-coordinated regeneration. Furthermore, nanofibrous scaffolds, as one of the most commonly used materials, show promise. The purpose of this article is to highlight the advantages of nanofibrous scaffolds and discuss the future modification and application of them.
1 Introduction
Untreated dental caries, tooth trauma, and dental anatomical variations such as dens invaginatus can result in endodontic infections and inflammatory pulpal reactions, a highly prevalent inflammatory condition worldwide (Bletsa et al., 2006; Agnihotry et al., 2016; Estefan et al., 2016). This kind of inflammation can be reversed with appropriate treatment at an early stage (Bjørndal et al., 2014). However, as inflammation progresses, irreversible pulpitis, which manifests as violently acute pain in the tooth, occurs frequently (Lin et al., 2020). If the irreversible pulpits are not treated promptly, it can induce the dental necrosis, apical periodontitis, abscess, and eventual tooth loss (Bjørndal et al., 2019). Current endodontic treatment concepts for fully developed necrotic permanent teeth include removing inflammatory or necrotic pulp tissue and replacing it with biomaterials (Jung et al., 2019). Nowadays, the most popular therapeutic strategy is root canal therapy (RCT), which includes debridement, instrumentation, and obturation of the pulp canal space (Ma et al., 2016; Manfredi et al., 2016). However, the traditional RCT is not recommended for the immature permanent teeth in children due to their thinner dentinal walls and unclosed apical foramen compared to mature permanent teeth (Mc et al., 2019). The apexogenesis/apexification (Figure 1) is an alternative method to solve this challenge. It entails removing all necrotic pulp tissues and utilizing biomaterials such as mineral trioxide aggregate (MTA) or calcium hydroxide (Ca(OH)2) to create an artificial barrier or stimulate the creation of a mineralized barrier in the root apex after enough debridement (Frank, 1966; Heithersay, 1975; Rafter, 2005). However, apexogenesis does not restore the pulp’s vitality and immunocompetence and cannot promote the root maturation (the closure of the apical foramen as mature permanent teeth and/or the thickening of the root canal walls), which may compromise tooth and root wall stability and eventually increase the likelihood of dental secondary trauma, even tooth loss (Andreasen et al., 2002; Kim et al., 2018; Mc et al., 2019). Likewise, apexification cannot ensure the root maturation completely and it need repeated return visits over a long period to determine the final effect for every patient (Rafter, 2005).
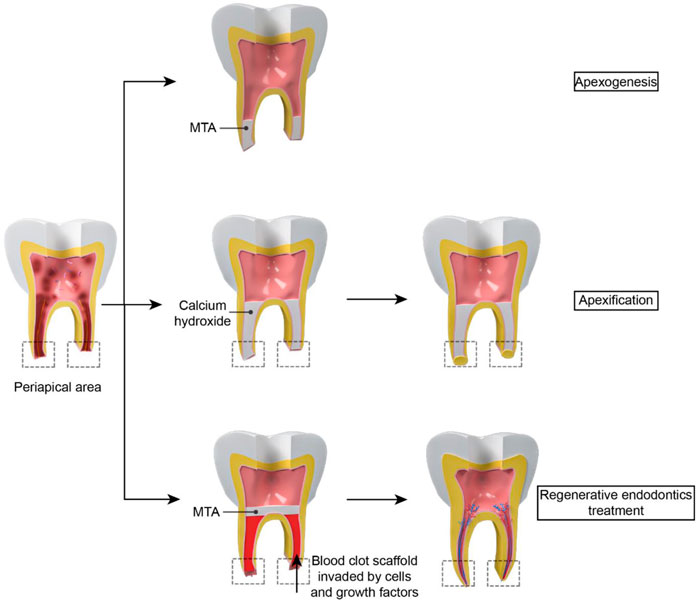
FIGURE 1. The difference between apexogenesis, apexification and RET: For apexogenesis, MTA is placed in the apical 1/3 of the root canal to form an unnatural barrier. For apexification, biomaterials such as calcium hydroxide is put in the root to stimulate the creation of a mineralized barrier in the root apex. However, vessels cannot regenerate in the pulp cavity. For RET, periapical tissue tearing results in a blood clot which acts as a natural scaffold for stem cells and growth factor to grow into. Then the closure of the apical foramen and/or the thickening of the root canal walls can be observed in the treatment.
Pulp regeneration (Figure 1) is another approach for immature permanent teeth necrosis. A healthy and alive pulp is required for long-term tooth survival and preservation (Schmalz et al., 2020). In 2001, Iwaya et al. (Iwaya et al., 2001) established a novel treatment technique to address a young permanent tooth which was immature and suffered from apical periodontitis and then named it as “revascularization”. In 2007, “regenerative endodontics”, which is based on a tissue engineering notion, was coined by the American Association of Endodontists, indicating this kind of regeneration included both soft and hard tissues rather than vessels (Murray et al., 2007).
Regenerative endodontics treatment (RET) refers to biologically based procedures to replace damaged tooth tissues with newborn tissues (Schmalz et al., 2020). Following a disinfecting phase, RET induces bleeding by periapical tissue tearing. Blood clot can act as a natural scaffold which provide a platform for stem cells to grow into. Various endodontic studies have shown that RET could improve root maturation and reinforce thin and weak young roots (Kahler and Rossi-Fedele, 2016; Chan et al., 2017). RET also led to comparable survival and success rates compared to apexification (Ribeiro et al., 2020). However, this treatment needs the supplement of innovative biomaterials to improve repeatability and encourage a well-orchestrated regeneration (Keller et al., 2015).
RET includes three crucial parts in regeneration engineering: stem cells, bioactive molecules, and scaffolds. Stem cells can serve as a source to differentiate into different cells and form new regeneration-related tissues. Bioactive molecules can promote the cell migration, growth. The scaffold is considered an essential factor in the RET to coordinate drug delivery of active biomolecules or antibiotics and offers a surface for cells to communicate, migrate, proliferate, and spatially organize (Nakashima and Akamine, 2005; Galler et al., 2011).
With the development of pulp regeneration, the scaffolds become one of the key factors to improve the RET’s success rate. Here we summarized the research and development of nanofibrous scaffolds in RET to highlight the advantages of nanofibrous and discuss the future modification and application of nanofibrous.
2 Nanofibrous scaffolds
An essential process of the RET is placing a scaffold to generate a three-dimensional (3D) microenvironment for supporting cells, resulting in better outcomes of restoring tissue function (Gupte and Ma, 2012). A variety of materials can be utilized to construct scaffolds in regeneration engineering (Galler et al., 2011). Synthetic polymers [such as polymers of glycolic acid (PGA), polymers of lactic acid (PLA)], and biological matrices (such as reconstituted collagen and fibrin) are two principal alternatives (Galler et al., 2011; Zein et al., 2019). A suitable scaffold should closely be similar to the natural extracellular matrix (ECM), which is the physiological microenvironment of the cells (Gupte and Ma, 2012). ECM is the cell-environmental nanofibrous protein network in all tissues and consists of various nano-scale proteins, such as fibronectin, vitronectin and collagen (Kwon et al., 2005). This matrix can serve as structural support for cell adhesion and ingrowth and give cells chemical signals to govern their activity and reinforce a specific phenotypic (Galler et al., 2011).
Recent breakthroughs in nanotechnology have tremendously aided in creating innovative ECM-mimicking materials. Nanofibrous scaffolds which are becoming increasingly common in the RET can improve adhesion, allow cells to manifest more normal 3D morphologies when compared with smooth materials, solid films, and tissue culture polymers (Gupte and Ma, 2012; Mc et al., 2019). A nanofibrous scaffold is characterized by the diameter ranging from 1 nm to 100 nm, similar to the size of collagen (Sheikh et al., 2015). And it has a large surface area inversely linked to diameter, facilitating cellular adhesion and migration. Furthermore, the pores of nanofibers are suitable for the cell adhesion and penetration. Cells grown on very porous nanofibrous meshes have been proved to increase cellular adhesion and multiply (Kwon et al., 2005). Thus, nanofibrous scaffolds become an ideal material for RET due to nano-scale fibre, large surface area, and interconnected porosity similar to the ECM.
Biodegradability is also an important character for tissue regeneration. Most nanofibrous scaffolds are biodegradable and can be chemically destroyed in the biological environment by enzymes such as lysozyme (Sheikh et al., 2015). The degradability of the nanofibers is determined by two significant factors: the chemical composition of the fibres and hydrophilicity. Therefore, by adjusting these two factors, the biodegradation speed of scaffolds can be controlled in line with the rate of tissue regeneration for better regeneration. The biocompatibility of scaffolds’ broken down chemical compounds matters as well. To better pulp regeneration, the material of the nanofibrous scaffold should be taken into consideration.
Acting as the reservoirs for stem cells, antimicrobial, anti-inflammatory molecules, and growth factors is a considerable advantage for nanofibrous scaffolds (Albuquerque et al., 2015; Keller et al., 2015). Thus, by providing physical and chemical stimulation, nanofibrous scaffolds have been shown to drive positive cell interactions, stimulate cell growth, preserve cell phenotypics, assist stem cell development, and activate cell-signaling pathways (Sun et al., 2010; Gupte and Ma, 2012; Li et al., 2020).
Currently, several materials processing techniques, including electrospinning, molecular self-assembly, and thermally induced phase separation, have also been developed for the production of nanofibrous scaffolds (Gupte and Ma, 2012; Albuquerque et al., 2014).
Electrospinning is extensively employed to make natural and synthetic polymer fibres because of its ease of usage and compatibility with practically any dissolvable polymer. A strong electric field is applied to control the deposition of polymer fibres with diameters ranging from nanometers to micrometers. Fibre morphology, pore size, and fibre alignment may be altered by adjusting electrospinning parameters as well (Yang et al., 2005; Gupte and Ma, 2012). However, it is frequently unable to make real nanofibers at 100 nm or less using commonly used biodegradable polymers. In addition to low production efficiency, factors such as air humidity, temperature, and air velocity in the spinning environment are difficult to control which affect the physical properties of nanofibrous scaffold can also be its shortcomings to achieve industrial production (Sill and von Recum, 2008).
Molecular self-assembly is a bottom-up technique that has been utilized to create nanofibres as small as 10 nm by using spontaneous molecular organization via weak non-covalent interactions (Dahlin et al., 2011). Molecular self-assembly nanofibers for RET benefit from being built into solutions and producing gels utilized for stem cell encapsulation (Rosa et al., 2013). Besides, the fluid may be administered via a minimally invasive technique, resulting in an in-suit nanofibrous structure. However, this method has limitations in controlling pore size/shape inside the hydrogel scaffold (Gupte and Ma, 2012) and poor mechanical properties in general (Zhang et al., 2012).
Thermally-induced phase separation (TIPS) involves drastically quenching a single-phase homogenous polymer solution, inducing separation into a solvent-rich phase and a polymer-rich phase and with fiber diameters between 50 and 500 nm. It is a technique that has been utilized for a long time to make membranes and scaffolds. This method is common to create nanofibrous scaffolds. TIPS can also be used with other approaches to create macro/micropore/channel networks within 3D nanofibrous scaffolds (Gupte and Ma, 2012).
The application of nanofiber scaffolds in RET can be devived into two parts in immature teeth based on tissue-engineering-techniques (Gupte and Ma, 2012): 1) bioactive nanofibrous scaffold with antibiotics for root canal disinfection. 2) nanofibrous scaffolds with stem cells and/or biomolecules for dental regeneration in a broad sense, which contains four parts: pulp connective-tissue formation; dentin formation; revascularization; reinnervation (Figure 2, Figure 3).
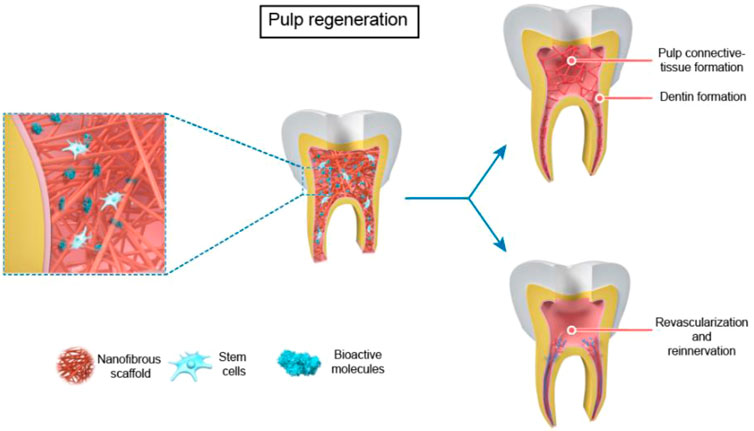
FIGURE 3. The use of nanofibrous scaffolds in pulp regeneration --- Pulp Connective -Tissue and Dentin Formation, Revascularization and Reinnervation.
3 Nanofibrous scaffolds for root canal disinfection
Pulp regeneration processes can be quite complex (Schmalz et al., 2020), and bacteria in the root canal further complicates the RET (Kim et al., 2018). Over 600 bacterial species have been identified as potentially involved with pulp infections (Sampaio-Maia et al., 2016). The microbial ecology in the pulp canals of infected young permanent teeth was comparable to that of mature permanen teeth (Nagata et al., 2014). Enterococcus faecalis (E. faecalis) is commonly isolated from early-root canal infections. Prophyromonas, Treponema, Bacteroides, Fusobacterium, Prevotella, Peptostreptococcos, Eubacterium, and Campylobacter species are the most commonly found endodontic bacteria in primary endodontic infections (Neelakantan et al., 2017). Bacteria can not only enter the dentinal canal tubules of infected teeth but form biofilms on the radicular canal walls (Lin et al., 2014). Previously, the most commonly used drug in clinical practice for successful disinfection is triple antibiotic paste (TAP), which is made up of equivalent doses of metronidazole (MET), ciprofloxacin (CIP) and minocycline (MINO) (Arruda et al., 2018). The antibacterial effects of this drug are widely recognized in the therapeutic setting, but it has garnered numerous complaints (Kim et al., 2018). One of the most crucial issues reported at TAP was dentin discolouration induced by the presence of MINO (Porter et al., 2016). Therefore, the double antibiotic paste (DAP) combining MET and CIP or the incorporation of ampicillin (AMP) into a new TAP formulation were used (Arslan et al., 2015). However, antibiotic pastes at therapeutically recommended doses will impair the viability of human apical papilla stem cells (SCAPs) (Ruparel et al., 2012). In view of the above disadvantages, Ca (OH)2 is commonly used in RET currently. However, it also has several disadvantages. For example, it can form a calcified barrier in the root canal, hindering the growth of pulp tissue (Song et al., 2017; Almutairi et al., 2022). And because of its high pH, it may destroy cells from the apical papilla and periapical tissue as well (Banchs and Trope, 2004; Jadhav et al., 2012).
Thus, it is crucial to employ biomaterials that reduce germs in the root canal while remaining non-cytotoxic and encouraging the creation of new tissues (Murray, 2018). New nano-scaffolds which are capable of serving as drug delivery vehicles in a regulated manner depending on therapeutic use are developed (Sundararaj et al., 2014). Compared to propylene glycol, which is the most often utilized carrier for delivering antimicrobial pastes to the root canal, nanofibers have the potential benefit of increasing the surface area and, presumably, allowing for controllable drug release over a more extended period (Bottino et al., 2013; Palasuk et al., 2014). Figure 2 and Table 1 summarized the use of nanofibrous scaffolds in root canal disinfection.
In 2013, Bottino et al. (Bottino et al., 2013) mixed polydioxanone monofilament solution material (PDS-Ⅱ) with MET or CIP in different concentrations (5% or 25%), and typical electrospinning procedures were applied. Almost all scaffolds demonstrated an early burst followed by a general linear continuous drug release. Within the first 48 h, all nanofibrous scaffolds only release a portion of the medication from 22.4% to 51.4% indicated the sustained drug release from the scaffolds. All CIP-containing scaffolds strongly reduced the growth of Porphyromonas gingivalis (P.gingivalis) and E. faecalis. However, MET-containing’s have no E. faecalis resistance. Although they all have good antibacterial properties, only the 25% wt CIP-containing extract had a substantial adverse effect on Human dental pulp stem cells (hDPSCs).
A nanofibrous scaffold by combining MET and CIP in different ratios and using PDS as a drug carrier is created in another study (Palasuk et al., 2014). All scaffolds containing antibiotics suppressed the growth of P. gingivalis, E. faecalis, and Fusobacterium nucleatum (F. nucleatum), except for the MET-containing scaffold. When compared to scaffolds integrated with individual antimicrobials, scaffolds with two antibiotics did not increase their antimicrobial abilities. Moreover, antibiotic-containing aliquots slightly reduced cell viability to hDPSC.
To further determine the drug release abilities of the new dual-mix antibacterial scaffolds and their effect on the proliferation and activity of hDPSCs. Kamocki et al. (Kamocki et al., 2015) uses electrospinning to create PDS-based nanofibrous scaffolds containing MET and CIP. Pure PDS scaffolds are utilized as the negative (non-toxic) controls, while saturated CIP/MET solution scaffolds are treated as the positive (toxic) groups. The results show no differences in cell proliferation in the pure PDS or MET scaffold groups. For pure CIP-containing scaffolds, increasing drug concentration led to decreased cell viability. However, no cells could be observed after exposing to the saturated CIP/MET solution after 3 days, indicating that antibiotic-containing nanofibrous scaffolds had considerably reduced impacts on hDPSC proliferation. Pankajakshan et al. (Pankajakshan et al., 2016) discovered a similar experimental phenomenon: antibiotic–containing (MINO, CIP, MET) polymer nanofibers resulted in numerous bacterial killing but had no effect on hDPSC adhesion and growth on dentin when compared to a saturated TAP solution. These studies suggest that nanofibrous scaffolds are more biologically friendly to cells when compared with antibiotic paste.
Karczewski et al. (Karczewski et al., 2018) changed the formula of triple antibiotics, including MET, CIP, and clindamycin (CLIN). Hydrated triple antibiotic-containing nanofibrous scaffolds showed pronounced antimicrobial activity against Actinomyces naeslundii (A. naeslundii), Aggregatibacter actinomycetemcomitans (A. actinomycetemcomitans), and E. faecalis, but with no cytotoxic effects on hDPSC indicating that this kind of triple antibiotic-containing scaffolds might be a feasible option in the RET. Bottino et al. (Mc et al., 2019) optimized electrospinning parameters and created a 3D tubular triple antibiotic-eluting construct to increase the antibacterial activity and the ability to remove bacterial biofilm. The 3D nanofibrous scaffold created an optimal environment with apical foramen closure and a thin layer of osteodentin-like tissue within the root canal.
In general, compared with antibiotic pastes, utilizing nanofibrous scaffolds as drug-carrying carriers is more conducive to drug release and increase the capacity of pathogen clearance in the first stage of pulp regeneration. Moreover, it has no toxic effects on stem cells during regeneration. However, there are also some limitations in these researches. The main problem is the lack of in vivo studies including antibacterial, degradability and compatibility. Only one article established a canine model of periapical disease. The rest of the studies were still limited to in vitro experiments which lack of enough reliability. At the same time, as drug-loaded scaffolds, they also lack the exploration of the best speed for drug release as well as the best degradation rate of the scaffolds which determine the long-term bactericidal ability.
4 Nanofibers for dental pulp regeneration in the broad sense
4.1 Nanofibrous scaffolds for pulp connective-tissue and dentin formation
Potential cell sources for the RET include hDPSCs, stem cells from human exfoliated deciduous teeth (SHED) and stem cells of the apical papilla (Huang et al., 2008; Morsczeck and Reichert, 2018). These stem cells were incubated with different materials to explore their ability in the RET. Table 2 summarized all the nanofibrous scaffolds used in Pulp Connective-Tissue and Dentin Formation.
Yang et al. (Yang et al., 2010) seeded hDPSCs on electrospun polycaprolactone (PCL) and gelatin scaffolds with or without nano-hydroxyapatite (nHA). The cells showed the growth and mineralization properties on both the scaffolds. Moreover, a thin fibrous tissue capsule enveloped all implants and osteocyte-like cells and osteoblast-like cells orderly arranged at the scaffolds’ surface in mice subcutaneous transplantation model. And, the incorporation of nHA into scaffolds could increase the expression of specific odontogenic genes.
(Qu and Liu, 2013) created 3D nanofibrous gelatin andsilica bioactive glass (NF-gelatin/SBG) hybrid scaffolds. The hDPSCs proliferated significantly faster on NF-gelatin/SBG scaffolds which promoted better cell differentiation and biomineralization compared to NF-gelatin scaffolds. And the same research group found the 3D NF-gelatin andmagnesium phosphate hybrid scaffolds had a similar effect on hDPSCs which was proved in animal experiments as well (Qu et al., 2014).
(Kim et al., 2014) utilized apatite to deal with the scaffolds’ surface and created mineralized PCL nanofibrous scaffolds. They tested their ability to induce odontogenic differentiation of human dental pulp cells (hDPCs) and the situation of cell adhesion, growth on the scaffolds’ suface. Mineralized PCL scaffold showed better capability in cell growth, mineralized nodule formation, and expression of odontoblastic marker genes compared with the pure PCL scaffolds. And these mineralized scaffolds were appealing for regenerating dentin tissue because they promoted odontogenic differentiation and growth of hDPCs via the integrin-mediated signaling pathway. And the same group proved that the bioactive glass nanoparticles - biopolymer blend PCL-gelatin composite nano matrix could promote the odontogenic differentiation of HDPCs by activating the BMP, integrin, and mitogen-activated protein kinase signaling pathway (Kim et al., 2015). Moreover, better ability of promoting cell adhesion, growth and odontoblastic differentiation of hDPCs on collagen nanofibrous matrix (Col_NFM) versus collagen flat film (Col-FF) were also observed by Zhang’s group (Zhang et al., 2019).
In addition to using different nanofibrous scaffolds, active biomolecules are also combined with scaffolds to enhance the ability of dental pulp regeneration.
Galler et al. (Galler et al., 2008) combined SHED and hDPSCs with peptide-amphiphile (PA) hydrogel scaffolds which could form 3D nanofibrous networks by self-assemble method. After 4 weeks of cultivating stem cells with different osteogenic supplements, SHED and hDPSCs could proliferate and differentiate within the scaffolds. In vitro experiment showed that SHED led to collagen production, while hDPSCs could expressed osteoblast marker genes, manifested an osteoblast-like phenotype, and deposited minerals.
Leite et al. (Leite et al., 2021) developed and evaluated fibronectin (FN)-loaded PCL nanofiber scaffolds of PCL on human apical papilla cells (hAPCs). FN has been postulated as a chemotactic agent that promotes cell migration, adhesion, growth, and differentiation (Grigoriou et al., 2017). The FN added to the scaffolds increase the cell viability, migration, adhesion, growth, and gene expression (ITGA5, ITGAV, COL1A1, COL3A1) at a dose dependent manner.
In order to examine the odontogenic development of hDPSCs, Wang et al. (Wang et al., 2010) fabricated highly porous nanofibrous PLLA scaffolds that resembled the structure of collagen type I fibres. Stem cells were sown onto the scaffolds and grown in various conditions to induce odontogenic differentiation. Both the “DXM” medium (medium containing dexamethasone (DXM)) and “BMP-7+DXM” medium [medium containing DXM, ascorbic acid, β-glycerophosphate plus bone morphogenetic protein 7 (BMP-7)] promoted DPSCs to odontoblast-like cells. After ectopic implantation in nude mice, the “BMP-7 + DXM” group showed greater ECM and complicated tissue development.
The dexamethasone (DEX) - releasing nanofiber matrices with bioactive glass nanoparticles (BGNs) scaffolds were considered a promising therapeutic nano matrix for RET by Lim’s group (Lim et al., 2016). These scaffolds were shown to enhance the odontogenic ability of hDPCs. Bone morphogenetic protein, the integrins, and mTOR signaling pathways were involved in this process (Lim et al., 2016). (Zijah et al., 2017a) aimed to assess and compare the effect of poly-caprolactone-poly-ethylene glycol-poly caprolactone (PCL-PEG-PCL) nanofibrous scaffold containing 3 different biofactors, including sodium fluoride (NaF), melanocyte-stimulating hormone (MSH), and simvastatin (SIM). They found that MSH- and SIM-treated cells had greater adhesive behavior, vitality, dentin-specific gene expression and alizarin red activity.
In terms of nanofibrous scaffolds promoting pulp connective-tissue and dentin formation, we can find scaffolds promoted better cell adhesion, growth, differentiation and biomineralization or high expression of odontoblastic marker genes in vitro experiments or mice subcutaneous transplantation model. However, we can’t clearly find the closure of the apical foramen and/or the thickening of the root canal walls in animal experiments. Thus, we need to complete more animal experiments to perfect this defect before clinical trials.
4.2 Nanofibrous scaffolds for revascularization and reinnervation
In addition to promoting cell growth, differentiate, nanofibrous scaffolds can also promote angiogenesis and reinnervation in pulp regeneration. The vascularization and reinnervation capabilities of a scaffold are crucial for RET. Table 3 summarized the scaffolds used in revascularization and reinnervation.
Dissanayaka et al. (Dissanayaka et al., 2015) utilized the peptide hydrogel PuraMatrix to test its vascularization ability. In 3D PuraMatrix, they enclosed Human umbilical vein endothelial cells (HUVECs), hDPSCs, or cocultures of these 2 cells and found that hDPSCs aided in developing early vascular networks by promoting the migration of HUVECs and boosting vascular endothelial growth factor (VEGF) expression. Both the hDPSC-only and coculture groups showed vascularized pulp-like tissue with patches of osteodentin in mice subcutaneous transplantation model. Also, Galler et al. (Galler et al., 2012) encapsulated hDPSCs in a self-assembling hydrogel integrated VEGF, fibroblast growth factor primary and transforming growth factor b1. A vascularized soft connective tissue compared to the tooth pulp generated after subcutaneous transplantation within dentin cylinders into mice. Likewise, Li et al. (Li et al., 2016) manufactured a novel hierarchical growth factor-loaded nanofibrous scaffold to solve the vascularization problem. Heparin-binding VEGF was encased in heparin-conjugated gelatin nanospheres, which were subsequently confined in the nanofibers of an injectable PLLA microsphere. This structure shielded the VEGF against degradation and denaturation, and also showed a precise control over its long-term release. After implantation of tooth loaded with nanofibrous scaffolds into immunocompromised nude, the pulp-like tissues filled the whole apical and 2/3 of the root canal, and reached the coronal third. The blood vessels could also be found in the canal.
Yun et al. (Yun et al., 2016) tested the impact of magnetic nanofibrous scaffolds (MNS) on the behaviors of hDPCs. The results indicated that the magnetic scaffolds increased hDPCs development and also promoted the hDPCs-induced angiogenesis of endothelial cells. Soares et al. (Soares et al., 2018) investigated the NF-PLLA scaffolds in mouse model and demonstrated that the addition of simvastatin to a hDPCs/NF-PLLA scaffold might dramatically suppress the production of pro-inflammatory mediators. The detrimental effects of LPS on odontoblastic marker expression might be reversed. Meanwhile, the NF-PLLA nanofibrous scaffolds allowed the HUVECs to form vessel-like structures inside.
For reinnervation, Yoo et al. (Yoo et al., 2018) seeded the hDPCs onto PCLF and PCLF/DMOG dentin slices before transplanting them into mice. The PCLF/DMOG scaffolds increased the expression of VEGF, dentin sialoprotein, bone sialoprotein in the hDPCs, mouse VEGF, mouse neurofilament light polypeptide and mouse platelet endothelial cell adhesion molecule one in the surrounding host cells. These data indicated that pulp-dentin complex regenerated by increasing odontoblastic differentiation of hDPCs, host cell recruitment, neurogenesis and angiogenesis was potentially promoted by PCLF/DMOG.
In conclusion, nanofibrous scaffolds with stem cells and/or active biomolecules promote stem cell-mediated pulp regeneration of pulp connective-tissue formation, dentin formation, revascularization, reinnervation. Currently, nanofibrous scaffolds used for vascular and nerve regeneration are relatively small, and all in vivo experiments were still limited to subcutaneous implantation in mice, not in either human or animal pulpless tooth roots. Though, their studies positively showed their regenerative potential to develop early pulp-like tissues by promoting the migration of stem cells and forming vascularized soft connective tissue. However, we still have a long way to go: Does bioparticle-loaded nanofibrous scaffolds have the same effect in immature pulpless teeth? What is the best apical opening size for the scaffolds? What is the optimal degradation rate of the scaffolds for pulp regeneration? In order to avoid cell damage while maintaining the support force of the scaffolds, how much hardness should be selected?
5 Conclusion
Currently, there are two significant challenges in the pulp regeneration, including the disinfection of the pulp and the regeneration of tooth-related issues. Regarding pulp disinfection, antibiotic pastes at therapeutically recommended doses impaired the viability of stem cells. And pastes cannot be released at a steady and lasting pace as well. As for the regeneration of tooth-related issues, RET has to be supplemented with scaffolds to improve repeatability and encourage a well-orchestrated regeneration. To address the challenges, nanofibrous scaffolds mimicking extra-cellular matrix (ECM) have been developed. They can act as antibacterial molecule reservoirs and provide growth factors, stem cells to guide the various cells’ migration, growth, and differentiation. However, most of the application of nanofibrous scaffolds is still at the laboratory research stage and lack of the in vivo and clinical investigation and evaluation. Meanwhile, we still have a lot of details to work out, including: the optimal degradation rate and drug release rate, the best hardness, suitable physical properties etc. Moreover, no single material can perfectly combine the antibacterial characteristics and stimulate regeneration of connective-tissue, dentin, nerves, and blood vessels simultaneously. New materials are still needed to construct the composite nanofibrous scaffolds for all needs in RET. Furthermore, the in vivo and clinical evaluation are also critical for the development of new nanofibrous scaffolds.
Author contributions
FH and BR conceived the layout of this manuscript. FH wrote the first draft of the manuscript that was iteratively improved by LC, JL, and BR.
Funding
This research was funded by National Natural Science Foundation of China, grant number: 81870778, 81870759, 82071106, 81991500, 81991501, Applied Basic Research Programs of Sichuan Province, grant number: 2020YJ0227, Technology Innovation R&D Project of Chengdu, grant number: 2022-YF05-01401-SN, and by Science & Technology Department of Sichuan Province, grant number: 2021YFQ0064.
Acknowledgments
Our special thanks go to Jiaojiao Yang and Fangjie Zhou for their help in suggesting the general direction of this manuscript.
Conflict of interest
The authors declare that the research was conducted in the absence of any commercial or financial relationships that could be construed as a potential conflict of interest.
Publisher’s note
All claims expressed in this article are solely those of the authors and do not necessarily represent those of their affiliated organizations, or those of the publisher, the editors and the reviewers. Any product that may be evaluated in this article, or claim that may be made by its manufacturer, is not guaranteed or endorsed by the publisher.
References
Agnihotry, A., Fedorowicz, Z., van Zuuren, E. J., Farman, A. G., and Al-Langawi, J. H. (2016). Antibiotic use for irreversible pulpitis. Cochrane Database Syst. Rev. 2, CD004969. doi:10.1002/14651858.CD004969.pub4
Albuquerque, M. T. P., Ryan, S. J., Münchow, E. A., Kamocka, M. M., Gregory, R. L., Valera, M. C., et al. (2015). Antimicrobial effects of novel triple antibiotic paste-mimic scaffolds on Actinomyces naeslundii biofilm. J. Endod. 41, 1337–1343. doi:10.1016/j.joen.2015.03.005
Albuquerque, M. T. P., Valera, M. C., Nakashima, M., Nör, J. E., and Bottino, M. C. (2014). Tissue-engineering-based strategies for regenerative endodontics. J. Dent. Res. 93, 1222–1231. doi:10.1177/0022034514549809
Almutairi, W., Al-Dahman, Y., Alnassar, F., and Albalawi, O. (2022). Intracanal calcification following regenerative endodontic treatment: A systematic review and meta-analysis. Clin. Oral Investig. 26, 3333–3342. doi:10.1007/s00784-021-04333-5
Andreasen, J. O., Farik, B., and Munksgaard, E. C. (2002). Long-term calcium hydroxide as a root canal dressing may increase risk of root fracture. Dent. Traumatol. 18, 134–137. doi:10.1034/j.1600-9657.2002.00097.x
Arruda, M. E. F., Neves, M. A. S., Diogenes, A., Mdala, I., Guilherme, B. P. S., Siqueira, J. F., et al. (2018). Infection control in teeth with apical periodontitis using a triple antibiotic solution or calcium hydroxide with chlorhexidine: A randomized clinical trial. J. Endod. 44, 1474–1479. doi:10.1016/j.joen.2018.07.001
Arslan, Hakan, Akcay, Merve, Çakir, Mustafa, Gok, Adem, Yasa, B., and Dalli, M. (2015). Comparison of bond strength of self-etch adhesive to pulp chamber dentin after placement of calcium hydroxide and various antibiotic pastes. Acta odontol. Scand. 73, 226–231. doi:10.3109/00016357.2014.992811
Banchs, F., and Trope, M. (2004). Revascularization of immature permanent teeth with apical periodontitis: New treatment protocol? J. Endod. 30, 196–200. doi:10.1097/00004770-200404000-00003
Bjørndal, L., Demant, S., and Dabelsteen, S. (2014). Depth and activity of carious lesions as indicators for the regenerative potential of dental pulp after intervention. J. Endod. 40, S76–S81. doi:10.1016/j.joen.2014.01.016
Bjørndal, L., Simon, S., Tomson, P. L., and Duncan, H. F. (2019). Management of deep caries and the exposed pulp. Int. Endod. J. 52, 949–973. doi:10.1111/iej.13128
Bletsa, A., Berggreen, E., Fristad, I., Tenstad, O., and Wiig, H. (2006). Cytokine signalling in rat pulp interstitial fluid and transcapillary fluid exchange during lipopolysaccharide-induced acute inflammation. J. Physiol. 573, 225–236. doi:10.1113/jphysiol.2006.104711
Bottino, M. C., Kamocki, K., Yassen, G. H., Platt, J. A., Vail, M. M., Ehrlich, Y., et al. (2013). Bioactive nanofibrous scaffolds for regenerative endodontics. J. Dent. Res. 92, 963–969. doi:10.1177/0022034513505770
Chan, E. K. M., Desmeules, M., Cielecki, M., Dabbagh, B., and Ferraz Dos Santos, B. (2017). Longitudinal cohort study of regenerative endodontic treatment for immature necrotic permanent teeth. J. Endod. 43, 395–400. doi:10.1016/j.joen.2016.10.035
Dahlin, R. L., Kasper, F. K., and Mikos, A. G. (2011). Polymeric nanofibers in tissue engineering. Tissue Eng. Part B Rev. 17, 349–364. doi:10.1089/ten.TEB.2011.0238
Dissanayaka, W. L., Hargreaves, K. M., Jin, L., Samaranayake, L. P., and Zhang, C. (2015). The interplay of dental pulp stem cells and endothelial cells in an injectable peptide hydrogel on angiogenesis and pulp regeneration in vivo. Tissue Eng. Part A 21, 550–563. doi:10.1089/ten.TEA.2014.0154
Estefan, B. S., El Batouty, K. M., Nagy, M. M., and Diogenes, A. (2016). Influence of age and apical diameter on the success of endodontic regeneration procedures. J. Endod. 42, 1620–1625. doi:10.1016/j.joen.2016.06.020
Frank, A. L. (1966). Therapy for the divergent pulpless tooth by continued apical formation. J. Am. Dent. Assoc. 72, 87–93. doi:10.14219/jada.archive.1966.0017
Galler, K. M., Cavender, A., Yuwono, V., Dong, H., Shi, S., Schmalz, G., et al. (2008). Self-assembling peptide amphiphile nanofibers as a scaffold for dental stem cells. Tissue Eng. Part A 14, 2051–2058. –2058. doi:10.1089/ten.tea.2007.0413
Galler, K. M., D’Souza, R. N., Hartgerink, J. D., and Schmalz, G. (2011). Scaffolds for dental pulp tissue engineering. Adv. Dent. Res. 23, 333–339. doi:10.1177/0022034511405326
Galler, K. M., Hartgerink, J. D., Cavender, A. C., Schmalz, G., and D’Souza, R. N. (2012). A customized self-assembling peptide hydrogel for dental pulp tissue engineering. Tissue Eng. Part A 18, 176–184. doi:10.1089/ten.TEA.2011.0222
Grigoriou, E., Cantini, M., Dalby, M. J., Petersen, A., and Salmeron-Sanchez, M. (2017). Cell migration on material-driven fibronectin microenvironments. Biomater. Sci. 5, 1326–1333. doi:10.1039/c7bm00333a
Gupte, M. J., and Ma, P. X. (2012). Nanofibrous scaffolds for dental and craniofacial applications. J. Dent. Res. 91, 227–234. doi:10.1177/0022034511417441
Heithersay, G. S. (1975). Calcium hydroxide in the treatment of pulpless teeth with associated pathology. Int. Endod. J. 8, 74–93. doi:10.1111/j.1365-2591.1975.tb01000.x
Huang, G. T.-J., and Lin, L. M. (2008). Letter to the editor: Comments on the use of the term “revascularization” to describe. J. Endod. 34, 511. author reply 511-512. doi:10.1016/j.joen.2008.02.009
Huang, G. T.-J., Sonoyama, W., Liu, Y., Liu, H., Wang, S., and Shi, S. (2008). The hidden treasure in apical papilla: The potential role in pulp/dentin regeneration and bioroot engineering. J. Endod. 34, 645–651. doi:10.1016/j.joen.2008.03.001
Iwaya, S. I., Ikawa, M., and Kubota, M. (2001). Revascularization of an immature permanent tooth with apical periodontitis and sinus tract. Dent. Traumatol. 17, 185–187. doi:10.1034/j.1600-9657.2001.017004185.x
Jadhav, G., Shah, N., and Logani, A. (2012). Revascularization with and without platelet-rich plasma in nonvital, immature, anterior teeth: A pilot clinical study. J. Endod. 38, 1581–1587. doi:10.1016/j.joen.2012.09.010
Jung, C., Kim, S., Sun, T., Cho, Y.-B., and Song, M. (2019). Pulp-dentin regeneration: Current approaches and challenges. J. Tissue Eng. 10, 204173141881926. doi:10.1177/2041731418819263
Kahler, B., and Rossi-Fedele, G. (2016). A review of tooth discoloration after regenerative endodontic therapy. J. Endod. 42, 563–569. doi:10.1016/j.joen.2015.12.022
Kamocki, K., Nör, J. E., and Bottino, M. C. (2015). Dental pulp stem cell responses to novel antibiotic-containing scaffolds for regenerative endodontics. Int. Endod. J. 48, 1147–1156. doi:10.1111/iej.12414
Karczewski, A., Feitosa, S. A., Hamer, E. I., Pankajakshan, D., Gregory, R. L., Spolnik, K. J., et al. (2018). Clindamycin-modified triple antibiotic nanofibers: A stain-free antimicrobial intracanal drug delivery system. J. Endod. 44, 155–162. doi:10.1016/j.joen.2017.08.024
Keller, L., Offner, D., Schwinté, P., Morand, D., Wagner, Q., Gros, C., et al. (2015). Active nanomaterials to meet the challenge of dental pulp regeneration. Mater. (Basel) 8, 7461–7471. doi:10.3390/ma8115387
Kim, G.-H., Park, Y.-D., Lee, S.-Y., El-Fiqi, A., Kim, J.-J., Lee, E.-J., et al. (2015). Odontogenic stimulation of human dental pulp cells with bioactive nanocomposite fiber. J. Biomater. Appl. 29, 854–866. doi:10.1177/0885328214546884
Kim, J.-J., Bae, W.-J., Kim, J.-M., Kim, J.-J., Lee, E.-J., Kim, H.-W., et al. (2014). Mineralized polycaprolactone nanofibrous matrix for odontogenesis of human dental pulp cells. J. Biomater. Appl. 28, 1069–1078. doi:10.1177/0885328213495903
Kim, S. G., Malek, M., Sigurdsson, A., Lin, L. M., and Kahler, B. (2018). Regenerative endodontics: A comprehensive review. Int. Endod. J. 51, 1367–1388. doi:10.1111/iej.12954
Kwon, I. K., Kidoaki, S., and Matsuda, T. (2005). Electrospun nano- to microfiber fabrics made of biodegradable copolyesters: Structural characteristics, mechanical properties and cell adhesion potential. Biomaterials 26, 3929–3939. doi:10.1016/j.biomaterials.2004.10.007
Leite, M. L., Soares, D. G., Anovazzi, G., Mendes Soares, I. P., Hebling, J., and de Souza Costa, C. A. (2021). Development of fibronectin-loaded nanofiber scaffolds for guided pulp tissue regeneration. J. Biomed. Mat. Res. 109, 1244–1258. doi:10.1002/jbm.b.34785
Li, Q.-M., Li, J.-L., Feng, Z.-H., Lin, H.-C., and Xu, Q. (2020). Effect of histone demethylase KDM5A on the odontogenic differentiation of human dental pulp cells. Bioengineered 11, 449–462. doi:10.1080/21655979.2020.1743536
Li, X., Ma, C., Xie, X., Sun, H., and Liu, X. (2016). Pulp regeneration in a full-length human tooth root using a hierarchical nanofibrous microsphere system. Acta Biomater. 35, 57–67. doi:10.1016/j.actbio.2016.02.040
Lim, H.-C., Nam, O. H., Kim, M.-J., El-Fiqi, A., Yun, H.-M., Lee, Y.-M., et al. (2016). Delivery of dexamethasone from bioactive nanofiber matrices stimulates odontogenesis of human dental pulp cells through integrin/BMP/mTOR signaling pathways. Int. J. Nanomedicine 11, 2557–2567. doi:10.2147/IJN.S97846
Lin, L. M., Ricucci, D., Saoud, T. M., Sigurdsson, A., and Kahler, B. (2020). Vital pulp therapy of mature permanent teeth with irreversible pulpitis from the perspective of pulp biology. Aust. Endod. J. 46, 154–166. doi:10.1111/aej.12392
Lin, L. M., Shimizu, E., Gibbs, J. L., Loghin, S., and Ricucci, D. (2014). Histologic and histobacteriologic observations of failed revascularization/revitalization therapy: A case report. J. Endod. 40, 291–295. doi:10.1016/j.joen.2013.08.024
Ma, X., Li, C., Jia, L., Wang, Y., Liu, W., Zhou, X., et al. (2016). Materials for retrograde filling in root canal therapy. Cochrane Database Syst. Rev. 12, CD005517. doi:10.1002/14651858.CD005517.pub2
Manfredi, M., Figini, L., Gagliani, M., and Lodi, G. (2016). Single versus multiple visits for endodontic treatment of permanent teeth. Cochrane Database Syst. Rev. 12, CD005296. doi:10.1002/14651858.CD005296.pub3
Mc, B., Mtp, A., A, A., Ea, M., Kj, S., Je, N., et al. (2019). A novel patient-specific three-dimensional drug delivery construct for regenerative endodontics. J. Biomed. Mat. Res. 107, 1576–1586. doi:10.1002/jbm.b.34250
Morsczeck, C., and Reichert, T. E. (2018). Dental stem cells in tooth regeneration and repair in the future. Expert Opin. Biol. Ther. 18, 187–196. doi:10.1080/14712598.2018.1402004
Murray, P. E., Garcia-Godoy, F., and Hargreaves, K. M. (2007). Regenerative endodontics: A review of current status and a call for action. J. Endod. 33, 377–390. doi:10.1016/j.joen.2006.09.013
Murray, P. E. (2018). Platelet-rich plasma and platelet-rich fibrin can induce apical closure more frequently than blood-clot revascularization for the regeneration of immature permanent teeth: A meta-analysis of clinical efficacy. Front. Bioeng. Biotechnol. 6, 139. doi:10.3389/fbioe.2018.00139
Nagata, J. Y., Soares, A. J., Souza-Filho, F. J., Zaia, A. A., Ferraz, C. C. R., Almeida, J. F. A., et al. (2014). Microbial evaluation of traumatized teeth treated with triple antibiotic paste or calcium hydroxide with 2% chlorhexidine gel in pulp revascularization. J. Endod. 40, 778–783. doi:10.1016/j.joen.2014.01.038
Nakashima, M., and Akamine, A. (2005). The application of tissue engineering to regeneration of pulp and dentin in endodontics. J. Endod. 31, 711–718. doi:10.1097/01.don.0000164138.49923.e5
Neelakantan, P., Romero, M., Vera, J., Daood, U., Khan, A. U., Yan, A., et al. (2017). Biofilms in endodontics-current status and future directions. Int. J. Mol. Sci. 18, E1748. doi:10.3390/ijms18081748
Palasuk, J., Kamocki, K., Hippenmeyer, L., Platt, J. A., Spolnik, K. J., Gregory, R. L., et al. (2014). Bimix antimicrobial scaffolds for regenerative endodontics. J. Endod. 40, 1879–1884. doi:10.1016/j.joen.2014.07.017
Pankajakshan, D., Albuquerque, M. T. P., Evans, J. D., Kamocka, M. M., Gregory, R. L., and Bottino, M. C. (2016). Triple antibiotic polymer nanofibers for intracanal drug delivery: Effects on dual species biofilm and cell function. J. Endod. 42, 1490–1495. doi:10.1016/j.joen.2016.07.019
Porter, M. L. A., Münchow, E. A., Albuquerque, M. T. P., Spolnik, K. J., Hara, A. T., and Bottino, M. C. (2016). Effects of novel 3-dimensional antibiotic-containing electrospun scaffolds on dentin discoloration. J. Endod. 42, 106–112. doi:10.1016/j.joen.2015.09.013
Qu, T., Jing, J., Jiang, Y., Taylor, R. J., Feng, J. Q., Geiger, B., et al. (2014). Magnesium-containing nanostructured hybrid scaffolds for enhanced dentin regeneration. Tissue Eng. Part A 20, 2422–2433. doi:10.1089/ten.TEA.2013.0741
Qu, T., and Liu, X. (2013). Nano-structured gelatin/bioactive glass hybrid scaffolds for the enhancement of odontogenic differentiation of human dental pulp stem cells. J. Mat. Chem. B 1, 4764–4772. doi:10.1039/C3TB21002B
Rafter, M. (2005). Apexification: A review. Dent. Traumatol. 21, 1–8. doi:10.1111/j.1600-9657.2004.00284.x
Ribeiro, J. S., Münchow, E. A., Ferreira Bordini, E. A., de Oliveira da Rosa, W. L., and Bottino, M. C. (2020). Antimicrobial therapeutics in regenerative endodontics: A scoping review. J. Endod. 46, S115–S127. doi:10.1016/j.joen.2020.06.032
Rosa, V., Zhang, Z., Grande, R. H. M., and Nör, J. E. (2013). Dental pulp tissue engineering in full-length human root canals. J. Dent. Res. 92, 970–975. doi:10.1177/0022034513505772
Ruparel, N. B., Teixeira, F. B., Ferraz, C. C. R., and Diogenes, A. (2012). Direct effect of intracanal medicaments on survival of stem cells of the apical papilla. J. Endod. 38, 1372–1375. doi:10.1016/j.joen.2012.06.018
Sampaio-Maia, B., Caldas, I. M., Pereira, M. L., Pérez-Mongiovi, D., and Araujo, R. (2016). The oral microbiome in Health and its implication in oral and systemic diseases. Adv. Appl. Microbiol. 97, 171–210. doi:10.1016/bs.aambs.2016.08.002
Schmalz, G., Widbiller, M., and Galler, K. M. (2020). Clinical perspectives of pulp regeneration. J. Endod. 46, S161–S174. doi:10.1016/j.joen.2020.06.037
Sheikh, Z., Najeeb, S., Khurshid, Z., Verma, V., Rashid, H., and Glogauer, M. (2015). Biodegradable materials for bone repair and tissue engineering applications. Mater. (Basel) 8, 5744–5794. doi:10.3390/ma8095273
Sill, T. J., and von Recum, H. A. (2008). Electrospinning: Applications in drug delivery and tissue engineering. Biomaterials 29, 1989–2006. doi:10.1016/j.biomaterials.2008.01.011
Soares, D. G., Zhang, Z., Mohamed, F., Eyster, T. W., de Souza Costa, C. A., and Ma, P. X. (2018). Simvastatin and nanofibrous poly(l-lactic acid) scaffolds to promote the odontogenic potential of dental pulp cells in an inflammatory environment. Acta Biomater. 68, 190–203. doi:10.1016/j.actbio.2017.12.037
Song, M., Cao, Y., Shin, S.-J., Shon, W.-J., Chugal, N., Kim, R. H., et al. (2017). Revascularization-associated intracanal calcification: Assessment of prevalence and contributing factors. J. Endod. 43, 2025–2033. doi:10.1016/j.joen.2017.06.018
Sun, H., Feng, K., Hu, J., Soker, S., Atala, A., and Ma, P. X. (2010). Osteogenic differentiation of human amniotic fluid-derived stem cells induced by bone morphogenetic protein-7 and enhanced by nanofibrous scaffolds. Biomaterials 31, 1133–1139. doi:10.1016/j.biomaterials.2009.10.030
Sundararaj, S. C., Thomas, M. V., Dziubla, T. D., and Puleo, D. A. (2014). Bioerodible system for sequential release of multiple drugs. Acta Biomater. 10, 115–125. doi:10.1016/j.actbio.2013.09.031
Wang, J., Liu, X., Jin, X., Ma, H., Hu, J., Ni, L., et al. (2010). The odontogenic differentiation of human dental pulp stem cells on nanofibrous poly(L-lactic acid) scaffolds in vitro and in vivo. Acta Biomater. 6, 3856–3863. doi:10.1016/j.actbio.2010.04.009
Yang, F., Murugan, R., Wang, S., and Ramakrishna, S. (2005). Electrospinning of nano/micro scale poly(L-lactic acid) aligned fibers and their potential in neural tissue engineering. Biomaterials 26, 2603–2610. doi:10.1016/j.biomaterials.2004.06.051
Yang, X., Yang, F., Walboomers, X. F., Bian, Z., Fan, M., and Jansen, J. A. (2010). The performance of dental pulp stem cells on nanofibrous PCL/gelatin/nHA scaffolds. J. Biomed. Mat. Res. A 93, 247–257. doi:10.1002/jbm.a.32535
Yoo, Y.-J., Oh, J.-H., Zhang, Q., Lee, W., and Woo, K. M. (2018). Dimethyloxalylglycine-embedded poly(ε-caprolactone) fiber meshes promote odontoblastic differentiation of human dental pulp-derived cells. J. Endod. 44, 98–103.e1. doi:10.1016/j.joen.2017.09.002
Yun, H.-M., Kang, S.-K., Singh, R. K., Lee, J.-H., Lee, H.-H., Park, K.-R., et al. (2016). Magnetic nanofiber scaffold-induced stimulation of odontogenesis and pro-angiogenesis of human dental pulp cells through Wnt/MAPK/NF-κB pathways. Dent. Mat. 32, 1301–1311. doi:10.1016/j.dental.2016.06.016
Zein, N., Harmouch, E., Lutz, J.-C., Fernandez De Grado, G., Kuchler-Bopp, S., Clauss, F., et al. (2019). Polymer-based instructive scaffolds for endodontic regeneration. Mater. (Basel) 12, E2347. doi:10.3390/ma12152347
Zhang, H., Liu, S., Zhou, Y., Tan, J., Che, H., Ning, F., et al. (2012). Natural mineralized scaffolds promote the dentinogenic potential of dental pulp stem cells via the mitogen-activated protein kinase signaling pathway. Tissue Eng. Part A 18, 677–691. doi:10.1089/ten.TEA.2011.0269
Zhang, Q. L., Dong, C. Y., Liu, L., Wen, S. P., and Wang, X. Y. (2019). Effects of electrospun collagen nanofibrous matrix on the biological behavior of human dental pulp cells. Beijing Da Xue Xue Bao Yi Xue Ban. 51, 28–34. doi:10.19723/j.issn.1671-167X.2019.01.006
Zijah, Vahid, Salehi, Roya, Aghazadeh, Marziyeh, Samiei, Mohammad, Alizadeh, Effat, and Davaran, S. (2017a). Towards optimization of odonto/osteogenic bioengineering: In vitro comparison of simvastatin, sodium fluoride, melanocyte-stimulating hormone. Vitro Cell. Dev. Biol. -Animal. 53, 502–512. doi:10.1007/s11626-017-0141-6
Zijah, V., Salehi, R., Aghazadeh, M., Samiei, M., Alizadeh, E., and Davaran, S. (2017b). Towards optimization of odonto/osteogenic bioengineering: In vitro comparison of simvastatin, sodium fluoride, melanocyte-stimulating hormone. Vitro Cell. Dev. Biol. -Animal. 53, 502–512. doi:10.1007/s11626-017-0141-6
Keywords: regenerative endodontics treatment, RET, nanofiber, scaffolds, nanomateials
Citation: Huang F, Cheng L, Li J and Ren B (2022) Nanofibrous scaffolds for regenerative endodontics treatment. Front. Bioeng. Biotechnol. 10:1078453. doi: 10.3389/fbioe.2022.1078453
Received: 24 October 2022; Accepted: 30 November 2022;
Published: 12 December 2022.
Edited by:
Hai Zhang, University of Washington, United StatesReviewed by:
Yongxi Liang, Boston University, United StatesMaobin Yang, Temple University Health System, Inc., United States
Copyright © 2022 Huang, Cheng, Li and Ren. This is an open-access article distributed under the terms of the Creative Commons Attribution License (CC BY). The use, distribution or reproduction in other forums is permitted, provided the original author(s) and the copyright owner(s) are credited and that the original publication in this journal is cited, in accordance with accepted academic practice. No use, distribution or reproduction is permitted which does not comply with these terms.
*Correspondence: Jiyao Li, aml5YW9saXNjdUAxNjMuY29t; Biao Ren, cmVuYmlhb0BzY3UuZWR1LmNu