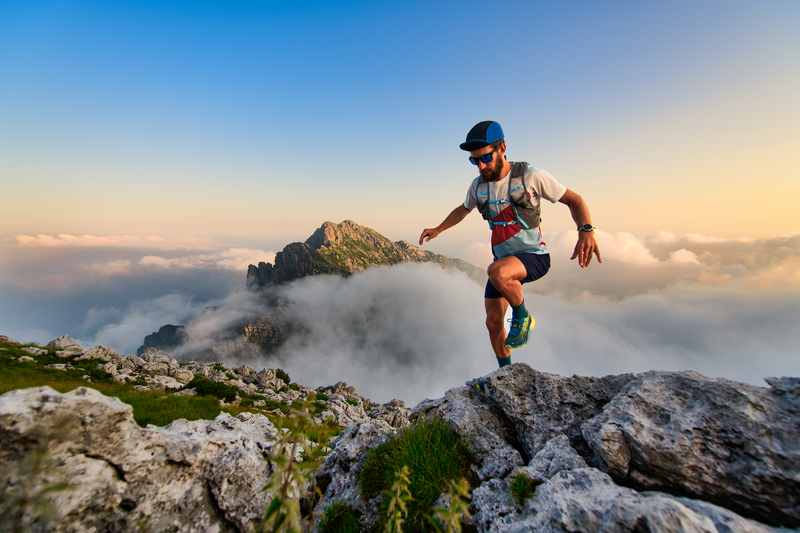
95% of researchers rate our articles as excellent or good
Learn more about the work of our research integrity team to safeguard the quality of each article we publish.
Find out more
REVIEW article
Front. Bioeng. Biotechnol. , 02 December 2022
Sec. Biomaterials
Volume 10 - 2022 | https://doi.org/10.3389/fbioe.2022.1071472
This article is part of the Research Topic Biomaterials for the Repair and Regeneration of Musculoskeletal Tissue View all 8 articles
Objectives: Stem cell-based tissue engineering approaches are promising for bone repair and regeneration. Periodontal ligament stem cells (PDLSCs) are a promising cell source for tissue engineering, especially for maxillofacial bone and periodontal regeneration. Many studies have shown potent results via PDLSCs in bone regeneration. In this review, we describe recent cutting-edge researches on PDLSC-based bone regeneration and periodontal tissue regeneration.
Data and sources: An extensive search of the literature for papers related to PDLSCs-based bioactive constructs for bone tissue engineering was made on the databases of PubMed, Medline and Google Scholar. The papers were selected by three independent calibrated reviewers.
Results: Multiple types of materials and scaffolds have been combined with PDLSCs, involving xeno genic bone graft, calcium phosphate materials and polymers. These PDLSC-based constructs exhibit the potential for bone and periodontal tissue regeneration. In addition, various osteo inductive agents and strategies have been applied with PDLSCs, including drugs, biologics, gene therapy, physical stimulation, scaffold modification, cell sheets and co-culture.
Conclusoin: This review article demonstrates the great potential of PDLSCs-based bioactive constructs as a promising approach for bone and periodontal tissue regeneration.
Bone defects due to congenital malformations, skeletal diseases, trauma, inflammation and tumor resections pose a critical challenge. Auto grafts are deemed as the gold-standard for bone regeneration; however, their application is impeded by harvest limitations and donor-site morbidity. To meet this need, stem cell-based tissue engineering has emerged as a promising option to regenerate bone defect. Research of stem cells has been a fascinating area of interest. Because of the capability of self-renewal and differentiation into multiple cell lineages, stem cells were regarded as powerful roles in regeneration of lost tissues (Avinash et al., 2017). Mesenchymal stem cells (MSCs) have been identified by capabilities of forming adherent fibroblast-like colonies and differentiating into multiple lineages including osteogenesis, adipogenesis and chondrogenesis (Queiroz et al., 2021). MSCs possess immunomodulatory ability, migratory activity and paracrine function on other type of cells (Queiroz et al., 2021).
When isolated from diverse tissues, different types of MSCs demonstrate source-dependent peculiarities. In recent years, many dentally-derived MSCs were found suitable for tissue engineering applications because of their accessibility and multilineage differentiation capacity (Lei et al., 2014). As dentally-derived MSCs are cells originating from migrating neural crest cells, they play a strategic role in dental and craniofacial tissue regeneration (Trubiani et al., 2019).
Periodontal ligament stem cells (PDLSCs) are isolated from periodontal ligaments (PDL). PDLSCs can be harvested from the extracted wisdom teeth, extracted supernumerary teeth and the teeth extracted for orthodontic treatment (Zhao et al., 2021). PDLSCs are a relative easily accessible and low-cost source of stem cells, without extra invasive procedures (like bone marrow aspiration) (Zhao et al., 2021). PDLSCs can differentiate into osteoblasts, chondrocytes, cementoblasts and adipocytes in vitro and regenerate PDL-like tissues in vivo (Qiu et al., 2020). It has been found that, as a kind of MSCs, PDLSCs participate in bone repair via three ways: osteodifferentiation, release of cytokines and extracellular vesicles, and immunomodulatory function (Zha et al., 2022). It has been well known that the effect of tissue regeneration relies on the oxygen and nutrient support supplied by the local vasculature (Yeasmin et al., 2014). Therefore, in the area of tissue engineering and regenerative therapy, angiogenic process has been in the spotlight these years. So far, many studies have demonstrated that PDLSCs have angiogenic properties, definitely, the capacity to support the construction of a functional vasculature (Yeasmin et al., 2014; Zhao et al., 2021; Iwasaki et al., 2021). These investigations highlight the vascular potential of PDLSCs. These advantages make PDLSCs a very attractive cell population for regenerative therapies.
Many pilot studies, pre-clinical studies as well as clinical trials have shown promising results regarding the effectiveness and the safety of PDLSCs in bone regeneration (Queiroz et al., 2021). In this review, we discuss current in vitro and in vivo advances in PDLSC-based bioactive constructs for bone tissue and periodontal tissue regeneration. Furthermore, the effects of osteoinductive strategies on PDLSC differentiation and regeneration capabilities were also discussed.
Currently, the most frequently utilized xenogenic bone grafts are of bovine origin, while grafts derived from porcine bone have shown potential, due to their architectural and compositional similarities to human bone (Bow et al., 2019). PDLSCs showed highly efficient cell proliferation, together with osteogenic differentiation when they were seeded on Bio-Oss scaffold (inorganic deproteinized bovine bone minerals) and Dual Block scaffold (collagenated porcine cortico-cancellous bone scaffold) (Yu et al., 2014; Diomede et al., 2016; Mazzoni et al., 2017). The seeding of PDLSCs can further improve the regenerative capacity of Bio-Oss scaffolds and Dual Block scaffolds (Yu et al., 2014; Diomede et al., 2016; Mazzoni et al., 2017). However, as organic components within the matrix have been removed, these grafts generally lack osteoinductivity (Bow et al., 2019). Therefore, for xenogeneic bone grafts, further investigation is needed to enhance their osteoinductive characteristics, and coupling with bioactive components could be an attractive approach (Musson et al., 2019).
Hydroxyapatite (HA), tricalcium phosphate (TCP), biphasic calcium phosphate (BCP) and calcium phosphate cements (CPC) are also promising for bone regeneration applications. Calcium phosphate materials possess similar chemical characteristics to natural bone minerals (Chen et al., 2013; Mao et al., 2015; Sohn and Oh, 2019). They have been applied in bone tissue regeneration due to their excellent bioactivity and osteoconductivity (Chen et al., 2013; Mao et al., 2015; Sohn and Oh, 2019). PDLSCs adhered and stretched well on HA, TCP and BCP scaffolds, and kept their original osteogenic phenotypes (He et al., 2011; Mao et al., 2015; Su et al., 2015; Shi et al., 2018; Sohn and Oh, 2019). The combination of PDLSCs with HA, TCP and BCP significantly promoted effective bone regeneration (He et al., 2011; Mao et al., 2015; Su et al., 2015; Shi et al., 2018; Sohn and Oh, 2019). One CPC consisted of tetracalcium phosphate and dicalcium phosphate anhydrous (Zhou et al., 2012). The injectability and body-temperature reactions are important characteristics of CPC, which facilitates its ability to mold and fill the bone defect (Sohn and Oh, 2019). CPC can bond to bone tissues to form a functional interface (Chen et al., 2013). Zhao et al. (2019b) seeded PDLSCs on CPC scaffolds, demonstrating that CPC supported PDLSCs attachment and proliferation (Figure 1). Chitosan can be used as a viscous binder to rendered CPC fast-setting and increase the load-bearing capability (Wang et al., 2016). CPC scaffold containing chitosan is an effective delivery vehicle for drugs and proteins (Zhao et al., 2019b; Zhao et al., 2019c). Qin et al. (2018) and Qiu et al. (2021) revealed that CPC-chitosan scaffolds can sustainably release small molecular drug and growth factors. Metformin (a small molecular glucose lowering drug) and platelet lysate (HPL) carried by CPC-chitosan scaffold can be sustainably released for 15–21 days (Qin et al., 2018; Qiu et al., 2021). Metformin and HPL released by CPC increased the osteogenesis of PDLSCs seeded on CPC scaffolds (Qin et al., 2018; Zhao et al., 2019c).
FIGURE 1. Typical SEM images of CPC scaffolds cultured with PDLSCs for 14 days. The image in (B) is a higher magnification of the green dotted frame in (A). Yellow arrows indicate a healthy cell spreading morphology.
To further increase the bone repair capability of CPC, alginate hydrogel was introduced into CPC scaffolds for cell delivery (Wang et al., 2016). The alginate hydrogel can encapsulate cells and protect them during the CPC mixing and setting reaction (Zhao et al., 2010). Moreover, after the CPC has set, the alginate hydrogel would degrade and release the seed cells and create macropores throughout the entire CPC scaffold (Zhao et al., 2010). Chen et al. (2020a) developed an injectable CPC-chitosan scaffold with PDLSCs in alginate microbeads. CPC-alginate-PDLSCs construct exhibited excellent injectability and mechanical strength (Chen et al., 2020). The released PDLSCs were able to adhere to the surfaces of CPC scaffolds and proliferate well (Chen et al., 2020) (Figure 2).
FIGURE 2. Live/dead images of PDLSCs encapsulated in alginate microbeads in CPC-chitosan scaffolds (A–L). Live cells (green) were numerous and dead cells (red) were very few. Percentages of live cells (M) and CCK-8 from 1 to 14 days showed good cell viability and proliferation, and cell viability (N). Values with dissimilar letters are significantly different from each other (p < 0.05).
Polymeric materials include natural polymers and synthetic polymers. Natural polymers such as collagen, chitosan, gelatin, fibrin, zein and alginate have been used in tissue engineering (Zhou et al., 2012; Chen et al., 2016; E et al., 2016; Fu et al., 2016; Kammerer et al., 2017; Ou et al., 2019; Arpornmaeklong et al., 2021; Tian et al., 2021). As these natural polymers can be fabricated as hydrogels, they would mimic the chemical and physical properties of natural extracellular matrix (ECM) to enhance the osteodifferentiation of the seed cells and promote bone regeneration (Chen et al., 2016; Wang et al., 2016; Thanasrisuebwong et al., 2020; Arpornmaeklong et al., 2021; Tian et al., 2021). When seeded with PDLSCs, these natural polymers showed excellent biocompatibility, and supported the proliferation and growth of the PDLSCs (Chen et al., 2016; E et al., 2016; Fu et al., 2016; Kammerer et al., 2017; Ou et al., 2019; Arpornmaeklong et al., 2021; Tian et al., 2021). Specific applications in bone tissue regeneration require certain modifications to the polymer structure (Bharadwaz and Jayasuriya, 2020). This is quite difficult for natural polymers, and hence synthetic polymers such as PLA [poly (lactic acid)], PLGA [poly (lactide-co-glycolide)] and polycaprolactone (PCL) offer excellent applicability in bone regeneration (Alizadeh-Osgouei et al., 2019; Zhao et al., 2020; Ding et al., 2021). However, many polymeric materials suffer from the problems of weak mechanical strength (Tian et al., 2021). Moreover, some synthetic polymers, such as PLA, suffer from shortcomings including low degradation rate, low cell adhesion and inflammatory reactions due to their degradation product lactic acid (Tajbakhsh and Hajiali, 2017).
Blending of HA or nanoHA with polymers can enhance the mechanical strength and cell reaction of polymer materials (Tian et al., 2021). It was reported that sodium alginate/gelatin/nanoHA, zein/gelatin/nanoHA, collagen/nanoHA, PLA/HA displayed high potential as a tissue engineering material for bone defect reconstruction when seeded with PDLSCs (He et al., 2011; E et al., 2016; Fu et al., 2016; Ou et al., 2019; Tian et al., 2021).
In recent years, drug repurposing has become a hot pot in bone tissue engineering. Drug repurposing is concerned with the identifying of new pharmacological indications of existing drugs and their application in the treatment of diseases except for the drug’s proposed therapeutic use (Divya et al., 2021). Aspirin (non-steroidal anti-inflammatory drug) (Abd Rahman et al., 2016), metformin (hypoglycemic drug) (Zhao et al., 2019b; Jia et al., 2020), simvastatin (cholesterol-lowering drug) (Zhao and Liu, 2014; Zhao et al., 2020) and bisphosphonates (osteoporosis drug) (Zhou et al., 2011) are all Food and Drug Administration approved drugs. These drugs were all identified to induce the osteogenic differentiation of PDLSCs (Zhao and Liu, 2014; Abd Rahman et al., 2016; Zhao et al., 2019b; Di Vito et al., 2020; Jia et al., 2020; Zhao et al., 2020). Many herbals of traditional Chinese medicine (TCM) were also widely acknowledged in drug discovery and development due to their function of promoting cell proliferation and modulating bone metabolism (Liu et al., 2018). So far, multiple of active components derived from natural herbs have been discovered to have positive effects on osteogenesis, such as osthole (Gao et al., 2013; Sun et al., 2017), zanthoxylum schinifolium (Kim et al., 2015), berberine (Liu et al., 2018), naringin (Wei et al., 2017) and ginsenoside Rg-1 (Yin et al., 2015). These components of herbs were identified to enhance the proliferation or osteogenic differentiation of PDLSCs, and they have the potential to be used as a mediator or therapeutic agent in PDLSC-based bone tissue engineering (Gao et al., 2013; Kim et al., 2015; Yin et al., 2015; Sun et al., 2017; Wei et al., 2017; Liu et al., 2018). Nevertheless, functions of many drugs are complicated and need to be explored further. Furthermore, the mechanisms of many herbal-drug and their derivatives are still not well understood, which hampers their clinical application in bone regeneration.
Vitamins and growth factors are both essential biologics that skeleton requires (Myneni and Mezey, 2017; Atarbashi-Moghadam et al., 2022). Vitamins, like Vitamin C, Vitamin D and Vitamin P, have been proven to improve the osteogenic properties of PDLSCs, including promoting proliferation, increasing ECM synthesis, enhancing expression of osteogenic markers or reducing inflammation (Wei et al., 2012; Zhao et al., 2019; Qi et al., 2021). However, both excessive and insufficient usage of vitamins may be associated with compromised bone formation (Ahmadieh and Arabi, 2011). Further investigations are still needed on the proper usage methods of vitamins in PDLSCs-based bone regeneration. Growth factors have been proven to have positive effects on the osteogenesis of PDLSCs, including stromal cell-derived factor (SDF) (Liang et al., 2021), transforming growth factor (TGF) (Maeda et al., 2013; Hyun et al., 2017; Huang et al., 2021), bone morphogenic protein (BMP) (Hyun et al., 2017), vascular endothelial growth factor (VEGF) (Lee et al., 2012), fibroblast growth factor (FGF) (Lee et al., 2012; Lee et al., 2015), progranulin (PGRN) (Chen et al., 2020; Yu et al., 2021), estrogen (E et al., 2016), oxysterol (Lee et al., 2017) and erythropoietin (EPO) (Zheng et al., 2019). Further studies in this field should be dedicated to determining the appropriate dosage and timing for the addition of growth factors to different cell culture environments (Hyun et al., 2017).
Bone regeneration is a complicated process which involves a variety of biologics (Zhao et al., 2019c). Several animal-derived reagents were applied in bone regeneration, including platelet-rich plasma (PRP), HPL, enamel matrix derivative (EMD) and exosomes. PRP and HPL contain a cocktail of growth factors, like FGF, VEGF, TGF, platelet-derived growth factor (PDGF) and insulin-like growth factor (IGF) (Zhao et al., 2019c). Xu et al. (2017) reported that PRP at a concentration of 1% was found to improve osteogenic differentiation, cell sheet formation and ECM production of human PDLSCs. Zhao et al. (2019c) reported that CPC-chitosan scaffold was a promising vehicle for HPL delivery, and HPL exerted excellent induction on PDLSCs for bone regeneration (Figures 3, 4). Enamel matrix derivative (EMD) is an acidic extract of extracellular enamel matrix (Tanimoto et al., 2012). Several previous studies demonstrated the effect of EMD on the osteogenesis including the induction of multiple bone markers, such as bone sialoprotein (BSP), osteocalcin (OCN) and alkaline phosphatase (ALP) (Tanimoto et al., 2012). Wang et al. (2016b) revealed that EMD-enhanced PDLSC sheets secreted richer ECM, and induced higher mRNA expression of osteogenic genes than the normal PDLSC sheets. Exosomes are extracellular vesicles with diameters that ranged from 30 nm to 120 nm (Petho et al., 2018). These lipid bilayer-enclosed vesicles transfer proteins, lipids, and noncoding RNAs (Al-Sowayan et al., 2020). The findings of Wang et al. (2020) indicated that the exosomes from human exfoliated deciduous teeth promoted PDLSCs osteodifferentiation and mineralization via Wnt/β-catenin and BMP/Smad signaling pathways. As PRP, PL, EMD and exosomes contain a multitude of molecules, further study is needed to clarify which specific contents are responsible for the observed osteoinductive effects (Albanese et al., 2013; Miron et al., 2014; Altaie et al., 2016; Wang and Thomsen, 2021).
FIGURE 3. Representative alizarin red staining images of bone mineral synthesis by PDLSCs on CPC. The red staining for the HPL groups was deeper and denser that without HPL at 7 and 14 days, as the mineralization was enhanced by incorporating PL into CPC.
FIGURE 4. PDLSC synthesis of bone minerals. The HPL groups accumulated more minerals than that at 0% HPL. Values with dissimilar letters are significantly different from each other (p < 0.05).
Gene modulation is a highly promising approach to promote bone regeneration. An abundance of previous data revealed that gene therapy could be applied to successfully help repair bone defects (Shapiro et al., 2018). By gene therapy, a variety of therapeutic genes can be delivered to stimulate bone regeneration (Lu et al., 2013). Distinct from direct protein delivery (such as growth factors), genes delivery supplies a potential means to enable protein expression for a longer time and temporal modulation of the transgene expression (Lu et al., 2013). Gu et al. (2020) used the green fluorescence protein lentivirus infection system to overexpress TAZ (a key regulator of osteogenesis) in PDLSCs to promote their osteogenesis. In the study of Jung et al. (2014), the osteogenesis of PDLSCs were effectively enhanced after being transduced using replication deficient recombinant adenovirus (rAd) encoding BMP-2. These findings indicate that gene delivery represented a novel and promising strategy for promoting the PDLSCs-based bone tissue engineering.
Besides genetic transformation to express particular protein-coding genes, one of the recent promising gene therapies to be investigated for bone tissue engineering is the use of regulatory noncoding RNAs (ncRNAs). Regulatory ncRNAs include microRNAs (miRNAs), long noncoding RNAs (lncRNAs), and circular RNAs (circRNAs). These regulatory ncRNAs can regulate DNA transcription and translation (Liu et al., 2019).
The miRNAs regulate many biological processes, including cell differentiation, proliferation, angiogenesis, and apoptosis (Pizzicannella et al., 2018). Currently, several miRNAs have been identified to induce the osteogenic ability of PDLSCs, including miRNA-214 (Cao et al., 2017) and miRNA-210 (Pizzicannella et al., 2018). Cao et al. (Cao et al., 2017) revealed that miRNA-214 promoted PDLSCs osteoblastic differentiation by regulating the Wnt/β-catenin signaling. Pizzicannella et al. (Pizzicannella et al., 2018) found that miRNA-210 mediated VEGF upregulation in PDLSCs.
Previous studies supported the possibility that lncRNA and circRNA may act as competing endogenous RNAs for miRNAs to regulate their targeting gene expression in the physiological and pathophysiological process of MSCs (Gu et al., 2017; Wu et al., 2020). For instance, Jia et al. (2015) promoted osteogenic differentiation of PDLSCs via down regulating anti-differentiation ncRNA, a lncRNA that keeps MSCs remain an undifferentiated cell state. Wu et al. (2020) found that overexpression of lncRNA-TUG1 can accelerate the osteogenic differentiation of PDLSCs through sponging miRNA-222-3p to negatively regulate Smad2/7 signaling. Gu et al. (2017) reported that circRNA-BANP and circRNA-ITCH were predicted to interplay with miRNA-34a and miRNA-146a to modulate PDLSC osteogenesis via the MAPK signaling pathway. More research is needed to investigate the safety of gene therapy, especially regarding biodistribution, toxicity, and tumorigenicity (Shapiro et al., 2018).
Cell sheet technique was based on culturing cells in hyperconfluency until they form extensive cell interactions and produce ECM (Yorukoglu et al., 2017). Cell sheets have a high cell density and a uniform cell distribution and thus can closely mimic native tissue (Lu et al., 2019). PDLSC sheet has been used clinically for bone regeneration and a few clinical studies revealed that patients treated with PDLSC sheets exhibited significant bone repairing in the alveolar bone (Zhang et al., 2021). However, as cell sheets possess weak mechanical strength, rebuilding bone tissue with cell sheets alone still remains a challenge (Lu et al., 2019). Thus, many studies have investigated the bone regeneration by combining cell sheets with scaffolds, which could supply initial mechanical strength and spatial integrity (Lu et al., 2019).
Multiple types of scaffolds have shown the potential of its therapeutic use with PDLSC sheets to improve bone repair, like HA-TCP (Gao et al., 2013), collagen (Safi et al., 2019), fibrin (Wang et al., 2016) and PCL (Zhao et al., 2020). However, the main limitation of cell sheet-based tissue engineering is the possible necrosis inside the cell sheet due to the insufficient nutrient and oxygen supply and the poor exchange of cell waste (Lu et al., 2019). Therefore, recent works focus mainly on the vascularization of cell sheet engineering (Yorukoglu et al., 2017). Currently, the constructed cell sheets usually involved a large number of cells while with a relatively small proportion of ECM, which are quite different from native tissues and is a challenge requiring further investigation (Lu et al., 2019).
Angiogenesis is vital for a successful therapeutic outcome in bone regeneration (Chen et al., 2018). Scaffold prevascularization is one of potential ways to increase the supply of inadequate oxygen and nutrition in implantation area (Chen et al., 2018). Endothelial cells (ECs) are main cellular component of the capillary walls, but ECs alone can only form incipient microvascular structures that resemble early capillaries (Liu et al., 2017). While vascular structures derived from co-cultured MSCs and ECs were proved to be stable (Liu et al., 2017). MSCs can secrete angiogenic growth factors (like VEGF and FGF) to induce the angiogenesis of ECs and form a pericyte-like coverage around endothelial tubes, which enables the immature vessels to remain stable (Zhao et al., 2021). Thus, co-culturing of MSCs and ECs is promising to achieve the prevascularization of scaffolds.
PDLSCs had vascular potential and were able to initiate in vitro angiogenesis of ECs (Yeasmin et al., 2014). Zhao et al. (2021b) co-cultured PDLSCs and ECs on CPC scaffolds and successfully formed microvascular-like structures (Figure 5). Their study indicated that the PDLSC-EC co-culture had better angiogenesis than monoculture (Zhao et al., 2021) (Figure 6). This indicated that CPC scaffolds prevacularization via PDLSC-EC are promising for enhancing bone tissue repairing. However, vessel network maturation and graft-host vessel anastomosis still remain the most critical challenges facing scaffold prevascularization, and further studies are needed to investigate PDLSC-based angiogenesis in animal models.
FIGURE 5. Representative SEM images of CPC scaffolds at 21 days. (B) Is a higher magnification image of the blue dotted frame in (A). Capillary-like structures can be observed on the surface of CPC scaffolds (yellow arrows), and some branch-like stretches were found.
FIGURE 6. Images of CD31 immunostaining and quantification of vessel length/junctions. Human umbilical vein endothelial cells (hUVECs) were identified by immunostaining with CD31 in green on the cell membrane, and the nuclei were stained with DAPI in blue. PDLSCs were stained by nuclei counterstaining with DAPI in blue but without green stains on the cell membrane. For hUVEC group, no capillary-like structure can be found after culturing 14 days. (A,C) Co-culture group formed capillary-like structures after culturing 14 days. (B,D) Additionally, the vessel length and vessel junction number of co-culture group increased with time. (E,F) ***On the top of indicated statistical significance between groups (p < 0.001).
Physical therapies have been popular in bone repair yield for many years due to its safety, non-invasiveness, economic benefits, easy access and controllability (Liu et al., 2022). Previous studies have shown that various kinds of physical stimuli contribute to osteogenic differentiation of PDLSCs, including mechanical stimulation, ultrasound stimuli and light stimuli (Zhang et al., 2012; Zhang et al., 2016; Li et al., 2021; Liu et al., 2022). Zhang et al. (2016) treated PDLSCs with static stress and found that exposing PDLSCs to hydraulic pressure enhanced their osteodifferentiation via regulating RANKL/OPG ratio by the Wnt/β-catenin pathway. Zhang et al. (2012) observed an increase in PDLSCs markers of osteogenesis after low-magnitude, high-frequency mechanical vibration stimulation. According to Li et al. (2021), low-intensity pulsed ultrasound can increase the ECM synthesis, osteogenic differentiation-related genes and proteins of PDLSCs that seeded on HA scaffolds. Yamauchi et al. (2018) revealed that 650-nm high-power red light-emitting diode increased PDLSCs proliferation, and osteogenic differentiation and mineralization by activating ERK1/2 signaling pathway. However, so far, the effect of most physical stimulation on PDLSCs is still at the preclinical stage. Further clinical trials about these physical stimulations are needed to test the optimal experimental conditions, including stimulus intensity, duration, and application frequency (Liu et al., 2022).
Surface topography on biomaterial scaffolds played an important role in regulating cell attachment, proliferation, differentiation and gene expression (Mao et al., 2015). Surface modification with micro/nano structures have been identified to be an effective method to further increase the regulating cell differentiation and cellular responses of scaffolds (Mao et al., 2015; Fu et al., 2016; Tansriratanawong et al., 2018; Daghrery et al., 2021). Daghrery et al. (2021) found that nanostructured fluorinated calcium phosphate coatings on PCL scaffolds upregulated osteogenic genes of seeded PDLSCs. Mao et al. (2015) fabricated HA bioceramics with micro-nano-hybrid surface (mnHA [the hybrid of nanorods and microrods]). Their data indicated that mnHA enhanced the cell attachment, spreading, proliferation, ALP activity and cementogenic differentiation of PDLSCs (Mao et al., 2015). Tansriratanawong et al. (2018) fabricated a novel form of calcium hydrogen phosphate with a HA-like surface (CHP-HA). PDLSCs cultivated with this novel CHP-HA show better attachment, proliferation and osteogenic differentiation than CHP alone (Tansriratanawong et al., 2018). Further research is needed to demonstrate the mechanisms of fate changes induced by nanosurface properties as well as the in vivo bone regeneration potential of PDLSC-based scaffolds.
Calvarial defect models are frequently used in bone regeneration research (Bigham-Sadegh and Oryan, 2015). Allogenic grafts, calcium phosphatase scaffolds and polymers have been combined with PDLSCs to repair calvarial defects in animals (Ge et al., 2012; Diomede et al., 2016; Yi et al., 2016; Ou et al., 2019). Diomede et al. (2016) suggested that in vivo implantation of PDLSCs-based porcine cortico-cancellous construct in the calvaria evidenced a precocious osteointegration and vascularization process. The presence of PDLSCs could potentiate the regenerative performance of the scaffolds (Diomede et al., 2016). Chitosan/nanoHA scaffold and Zein/gelatin/nanoHA scaffold seeded with PDLSCs showed more new bone formation than the scaffold groups without PDLSCs in a calvarial defect model (Ge et al., 2012; Ou et al., 2019). However, Yi et al. (2016)’s findings suggested that the in vivo bone regenerative potential of PDLSCs-based BCP could be compromised in a critical-size rat calvarial bone defect model. They indicated that the inhibitory regulation of the osteogenic effect possibly caused by soluble factors released from PDLSCs, such as chordin and PDL-associated protein-1 (Yi et al., 2016).
As PDLSCs can actively differentiate into osteoblast-like cells, cementoblasts and fibroblasts, many researchers investigated the effectiveness of PDLSC-based grafts in regenerating alveolar bone and periodontal tissue (Trubiani et al., 2019). It was reported that PDLSC-seeded bovine-derived bone mineral, β-TCP, BCP, gelatin sponge and PLA/PLGA formed more bone formation than groups without scaffolds or cells after implanted into alveolar bone defect (Han et al., 2014; Su et al., 2015; Chen et al., 2016; Shi et al., 2018; Ding et al., 2020; Ding et al., 2021). Furthermore, these PDLSCs-based scaffolds facilitated the regeneration of the periodontal ligament and cementum tissue in vivo (Han et al., 2014; Su et al., 2015; Shi et al., 2018; Ding et al., 2020; Ding et al., 2021). In periodontal tissue regeneration, challenges remain in regenerating bone-PDL-cementum complex (Liu et al., 2019). The oriented PDL would need to be inserted into newly formed cementum-like tissue and the alveolar bone, which is especially difficult to achieve in the laboratory (Liu et al., 2019).
Tomography properties of scaffolds effect on periodontium regeneration (Lee et al., 2014). Gao et al. (2015) found that titania nanotubes (NTs) layered on titanium/HA scaffolds enhanced the periodontal regeneration of PDLSCs, leading to the formation of collagen fiber bundles. It indicates that the effect of nanotopographical cues can influence the functions of PDLSCs-based periodontal regeneration (Gao et al., 2015). Topographical cell guidance facilitates the geometric design of composite materials (Yang et al., 2018). It has been utilized as a tissue bionic technique in periodontal regeneration (Yang et al., 2018). Yang et al. (2018) manufactured multilayered scaffolds by cementing aligned PCL nanofibers together with gelatin (Figure 7). The scaffold mimicked the natural structure of periodontal ligaments (Yang et al., 2018). This scaffold could provide good attachment for PDLSCs (Yang et al., 2018). Yang et al. (2018) evaluated the angular distribution of regenerated PDL-like tissue by the arrangement of nuclear and cell shapes against the root surfaces, and compared it with that of physiological periodontal tissue. The in vivo results demonstrated that construct seeded with PDLSCs could oriented neogenesis of periodontium and facilitate collagen formation and maturation at periodontal fenestration defects (Yang et al., 2018) (Figure 8).
FIGURE 7. SEM photographs of 2D and 3D scaffold. (A) Surface topographies of aligned (AL) nanofibre. (B) Surface topographies of random (RD) nanofibre. (C) Transversal image of 3D scaffold.
FIGURE 8. Evaluation of arrangement of regenerated ligament tissue. (A) Hematoxylin and eosin (H and E) staining of 3D-AL 3D-RD and blank control group at 3 and 6 weeks. The images on the right are higher magnifications of images in the black frames on the left. Black lines indicated well aligned regenerated PDL-like tissue. Black triangle represented random and irregular ligament orientation. “D” denoted “dentin,” “B” denoted “bone,” “C” denoted “cementum.” (B) Statistical analysis of angular distribution of regenerated PDL-like tissue in different groups. *On the top of indicated statistical significance between groups (p < 0.05). # On the top suggested statistical difference (p < 0.01) in comparison to natural PDL.
The favorable regenerative microenvironment was essential for inducing PDLSCs migration, differentiation, proliferation in periodontal tissue regeneration (Zhu et al., 2017). An optimal combination of suitable growth factors/cytokines and the sequential release at the right time could favor periodontal tissue regeneration (Ding et al., 2020). Cho et al. (Cho et al., 2016) used 3D-printing to fabricate PCL/PLGA scaffolds encapsulating bone morphogenetic protein-7 (BMP-7). Then the scaffolds were cultured with PDLSCs and placed on the exposed dentin (Cho et al., 2016). The results indicated that scaffolds can constantly release BMP-7 and show promise for the ability for guiding PDL and alveolar bone regeneration (Cho et al., 2016). Ding et al. developed a fibrous framework with a PLA/PLGA core/shell structure with rapid release of basic fibroblast growth factor (bFGF) and the relatively slower and sustained release of BMP-2 (Ding et al., 2020). PLA was used as the core material because of its longer degradation time, while PLGA was designed as the shell material due to its faster degradation rate (Ding et al., 2020). This sequential delivery system could stimulate the recruitment of PDLSCs, which could not only achieve bone regeneration but also restore the cementum and PDL to their native structures (Ding et al., 2020).
The cross-talk between PDLSCs and other types of cells likely affect the outcome of PDLSC-based periodontal regeneration by regulating cell growth and differentiation (Yu et al., 2017). These interactions may exist through secreted biologics or/and direct cell-to-cell contact (Yu et al., 2017). Osteoblast progenitors (Yu et al., 2017), jawbone-derived MSCs (Zhu et al., 2017) and apical tooth germ cells (Yang et al., 2009) were all found to promote the periodontium regeneration of PDLSCs. PDLSCs sheets offers advantages by secreting ECM, which provides a wide range of biochemical and mechanical cues to the cells and acts as a reservoir for many signal molecules that are critical in periodontal regeneration (Guo et al., 2014).
3D PDLSC pellets were formed by tightly condensed PDLSC sheets (Guo et al., 2014). The collagen fibers in 3D PDLSC pellets increased logistically and oriented more regularly, thus making it less resistant to stretch (Guo et al., 2014). 3D multilayered PDLSC pellet was capable to reconstruct the physiological architecture of the cementum/periodontal ligament complex (Guo et al., 2014).
Ectopic bone formation refers to the ossification of tissues outside their usual origins (Scott et al., 2012). Ectopic bone formation has unique advantages over orthotopic environments, including a relative lack of bone cytokine stimulation and cell-to-cell interaction with host bone-forming cells (Scott et al., 2012). Subcutaneous implantation is the most commonly used ectopic bone formation method in bone tissue engineering (Scott et al., 2012). Many types of scaffolds have been seeded with PDLSCs and transplanted subcutaneously, including polymers, calcium phosphate scaffolds and xenografts (He et al., 2011; Moshaverinia et al., 2013; Yu et al., 2014; Chen et al., 2016; E et al., 2016; Wang et al., 2016). PDLSCs were suitable seed cells for ectopic bone regeneration in the context of gelatin methacrylate/nanoHA, collagen/PLA/nanoHA, PLA/nanoHA, TCP/HA, alginate and anorganic deproteinized bovine bone minerals, and constructs promoted better bone regeneration than scaffold alone (He et al., 2011; Moshaverinia et al., 2013; Yu et al., 2014; Chen et al., 2016; E et al., 2016; Wang et al., 2016).
There is preclinical evidence that the use of PDLSC-based scaffolds improves osteogenesis when treating bone defects. However, some studies also demonstrated that the complexity of osteoinductive function of PDLSCs depend on different in vivo tissue environment (Yi et al., 2016). For example, Yi et al. (2016) found that PDLSCs promoted BMP-2-induced osteogenesis in subcutaneous transplantation but negatively regulated bone formation in a critical size bone defect. Thus, more extensive investigations in a variety of pathophysiological conditions are required (Yi et al., 2016). In addition, there is limited clinical evidence that these PDLSC-based scaffolds can be used safely and effectively to repair bone defects in clinical use. Further investigation is needed to acknowledge the in vivo regenerative properties of PDLSCs and to make their clinical use for bone tissue engineering.
In this paper, the summarized literature, which involves scaffolds for PDLSC-based bone tissue engineering, has been reviewed. The strategies and agents used to induce PDLSC osteogenesis have also been elaborated.
The purpose of using scaffold is to mimic the structure and function of ECM, which can provide a 3D environment to enhance the attachment, population, and differentiation of cells and to supply proper biological and physical condition for bone repair (Porter et al., 2009; Qu et al., 2019). Scaffolds for delivering PDLSCs were shown in Table 1. Xenogenic bone grafts and calcium phosphate materials are widely used as bioactive biomaterials due to their similarity to the inorganic component of natural bone, excellent biological reactions to cells, and well osteoconductivity (Samavedi et al., 2013). However, much work remains to be done in further enhancing their degradability and osteoinductivity (Samavedi et al., 2013). Different from calcium phosphate materials, natural polymers are extensively used in bone regeneration due to their capability to mimic ECM (Bharadwaz and Jayasuriya, 2020). They have specific degradation rates and superior biological properties (Bharadwaz and Jayasuriya, 2020). Nevertheless, most natural polymers have weak mechanical strength (Tian et al., 2021). In addition, it is quite difficult to do certain modifications with natural polymers for specific applications in bone tissue regeneration (Bharadwaz and Jayasuriya, 2020). While synthetic polymers are much easier to be modified (Bharadwaz and Jayasuriya, 2020). But many synthetic polymers suffer from the problems including low cell adhesion and inflammatory reactions (Tajbakhsh and Hajiali, 2017). Fabricating an ideal scaffold that simultaneously possess strong mechanical characteristics, interconnected porosis, well osseointegration, vascularization, controlled biodegradability and remodeling ability is still a challenge (Zhao et al., 2021). Composite biomaterials that designed to combine two or more materials might be a promising approach to increase the performance of these scaffolds (Samavedi et al., 2013).
To further increase the osteogenesis of PDLSC-based bone repair, multiple strategies and agents were applied. In bone tissue engineering, three factors are essential: ideal microenvironment, appropriate scaffolds, and viable cell populations (Zha et al., 2022). Effects of drugs and bioactive agents on PDLSCs were shown in Table 2. Strategies of osteoinductive strategies on PDLSCs were shown in Table 3.
Osteoinductive drugs/bioactive molecules/reagent were applied in the living microenvironment of PDLSCs to further enhance osteogenesis effect. These additives were immediately mixed with medium or loaded by scaffolds. Further studies in this field need to set insight on the dosage, addition time, mechanisms and possible side effects. In addition, delivery systems with an ability to locally control over spatial distribution and sustained release of these drugs or biological agents also need to be investigated (Porter et al., 2009; Oliveira et al., 2021). Physician stimulation is a low cost and noninvasive therapy that can change cell microenvironment (Liu et al., 2022). However, due to the complexity of the interaction between physical agents and biological systems, a lot work is still needed to find out the specific mechanisms (Massari et al., 2019). Furthermore, great care has to be given to the design of dedicated experimental set-ups, in which the stimulation contributions can be controlled (Padilla et al., 2014).
As interface plays a significant role in osseointegration of implanted scaffolds, surface modification can effectively enhance the osteogenesis (Walmsley et al., 2015). Nanoparticle modifications of scaffolds enhance their capacity to mimic complex properties of the natural bone and provide a more favorable milieu for cellular adherent, migration, and bone regeneration (Walmsley et al., 2015). While further in-depth structural and functional studies are required to understand the underlying mechanisms for cell responses to different surface topographies (Gui et al., 2018).
Several strategies focused on the tissue formation capability of PDLSCs, including cell sheets and scaffold vascularization. As the cellular attachment proteins and extracellular matrix were kept, PDLSC sheet yielded greater bone regeneration than single PDLSCs (Li et al., 2019). However, so far, a single cell sheet still cannot sufficiently regenerate a large-scale bone defect (Li et al., 2019). In addition, possible necrosis and insufficient ECM are still the main problems of PDLSC sheet (Yorukoglu et al., 2017; Lu et al., 2019). Scaffold prevascularization can enhance bone regeneration via increasing oxygen and nutrition supply and helping remove waste (Zhao et al., 2021). PDLSCs showed great vascular potential when they were co-cultured with ECs (Zhao et al., 2021). However, further investigation about vessel network maturation and graft-host vessel anastomosis are still needed. Gene therapy is a breaking new technology with the aim of regenerating tissues by acting as a delivery system for therapeutic genes (Fliefel et al., 2017). But functional investigation is necessary to find out both safety and efficacy prior to its future clinical application.
PDLSC-based constructs showed potential effect on the in vivo tissue regeneration of calvarial bone, alveolar bone and ectopic bone. Moreover, PDLSC-based constructs also demonstrated excellent capacity of periodontal tissue repair. This indicated that PDLSC-based construct is promising as a tissue engineering scaffold for bone regeneration, especially the repair of craniofacial bone. However, bone is a dynamic organ, which interacts with the immune system and vascular system, future research needs to focus not only on osteogenic effect, but also on immune reaction and vascular (Madsen et al., 2020). Additionally, future in vivo study design would center on the performance of PDLSC-based constructs in various pathophysiological environments in order to achieve optimal effects.
PDLSCs are a relatively new, readily accessible, and highly promising MSC source for bone and dental tissue engineering. PDLSCs showed higher growth potential than borrow mesenchymal stem cells (BMSCs). For example, BMSCs stopped proliferation at approximately 50 population doublings, while PDLSCs maintained the proliferative capacity even beyond 100 population doublings (Tomokiyo et al., 2019). In delivering PDLSCs, scaffolds including calcium phosphates and polymers exhibited excellent osteobiological properties for bone grafts. PDLSC-based bioactive constructs showed great potential in regenerating calvarial bone, alveolar bone, ectopic bone and the periodontal complex. Several novel approaches for PDLSCs-based bone regeneration showed excellent potential, including the co-delivery of drugs and biologics, and the employment of gene therapy, physical stimulation, cell sheets, scaffold surface modification and scaffold prevascularization. However, more standardized preclinical and clinical studies are still needed to understand their in vitro performance, in vivo effects, clinical usage and safety. Nonetheless, the novel PDLSCs-based bone grafts are highly promising for the regeneration of bone, dental and periodontal tissues.
Conceptualization, YB, KZ and HX; writing—original draft preparation, ZZ and JL; writing—review and editing, HX, MW, AS, TM and TO; funding acquisition, YB and ZZ All authors have read and agreed to the published version of the manuscript.
This study was supported by the National Natural Science Foundation of China (No. 82071144) (YB) and Beijing Stomatological Hospital, Capital Medical University Young Scientist Program (No. YSP202010) (ZZ).
The authors declare that the research was conducted in the absence of any commercial or financial relationships that could be construed as a potential conflict of interest.
All claims expressed in this article are solely those of the authors and do not necessarily represent those of their affiliated organizations, or those of the publisher, the editors and the reviewers. Any product that may be evaluated in this article, or claim that may be made by its manufacturer, is not guaranteed or endorsed by the publisher.
Abd Rahman, F., Mohd Ali, J., Abdullah, M., Abu Kasim, N. H., and Musa, S. (2016). Aspirin enhances osteogenic potential of periodontal ligament stem cells (PDLSCs) and modulates the expression profile of growth factor-associated genes in PDLSCs. J. Periodontol. 87 (7), 837–847. doi:10.1902/jop.2016.150610
Ahmadieh, H., and Arabi, A. (2011). Vitamins and bone health: Beyond calcium and vitamin D. Nutr. Rev. 69 (10), 584–598. doi:10.1111/j.1753-4887.2011.00372.x
Al-Sowayan, B., Alammari, F., and Alshareeda, A. (2020). Preparing the bone tissue regeneration ground by exosomes: From diagnosis to therapy. Molecules 25 (18), 4205. doi:10.3390/molecules25184205
Albanese, A., Licata, M. E., Polizzi, B., and Campisi, G. (2013). Platelet-rich plasma (PRP) in dental and oral surgery: From the wound healing to bone regeneration. Immun. Ageing 10 (1), 23. doi:10.1186/1742-4933-10-23
Alizadeh-Osgouei, M., Li, Y., and Wen, C. (2019). A comprehensive review of biodegradable synthetic polymer-ceramic composites and their manufacture for biomedical applications. Bioact. Mat. 4 (1), 22–36. doi:10.1016/j.bioactmat.2018.11.003
Altaie, A., Owston, H., and Jones, E. (2016). Use of platelet lysate for bone regeneration - are we ready for clinical translation? World J. Stem Cells 8 (2), 47–55. doi:10.4252/wjsc.v8.i2.47
Arpornmaeklong, P., Sareethammanuwat, M., Apinyauppatham, K., and Boonyuen, S. (2021). Characteristics and biologic effects of thermosensitive quercetin-chitosan/collagen hydrogel on human periodontal ligament stem cells. J. Biomed. Mat. Res. 109 (10), 1656–1670. doi:10.1002/jbm.b.34823
Atarbashi-Moghadam, F., Rezai Rad, M., Sijanivandi, S., Khodayari, P., and Mahmoum, M. (2022). Growth factors in periodontal complex regeneration. Chin. J. Dent. Res. 25 (2), 85–92. doi:10.3290/j.cjdr.b3086335
Avinash, K., Malaippan, S., and Dooraiswamy, J. N. (2017). Methods of isolation and characterization of stem cells from different regions of oral cavity using markers: A systematic review. Int. J. Stem Cells 10 (1), 12–20. doi:10.15283/ijsc17010
Bharadwaz, A., and Jayasuriya, A. C. (2020). Recent trends in the application of widely used natural and synthetic polymer nanocomposites in bone tissue regeneration. Mater. Sci. Eng. C 110, 110698. doi:10.1016/j.msec.2020.110698
Bigham-Sadegh, A., and Oryan, A. (2015). Selection of animal models for pre-clinical strategies in evaluating the fracture healing, bone graft substitutes and bone tissue regeneration and engineering. Connect. Tissue Res. 56 (3), 175–194. doi:10.3109/03008207.2015.1027341
Bow, A., Anderson, D. E., and Dhar, M. (2019). Commercially available bone graft substitutes: The impact of origin and processing on graft functionality. Drug Metab. Rev. 51 (4), 533–544. doi:10.1080/03602532.2019.1671860
Cao, F., Zhan, J., Chen, X., Zhang, K., Lai, R., and Feng, Z. (2017). miR-214 promotes periodontal ligament stem cell osteoblastic differentiation by modulating Wnt/β-catenin signaling. Mol. Med. Rep. 16 (6), 9301–9308. doi:10.3892/mmr.2017.7821
Chen, F. M., Gao, L. N., Tian, B. M., Zhang, X. Y., Zhang, Y. J., Dong, G. Y., et al. (2016a). Treatment of periodontal intrabony defects using autologous periodontal ligament stem cells: A randomized clinical trial. Stem Cell. Res. Ther. 7, 33. doi:10.1186/s13287-016-0288-1
Chen, W., Liu, J., Manuchehrabadi, N., Weir, M. D., Zhu, Z., and Xu, H. H. (2013). Umbilical cord and bone marrow mesenchymal stem cell seeding on macroporous calcium phosphate for bone regeneration in rat cranial defects. Biomaterials 34 (38), 9917–9925. doi:10.1016/j.biomaterials.2013.09.002
Chen, W., Liu, X., Chen, Q., Bao, C., Zhao, L., Zhu, Z., et al. (2018). Angiogenic and osteogenic regeneration in rats via calcium phosphate scaffold and endothelial cell co-culture with human bone marrow mesenchymal stem cells (MSCs), human umbilical cord MSCs, human induced pluripotent stem cell-derived MSCs and human embry. J. Tissue Eng. Regen. Med. 12 (1), 191–203. doi:10.1002/term.2395
Cho, H., Tarafder, S., Fogge, M., Kao, K., and Lee, C. H. (2016). Periodontal ligament stem/progenitor cells with protein-releasing scaffolds for cementum formation and integration on dentin surface. Connect. Tissue Res. 57 (6), 488–495. doi:10.1080/03008207.2016.1191478
Chen, X., Bai, S., Li, B., Liu, H., Wu, G., Liu, S., et al. (2016b). Fabrication of gelatin methacrylate/nanohydroxyapatite microgel arrays for periodontal tissue regeneration. Int. J. Nanomedicine 11, 4707–4718. doi:10.2147/IJN.S111701
Chen, H., Yang, H., Weir, M. D., Schneider, A., Ren, K., Homayounfar, N., et al. (2020a). An antibacterial and injectable calcium phosphate scaffold delivering human periodontal ligament stem cells for bone tissue engineering. RSC Adv. 10 (66), 40157–40170. doi:10.1039/d0ra06873j
Chen, J., Yu, M., Li, X., Sun, Q. F., Yang, C. Z., and Yang, P. S. (2020b). Progranulin promotes osteogenic differentiation of human periodontal ligament stem cells via tumor necrosis factor receptors to inhibit TNF-alpha sensitized NF-kB and activate ERK/JNK signaling. J. Periodontal Res. 55 (3), 363–373. doi:10.1111/jre.12720
Daghrery, A., Ferreira, J. A., de Souza Araujo, I. J., Clarkson, B. H., Eckert, G. J., Bhaduri, S. B., et al. (2021). A highly ordered, nanostructured fluorinated CaP-coated melt electrowritten scaffold for periodontal tissue regeneration. Adv. Healthc. Mat. 10 (21), e2101152. doi:10.1002/adhm.202101152
Di Vito, A., Chiarella, E., Baudi, F., Scardamaglia, P., Antonelli, A., Giudice, D., et al. (2020). Dose-dependent effects of zoledronic acid on human periodontal ligament stem cells: An in vitro pilot study. Cell. Transpl. 29, 096368972094849. doi:10.1177/0963689720948497
Ding, T., Li, J., Zhang, X., Du, L., Li, Y., Li, D., et al. (2020). Super-assembled core/shell fibrous frameworks with dual growth factors for in situ cementum-ligament-bone complex regeneration. Biomater. Sci. 8 (9), 2459–2471. doi:10.1039/d0bm00102c
Ding, T., Kang, W., Li, J., Yu, L., and Ge, S. (2021). An in situ tissue engineering scaffold with growth factors combining angiogenesis and osteoimmunomodulatory functions for advanced periodontal bone regeneration. J. Nanobiotechnology 19 (1), 247. doi:10.1186/s12951-021-00992-4
Diomede, F., Zini, N., Gatta, V., Fulle, S., Merciaro, I., D'Aurora, M., et al. (2016). Human periodontal ligament stem cells cultured onto cortico-cancellous scaffold drive bone regenerative process. Eur. Cell. Mat. 32, 181–201. doi:10.22203/ecm.v032a12
Divya, T. C., Muddappa, S. C., Singh, P., Rajan, R. R., Remya, M., and Sreehari, D. (2021). Drug repurposing for tooth regeneration: The promising premises. J. Pharm. Bioallied Sci. 13 (2), S957–S959. doi:10.4103/jpbs.jpbs_67_21
E, L. L., Xu, W. H., Feng, L., Liu, Y., Cai, D. Q., Wen, N., et al. (2016). Estrogen enhances the bone regeneration potential of periodontal ligament stem cells derived from osteoporotic rats and seeded on nano-hydroxyapatite/collagen/poly(L-lactide). Int. J. Mol. Med. 37 (6), 1475–1486. doi:10.3892/ijmm.2016.2559
Fliefel, R., Kuhnisch, J., Ehrenfeld, M., and Otto, S. (2017). Gene therapy for bone defects in oral and maxillofacial surgery: A systematic review and meta-analysis of animal studies. Stem Cells Dev. 26 (4), 215–230. doi:10.1089/scd.2016.0172
Fu, Y., Liu, S., Cui, S. J., Kou, X. X., Wang, X. D., Liu, X. M., et al. (2016). Surface chemistry of nanoscale mineralized collagen regulates periodontal ligament stem cell fate. ACS Appl. Mat. Interfaces 8 (25), 15958–15966. doi:10.1021/acsami.6b04951
Gao, L. N., An, Y., Lei, M., Li, B., Yang, H., Lu, H., et al. (2013). The effect of the coumarin-like derivative osthole on the osteogenic properties of human periodontal ligament and jaw bone marrow mesenchymal stem cell sheets. Biomaterials 34 (38), 9937–9951. doi:10.1016/j.biomaterials.2013.09.017
Gao, H., Li, B., Zhao, L., and Jin, Y. (2015). Influence of nanotopography on periodontal ligament stem cell functions and cell sheet based periodontal regeneration. Int. J. Nanomedicine 10, 4009–4027. doi:10.2147/IJN.S83357
Ge, S., Zhao, N., Wang, L., Yu, M., Liu, H., Song, A., et al. (2012). Bone repair by periodontal ligament stem cellseeded nanohydroxyapatite-chitosan scaffold. Int. J. Nanomedicine 7, 5405–5414. doi:10.2147/IJN.S36714
Gu, X., Li, M., Jin, Y., Liu, D., and Wei, F. (2017). Identification and integrated analysis of differentially expressed lncRNAs and circRNAs reveal the potential ceRNA networks during PDLSC osteogenic differentiation. BMC Genet. 18 (1), 100. doi:10.1186/s12863-017-0569-4
Gu, K., Fu, X., Tian, H., Zhang, Y., Li, A., Wang, Y., et al. (2020). TAZ promotes the proliferation and osteogenic differentiation of human periodontal ligament stem cells via the p-SMAD3. J. Cell. Biochem. 121 (2), 1101–1113. doi:10.1002/jcb.29346
Gui, N., Xu, W., Myers, D. E., Shukla, R., Tang, H. P., and Qian, M. (2018). The effect of ordered and partially ordered surface topography on bone cell responses: A review. Biomater. Sci. 6 (2), 250–264. doi:10.1039/c7bm01016h
Guo, W., He, Y., Tang, X., Chen, G., Shi, H., Gong, K., et al. (2014). Scaffold-free cell pellet transplantations can be applied to periodontal regeneration. Cell. Transpl. 23 (2), 181–194. doi:10.3727/096368912X662426
Han, J., Menicanin, D., Marino, V., Ge, S., Mrozik, K., Gronthos, S., et al. (2014). Assessment of the regenerative potential of allogeneic periodontal ligament stem cells in a rodent periodontal defect model. J. Periodontal Res. 49 (3), 333–345. doi:10.1111/jre.12111
He, H., Yu, J., Cao, J., E, L., Wang, D., Zhang, H., et al. (2011). Biocompatibility and osteogenic capacity of periodontal ligament stem cells on nHAC/PLA and HA/TCP scaffolds. J. Biomater. Sci. Polym. Ed. 22 (1-3), 179–194. doi:10.1163/092050609X12587018007767
Huang, S., Yu, F., Cheng, Y., Li, Y., Chen, Y., Tang, J., et al. (2021). Transforming growth factor-β3/recombinant human-like collagen/chitosan freeze-dried sponge primed with human periodontal ligament stem cells promotes bone regeneration in calvarial defect rats. Front. Pharmacol. 12, 678322. doi:10.3389/fphar.2021.678322
Hyun, S. Y., Lee, J. H., Kang, K. J., and Jang, Y. J. (2017). Effect of FGF-2, TGF-beta-1, and BMPs on teno/ligamentogenesis and osteo/cementogenesis of human periodontal ligament stem cells. Mol. Cells 40 (8), 550–557. doi:10.14348/molcells.2017.0019
Iwasaki, K., Akazawa, K., Nagata, M., Komaki, M., Peng, Y., Umeda, M., et al. (2021). Angiogenic effects of secreted factors from periodontal ligament stem cells. Dent. J. (Basel). 9 (1), 9. doi:10.3390/dj9010009
Jia, Q., Jiang, W., and Ni, L. (2015). Down-regulated non-coding RNA (lncRNA-ANCR) promotes osteogenic differentiation of periodontal ligament stem cells. Arch. Oral Biol. 60 (2), 234–241. doi:10.1016/j.archoralbio.2014.10.007
Jia, L., Xiong, Y., Zhang, W., Ma, X., and Xu, X. (2020). Metformin promotes osteogenic differentiation and protects against oxidative stress-induced damage in periodontal ligament stem cells via activation of the Akt/Nrf2 signaling pathway. Exp. Cell. Res. 386 (2), 111717. doi:10.1016/j.yexcr.2019.111717
Jung, I. H., Lee, S. H., Jun, C. M., Oh, N., and Yun, J. H. (2014). Characterization of the enhanced bone regenerative capacity of human periodontal ligament stem cells engineered to express the gene encoding bone morphogenetic protein 2. Tissue Eng. Part A 20 (15-16), 2189–2199. doi:10.1089/ten.TEA.2013.0648
Kammerer, P. W., Scholz, M., Baudisch, M., Liese, J., Wegner, K., Frerich, B., et al. (2017). Guided bone regeneration using collagen scaffolds, growth factors, and periodontal ligament stem cells for treatment of peri-implant bone defects in vivo. Stem Cells Int. 2017, 1–9. doi:10.1155/2017/3548435
Kim, S. Y., An, S. Y., Lee, J. S., and Heo, J. S. (2015). Zanthoxylum schinifolium enhances the osteogenic potential of periodontal ligament stem cells. Vitro Cell. Dev. Biol. -Animal. 51 (2), 165–173. doi:10.1007/s11626-014-9824-4
Lee, J. H., Um, S., Jang, J. H., and Seo, B. M. (2012). Effects of VEGF and FGF-2 on proliferation and differentiation of human periodontal ligament stem cells. Cell. Tissue Res. 348 (3), 475–484. doi:10.1007/s00441-012-1392-x
Lee, C. H., Hajibandeh, J., Suzuki, T., Fan, A., Shang, P., and Mao, J. J. (2014). Three-dimensional printed multiphase scaffolds for regeneration of periodontium complex. Tissue Eng. Part A 20 (7-8), 1342–1351. doi:10.1089/ten.TEA.2013.0386
Lee, T. H., Kim, W. T., Ryu, C. J., and Jang, Y. J. (2015). Optimization of treatment with recombinant FGF-2 for proliferation and differentiation of human dental stem cells, mesenchymal stem cells, and osteoblasts. Biochem. Cell. Biol. 93 (4), 298–305. doi:10.1139/bcb-2014-0140
Lee, J. S., Kim, E., Han, S., Kang, K. L., and Heo, J. S. (2017). Evaluating the oxysterol combination of 22(S)-hydroxycholesterol and 20(S)-hydroxycholesterol in periodontal regeneration using periodontal ligament stem cells and alveolar bone healing models. Stem Cell. Res. Ther. 8 (1), 276. doi:10.1186/s13287-017-0725-9
Lei, M., Li, K., Li, B., Gao, L. N., Chen, F. M., and Jin, Y. (2014). Mesenchymal stem cell characteristics of dental pulp and periodontal ligament stem cells after in vivo transplantation. Biomaterials 35 (24), 6332–6343. doi:10.1016/j.biomaterials.2014.04.071
Li, M., Ma, J., Gao, Y., and Yang, L. (2019). Cell sheet technology: A promising strategy in regenerative medicine. Cytotherapy 21 (1), 3–16. doi:10.1016/j.jcyt.2018.10.013
Li, H., Zhou, J., Zhu, M., Ying, S., Li, L., Chen, D., et al. (2021). Low-intensity pulsed ultrasound promotes the formation of periodontal ligament stem cell sheets and ectopic periodontal tissue regeneration. J. Biomed. Mat. Res. A 109 (7), 1101–1112. doi:10.1002/jbm.a.37102
Liang, Q., Du, L., Zhang, R., Kang, W., and Ge, S. (2021). Stromal cell-derived factor-1/Exendin-4 cotherapy facilitates the proliferation, migration and osteogenic differentiation of human periodontal ligament stem cells in vitro and promotes periodontal bone regeneration in vivo. Cell. Prolif. 54 (3), e12997. doi:10.1111/cpr.12997
Liu, X., Chen, W., Zhang, C., Thein-Han, W., Hu, K., Reynolds, M. A., et al. (2017). Co-seeding human endothelial cells with human-induced pluripotent stem cell-derived mesenchymal stem cells on calcium phosphate scaffold enhances osteogenesis and vascularization in rats. Tissue Eng. Part A 23 (11-12), 546–555. doi:10.1089/ten.tea.2016.0485
Liu, J., Zhao, X., Pei, D., Sun, G., Li, Y., Zhu, C., et al. (2018). The promotion function of Berberine for osteogenic differentiation of human periodontal ligament stem cells via ERK-FOS pathway mediated by EGFR. Sci. Rep. 8 (1), 2848. doi:10.1038/s41598-018-21116-3
Liu, J., Ruan, J., Weir, M. D., Ren, K., Schneider, A., Wang, P., et al. (2019a). Periodontal bone-ligament-cementum regeneration via scaffolds and stem cells. Cells 8 (6), 537. doi:10.3390/cells8060537
Liu, Y., Cheng, Z., Pang, Y., Cui, L., Qian, T., Quan, L., et al. (2019b). Role of microRNAs, circRNAs and long noncoding RNAs in acute myeloid leukemia. J. Hematol. Oncol. 12 (1), 51. doi:10.1186/s13045-019-0734-5
Liu, Z., Liu, Q., Guo, H., Liang, J., and Zhang, Y. (2022). Overview of physical and pharmacological therapy in enhancing bone regeneration formation during distraction osteogenesis. Front. Cell. Dev. Biol. 10, 837430. doi:10.3389/fcell.2022.837430
Lu, C. H., Chang, Y. H., Lin, S. Y., Li, K. C., and Hu, Y. C. (2013). Recent progresses in gene delivery-based bone tissue engineering. Biotechnol. Adv. 31 (8), 1695–1706. doi:10.1016/j.biotechadv.2013.08.015
Lu, Y., Zhang, W., Wang, J., Yang, G., Yin, S., Tang, T., et al. (2019). Recent advances in cell sheet technology for bone and cartilage regeneration: From preparation to application. Int. J. Oral Sci. 11 (2), 17. doi:10.1038/s41368-019-0050-5
Madsen, E., Mededovic, M., and Kohn, D. H. (2020). Review on material parameters to enhance bone cell function in vitro and in vivo. Biochem. Soc. Trans. 48 (5), 2039–2050. doi:10.1042/BST20200210
Maeda, H., Wada, N., Tomokiyo, A., Monnouchi, S., and Akamine, A. (2013). Prospective potency of TGF-β1 on maintenance and regeneration of periodontal tissue. Int. Rev. Cell. Mol. Biol. 304, 283–367. doi:10.1016/B978-0-12-407696-9.00006-3
Mao, L. X., Liu, J., Zhao, J., Xia, L., Jiang, L., Wang, X., et al. (2015). Effect of micro-nano-hybrid structured hydroxyapatite bioceramics on osteogenic and cementogenic differentiation of human periodontal ligament stem cell via Wnt signaling pathway. Int. J. Nanomedicine 10, 7031–7044. doi:10.2147/IJN.S90343
Massari, L., Benazzo, F., Falez, F., Perugia, D., Pietrogrande, L., Setti, S., et al. (2019). Biophysical stimulation of bone and cartilage: State of the art and future perspectives. Int. Orthop. 43 (3), 539–551. doi:10.1007/s00264-018-4274-3
Mazzoni, S., Mohammadi, S., Tromba, G., Diomede, F., Piattelli, A., Trubiani, O., et al. (2017). Role of cortico-cancellous heterologous bone in human periodontal ligament stem cell xeno-free culture studied by synchrotron radiation phase-contrast microtomography. Int. J. Mol. Sci. 18 (2), 364. doi:10.3390/ijms18020364
Miron, R. J., Guillemette, V., Zhang, Y., Chandad, F., and Sculean, A. (2014). Enamel matrix derivative in combination with bone grafts: A review of the literature. Quintessence Int. 45 (6), 475–487. doi:10.3290/j.qi.a31541
Moshaverinia, A., Chen, C., Akiyama, K., Xu, X., Chee, W. W., Schricker, S. R., et al. (2013). Encapsulated dental-derived mesenchymal stem cells in an injectable and biodegradable scaffold for applications in bone tissue engineering. J. Biomed. Mat. Res. A 101 (11), 3285–3294. doi:10.1002/jbm.a.34546
Musson, D. S., Gao, R., Watson, M., Lin, J. M., Park, Y. E., Tuari, D., et al. (2019). Bovine bone particulates containing bone anabolic factors as a potential xenogenic bone graft substitute. J. Orthop. Surg. Res. 14 (1), 60. doi:10.1186/s13018-019-1089-x
Myneni, V. D., and Mezey, E. (2017). Regulation of bone remodeling by vitamin K2. Oral Dis. 23 (8), 1021–1028. doi:10.1111/odi.12624
Oliveira, E. R., Nie, L., Podstawczyk, D., Allahbakhsh, A., Ratnayake, J., Brasil, D. L., et al. (2021). Advances in growth factor delivery for bone tissue engineering. Int. J. Mol. Sci. 22 (2), 903. doi:10.3390/ijms22020903
Ou, Q., Miao, Y., Yang, F., Lin, X., Zhang, L. M., and Wang, Y. (2019). Zein/gelatin/nanohydroxyapatite nanofibrous scaffolds are biocompatible and promote osteogenic differentiation of human periodontal ligament stem cells. Biomater. Sci. 7 (5), 1973–1983. doi:10.1039/c8bm01653d
Padilla, F., Puts, R., Vico, L., and Raum, K. (2014). Stimulation of bone repair with ultrasound: A review of the possible mechanic effects. Ultrasonics 54 (5), 1125–1145. doi:10.1016/j.ultras.2014.01.004
Petho, A., Chen, Y., and George, A. (2018). Exosomes in extracellular matrix bone biology. Curr. Osteoporos. Rep. 16 (1), 58–64. doi:10.1007/s11914-018-0419-y
Pizzicannella, J., Cavalcanti, M., Trubiani, O., and Diomede, F. (2018). MicroRNA 210 mediates VEGF upregulation in human periodontal ligament stem cells cultured on 3DHydroxyapatite ceramic scaffold. Int. J. Mol. Sci. 19 (12), 3916. doi:10.3390/ijms19123916
Porter, J. R., Ruckh, T. T., and Popat, K. C. (2009). Bone tissue engineering: A review in bone biomimetics and drug delivery strategies. Biotechnol. Prog. 25 (6), 1539–1560. doi:10.1002/btpr.246
Qi, G., Yu, K., Feng, Y., Zhang, Y., Shao, Q., Yu, M., et al. (2021). 1α, 25-dihydroxyvitamin D3 promotes early osteogenic differentiation of PDLSCs and a 12-year follow-up case of early-onset vitamin D deficiency periodontitis. J. Steroid Biochem. Mol. Biol. 208, 105805. doi:10.1016/j.jsbmb.2020.105805
Qin, W., Chen, J. Y., Guo, J., Ma, T., Weir, M. D., Guo, D., et al. (2018). Novel calcium phosphate cement with metformin-loaded chitosan for odontogenic differentiation of human dental pulp cells. Stem Cells Int. 2018, 7173481–7173510. doi:10.1155/2018/7173481
Qiu, W., Wu, B. L., and Fang, F. C. (2020). Overview of noncoding RNAs involved in the osteogenic differentiation of periodontal ligament stem cells. World J. Stem Cells 12 (4), 251–265. doi:10.4252/wjsc.v12.i4.251
Qiu, G., Wu, H., Huang, M., Ma, T., Schneider, A., Oates, T. W., et al. (2021). Novel calcium phosphate cement with biofilm-inhibition and platelet lysate delivery to enhance osteogenesis of encapsulated human periodontal ligament stem cells. Mater. Sci. Eng. C 128, 112306. doi:10.1016/j.msec.2021.112306
Qu, H., Fu, H., Han, Z., and Sun, Y. (2019). Biomaterials for bone tissue engineering scaffolds: A review. RSC Adv. 9 (45), 26252–26262. doi:10.1039/c9ra05214c
Queiroz, A., Albuquerque-Souza, E., Gasparoni, L. M., de Franca, B. N., Pelissari, C., Trierveiler, M., et al. (2021). Therapeutic potential of periodontal ligament stem cells. World J. Stem Cells 13 (6), 605–618. doi:10.4252/wjsc.v13.i6.605
Safi, I. N., Mohammed Ali Hussein, B., and Al-Shammari, A. M. (2019). In vitro periodontal ligament cell expansion by co-culture method and formation of multi-layered periodontal ligament-derived cell sheets. Regen. Ther. 11, 225–239. doi:10.1016/j.reth.2019.08.002
Samavedi, S., Whittington, A. R., and Goldstein, A. S. (2013). Calcium phosphate ceramics in bone tissue engineering: A review of properties and their influence on cell behavior. Acta Biomater. 9 (9), 8037–8045. doi:10.1016/j.actbio.2013.06.014
Scott, M. A., Levi, B., Askarinam, A., Nguyen, A., Rackohn, T., Ting, K., et al. (2012). Brief review of models of ectopic bone formation. Stem Cells Dev. 21 (5), 655–667. doi:10.1089/scd.2011.0517
Shapiro, G., Lieber, R., Gazit, D., and Pelled, G. (2018). Recent advances and future of gene therapy for bone regeneration. Curr. Osteoporos. Rep. 16 (4), 504–511. doi:10.1007/s11914-018-0459-3
Shi, H., Zong, W., Xu, X., and Chen, J. (2018). Improved biphasic calcium phosphate combined with periodontal ligament stem cells may serve as a promising method for periodontal regeneration. Am. J. Transl. Res. 10 (12), 4030.
Sohn, H. S., and Oh, J. K. (2019). Review of bone graft and bone substitutes with an emphasis on fracture surgeries. Biomater. Res. 23, 9. doi:10.1186/s40824-019-0157-y
Su, F., Liu, S. S., Ma, J. L., Wang, D. S., E, L. L., and Liu, H. C. (2015). Enhancement of periodontal tissue regeneration by transplantation of osteoprotegerin-engineered periodontal ligament stem cells. Stem Cell. Res. Ther. 6, 22. doi:10.1186/s13287-015-0023-3
Sun, J., Dong, Z., Zhang, Y., He, X., Fei, D., Jin, F., et al. (2017). Osthole improves function of periodontitis periodontal ligament stem cells via epigenetic modification in cell sheets engineering. Sci. Rep. 7 (1), 5254. doi:10.1038/s41598-017-05762-7
Tajbakhsh, S., and Hajiali, F. (2017). A comprehensive study on the fabrication and properties of biocomposites of poly(lactic acid)/ceramics for bone tissue engineering. Mater. Sci. Eng. C 70 (1), 897–912. doi:10.1016/j.msec.2016.09.008
Tanimoto, K., Huang, Y. C., Tanne, Y., Kunimatsu, R., Michida, M., Yoshioka, M., et al. (2012). Amelogenin enhances the osteogenic differentiation of mesenchymal stem cells derived from bone marrow. Cells Tissues Organs 196 (5), 411–419. doi:10.1159/000335912
Tansriratanawong, K., Wongwan, P., Ishikawa, H., Nakahara, T., and Wongravee, K. (2018). Cellular responses of periodontal ligament stem cells to a novel synthesized form of calcium hydrogen phosphate with a hydroxyapatite-like surface for periodontal tissue engineering. J. Oral Sci. 60 (3), 428–437. doi:10.2334/josnusd.17-0343
Thanasrisuebwong, P., Kiattavorncharoen, S., Surarit, R., Phruksaniyom, C., and Ruangsawasdi, N. (2020). Red and yellow injectable platelet-rich fibrin demonstrated differential effects on periodontal ligament stem cell proliferation, migration, and osteogenic differentiation. Int. J. Mol. Sci. 21 (14), 5153. doi:10.3390/ijms21145153
Tian, Y., Liu, M., Liu, Y., Shi, C., Wang, Y., Liu, T., et al. (2021). The performance of 3D bioscaffolding based on a human periodontal ligament stem cell printing technique. J. Biomed. Mat. Res. A 109 (7), 1209–1219. doi:10.1002/jbm.a.37114
Tomokiyo, A., Wada, N., and Maeda, H. (2019). Periodontal ligament stem cells: Regenerative potency in periodontium. Stem Cells Dev. 28 (15), 974–985. doi:10.1089/scd.2019.0031
Trubiani, O., Pizzicannella, J., Caputi, S., Marchisio, M., Mazzon, E., Paganelli, R., et al. (2019). Periodontal ligament stem cells: Current knowledge and future perspectives. Stem Cells Dev. 28 (15), 995–1003. doi:10.1089/scd.2019.0025
Walmsley, G. G., McArdle, A., Tevlin, R., Momeni, A., Atashroo, D., Hu, M. S., et al. (2015). Nanotechnology in bone tissue engineering. Nanomedicine Nanotechnol. Biol. Med. 11 (5), 1253–1263. doi:10.1016/j.nano.2015.02.013
Wang, L., Zhang, C., Li, C., Weir, M. D., Wang, P., Reynolds, M. A., et al. (2016a). Injectable calcium phosphate with hydrogel fibers encapsulating induced pluripotent, dental pulp and bone marrow stem cells for bone repair. Mater. Sci. Eng. C 69, 1125–1136. doi:10.1016/j.msec.2016.08.019
Wang, Z., Feng, Z., Wu, G., Bai, S., Dong, Y., and Zhao, Y. (2016b). In vitro studies on human periodontal ligament stem cell sheets enhanced by enamel matrix derivative. Colloids Surfaces B Biointerfaces 141, 102–111. doi:10.1016/j.colsurfb.2016.01.036
Wang, Z. S., Feng, Z. H., Wu, G. F., Bai, S. Z., Dong, Y., Chen, F. M., et al. (2016c). The use of platelet-rich fibrin combined with periodontal ligament and jaw bone mesenchymal stem cell sheets for periodontal tissue engineering. Sci. Rep. 6, 28126. doi:10.1038/srep28126
Wang, M., Li, J., Ye, Y., He, S., and Song, J. (2020). SHED-derived conditioned exosomes enhance the osteogenic differentiation of PDLSCs via Wnt and BMP signaling in vitro. Differentiation 111, 1–11. doi:10.1016/j.diff.2019.10.003
Wang, X., and Thomsen, P. (2021). Mesenchymal stem cell-derived small extracellular vesicles and bone regeneration. Basic Clin. Pharmacol. Toxicol. 128 (1), 18–36. doi:10.1111/bcpt.13478
Wei, F., Qu, C., Song, T., Ding, G., Fan, Z., Liu, D., et al. (2012). Vitamin C treatment promotes mesenchymal stem cell sheet formation and tissue regeneration by elevating telomerase activity. J. Cell. Physiol. 227 (9), 3216–3224. doi:10.1002/jcp.24012
Wei, K., Xie, Y., Chen, T., Fu, B., Cui, S., Wang, Y., et al. (2017). ERK1/2 signaling mediated naringin-induced osteogenic differentiation of immortalized human periodontal ligament stem cells. Biochem. Biophys. Res. Commun. 489 (3), 319–325. doi:10.1016/j.bbrc.2017.05.130
Wu, D., Yin, L., Sun, D., Wang, F., Wu, Q., Xu, Q., et al. (2020). Long noncoding RNA TUG1 promotes osteogenic differentiation of human periodontal ligament stem cell through sponging microRNA-222-3p to negatively regulate Smad2/7. Arch. Oral Biol. 117, 104814. doi:10.1016/j.archoralbio.2020.104814
Xu, Q., Li, B., Yuan, L., Dong, Z., Zhang, H., Wang, H., et al. (2017). Combination of platelet-rich plasma within periodontal ligament stem cell sheets enhances cell differentiation and matrix production. J. Tissue Eng. Regen. Med. 11 (3), 627–636. doi:10.1002/term.1953
Yamauchi, N., Taguchi, Y., Kato, H., and Umeda, M. (2018). High-power, red-light-emitting diode irradiation enhances proliferation, osteogenic differentiation, and mineralization of human periodontal ligament stem cells via ERK signaling pathway. J. Periodontol. 89 (3), 351–360. doi:10.1002/JPER.17-0365
Yang, Z. H., Zhang, X. J., Dang, N. N., Ma, Z. F., Xu, L., Wu, J. J., et al. (2009). Apical tooth germ cell-conditioned medium enhances the differentiation of periodontal ligament stem cells into cementum/periodontal ligament-like tissues. J. Periodontal Res. 44 (2), 199–210. doi:10.1111/j.1600-0765.2008.01106.x
Yang, M., Gao, X., Shen, Z., Shi, X., and Lin, Z. (2018). Gelatin-assisted conglutination of aligned polycaprolactone nanofilms into a multilayered fibre-guiding scaffold for periodontal ligament regeneration. RSC Adv. 9 (1), 507–518. doi:10.1039/c8ra09073d
Yeasmin, S., Ceccarelli, J., Vigen, M., Carrion, B., Putnam, A. J., Tarle, S. A., et al. (2014). Stem cells derived from tooth periodontal ligament enhance functional angiogenesis by endothelial cells. Tissue Eng. Part A 20 (7-8), 1188–1196. doi:10.1089/ten.TEA.2013.0512
Yi, T., Jun, C. M., Kim, S. J., and Yun, J. H. (2016). Evaluation of in vivo osteogenic potential of bone morphogenetic protein 2-overexpressing human periodontal ligament stem cells combined with biphasic calcium phosphate Block scaffolds in a critical-size bone defect model. Tissue Eng. Part A 22 (5-6), 501–512. doi:10.1089/ten.TEA.2015.0337
Yin, L. H., Cheng, W. X., Qin, Z. S., Sun, K. M., Zhong, M., Wang, J. K., et al. (2015). Effects of ginsenoside Rg-1 on the proliferation and osteogenic differentiation of human periodontal ligament stem cells. Chin. J. Integr. Med. 21 (9), 676–681. doi:10.1007/s11655-014-1856-9
Yorukoglu, A. C., Kiter, A. E., Akkaya, S., Satiroglu-Tufan, N. L., and Tufan, A. C. (2017). A concise review on the use of mesenchymal stem cells in cell sheet-based tissue engineering with special emphasis on bone tissue regeneration. Stem Cells Int. 2017, 2374161–2374213. doi:10.1155/2017/2374161
Yu, B. H., Zhou, Q., and Wang, Z. L. (2014). Periodontal ligament versus bone marrow mesenchymal stem cells in combination with bio-oss scaffolds for ectopic and in situ bone formation: A comparative study in the rat. J. Biomater. Appl. 29 (2), 243–253. doi:10.1177/0885328214521846
Yu, M., Wang, L., Ba, P., Li, L., Sun, L., Duan, X., et al. (2017). Osteoblast progenitors enhance osteogenic differentiation of periodontal ligament stem cells. J. Periodontol. 88 (10), e159–e168. doi:10.1902/jop.2017.170016
Yu, M., Sun, L., Ba, P., Li, L., Chen, J., and Sun, Q. (2021). Progranulin promotes osteogenic differentiation of periodontal membrane stem cells in both inflammatory and non-inflammatory conditions. J. Int. Med. Res. 49 (8), 030006052110325. doi:10.1177/03000605211032508
Zha, K., Tian, Y., Panayi, A. C., Mi, B., and Liu, G. (2022). Recent advances in enhancement strategies for osteogenic differentiation of mesenchymal stem cells in bone tissue engineering. Front. Cell. Dev. Biol. 10, 824812. doi:10.3389/fcell.2022.824812
Zhang, C., Li, J., Zhang, L., Zhou, Y., Hou, W., Quan, H., et al. (2012). Effects of mechanical vibration on proliferation and osteogenic differentiation of human periodontal ligament stem cells. Arch. Oral Biol. 57 (10), 1395–1407. doi:10.1016/j.archoralbio.2012.04.010
Zhang, L., Liu, W., Zhao, J., Ma, X., Shen, L., Zhang, Y., et al. (2016). Mechanical stress regulates osteogenic differentiation and RANKL/OPG ratio in periodontal ligament stem cells by the Wnt/β-catenin pathway. Biochimica Biophysica Acta - General Subj. 1860 (10), 2211–2219. doi:10.1016/j.bbagen.2016.05.003
Zhang, Y., Wang, P., Wang, Y., Li, J., Qiao, D., Chen, R., et al. (2021). Gold nanoparticles promote the bone regeneration of periodontal ligament stem cell sheets through activation of autophagy. Int. J. Nanomedicine 16, 61–73. doi:10.2147/IJN.S282246
Zhao, L., Weir, M. D., and Xu, H. H. (2010). An injectable calcium phosphate-alginate hydrogel-umbilical cord mesenchymal stem cell paste for bone tissue engineering. Biomaterials 31 (25), 6502–6510. doi:10.1016/j.biomaterials.2010.05.017
Zhao, B. J., and Liu, Y. H. (2014). Simvastatin induces the osteogenic differentiation of human periodontal ligament stem cells. Fundam. Clin. Pharmacol. 28 (5), 583–592. doi:10.1111/fcp.12050
Zhao, B., Zhang, Y., Xiong, Y., and Xu, X. (2019a). Rutin promotes the formation and osteogenic differentiation of human periodontal ligament stem cell sheets in vitro. Int. J. Mol. Med. 44 (6), 2289–2297. doi:10.3892/ijmm.2019.4384
Zhao, Z., Liu, J., Schneider, A., Gao, X., Ren, K., Weir, M. D., et al. (2019b). Human periodontal ligament stem cell seeding on calcium phosphate cement scaffold delivering metformin for bone tissue engineering. J. Dent. 91, 103220. doi:10.1016/j.jdent.2019.103220
Zhao, Z., Liu, J., Weir, M. D., Zhang, N., Zhang, L., Xie, X., et al. (2019c). Human periodontal ligament stem cells on calcium phosphate scaffold delivering platelet lysate to enhance bone regeneration. RSC Adv. 9 (70), 41161–41172. doi:10.1039/c9ra08336g
Zhao, B., Chen, J., Zhao, L., Deng, J., and Li, Q. (2020). A simvastatin-releasing scaffold with periodontal ligament stem cell sheets for periodontal regeneration. J. Appl. Biomater. Funct. Mat. 18, 228080001990009. doi:10.1177/2280800019900094
Zhao, R., Yang, R., Cooper, P. R., Khurshid, Z., Shavandi, A., and Ratnayake, J. (2021a). Bone grafts and substitutes in dentistry: A review of current trends and developments. Molecules 26 (10), 3007. doi:10.3390/molecules26103007
Zhao, Z., Sun, Y., Qiao, Q., Zhang, L., Xie, X., Weir, M. D., et al. (2021b). Human periodontal ligament stem cell and umbilical vein endothelial cell Co-culture to prevascularize scaffolds for angiogenic and osteogenic tissue engineering. Int. J. Mol. Sci. 22, 12363. doi:10.3390/ijms222212363
Zheng, D. H., Wang, X. X., Ma, D., Zhang, L. N., Qiao, Q. F., and Zhang, J. (2019). <p>Erythropoietin enhances osteogenic differentiation of human periodontal ligament stem cells via Wnt/β-catenin signaling pathway</p>. Drug Des. devel. Ther. 13, 2543–2552. doi:10.2147/DDDT.S214116
Zhou, Q., Zhao, Z. N., Cheng, J. T., Zhang, B., Xu, J., Huang, F., et al. (2011). Ibandronate promotes osteogenic differentiation of periodontal ligament stem cells by regulating the expression of microRNAs. Biochem. Biophys. Res. Commun. 404 (1), 127–132. doi:10.1016/j.bbrc.2010.11.079
Zhou, H., Chen, W., Weir, M. D., and Xu, H. H. K. (2012). Biofunctionalized calcium phosphate cement to enhance the attachment and osteodifferentiation of stem cells released from fast-degradable alginate-fibrin microbeads. Tissue Eng. Part A 18 (15-16), 1583–1595. doi:10.1089/ten.tea.2011.0604
Keywords: periodontal ligament stem cells, scaffold, bone regeneration, periodontal tissue regeneration, tissue engineering
Citation: Zhao Z, Liu J, Weir MD, Schneider A, Ma T, Oates TW, Xu HHK, Zhang K and Bai Y (2022) Periodontal ligament stem cell-based bioactive constructs for bone tissue engineering. Front. Bioeng. Biotechnol. 10:1071472. doi: 10.3389/fbioe.2022.1071472
Received: 16 October 2022; Accepted: 17 November 2022;
Published: 02 December 2022.
Edited by:
Chaenyung Cha, Ulsan National Institute of Science and Technology, South KoreaReviewed by:
Jingang Xiao, Southwest Medical University, ChinaCopyright © 2022 Zhao, Liu, Weir, Schneider, Ma, Oates, Xu, Zhang and Bai. This is an open-access article distributed under the terms of the Creative Commons Attribution License (CC BY). The use, distribution or reproduction in other forums is permitted, provided the original author(s) and the copyright owner(s) are credited and that the original publication in this journal is cited, in accordance with accepted academic practice. No use, distribution or reproduction is permitted which does not comply with these terms.
*Correspondence: Yuxing Bai, Ynl1eGluZ0BjY211LmVkdS5jbg==; Ke Zhang, dHV6aXpoYW5na2VAMTYzLmNvbQ==
Disclaimer: All claims expressed in this article are solely those of the authors and do not necessarily represent those of their affiliated organizations, or those of the publisher, the editors and the reviewers. Any product that may be evaluated in this article or claim that may be made by its manufacturer is not guaranteed or endorsed by the publisher.
Research integrity at Frontiers
Learn more about the work of our research integrity team to safeguard the quality of each article we publish.