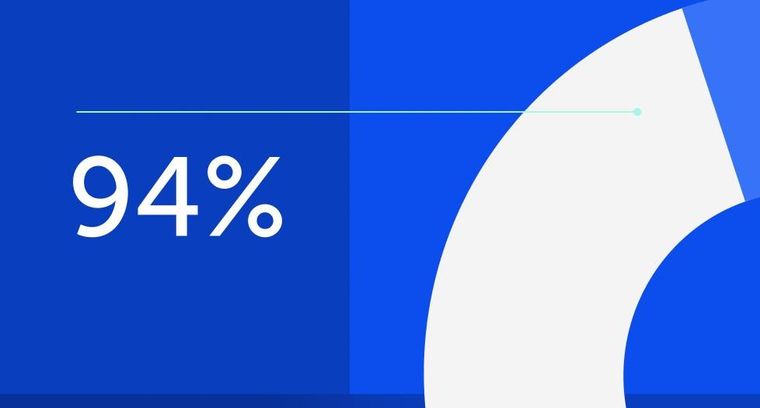
94% of researchers rate our articles as excellent or good
Learn more about the work of our research integrity team to safeguard the quality of each article we publish.
Find out more
ORIGINAL RESEARCH article
Front. Bioeng. Biotechnol., 10 November 2022
Sec. Biomaterials
Volume 10 - 2022 | https://doi.org/10.3389/fbioe.2022.1060467
This article is part of the Research TopicAdvances in Smart Bioactive Surfaces for Wounding Healing and Tissue RepairView all 5 articles
Insufficient oxygen supply at the tumor site and hypoxia caused during tumor treatment lead to a poor therapeutic effect and poor prognosis. Therefore, effectively overcoming the problem of hypoxia in tumors and avoiding hypoxia that compromises the efficacy of the treatment could improve the anti-tumor therapeutic effect. Thus, this study reports the ability of W18O49@EP nanoparticles to release reactive oxygen species (ROS) during the combined tumor radiotherapy (RT) and photodynamic therapy (PDT). The release of ROS by the nanoparticles during near infrared light (NIR) irradiation was demonstrated by in vitro and in vivo experiments, realizing an effective PDT without inducing hypoxia. Indeed, the ROS did not derive from the oxygen in the tumor microenvironment but they were released by the nanoparticles. Thus, ROS could improve the therapeutic effect of RT avoiding the problem of hypoxia after RT. Hence, W18O49@EP nanoparticles greatly improved the anti-tumor effect due to their effectiveness despite the insufficient oxygen supply and hypoxia caused by traditional RT and PDT.
Radiotherapy (RT) is an effective approach in the treatment of tumors, and more than 50% of all cancer patients receive RT (Allen et al., 2017). RT potently kills cells mainly through the damage of DNA by ionization (Atun et al., 2015; De Ruysscher et al., 2019). RT also generates reactive oxygen species (ROS), thereby indirectly leading to DNA damage (De Ruysscher et al., 2019). Another significant effect of ROS is that they trigger apoptosis by inducing stress responses in the subcellular organelles, such as mitochondria and the endoplasmic reticulum, which effectively enhance the cell-killing effect of RT (Rey et al., 2017). However, tumor cells have a strong self-regulation ability of detoxify and eliminate the effect of ROS through the transcription of antioxidant enzymes when the level of ROS is relatively low (Kim et al., 2019). The apoptotic process is activated only when the ROS levels are high enough to inhibit the self-regulation of the cells, thus killing the abnormal cells. Unfortunately, the excessive production of ROS leads to hypoxia, which is the main factor leading of the resistance of tumor cells to RT, consequently causing a poor clinical outcome (Kim et al., 2019; Liu et al., 2020). Tumor cells that survive RT are transformed to a radioresistant phenotype, with an increased ability of invasion and metastasis (Kim et al., 2014; Liu et al., 2017). Therefore, clinical methods that increase ROS levels without causing hypoxia or alleviating hypoxia during RT, could greatly improve the therapeutic outcomes of RT.
Hypoxia is as an important characteristic of the tumor microenvironment caused by the consumption of oxygen by the rapidly growing tumor cells and insufficient oxygen supply (Peter and Arnulf, 2007; Masuda and Belmonte, 2013). Treatments that produce ROS and rely on ROS to be effective, such as RT or photodynamic therapy (PDT), can also cause hypoxia. Hypoxia markedly reduces the therapeutic effect of the treatments mentioned above (Peter and Arnulf, 2007; Yao et al., 2017); therefore, a series of measures have been explored to reverse or reduce the hypoxic environment (Huo et al., 2019). The main strategies involve the direct supply of oxygen (Xie et al., 2019), in situ production of oxygen (Chen et al., 2016), and the inhibition of oxygen consumption (Xia et al., 2019). Although these methods reduce the limitation of hypoxia on the therapeutic effect to some extent, they increase the complexity of the system, and the uncertainty of the treatment. A smarter system that not only reduces hypoxia during the treatment process but also enhances the treatment effect through adjuvant therapy, is of utmost importance. Thus, an effective tumor treatment should be explored in this direction.
Avoiding oxygen consumption by carrying oxygen (Cheng et al., 2015; Chen et al., 2017) or carrying ROS releasing groups (Mahmoud Asadirad et al., 2013; Kolemen et al., 2016) in the tumor microenvironment is an effective approach to overcome the insufficient oxygen supply at the tumor site and hypoxia caused during PDT. The release of ROS could not only overcome the limitation of hypoxia on PDT, but also solve the problem of insufficient in vivo penetration of PDT, since it is excited by visible light (Muhammad Idris et al., 2012).
This work reports a combination of photothermal effect and RT that effectively reduced the hypoxic microenvironment of the tumor. W18O49 with dual effects of photothermal effect and RT sensitization was synthesized, and then the anthracene endoperoxide derivative compound (abbreviated as EP) that releases singlet oxygen in a thermal-controlled manner was covalently connected to it (Mahmoud Asadirad et al., 2013). As shown in Scheme 1, this system (named as W18O49@EP) has multiple advantages. First, it combined PDT with RT. Secondly, the ROS released by the photothermal effect was converted into oxygen in vivo to reduce the hypoxic microenvironment of the tumor. Thirdly, the singlet oxygen released by the photothermal effect synergistically acted with the ROS produced by RT to enhance the effect of ROS on inducing apoptosis. Finally, ROS were induced by NIR, which has a better penetration depth than visible light, the therapeutic effect on the tumor was enhanced through these multiple effects and an effective therapeutic protocol could be considered for an effective tumor therapy.
Tungsten hexachloride (WCl6), poly acrylic acid (PAA, Mw = 2000 D), diethylene glycol (DEG), ethyl dimethylaminopropyl carbodiimide (EDC) and sulfo-NHS were obtained from Sigma Aldrich (St. Louis, MO, United States). Methyl 4-(4,4,5,5-tetramethyl-1,3,2-dioxaborolan-2-yl)benzoate, methylene blue, 9-bromo-10-phenylanthracene, and H2N-PEG-OH (MW: 3,000 g/mol) were purchased from Sigma-Aldrich. All chemicals were analytically pure and used as purchased without further purification, unless otherwise noted.
W18O49 NPs were prepared according to a procedure previously reported (Huo et al., 2014). Briefly, 500 mg WCl6 and 200 mg PAA were dissolved in 100 ml DEG. The mixture was stirred under the protection of N2 to fully dissolve the substances, and then placed at 180°C for approximately 30 min to allow the reaction. When the solution turned colorless to dark green, it was allowed to cool to room temperature. Subsequently, the mixture was centrifuged, the supernatant was removed and the pellet was dissolved in 50 ml deionized water. This procedure was repeated three times.
Compound 3 was prepared according to a method previously reported (Mahmoud Asadirad et al., 2013; Kolemen et al., 2016). Next, compound 3 (50 mg, 0.12 mmol) and H2N-PEG-OH (292 mg, 0.086 mmol, MW: 3,000 g/mol) were dissolved in 5 ml dry tetrahydrofuran. N,N′-dicyclohexylcarbodiimide (26 mg, 0.12 mmol) and 4-dimethylaminopyridine (11 mg, 0.15 mmol) were added to the solution. The reaction mixture was stirred for 2 h until the formation of a precipitate. The precipitate was filtered and removed, and cold diethyl ether was added to the solution to precipitate the product (EP). Pure EP was filtered (187.1 mg, 42%).
W18O49 (50 mg) NPs dissolved in PBS (10 ml) were activated with EDC (100 mg) and sulfo-NHS (50 mg). Subsequently, EP (75 mg) was added into the above solution. The mixture was allowed to react for 10 h at 4°C under vigorous stirring. Then, the as-obtained W18O49@EP NPs were harvested by three cycles of centrifugation and washing with PBS to remove the unreacted materials and excess of the reagents. Purified W18O49@EP NPs were re-suspended in PBS and stored at 4°C for further application.
Images from transmission electron microscopy (TEM) were obtained using JEOL JEM-2010 (HR). Absorption spectra were recorded using a UV-Vis spectrophotometer (Persee DU1900, Beijing, China). The hydrodynamic particle size was characterized using dynamic light scattering (DLS) by ZetaSizer Nano-ZS90 (Malvern Instrument). The X-ray diffraction (XRD) pattern was recorded in the 2θ range of 20–60°.
Human gastric carcinoma cells (MNK cells) were routinely cultured in Dulbecco’s Modified Eagle Medium (DMEM) at 37 and 5% CO2. Subsequently, they were cultured in a six-well plate to evaluate the ability of W18O49@EP NPs to produce intracellular ROS. When cells reached a confluence over 80%, the medium was removed and fresh medium containing the following substances was added: 1) PBS, without any treatment, used as control; 2) H2O2 (0.1 mM) without any other treatment; 3) PBS and laser irradiation (808 nm, 0.48 W/cm2) for 5 min; 4) W18O49 NPs (0.1 mg/ml) and laser irradiation (808 nm, 0.48 W/cm2) for 5 min; 5) W18O49@EP NPs (0.1 mg/ml) and laser irradiation (808 nm, 0.48 W/cm2) for 5 min. The fluorescent probe H2DCFDA was added after 12 h and incubated for 2 h. Confocal laser microscopy was used to evaluate the fluorescence of the cells after the treatments and images were collected.
The method used for this experiment was similar to the one above but using different groups. Cells were divided into six groups and treated as follows: 1) control: without any treatment; 2) W18O49 + RT: W18O49 NPs (0.1 mg/ml) and X-ray exposure (5 Gy) for 5 min; 3) W18O49 + Laser: W18O49 NPs (0.1 mg/ml) and laser irradiation (808 nm, 0.48 W/cm2) for 5 min; 4) W18O49@EP + Laser: W18O49@EP NPs (0.1 mg/ml) and laser irradiation (808 nm, 0.48 W/cm2) for 5 min; 5) W18O49 + Laser + RT: W18O49 NPs (0.1 mg/ml) and laser irradiation (808 nm, 0.48 W/cm2) simultaneous to X-ray exposure (5 Gy) for 5 min; 6) W18O49@EP + Laser + RT: W18O49@EP NPs (0.1 mg/ml) and laser irradiation (808 nm, 0.48 W/cm2) simultaneous to X-ray exposure (5 Gy) for 5 min. Different fluorescent probes were added after 12 h and incubated for 2 h. Confocal laser microscopy was used to evaluate the fluorescence of the cells after the treatments and images were collected.
A total of female BALB/c athymic nude mice, 6–8 week old, weighing 20 g were provided by the Comparative Medical Center of Yangzhou University (Yangzhou, China) and housed in a conventional clean, specific pathogen-free (SPF) facility, under a 12-h light/dark cycle with food and water at libitum, where food, water, bedding, and cages were irradiated before use. Room temperature and humidity at ∼25°C and ∼50% were continuously monitored. All procedures using animals were approved by the animal Protection Committee of Nanjing University (Nanjing, China). Next, 2 ×106 hepatocellular carcinoma (HCC-4) cells suspended in 50 μl PBS were subcutaneously injected into the back of each mouse to establish the tumor model.
Mice were treated with 100 μL PBS, W18O49 (0.1 mg/ml), and W18O49@EP (0.1 mg/ml). The analysis was carried out at 6 h after injection using Hiscan XM Micro CT with X-ray tube settings at a current of 133 μA and a voltage of 60 kV (Suzhou Hiscan Information Technology, Jiangsu, China).
When the tumor size reached approximately 100 mm3, mice were randomly divided into six groups (n = 5 per group). The treatment of the mice in each group was identical as in the in vitro experiment described in the paragraph 2.7. The body weight of the mice was recorded, and the tumor size was measured every 2 days. The tumor volume was calculated according to the following Eq. 1:
where L is the shortest diameter of the tumor and W is the shortest diameter of the tumor.
Normal mice were intravenously (i.v.) injected with W18O49@EP NPs, sacrificed, and blood and major organs (kidney, lung, spleen, liver, and heart) were harvested at day 1, 7, and 21. They were fixed, embedded into paraffin, cut into sections, stained with H&E using a standard protocol, and visualized under light microscopy. Blood was collected in anticoagulant tubes containing sodium EDTA and separation gel were analyzed.
W18O49 NPs were synthesized according to a method previously reported (Huo et al., 2014; Huo et al., 2017). W18O49@EP NPs were synthesized as shown in Scheme 2.
The introduction of a bridging oxygen structure (compound 2–3) was confirmed by UV-Vis spectra (Figure 1A). The disappearance of the absorption peaks at 358 nm, 376 nm, and 396 nm indicated the destruction of the anthracene ring as the introduction of the bridging oxygen (Chen et al., 2017). The morphology and size of W18O49 NPs and W18O49@EP NPs were analyzed by TEM (Figures 1B,C), DLS (Figures 1B,C insert), XRD (Figure 1D) and UV-Vis spectra (Figure 1E). W18O49 NPs had good mono-dispersibility in PBS with a size of approximately 10 nm according to TEM analysis, as shown in Figure 1B. DLS analysis indicated that W18O49 NPs were fairly uniform in size: their mean hydrodynamic diameter was 7.5 nm, with a poly-dispersity index of 0.132. W18O49 NPs were confirmed by XRD and UV-Vis-NIR spectrum analysis (Figures 1D,E) through the comparison with the data presented in a previous report (Kolemen et al., 2016). The diameter of W18O49@EP NPs increased after the modification of W18O49 NPs with EP and was approximately 25 nm as determined by the TEM images. The mean hydrodynamic diameter was 43.8 nm, with a poly-dispersity index of 0.108, which was bigger than that observed by TEM due to the influence of the surface organics. The XRD and UV-Vis-NIR spectrum of W18O49@EP NPs did not change (Figures 1D,E) compared with that of W18O49 NPs. EP had thermal response ability, thereby releasing its bridging oxygen, as shown in Figure 1F. A water bath at 65°C for 10 min resulted in the reappearance of the three absorption peaks that disappeared in Figure 1A, indicating that the bridging oxygen was released under the heating conditions and compound 3 was converted to compound 2. The three absorption peaks also reappeared in W18O49@EP NPs after 10 min of irradiation with an 808 nm laser, due to the photothermal conversion ability of W18O49 NPs (Kolemen et al., 2016).
FIGURE 1. Characterization of materials. (A) UV-Vis spectra of compound 2 and 3. (B) TEM image of W18O49 NPs, (the upper right insert is its DLS result). (C) TEM image of W18O49@EP NPs, (the upper right insert is its DLS result). (D) XRD spectra of W18O49 NPs and W18O49@EP NPs. (E) UV-Vis-NIR spectra of W18O49 NPs and W18O49@EP NPs. (F) UV-Vis spectra of EP, as well as the product of EP after heating, and the product of W18O49@EP NPs after heating.
The photothermal conversion effect of W18O49@EP NPs using an 808 nm laser was evaluated to ensure the photothermal effect of W18O49@EP NPs. The in vitro results showed that the temperature of the W18O49@EP solution (1 mg/ml) rapidly increased under laser irradiation (808 nm, 0.48 W/cm2) from 27.2°C to 35.1°C in 10°s, reaching 62.3°C after 5 min (Figure 2A). Next, 0.1 ml W18O49@EP solution (10 mg/ml) was injected into the tumor of tumor-bearing mice. The temperature of the tumor changed after 30 min after laser irradiation (808 nm, 0.48 W/cm2) as monitored by a thermal imaging camera. The temperature at the tumor site increased from 31.2°C to 55.7°C within 5°min, indicating that W18O49@EP NPs possessed a photothermal therapeutic effect in vivo (Figure 2B). These results suggested that W18O49@EP NPs released enough heat under laser irradiation both in vitro and in vivo through the photothermal conversion effect, which was sufficient to release ROS by EP (Chen et al., 2017).
FIGURE 2. Photothermal conversion effect of W18O49@EP NPs. (A) Photothermal conversion effect of W18O49@EP solution (1 mg/ml) tested in a centrifuge tube; the data came from a thermal imaging camera. (B) A total of 0.1 ml W18O49@EP solution (10 mg/ml) was injected into the tumor of a tumor-bearing mouse; the data came from a thermal imaging camera. The laser irradiation was 808 nm and 0.48 W/cm2.
The above-mentioned experimental analysis showed the successful synthesis of W18O49@EP NPs, and the presence of EP did not affect the basic performance and photothermal conversion capacity of W18O49 NPs. The photothermal effect generated by W18O49 NPs was sufficient to release the loaded bridging oxygen by EP.
Since the effectiveness of PDT relies on the action of ROS (Dougherty et al., 1998; Dolmans et al., 2003), the ROS producing ability plays a decisive role in the photodynamic effect. Therefore, the ROS production ability of W18O49@EP NPs was evaluated. The intracellular ROS producing ability of W18O49@EP NPs by H2DCFDA revealed that the combination of EP and W18O49 NPs greatly increased the amount of ROS (green fluorescence) produced under laser irradiation, which enhanced the PDT effect (Figure 3). In addition, cells were not able to detoxify and eliminate ROS through their self-regulation because of the increase in ROS content, and the effect of RT on tumor killing through ROS was increased, thus greatly enhancing the RT effect.
Only RT (W18O49+X-ray) and PDT (W18O49 + Laser) could kill a small number of cells without any additional oxygen supply, and the ability to kill cells was enhanced when a combination of RT and PDT (W18O49 + Laser + X-ray) was used (Figure 4A). However, almost all cells died after RT and PDT when the sensitizer W18O49 was replaced by W18O49@EP (W18O49@EP + Laser + X-ray), suggesting that W18O49@EP exerted a very significant effect when used in a combined treatment. This result might be due to the additional effects of EP in the material. The above-mentioned comparison experiments revealed that the ROS released by EP significantly enhanced the therapeutic effect of PDT and RT.
FIGURE 4. Anti-tumor experiment in vitro. (A) Cell death after different treatments. Red: dead cells; green: living cells.(B) Intracellular ROS content and hypoxia status after different treatments. (C) HIF-1 expression in cells after different treatments. HIF-1 is marked with red fluorescence. (D) Ki67 expression in cells after different treatments. Ki67 is marked with red fluorescence. (E) Cell survival after different treatments.
A series of in vitro experiments was performed to further elucidate the enhanced therapeutic effect of W18O49@EP NPs and to explore the underlying mechanism of action for its enhanced therapeutic effect. The amount of ROS production in the cells was significantly enhanced after the treatment with W18O49@EP NPs. Since ROS production was the main reason for RDT, the increased ROS production exerted an enhanced effect, representing an important aspect for the successful effect of RT (Figure 4A). Moreover, the hypoxia status in tumor cells was greatly improved after the treatment with W18O49@EP NPs, and almost no hypoxia was observed in the “W18O49@EP + Laser” group and “W18O49@EP + Laser + X-ray” group (Figure 4B). The hypoxia status after different treatments was also evaluated by staining the important hypoxia marker hypoxia inducible factor-1α (HIF-1α) (Dachs and Tozer, 2000; Liu et al., 2017), which further indicated that the hypoxia status after the treatment with W18O49@EP NPs was greatly reduced (Figure 4C). Since hypoxia is an important limiting factor for the effect of PDT and RT, overcoming hypoxia was another factor to consider for improving the treatment effect. Taken together, the therapeutic effect of W18O49@EP NPs was greatly improved through the above two approaches.
Ki67 is an important marker of tumor progression (Scholzen and Gerdes, 2000) to prove that our treatment involves the ability to inhibit the proliferation of the tumor (Figure 4D), and the results further proved that the treatment with W18O49@EP + Laser + X-ray effectively inhibited tumor proliferation. The combined treatment group (W18O49@EP + Laser + X-ray) was the one that showed the most reduced Ki67 expression, which proved that the combination treatment group was effective in inhibiting tumor proliferation.
Next, NaN3 was used to remove ROS to further confirm that the improvement of the treatment effect in the combination treatment group was mainly caused by an increase in ROS production during the treatment due to the introduction of EP. Our results showed that the treatment effect was greatly inhibited by adding NaN3 (Figure 4E), which further proved the role of ROS production in the combination therapy of RT and PDT. Therefore, W18O49@EP NPs, which were capable of self-supplying ROS, had a significant advantage in the combination therapy of RT and PDT.
Since W18O49 NPs had a CT enhancement effect (Kolemen et al., 2016), both the W18O49 NPs and the W18O49@EP NPs were significantly enhanced at the tumor site, indicating that W18O49@EP NPs had enhanced CT angiography (Figure 5). These results also indicated that W18O49@EP NPs were enriched at the tumor site through passive targeting, thereby providing the possibility for subsequent in vivo treatment.
FIGURE 5. CT images of tumor-bearing mice after i.v. injection of 100 μL PBS, W18O49 (0.1 mg/ml) and W18O49@EP (0.1 mg/ml).
The anti-tumor effect in vivo was evaluated on HCC-4 tumor bearing mice. After the tumor volume reached 100 mm3, mice were subjected to different treatments as shown in Figure 6. The body weight of all mice did not significantly change during the treatment (Figure 6A), which indicated that the treatment was not toxic to the mice. Figure 6B shows that the tumor volume after different treatments was reduced in the combination treatment group, and the tumor almost disappeared after 2 weeks of treatment. The tumor growth was inhibited to some extent in the other treatment groups, but the effect was not significant. Figure 6C shows the morphological changes of tumor tissues in different treatment groups. We found that in the ‘‘W18O49@EP Laser + X-ray” group, there was a lot of necrosis of the tumor cells, the cells were no longer tightly connected and the nuclei were huddled together. Indicating that the combination therapy was the most effective and effectively kills tumor cells. These findings were consistent with the results of the in vitro experiments, which also indicated that W18O49@EP NPs exerted an excellent therapeutic effect through the combination therapy.
FIGURE 6. Anti-tumor effect in vivo. (A) Body weight of tumor-bearing mice after different treatments. (B) Tumor volumes of mice receiving different treatments. (C) Sections of tumor tissue after different treatments, stained by H&E.
Although W18O49@EP NPs exerted a very good therapeutic effect both in vitro and in vivo, their long-term in vivo compatibility should be evaluated prior to clinical applications. Therefore, a pathological evaluation was performed on several major organs of normal mice that received W18O49@EP NPs to test their potential cytotoxicity. The morphology of cells in these five organs was hardly affected, indicating that the above NPs did not affect these organs (Figure 7A). In general, changes in blood cells are used to measure whether the various functions in the body are affected. The results showed that the treatment with these NPs hardly affected the content of various blood cells in the body of mice, as well as the function of some organs (Figures 7B,C). Thus, these results indicated that W18O49@EP was safe and feasible for in vivo treatment.
FIGURE 7. Long-term pathological study (A) Effect of W18O49@EP NPs in the main organs by H&E staining (kidney, lung, spleen, liver, and heart). (B) Serum biochemical study and hematological assay at day 1, 7, and 21. (C) Change in blood corpuscle at day 1, 7, and 21.
In conclusion, this work demonstrated that a novel NPs could provide ROS during RT and PDT for an effective anti-tumor therapy, effectively overcoming the problem of insufficient ROS production in PDT caused by an insufficient oxygen supply in tumors. Thus, the ROS production during the treatment enhanced the therapeutic effect. The effect of ROS in RT was highlighted with the increase in ROS production, which greatly enhances the RT effect of the tumor. This treatment strategy effectively overcame the hypoxia problem during the treatment, potentially preventing the poor prognosis caused by hypoxia. Therefore, the above-mentioned strategies could greatly improve the therapeutic effect on tumors, providing an effective approach for clinical anti-tumor studies.
The raw data supporting the conclusions of this article will be made available by the authors, without undue reservation.
The animal study was reviewed and approved by the animal Protection Committee of Nanjing University. Written informed consent was obtained from the owners for the participation of their animals in this study.
JW and LH contributed to conception and design of the study, XL and SL organized the database and performed the statistical analysis, JW wrote the draft of the manuscript. All authors contributed to manuscript revision, read, and approved the submitted version.
This work was supported by the Natural Science Foundation of Jiangsu Province (BK20201070), the Foundation of Jinling Institute of Technology (No. jit-b-202219).
The authors declare that the research was conducted in the absence of any commercial or financial relationships that could be construed as a potential conflict of interest.
All claims expressed in this article are solely those of the authors and do not necessarily represent those of their affiliated organizations, or those of the publisher, the editors and the reviewers. Any product that may be evaluated in this article, or claim that may be made by its manufacturer, is not guaranteed or endorsed by the publisher.
Allen, C., Her, S., and Jaffray, D. A. (2017). Radiotherapy for cancer: Present and Future. Adv. Drug Deliv. Rev. 109, 1–2. doi:10.1016/j.addr.2017.01.004
Atun, R., Jaffray, D. A., Barton, M. B., Bray, F., Baumann, M., Vikram, B., et al. (2015). Expanding global access to radiotherapy. Lancet Oncol. 16 (10), 1153–1186. doi:10.1016/s1470-2045(15)00222-3
Chen, J., Luo, H., Liu, Y., Zhang, W., Li, H., Luo, T., et al. (2017). Oxygen-self-produced nanoplatform for relieving hypoxia and breaking resistance to sonodynamic treatment of pancreatic cancer. ACS Nano 11 (12), 12849–12862. doi:10.1021/acsnano.7b08225
Chen, Q., Feng, L., Liu, J., Zhu, W., Dong, Z., Wu, Y., et al. (2016). Intelligent Albumin–MnO2 nanoparticles as pH-/H2O2-Responsive Dissociable Nanocarriers to Modulate tumor hypoxia for effective combination therapy. Adv. Mat. 28 (33), 7129–7136. doi:10.1002/adma.201601902
Cheng, Y., Cheng, H., Jiang, C., Qiu, X., Wang, K., Huan, W., et al. (2015). Perfluorocarbon nanoparticles enhance reactive oxygen levels and tumour growth inhibition in photodynamic therapy. Nat. Commun. 6 (1), 8785–8788. doi:10.1038/ncomms9785
Dachs, G. U., and Tozer, G. M. (2000). Hypoxia modulated gene expression: Angiogenesis, metastasis and therapeutic exploitation. Eur. J. Cancer 36 (36), 1649–1660. doi:10.1016/s0959-8049(00)00159-3
De Ruysscher, D., Niedermann, G., Burnet, N. G., Siva, S., Lee, A. W. M., and Hegi-Johnson, F. (2019). Radiotherapy toxicity. Nat. Rev. Dis. Prim. 5 (1), 13. doi:10.1038/s41572-019-0064-5
Dolmans, D. E., Dai, F., and Jain, R. K. (2003). Photodynamic therapy for cancer. Nat. Rev. Cancer 3 (5), 380–387. doi:10.1038/nrc1071
Dougherty, T. J., Gomer, C. J., Henderson, B. W., Jori, G., Kessel, D., Korbelik, M., et al. (1998). Photodynamic therapy. JNCI J. Natl. Cancer Inst. 12 (6), 889–905. doi:10.1093/jnci/90.12.889
Huo, D., He, J., Li, H., Huang, A. J., Zhao, H. Y., Ding, Y., et al. (2014). X-ray CT guided fault-free photothermal ablation of metastatic lymph nodes with ultrafine HER-2 targeting W18O49 nanoparticles. Biomaterials 35 (35), 9155–9166. doi:10.1016/j.biomaterials.2014.07.034
Huo, D., Jiang, X., and Hu, Y. (2019). Recent Advances in Nanostrategies capable of overcoming Biological Barriers for tumor Management. Adv. Mat. 32, 1904337. doi:10.1002/adma.201904337
Huo, D., Liu, S., Zhang, C., He, J., Zhou, Z., Zhang, H., et al. (2017). Hypoxia-targeting, tumor microenvironment responsive Nanocluster Bomb for Radical-enhanced radiotherapy. ACS Nano 11, 10159–10174. doi:10.1021/acsnano.7b04737
Kim, C., Yang, H., Fukushima, Y., Er Saw, P., Lee, J., Park, J.-S., et al. (2014). Vascular RhoJ is an effective and selective target for tumor angiogenesis and vascular disruption. Cancer Cell 25, 102–117. doi:10.1016/j.ccr.2013.12.010
Kim, W., Lee, S., Seo, D., Kim, D., Kim, K., Kim, E., et al. (2019). Cellular stress responses in radiotherapy. Cells 8 (9), 1105. doi:10.3390/cells8091105
Kolemen, S., Ozdemir, T., Lee, D., Kim, G. M., Karatas, T., Yoon, J., et al. (2016). Remote-controlled release of singlet oxygen by the Plasmonic heating of endoperoxide-Modified gold Nanorods: Towards a Paradigm change in photodynamic therapy. Angew. Chem. Int. Ed. 55, 3606–3610. doi:10.1002/anie.201510064
Liu, S., Li, H., Xia, L., Xu, P., Ding, Y., Huo, D., et al. (2017). Anti-RhoJ antibody functionalized Au@I nanoparticles as CT-guided tumor vessel-targeting radiosensitizers in patient-derived tumor xenograft model. Biomaterials 141, 1–12. doi:10.1016/j.biomaterials.2017.06.036
Liu, T., Lu, T.-Y., Yang, Y.-C., Chang, S.-H., Chen, H.-H., Lu, L., et al. (2020). New combination treatment from ROS-Induced sensitized radiotherapy with nanophototherapeutics to fully eradicate orthotopic breast cancer and inhibit metastasis. Biomaterials 257, 120229. doi:10.1016/j.biomaterials.2020.120229
Mahmoud Asadirad, A., Erno, Z., and Branda, N. R. (2013). Photothermal release of singlet oxygen from gold nanoparticles. Chem. Commun. 49 (50), 5639–5641. doi:10.1039/c3cc42217h
Masuda, Shigeo, and Belmonte, Juan Carlos Izpisua (2013). The microenvironment and resistance to personalized cancer therapy. Nat. Rev. Clin. Oncol. 10 (2), 79–515. doi:10.1038/nrclinonc.2012.127-c1
Muhammad Idris, N., Kumara Gnanasammandhan, M., Zhang, J., Ho, P. C., Mahendran, R., and Zhang, Y. (2012). In vivo photodynamic therapy using upconversion nanoparticles as remote-controlled nanotransducers. Nat. Med. 18 (10), 1580–1585. doi:10.1038/nm.2933
Peter, V., and Arnulf, M. (2007). Hypoxia in cancer: Significance and Impact on clinical outcome. Cancer Metastasis Rev. 26, 225–239. doi:10.1007/s10555-007-9055-1
Rey, S., Schito, L., Koritzinsky, M., and Bradly, G. (2017). Molecular targeting of hypoxia in radiotherapy. Adv. Drug Deliv. Rev. 109, 45–62. doi:10.1016/j.addr.2016.10.002
Scholzen, T., and Gerdes, J. (2000). The Ki‐67 protein: From the known and the unknown. J. Cell. Physiol. 182 (182), 311–322. doi:10.1002/(sici)1097-4652(200003)182:3<311::aid-jcp1>3.0.co;2-9
Xia, D., Xu, P., Luo, X., Zhu, J., Gu, H., Huo, D., et al. (2019). Overcoming hypoxia by Multistage nanoparticle Delivery system to inhibit mitochondrial Respiration for photodynamic therapy. Adv. Funct. Mat. 29, 1807294. doi:10.1002/adfm.201807294
Xie, Z., Cai, X., Sun, C., Liang, S., Shao, S., Huang, S., et al. (2019). O2 loaded pH-Responsive Multifunctional Nanodrug carrier for overcoming hypoxia and highly Efficient Chemo-photodynamic cancer therapy. Chem. Mat. 31 (2), 483–490. doi:10.1021/acs.chemmater.8b04321
Keywords: W18O49, nanoparticles, hypoxia, ROS, RT, PDT, tumor microenvironment
Citation: Wang J, Hao L, Li X and Liu S (2022) W18O49@EP nanoparticles improve the anti-tumor effect of radiotherapy and photodynamic therapy by avoiding the limitation of hypoxia. Front. Bioeng. Biotechnol. 10:1060467. doi: 10.3389/fbioe.2022.1060467
Received: 03 October 2022; Accepted: 26 October 2022;
Published: 10 November 2022.
Edited by:
Ying Yang, University of Michigan, United StatesReviewed by:
Xuan Shouhu, University of Science and Technology of China, ChinaCopyright © 2022 Wang, Hao, Li and Liu. This is an open-access article distributed under the terms of the Creative Commons Attribution License (CC BY). The use, distribution or reproduction in other forums is permitted, provided the original author(s) and the copyright owner(s) are credited and that the original publication in this journal is cited, in accordance with accepted academic practice. No use, distribution or reproduction is permitted which does not comply with these terms.
*Correspondence: Lingyun Hao, aGx5QGppdC5lZHUuY24=
Disclaimer: All claims expressed in this article are solely those of the authors and do not necessarily represent those of their affiliated organizations, or those of the publisher, the editors and the reviewers. Any product that may be evaluated in this article or claim that may be made by its manufacturer is not guaranteed or endorsed by the publisher.
Research integrity at Frontiers
Learn more about the work of our research integrity team to safeguard the quality of each article we publish.