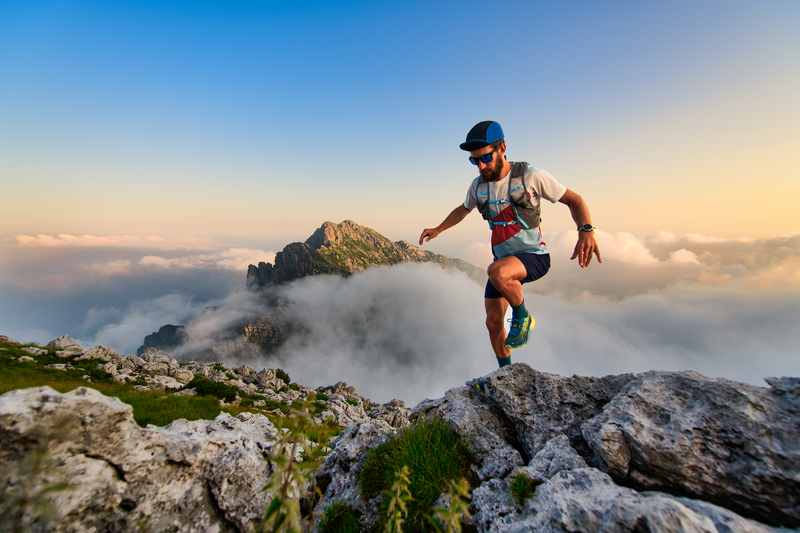
95% of researchers rate our articles as excellent or good
Learn more about the work of our research integrity team to safeguard the quality of each article we publish.
Find out more
ORIGINAL RESEARCH article
Front. Bioeng. Biotechnol. , 23 November 2022
Sec. Biosensors and Biomolecular Electronics
Volume 10 - 2022 | https://doi.org/10.3389/fbioe.2022.1068533
This article is part of the Research Topic Optical Imaging Technologies for Oxidative Stress-mediated Disease Diagnosis and Therapy View all 5 articles
Kidneys play an important part in drug metabolism and excretion. High local concentration of drugs or drug allergies often cause acute kidney injury (AKI). Identification of effective biomarkers of initial stage AKI and constructing activable molecular probes with excellent detection properties for early evaluation of AKI are necessary, yet remain significant challenges. Alkaline phosphatase (ALP), a key hydrolyzing protease, exists in the epithelial cells of the kidney and is discharged into the urine following kidney injury. However, no studies have revealed its level in drug-induced AKI. Existing ALP fluorescent molecular probes are not suitable for testing and imaging of ALP in the AKI model. Drug-induced AKI is accompanied by oxidative stress, and many studies have indicated that a large increase in reactive oxygen species (ROS) occur in the AKI model. Thus, the probe used for imaging of AKI must be chemically stable in the presence of ROS. However, most existing near-infrared fluorescent (NIRF) ALP probes are not stable in the presence of ROS in the AKI model. Hence, we built a chemically stable molecular sensor (CS-ALP) to map ALP level in cisplatin-induced AKI. This novel probe is not destroyed by ROS generated in the AKI model, thus allowing high-fidelity imaging. In the presence of ALP, the CS-ALP probe generates a new absorbance peak at 685 nm and a fluorescent emission peak at 716 nm that could be used to “turn on” photoacoustic (PA) and NIRF imaging of ALP in AKI. Levels of CS-ALP build up rapidly in the kidney, and CS-ALP has been successfully applied in NIRF/PA bimodal in vivo imaging. Through the NIRF/PA bimodal imaging results, we demonstrate that upregulated expression of ALP occurs in the early stages of AKI and continues with injury progression.
Drug-induced acute kidney injury (AKI) leads to serious morbidity and mortality (Blatt and Liebman, 2013). In the AKI process, drugs, such as cisplatin, first induce oxidative stress, which is followed by lysosomal damage and apoptosis. A recent survey indicated that AKI often continues unabated (Hsu et al., 2013). Unfortunately, the therapeutic schedule for AKI has not been highly effective due to lack of efficient biomarkers for early diagnosis and incomplete understanding of AKI pathogenesis (Charlton et al., 2014). Current clinical testing generally depends on the detection of urea nitrogen and serum creatinine in serum samples, which are often untimely, insensitive, and an indirect indicator of AKI (Charlton et al., 2014). In recent years, there has been extensive research focused on identification and verification of effective biomarkers for AKI. Previous studies have listed both potential and validated biomarkers of AKI, including small molecules, reactive oxygen species (O2•−, ONOO−, and ClO−), proteins, and enzymes (Liu H.-W. et al., 2020; Liu L. et al., 2020; Cheng et al., 2020; Huang and Pu, 2021). Among them, several enzymatic biomarkers, including N-acetyl-β-glucosaminidase (NAG), alanine aminopeptidase (AAP), and alkaline phosphatase (ALP), exist in the epithelial cells of the kidney and are discharged into the urine following kidney damage (Charlton et al., 2014). Additionally, fluorescent sensors have been developed for testing NAG, gamma glutamyl transferase (GGT), and AAP in AKI, and the results have demonstrated the overexpression of these enzymes in the early stages of AKI (Lv et al., 2018; Huang et al., 2019; Huang et al., 2020; Tan et al., 2021). However, the expression level of ALP in AKI has not been adequately assessed. Therefore, there is a need for construction of an activable molecular probe for detection of ALP in AKI to improve early detection of AKI.
ALP catalyzes the hydrolytic reaction and transphosphorylation of substrates. It is widely expressed, at low levels, in human tissues, including bone, liver, and kidney. However, its activity is remarkably increased in several human diseases (Orimo, 2010). Despite abundant research on ALP fluorescent probes (Supplementary Table S1, ESI) (Li et al., 2012; Zhou et al., 2016; Li et al., 2017; Liu et al., 2017; Liu et al., 2018; Zhang P. et al., 2019; Zhang Q. et al., 2019; Gao et al., 2019; Wang et al., 2021b), its role in AKI remains unclear due to the lack of a kidney-specific molecular probe. Furthermore, existing ALP fluorescent molecular probes are not suitable for testing and imaging of ALP in the AKI model. As summarized in Supplementary Table S1, previously developed near-infrared fluorescent (NIRF) ALP probes were based on cyanine dye, which is not stable in the presence of ROS in the AKI model; many studies have indicated that there is a large increase in ROS in AKI (Scheme 1A) (Jia et al., 2016; Cheng et al., 2019; Cheng et al., 2021; Li et al., 2021). In addition, the existing probes are hydrophobic and metabolized by the liver; therefore, no probe enrichment can occur in the kidney (Huang et al., 2017; Chen et al., 2021; Du et al., 2021; Yang et al., 2021). Development of a novel ALP fluorescent probe that is water soluble, chemically stable, and useful for in situ monitoring of ALP activity in the AKI model remains a significant challenge.
SCHEME 1. (A) Proposed reasons for choosing chemically stable dyes for constructing imaging probes for analysis of the drug-induced AKI mouse model. (B) Selection of a chemically stable NIR dye to construct a water-soluble probe, the structure of the proposed CS-ALP probe, and the mechanism of the reaction between CS-ALP and ALP and its application for in vivo NIRF/PA bimodal imaging.
Herein, we report construction of a new NIRF probe, a chemically stable molecular sensor (CS-ALP), for studying AKI through measurement of ALP. CS-ALP was designed based on intramolecular charge transfer (ICT) and is composed of the chemically stable (CS) hemicyanine dye with a NIRF reporter and monophosphate linked to the hydroxyl group of the CS dye as an ALP cleavage site (Scheme 1B). Preliminary experiments and previous work have indicated that CS dye is stable in the presence of ROS at physiological concentration and shows mild water-solubility (Lv et al., 2018); thus, we chose CS dye for use in construction of an activable probe for high-fidelity imaging of ALP in AKI. In the presence of increasing concentrations of ALP, the monophosphate moiety is gradually hydrolyzed, resulting in gradual increase of the absorption maxima at 685 nm, which can be used for photoacoustic (PA) imaging. At the same time, the fluorescent emission peak at 716 nm was gradually enhanced, generating turn-on NIRF imaging. The probe displayed rapid response to ALP, high sensitivity (limit of detection: 0.26 U/L cal), and excellent specificity. Moreover, CS-ALP was successfully used for NIRF/PA bimodal imaging of ALP generated in cisplatin-stimulated human renal cortex proximal tubule epithelial cells (HK-2). Furthermore, using CS-ALP, the NIRF/PA bimodal in vivo visualization of ALP level in cisplatin-induced AKI was achieved for the first time, with results demonstrating that upregulated expression of ALP occurs at the early stages of AKI and continues with AKI progression.
General reagents and all required materials, instruments, the probe synthesis protocol, and experimental data are provided in the Supporting Information (ESI).
The probe was dissolved in DMSO to produce a stock solution (1.0 × 10−4 M). To obtain good optical performance of the probe, we used 5% DMSO as co-solvent. Since phosphate could inhibit the activity of ALP, we used 10 mM tris-buffered saline (TBS, pH 8.0) for in vitro experiments. Both the UV-Vis absorption and fluorescent spectrum experiments were carried out in 10 mM TBS (pH 8.0). The fluorescent emission spectra were tested using a Hitachi F7000 spectrophotometer, with the excitation wavelength at 680 nm and the emission wavelength ranging from 700 to 900 nm. The test solutions were kept at 37°C for 30 min and then analyzed using spectrophotometry.
Cells were co-incubated with the probe in 10 mM TBS (pH 7.4). Cytotoxicity was assessed using the MTS assay. Cells were seeded in a 25-mm glass-bottom dish and grown for 24 h to approximately 85% confluency, followed by the live-cell confocal imaging experiments, for which the cells were divided into three groups as follows. The first group included HeLa cells that were incubated with 5 μM CS-ALP for 30 min. The cells were then washed with TBS buffer before imaging. In the second group, after pretreatment with Na3VO4 for 4 h, HeLa cells were incubated with 5 μM CS-ALP for 0.5 h and then washed with TBS prior to imaging. The third group included control cells without CS-ALP. For the cisplatin-induced nephrotoxicity model in HK-2 cells, the experiment was divided into four groups. The first group included HK-2 cells that were treated with 5 μM CS-ALP. The second and third groups included HK-2 cells that were incubated with cisplatin, 500 μM or 1000 μM, for 4 h, respectively, and then co-incubated with 5 μM CS-ALP for 0.5 h. The fourth group included cells that were pre-incubated with 50 μM Na3VO4 in the presence of cisplatin (1 mM) for 4 h and then treated with 5 μM CS-ALP for 0.5 h. Confocal fluorescence imaging of cells was performed using an Olympus FV1000 laser confocal microscope (Japan).
The drug-induced AKI mouse model was constructed following methods detailed in our previous report. Liu et al. (2020a) cisplatin was dissolved in 0.9% saline. BALB/c mice were intraperitoneally preinjected with cisplatin (20 mg/kg) for 24 h or 48 h. Live cells and live mouse experiments were in agreement with institutional animal care and use regulations, according to protocol No. SYXK (Xiang) 2022-0007, approved by Laboratory Animal Center of Hunan.
Six BALB/c mice, with average initial body weight of 20 g, were fed in a metabolic cage after intraperitoneal preinjection of cisplatin (20 mg/kg), with urine samples collected at 24 h and 48 h after injection. Blood samples were drawn via the retro-orbital sinus at 24 h and 48 h after intraperitoneal administration of cisplatin. the samples were then tested immediately by adding 50 or 100 μL of the urine or blood sample to the test solution (total volume 1 ml) followed by recording of the fluorescence emission spectrum. The urine and blood of heathy mice were also tested as controls.
The 4-MUP probe was purchased from Sigma. The ALP level in urine and blood samples was then determined by measuring the fluorescence emission peak at 450 nm with λex at 360 nm using a 4-MUP probe.
After intraperitoneal administration of cisplatin (20 mg/kg) for 24 h or 48 h, the CS-ALP probe (0.15 mg/kg in 20% DMSO/saline solution) was intravenously injected. The probe could not be completely solubilized in water at such a high concentration, so DMSO was added to improve solubility. Next, in vivo NIRF and PA imaging were carried out.
Drug-induced AKI is accompanied by oxidative stress. Many studies have indicated that high levels of ROS are generated by cells under oxidative stress (Mao et al., 2017; Mao et al., 2022). Thus, the probe used for imaging of AKI must be chemically stable in the presence of ROS. As proof of concept, we first studied the reactivity of the CS dye with typical oxidants and nucleophiles, including H2S, Cys, GSH, H2O2, O2•−, •OH, ONOO−, and ClO−. As exhibited in Figure 1, the absorption intensity of CS at 685 nm remained unchanged with the addition of test species at physiological concentrations. In contrast, seven other kinds of NIR dyes, five of which (dyes 1–5) have been used for constructing NIR ALP fluorescent probes (Li et al., 2012; Li et al., 2017; Zhang P. et al., 2019; Zhang Q. et al., 2019; Gao et al., 2019; Wang et al., 2021b), were also tested. The results indicated that dyes 1–7 were not stable in the presence of O2•−, •OH, ONOO−, and ClO−. These ROS have been shown to increase in concentration during drug-induced AKI (Lv et al., 2018; Huang et al., 2020). The results demonstrated that the existing NIRF ALP probes are not suitable for in vivo imaging and measurement of ALP levels in the drug-induced AKI model. In addition, for AKI in vivo imaging, probes must be water soluble at low concentration to allow enrichment in the kidney. We generated the probe through phosphorylation of the hydroxyl group of the CS fluorophore. The log P values of the probe and CS dye were 2.238 and 3.644, respectively, under pH 8.0 buffer conditions; as a control, the log P value of rhodamine B, a well know water-soluble dye, was calculated to be 2.032 under the experimental condition. The log P values indicated that CS-ALP has good water solubility and the potential for use in imaging to assess renal metabolism. To our knowledge, CS-ALP is the first molecular probe suitable for NIRF/PA bimodal in vivo detection of ALP in drug-induced AKI. The synthesis methodology for CS-ALP is shown in Supplementary Scheme S1, with structures assessed by 1H NMR and MS (ESI).
FIGURE 1. (A) Structure of hydroxyl containing NIR dyes 1–7 and the CS dye; dyes 1–5 were used for the constructed NIR ALP fluorescent probes. (B) Absorption intensities of the aforementioned dyes (5 μM) compared to various agents in an aqueous solution (PBS/EtOH = 8/2, pH 7.4). The absorption intensity of free dye (5 µM) was defined as 1.0. The tested solution was kept at room temperature for 0.5 h before analysis and data collection.
After synthesizing the probe, we conducted a spectral study of the reaction between CS-ALP and ALP. CS-ALP showed a maximum absorption peak at 600 nm. After addition of ALP, a new absorption peak appeared at 685 nm. The increased absorption intensity displayed a good linear relationship with the activity of ALP at 0–10 U/L (Figure 2A, and Supplementary Figure S1, ESI). Concomitantly, the fluorescence emission peak at 716 nm grew dramatically upon increased ALP activity (Figure 2B). According to the fluorescence titration experiment, the fluorescence intensity at 716 nm exhibited a good linear relationship with ALP at 0–10 U/L (Figure 2C), with a calculated detection limit of 0.26 U/L. The catalytic activity of the probe in response to ALP was then assessed. Figure 2D depicts the fluorescence dynamics of the reaction of the probe to ALP at different concentrations (0, 2, 20, and 40 U/L). The results indicate that high-activity ALP allows the probe to crack quickly, resulting in a dramatic increase in fluorescence intensity. The fluorescence intensity of the reaction system is essentially balanced at about 26 min. At the same time, in the absence of ALP, there was no significant change in the fluorescence intensity of the reaction system (Supplementary Figure S2, ESI), and the photo-stability of the probe and the CS dye is good (Supplementary Figure S3). Together, these results indicate that CS-ALP is stable at physiological pH and could be applied in bioimaging.
FIGURE 2. (A) Absorption and (B) emission spectra of 5 μM CS-ALP in the presence of different concentrations of ALP. (C) Calibration curves. (D) Fluorescence intensity at 716 nm vs. the reaction time with varying levels of ALP activity. The tests were carried out in aqueous solution (5% DMSO TBS buffer solution, pH 8.0) after incubation at 37°C for 0.5 h. λex = 680 nm.
To further demonstrate the specificity of the reaction between CS-ALP and the enzyme, the specificity of the probe for various potentially biologically relevant substances was assessed. As shown in Supplementary Figure S4, the signal intensity is not significantly affected by other biological interference. Na3VO4, a commonly used and competitive phosphatase inhibitor (Liu et al., 2017), was then co-incubated with ALP for 30 min before addition of the probe to the test solution. As shown in Supplementary Figure S5, addition of 50 μM Na3VO4 resulted in a significant reduction in fluorescence intensity. These results confirm that ALP-induced phosphate group-specific cleavage of the probe contributes to the enhancement of fluorescence intensity. Next, the effect of pH on CS-ALP and the enzymatic reaction was also evaluated, with results demonstrating that CS-ALP could perform well at physiological pH (Supplementary Figure S6).
The detectability of CS-ALP using PA imaging was then tested. We first tested the PA spectra for CS-ALP in the presence of varied ALP activity. The results showed that it was generally consistent with its absorption spectra. As displayed in Figure 3A, the PA signal intensity at 680 nm (PA680) exhibited 1.8-fold enhancement at the saturation point. PA680 images of CS-ALP were obtained (Figure 3B) by treating the probe with increasing concentrations of ALP (0–30 U/L). The PA680 signals displayed linear responses to ALP in the 0–30 U/L concentration range, with a calculated detection limit of 2.5 U/L (Figure 3C).
FIGURE 3. (A) PA spectra of 5 μM CS-ALP in the presence or absence of 40 U/L ALP. (B) PA680 images of CS-ALP (5 μM) in the presence of different concentrations of ALP (0, 10, 20, 30, 40, and 50 U/L). (C) Plot of PA680 intensity vs. ALP concentration.
The cytotoxicity of CS-ALP was first assessed using the MTS assay. The results showed that high cell viability was maintained after co-incubation with 2–10 μM probe, indicating that CS-ALP had good biocompatibility (Supplementary Figure S7). Next, we used HeLa cells for co-incubation with the probe for live-cell imaging experiments because ALP is overexpressed in HeLa cells. As shown in Supplementary Figure S8, when excited at 635 nm, HeLa cells showed bright fluorescence. However, when pretreated with Na3VO4 before co-incubation with CS-ALP, HeLa cells showed negligible fluorescence. The results demonstrate that the signal enhancement in HeLa cells is due to ALP activity. We then constructed an AKI cell model using cisplatin, a drug known to induce AKI. HK2 cells treated with CS-ALP alone were excited at 635 nm and showed virtually no fluorescence (Figure 4A). At the same time, we observed that, when pretreating HK2 cells with 0.5 mM or 1 mM cisplatin, signal variations indicated cisplatin dose dependence (Figures 4B,C) and the fluorescence intensity ratio increased by 2.6 and 3.4 times, respectively (Figure 4E). Furthermore, when the cisplatin-treated HK2 cells were co-incubated with Na3VO4 for 30 min before addition of CS-ALP, there was almost no fluorescence (Figure 4D). These results suggest that CS-ALP could be used for measurement of endogenous ALP in cisplatin-treated HK2 cells, and that the activity of ALP is directly related to the process of cisplatin-induced AKI.
FIGURE 4. Images of HK-2 cells pretreated with (A) 0 mM, (B) 0.5 mM, and (C) 1 mM cisplatin for 4 h, and then incubated with 5 μM probe for 30 min. (D) Images of HK-2 cells incubated with 1 mM cisplatin and 50 μM Na3VO4 for 4 h, and then incubated with 5 μM CS-ALP for 30 min. (E) Normalized average signal intensity in the aforementioned images A–D. λex = 635 nm, λem = 680−750 nm, scale bar: 20 μm.
The AKI mouse model was established by intraperitoneal injection of 20 mg/kg cisplatin or normal saline as control. We first tested the urine samples of mice and found that the signal intensity from mice treated with cisplatin for 24 h and 48 h was 1.51-fold and 2.23-fold higher, respectively, than that of the control group. Blood sample analysis showed limited signal increase in the experimental groups compared to control. This result is consistent with that observed when using the commercially available ALP probe 4-MUP (Figure 5). These results are also consistent with a previous study showing that ALP was discharged into the urine following cellular injury due to early stage AKI (Charlton et al., 2014). According to the fluorescence correction curve for CS-ALP to ALP, the calculated ALP activity levels in the urine of AKI mice were 30.1 U/L (cisplatin-treated 24 h) and 45.6 U/L (cisplatin-treated 48 h), respectively. Thus, the CS-ALP probe may be an effective tool for early detection of AKI.
FIGURE 5. Using CS-ALP/4-MUP to test the ALP activity in urine and blood samples. The fluorescence intensity of CS-ALP/4-MUP at 716 nm and 450 nm in mouse urine samples (A) and blood samples (B) excreted by healthy and AKI mice. The mouse urine samples were obtained using metabolic cages, and the blood samples were obtained through retro-orbital sampling. The 50 or 100 μL urine or blood samples were immediately added to the test solution (total volume 1 ml). Fluorescence intensity in the healthy mouse was defined as 1.0.
After successful NIRF imaging of ALP in the AKI cell model, we applied CS-ALP to in vivo bimodal NIRF/PA imaging using commercial in vivo imaging systems. First, we constructed an AKI mouse model according to our previous protocol (Liu H.-W. et al., 2020). The biodistribution of CS-ALP after intravenous (i.v.) injection showed that CS-ALP remained inactive in the control mouse but was activated in the AKI model mouse (Supplementary Figure S9A). In another group, imaging of isolated organs of the AKI mouse at 1.5 h post injection showed that CS-ALP accumulated primarily in the kidneys and liver (Supplementary Figure S9B), indicating that cisplatin may induce liver injury and kidney injury at the same time (Palipoch and Punsawad, 2013). As mentioned earlier, the log P value of CS-ALP is 2.238, which indicates that the probe has good water solubility and shows the potential for use in imaging to assess renal metabolism. Subsequently, 0.15 mg/kg CS-ALP was intravenously administered at different cisplatin post-treatment time points (24, 48, and 72 h). Real-time in vivo imaging showed that CS-ALP could be rapidly activated in the kidneys at 10 min post injection and that the signal intensity was stable at 10–30 min, followed by an obvious decrease at 120 min post injection (Supplementary Figure S10). Therefore, we timed the in vivo NIRF imaging to be at 30 min after injection of the probe. As displayed in Figures 6A,B, the NIRF signal intensity gradually enhanced, mainly in the kidneys of mice treated with cisplatin for 24 h or 48 h. The signal ratios for the 24 h and 48 h groups were 3.52-fold and 3.92-fold higher, respectively, than that of control group, indicating that ALP was upregulated in the kidneys of cisplatin-treated mice. We also carried out PA imaging, and the results are shown in Figure 6C and Supplementary Figure S11. The PA imaging data indicate that the kidney regions of the control group (treated with normal saline) were low contrast at 1 h post injection of CS-ALP, while the PA signal in the kidney regions of cisplatin-treated mice gradually increased. The average signal intensity is displayed in Figure 6D, which indicates that the probe could be used for semi-quantitative analysis of ALP in drug-induced AKI. The PA imaging results were consistent with the NIRF imaging results, but the in vivo PA imaging required a longer time to obtain high contrast results compared to NIRF imaging due to the high background signal with PA imaging in this region, hence the advantage of dual-mode imaging for acquisition of more reliable in vivo imaging results (Luo et al., 2020; Zhang et al., 2020). Hematoxylin–eosin (H&E) staining revealed normal tubular morphology in the control group, compared to widely spaced tubules, damage to the brush border, and formation of hyaline casts within the damaged tubules in the experimental group 24 h after treatment with cisplatin (Supplementary Figure S12). Considering these results, we conclude that upregulation of ALP expression will occur at the early stages of AKI and will continue to be expressed with the progression of AKI. Additionally, we demonstrated the development and validation of use of CS-ALP in NIRF/PA bimodal imaging as a tool for evaluating drug-induced AKI in response to ALP.
FIGURE 6. (A) In vivo imaging of a mouse after treatment with saline or cisplatin (20 mg/kg) for 24 h or 48 h, followed by injection (i.v.) of 0.15 mg/kg CS-ALP (in DMSO/DPBS, 1:4 v/v) for 20 min. Excitation filter 640, emission filter ICG. (B) Mean intensity ratio in (A). (C) PA680 nm images from the kidneys of mice after pre-injection (i.p.) with saline or cisplatin (20 mg/kg) for 24 h or 48 h, followed by injection (i.v.) of 0.15 mg/kg CS-ALP (in DMSO/DPBS, 4:1 v/v) for 1 h. (D) Mean intensity ratio in (C). Values are the mean ± SD, n = 3.
In summary, we constructed a NIRF/PA bimodal sensor CS-ALP for the detection and imaging of ALP in cisplatin-induced AKI that has high sensitivity and specificity both in vitro and in vivo. The probe is chemically stable in the presence of ROS at physiological concentrations and allows high-fidelity imaging results. Upon addition and with increasing activity of ALP, the absorption intensity of the probe at 685 nm gradually increased and was accompanied by a NIRF enhancement with a peak at 716 nm, which were used for “turn-on” PA and NIRF imaging, respectively. According to the fluorescence titration experiment, the calculated detection limit was 0.26 U/L. CS-ALP was used in the imaging of ALP in HK2 cells after stimulation by cisplatin. Furthermore, CS-ALP is water-soluble and showed good renal-targeting capability for NIRF/PA bimodal imaging of ALP in a cisplatin-induced AKI mouse model. The results also indicate that upregulated expression of ALP occurs at early stages of AKI and continues with the progression of AKI. Our work in development of the CS-ALP bimodal molecular probe will not only facilitate mechanistic study of the roles of ALP in drug-induced AKI but may also assist in early diagnosis of AKI.
The original contributions presented in the study are included in the article/Supplementary Material; further inquiries can be directed to the corresponding authors.
The animal study was reviewed and approved by No. SYXK (Xiang) 2022-0007, approved by Laboratory Animal Center of Hunan.
XC: Investigation, methodology, data curation, and writing—original draft. ZY: Offered much help in the process of experiments. KC: Review and editing. HL: Funding acquisition. HL: Data curation, conceptualization, supervision, funding acquisition, and writing—review and editing.
This work was supported by the National Natural Science Foundation of China (Grant 22104036); the Project of Improving the Diagnosis and Treatment Capacity of Hepatobiliary, Pancreas and Intestine Diseases in Hunan Province (Xiangwei [2019] No. 118); the Natural Science Foundation of Hunan Province (2022JJ40216); the Natural Science Foundation of Changsha City (kq2202447); and the Postgraduate Scientific Research Innovation Project of Hunan Province (CX20190341).
The authors declare that the research was conducted in the absence of any commercial or financial relationships that could be construed as a potential conflict of interest.
All claims expressed in this article are solely those of the authors and do not necessarily represent those of their affiliated organizations, or those of the publisher, the editors, and the reviewers. Any product that may be evaluated in this article, or claim that may be made by its manufacturer, is not guaranteed or endorsed by the publisher.
The Supplementary Material for this article can be found online at: https://www.frontiersin.org/articles/10.3389/fbioe.2022.1068533/full#supplementary-material
Blatt, A. E., and Liebman, S. E. (2013). Drug induced acute kidney injury. Hosp. Med. Clin. 2, e525–e541. doi:10.1016/j.ehmc.2013.04.003
Charlton, J. R., Portilla, D., and Okusa, M. D. (2014). A basic science view of acute kidney injury biomarkers. Nephrol. Dial. Transpl. 29, 1301–1311. doi:10.1093/ndt/gft510
Chen, Y., Pei, P., Lei, Z., Zhang, X., Yin, D., and Zhang, F. (2021). A promising NIR-II fluorescent sensor for peptide-mediated long-term monitoring of kidney dysfunction. Angew. Chem. Int. Ed. Engl. 60, 15943–15949. doi:10.1002/ange.202103071
Cheng, D., Peng, J., Lv, Y., Su, D., Liu, D., Chen, M., et al. (2019). De novo design of chemical stability near-infrared molecular probes for high-fidelity hepatotoxicity evaluation in vivo. J. Am. Chem. Soc. 141, 6352–6361. doi:10.1021/jacs.9b01374
Cheng, D., Xu, W., Gong, X., Yuan, L., and Zhang, X.-B. (2021). Design strategy of fluorescent probes for live drug-induced acute liver injury imaging. Acc. Chem. Res. 54, 403–415. doi:10.1021/acs.accounts.0c00646
Cheng, P., Miao, Q., Huang, J., Li, J., and Pu, K. (2020). Multiplex optical urinalysis for early detection of drug-induced kidney injury. Anal. Chem. 92, 6166–6172. doi:10.1021/acs.analchem.0c00989
Du, B., Chong, Y., Jiang, X., Yu, M., Lo, U.-G., Dang, A., et al. (2021). Hyperfluorescence imaging of kidney cancer enabled by renal secretion pathway dependent efflux transport. Angew. Chem. Int. Ed. Engl. 60, 355–363. doi:10.1002/ange.202010187
Gao, X., Ma, G., Jiang, C., Zeng, L., Jiang, S., Huang, P., et al. (2019). In vivo near-infrared fluorescence and photoacoustic dual-modal imaging of endogenous alkaline phosphatase. Anal. Chem. 91, 7112–7117. doi:10.1021/acs.analchem.9b00109
Hsu, R. K., McCulloch, C. E., Dudley, R. A., Lo, L. J., and Hsu, C.-y. (2013). Temporal changes in incidence of dialysis-requiring AKI. J. Am. Soc. Nephrol. 24, 37–42. doi:10.1681/asn.2012080800
Huang, J., Huang, J., Cheng, P., Jiang, Y., and Pu, K. (2020). Near-infrared chemiluminescent reporters for in vivo imaging of reactive oxygen and nitrogen species in kidneys. Adv. Funct. Mat. 30, 2003628. doi:10.1002/adfm.202003628
Huang, J., Lyu, Y., Li, J., Cheng, P., Jiang, Y., and Pu, K. (2019). A renal-clearable duplex optical reporter for real-time imaging of contrast-induced acute kidney injury. Angew. Chem. Int. Ed. Engl. 58, 17960–17968. doi:10.1002/ange.201910137
Huang, J., and Pu, K. (2021). Near-infrared fluorescent molecular probes for imaging and diagnosis of nephro-urological diseases. Chem. Sci. 12, 3379–3392. doi:10.1039/d0sc02925d
Huang, J., Weinfurter, S., Daniele, C., Perciaccante, R., Federica, R., Della Ciana, L., et al. (2017). Zwitterionic near infrared fluorescent agents for noninvasive real-time transcutaneous assessment of kidney function. Chem. Sci. 8, 2652–2660. doi:10.1039/c6sc05059j
Jia, X., Chen, Q., Yang, Y., Tang, Y., Wang, R., Xu, Y., et al. (2016). FRET-Based mito-specific fluorescent probe for ratiometric detection and imaging of endogenous peroxynitrite: Dyad of Cy3 and Cy5. J. Am. Chem. Soc. 138, 10778–10781. doi:10.1021/jacs.6b06398
Li, L., Ge, J., Wu, H., Xu, Q.-H., and Yao, S. Q. (2012). Organelle-specific detection of phosphatase activities with two-photon fluorogenic probes in cells and tissues. J. Am. Chem. Soc. 134, 12157–12167. doi:10.1021/ja3036256
Li, S.-J., Li, C.-Y., Li, Y.-F., Fei, J., Wu, P., Yang, B., et al. (2017). Facile and sensitive near-infrared fluorescence probe for the detection of endogenous alkaline phosphatase activity in vivo. Anal. Chem. 89, 6854–6860. doi:10.1021/acs.analchem.7b01351
Li, W., Shen, Y., Gong, X., Zhang, X.-B., and Yuan, L. (2021). Highly selective fluorescent probe design for visualizing hepatic hydrogen sulfide in the pathological progression of nonalcoholic fatty liver. Anal. Chem. 93, 16673–16682. doi:10.1021/acs.analchem.1c04246
Liu, H.-W., Chen, L., Xu, C., Li, Z., Zhang, H., Zhang, X.-B., et al. (2018). Recent progresses in small-molecule enzymatic fluorescent probes for cancer imaging. Chem. Soc. Rev. 47, 7140–7180. doi:10.1039/c7cs00862g
Liu, H.-W., Li, K., Hu, X.-X., Zhu, L., Rong, Q., Liu, Y., et al. (2017). In situ localization of enzyme activity in live cells by a molecular probe releasing a precipitating fluorochrome. Angew. Chem. Int. Ed. Engl. 56, 11950–11954. doi:10.1002/ange.201705747
Liu, H.-W., Zhang, H., Lou, X., Teng, L., Yuan, J., Yuan, L., et al. (2020a). Imaging of peroxynitrite in drug-induced acute kidney injury with a near-infrared fluorescence and photoacoustic dual-modal molecular probe. Chem. Commun. 56, 8103–8106. doi:10.1039/d0cc01621g
Liu, L., Jiang, L., Yuan, W., Liu, Z., Liu, D., Wei, P., et al. (2020b). Dual-modality detection of early-stage drug-induced acute kidney injury by an activatable probe. ACS Sens. 5, 2457–2466. doi:10.1021/acssensors.0c00640
Luo, X., Chen, M., and Yang, Q. (2020). Research progress on near infrared II technology for in vivo imaging. Acta Chim. Sin. 78, 373. doi:10.6023/a20020045
Lv, Y., Dan, C., Dongdong, S., Chen, M., Yin, B.-C., Yuan, L., et al. (2018). Visualization of oxidative injury in the mouse kidney using selective superoxide anion fluorescent probes. Chem. Sci. 9, 7606–7613. doi:10.1039/c8sc03308k
Mao, Z., Jiang, H., Li, Z., Zhong, C., Zhang, W., and Liu, Z. (2017). An N-nitrosation reactivity-based two-photon fluorescent probe for the specific in situ detection of nitric oxide. Chem. Sci. 8, 4533–4538. doi:10.1039/c7sc00416h
Mao, Z., Xiong, J., Wang, P., An, J., Zhang, F., Liu, Z., et al. (2022). Activity-based fluorescence probes for pathophysiological peroxynitrite fluxes. Coord. Chem. Rev. 454, 214356. doi:10.1016/j.ccr.2021.214356
Orimo, H. (2010). The mechanism of mineralization and the role of alkaline phosphatase in health and disease. J. Nippon. Med. Sch. 77, 4–12. doi:10.1272/jnms.77.4
Palipoch, S., and Punsawad, C. (2013). Biochemical and histological study of rat liver and kidney injury induced by cisplatin. J. Toxicol. Pathol. 26, 293–299. doi:10.1293/tox.26.293
Tan, J., Yin, K., Ouyang, Z., Wang, R., Pan, H., Wang, Z., et al. (2021). Real-time monitoring renal impairment due to drug-induced AKI and diabetes-caused CKD using an NAG-activatable NIR-II nanoprobe. Anal. Chem. 93, 16158–16165. doi:10.1021/acs.analchem.1c03926
Wang, W.-X., Jiang, W.-L., Guo, H., Li, Y., and Li, C.-Y. (2021b). Real-time imaging of alkaline phosphatase activity of diabetes in mice via a near-infrared fluorescent probe. Chem. Commun. 57, 480–483. doi:10.1039/d0cc07292c
Yang, C., Wang, H., Yokomizo, S., Hickey, M., Chang, H., Kang, H., et al. (2021). ZW800-PEG: A renal clearable zwitterionic near-infrared fluorophore for potential clinical translation. Angew. Chem. Int. Ed. Engl. 60, 13966–13971. doi:10.1002/ange.202102640
Zhang, P., Fu, C., Zhang, Q., Li, S., and Ding, C. (2019a). Ratiometric fluorescent strategy for localizing alkaline phosphatase activity in mitochondria based on the ESIPT process. Anal. Chem. 91, 12377–12383. doi:10.1021/acs.analchem.9b02917
Zhang, Q., Li, S., Fu, C., Xiao, Y., Zhang, P., and Ding, C. (2019b). Near-infrared mito-specific fluorescent probe for ratiometric detection and imaging of alkaline phosphatase activity with high sensitivity. J. Mat. Chem. B 7, 443–450. doi:10.1039/c8tb02799d
Zhang, Y., Wang, Y., Yang, X., Yang, Q., Li, J., and Tan, W. (2020). Polyaniline nanovesicles for photoacoustic imaging-guided photothermal-chemo synergistic therapy in the second near-infrared window. Small 16, 2001177. doi:10.1002/smll.202001177
Keywords: oxidative stress, acute kidney injury, alkaline phosphatase, fluorescent probe, photoacoustic (optoacoustic) imaging
Citation: Chen X, Yuwen Z, Zhao Y, Li H, Chen K and Liu H (2022) In situ detection of alkaline phosphatase in a cisplatin-induced acute kidney injury model with a fluorescent/photoacoustic bimodal molecular probe. Front. Bioeng. Biotechnol. 10:1068533. doi: 10.3389/fbioe.2022.1068533
Received: 13 October 2022; Accepted: 01 November 2022;
Published: 23 November 2022.
Edited by:
Hua Chen, Guangxi Normal University, ChinaCopyright © 2022 Chen, Yuwen, Zhao, Li, Chen and Liu. This is an open-access article distributed under the terms of the Creative Commons Attribution License (CC BY). The use, distribution or reproduction in other forums is permitted, provided the original author(s) and the copyright owner(s) are credited and that the original publication in this journal is cited, in accordance with accepted academic practice. No use, distribution or reproduction is permitted which does not comply with these terms.
*Correspondence: Hongwen Liu, bGl1aG9uZ3dlbkBobnUuZWR1LmNu; Kang Chen, Y2hlbmthbmdAaHVubnUuZWR1LmNu; Haixia Li, NDcyMTc2MDYxQHFxLmNvbQ==
Disclaimer: All claims expressed in this article are solely those of the authors and do not necessarily represent those of their affiliated organizations, or those of the publisher, the editors and the reviewers. Any product that may be evaluated in this article or claim that may be made by its manufacturer is not guaranteed or endorsed by the publisher.
Research integrity at Frontiers
Learn more about the work of our research integrity team to safeguard the quality of each article we publish.