- Department of Chemistry, CICECO—Aveiro Institute of Materials, University of Aveiro, Aveiro, Portugal
Polysaccharides and proteins are extensively used for the design of advanced sustainable materials. Owing to the high aspect ratio and specific surface area, ease of modification, high mechanical strength and thermal stability, renewability, and biodegradability, biopolymeric nanofibrils are gaining growing popularity amongst the catalog of nanostructures exploited in a panoply of fields. These include the nanocomposites, paper and packaging, environmental remediation, electronics, energy, and biomedical applications. In this review, recent trends on the use of cellulose and protein nanofibrils as versatile substrates for the design of high-performance nanomaterials are assessed. A concise description of the preparation methodologies and characteristics of cellulosic nanofibrils, namely nanofibrillated cellulose (NFC), bacterial nanocellulose (BNC), and protein nanofibrils is presented. Furthermore, the use of these nanofibrils in the production of sustainable materials, such as membranes, films, and patches, amongst others, as well as their major domains of application, are briefly described, with focus on the works carried out at the BioPol4Fun Research Group (Innovation in BioPolymer based Functional Materials and Bioactive Compounds) from the Portuguese associate laboratory CICECO–Aveiro Institute of Materials (University of Aveiro). The potential for partnership between both types of nanofibrils in advanced material development is also reviewed. Finally, the critical challenges and opportunities for these biobased nanostructures for the development of functional materials are addressed.
Introduction
The transition towards a more sustainable society, aligned with the 2030 Agenda for Sustainable Development (United Nations, 2015), demands a considerable change in the overall design and manufacturing practices, regarding consumer items and materials with advanced functionalities (Silva et al., 2021b). In this perspective, naturally abundant and renewable biobased feedstocks are increasingly being exploited as eco-friendly building blocks for the development of sustainable materials with diverse morphologies and applications (Silva et al., 2014a; Silva et al., 2021b; Shaghaleh et al., 2018; Mohammadian et al., 2020; Shen et al., 2021; Teixeira et al., 2022a).
The advent of nanotechnology and nanoscience has created an opportunity to control materials at the nanoscale, enabling the tailored design of complex nanostructures such as nanotubes, nanowires, nanofibrils, and nanoparticles (Krishnaswamy and Orsat, 2017; Carvalho et al., 2021b). Nanofibrils, i.e., fibers with diameter in the nanoscale and significantly longer lengths (up to several micrometers), have attracted the interest of researchers as essential building blocks in the development of innovative functional materials, owing to their outstanding mechanical properties (Barhoum et al., 2018; Barhoum et al., 2019). Particularly, nanofibrils obtained from polysaccharides (e.g., cellulose, chitosan/chitin) (Raza et al., 2021) and proteins (e.g., silk fibroin, soy protein, zein) (Babitha et al., 2017) combine the unique features of nanofibrils with biocompatibility, biodegradability, and in some cases specific biological properties, that can be exploited for the preparation of functional materials, such as membranes (Galiano et al., 2018), hydrogels (Ling et al., 2018b; Du et al., 2019), and films (Lendel and Solin, 2021), among others (Knowles and Mezzenga, 2016; Owolabi et al., 2020; Santos et al., 2021). These nanomaterials find application in high-tech applications, as for example, in enzyme immobilization (Frazão et al., 2014), functional textiles (Silva et al., 2021a), active food packaging (Cazón and Vázquez, 2021; Daniloski et al., 2021), tissue engineering and wound healing (Carvalho et al., 2019; Chen et al., 2022; Soroush et al., 2022), drug delivery (Almeida et al., 2014; Silvestre et al., 2014), cosmetic applications (Almeida et al., 2021; Meftahi et al., 2022), sensors and semiconductors (Cherian et al., 2022; Qiu et al., 2022), fuel cells (Vilela et al., 2019e), and environmental remediation (Muqeet et al., 2020; Abdelhamid and Mathew, 2021; Peydayesh and Mezzenga, 2021; Raza et al., 2021).
As the most abundant polysaccharide on Earth, cellulose has attracted increased scientific and economic interest in materials development (Charreau et al., 2020; Meftahi et al., 2021), particularly in its nanometric forms, viz. cellulose nanocrystals (CNCs), nanofibrillated cellulose (NFC) and bacterial nanocellulose (BNC) (Freire et al., 2013; Figueiredo et al., 2014; Freire and Vilela, 2022). The majority of literature in this field addresses both established and novel methods for isolating and modifying nanocelluloses (Abdul Khalil et al., 2012; Meftahi et al., 2021; Sayyed et al., 2021; Hamimed et al., 2022; Pradhan et al., 2022) and their application in materials science (Carvalho et al., 2019; Vilela et al., 2019e; Wang et al., 2020; Subhedar et al., 2021; Wang et al., 2022). Thus, NFC and BNC will be the focus of this review concerning cellulose nanofibrils application in this field.
On the other hand, owing to the recent advances in protein fibrillation mechanisms and methodologies (Wang et al., 2021), protein nanofibrils (also known as amyloid fibrils) obtained from both animal and plant-based origins (Meng et al., 2022) have stepped forward as promising nanostructures for the development of materials with a variety of functional properties that are gaining attention for packaging purposes (Daniloski et al., 2021; Hadidi et al., 2022), environmental remediation (Peydayesh and Mezzenga, 2021; Vinayagam et al., 2022), and biomedical applications (Silva et al., 2014a; Mohammadian et al., 2020), amongst others.
The production, modification, and applications of cellulose and protein nanofibrils have already been extensively reviewed, as proven by the number of review articles and book chapters supra cited. Nevertheless, as far as our research could go, only one review by Ling et al. (2018a) provided an overview of both polysaccharide and protein-based nanofibrils, specifically cellulose, chitin, silk, and collagen nanofibrils, for material production. This comprehensive work provides a detailed outline of the four most abundant biopolymer nanofibrils in terms of structure, computational models, processing methodologies, and applications. Nonetheless, the authors only offer information regarding fibrous proteins (i.e., silk and collagen) despite the growing interest in the fibrillation process of globular proteins [e.g., β-lactoglobulin (Loveday et al., 2017), lysozyme (Mohammadian and Madadlou, 2018)] with more complex structural levels and essential biological properties.
The present review will focus on nanocellulose fibrils, namely NFC and BNC, and protein amyloid nanofibrils, to design advanced and sustainable materials. Though cellulose nanocrystals (CNCs) are isolated from cellulosic feedstocks, the resulting highly-crystalline rod-like nanostructures contain all three exterior dimensions at the nanoscale and are classified as cellulose nanoparticles (Barhoum et al., 2019). As a result, this cellulose nanoform is beyond the scope of this review. Nonetheless, the reader can find more information about recent advances in the preparation and modification of CNCs, material fabrication, and significant application areas elsewhere (Long et al., 2021; Rana et al., 2021; Shojaeiarani et al., 2021).
Thus, this review provides a succinct overview regarding the fabrication methodologies and properties of nanocellulose and protein nanofibrils, their use in materials design, and critical areas of application (Figure 1), with emphasis on the works developed in the latter years at the BioPol4Fun Research Group (Innovation in BioPolymer based Functional Materials and Bioactive Compounds) from the Portuguese associate laboratory CICECO—Aveiro Institute of Materials (University of Aveiro). The combination of both types of nanofibrils is also examined before concluding with some prospects for the exploitation of these nanostructures for materials research. Since it is impossible to dive into the detail of every cited work, a few key examples and features of representative materials have been chosen for a more elaborated discussion in the following sections. Further information concerning the type, composition, fabrication methodologies, and critical properties and applications of each material is summarized on Tables 1–5.
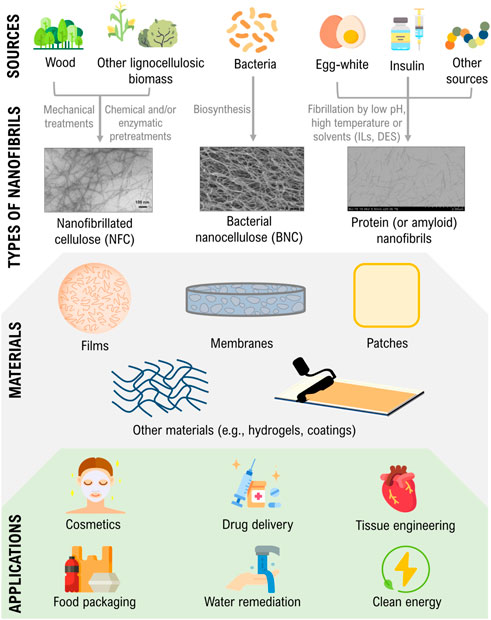
FIGURE 1. Illustration of the sources and production of nanocellulose and protein nanofibrils, their use in materials design, and critical areas of application. Transmission electron microscopy (TEM) micrographs are adapted and reprinted with permission from (Nazrin et al., 2020). Copyright Frontiers, 2020; and (Silva et al., 2018c). Copyright Elsevier, 2018.
Exploiting nanocellulose fibrils for the production of novel materials
With an estimated annual production of 1011–1012 tonnes, cellulose is considered the most prevalent natural polymer and can be extracted from many sources, mostly from plants (lignocellulosic biomass), but also from algae, bacteria, and tunicates (Nechyporchuk et al., 2016; Trache et al., 2020). This linear polysaccharide is comprised of D-glucose units linked via β-(1→4) glycosidic bonds. Due to the strong intra- and intermolecular hydrogen bond network and the intermolecular van der Waals forces, the cellulose chains arrange themselves into a distinctive three-dimensional structure of microfibrils that contain crystalline (highly ordered) and amorphous domains (Figure 2A) (Noremylia et al., 2022). The intertwining of microfibrils culminates in the formation of macrofibrils.
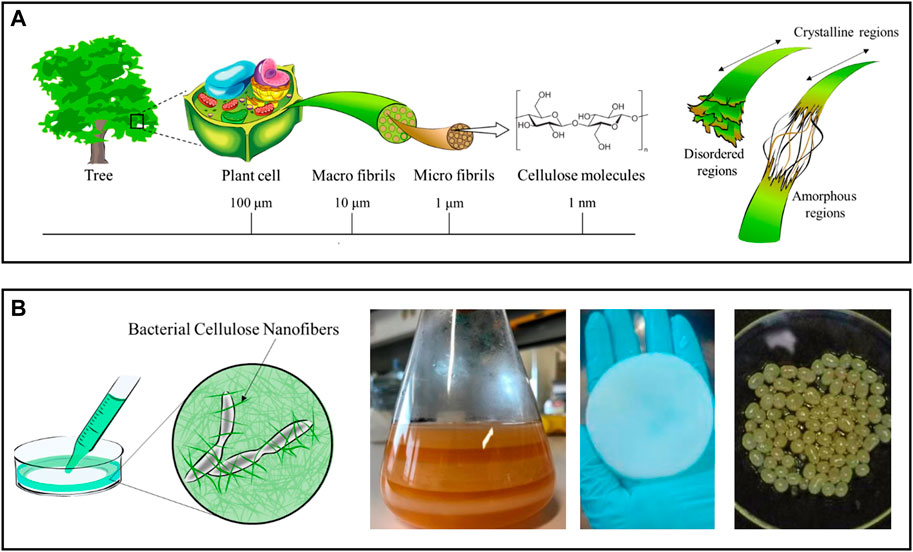
FIGURE 2. (A) Schematic representation of the hierarchical structure of cellulose obtained from plants, with detail in the crystalline and amorphous regions of the cellulose nanofibrils. Adapted and reprinted with permission from (Miyashiro et al., 2020). Copyright MDPI, 2020; (B) Diagram of a bacterial nanocellulose culture and digital photographs of membranes produced in static culture before (left) and after cleaning (center), and of spherical particles obtained in agitated culture conditions (right). Adapted and reprinted with permission from (Miyashiro et al., 2020). Copyright MDPI, 2020; and (Almeida et al., 2021). Copyright MDPI, 2021.
As overviewed by Klemm et al. (2018), Thomas et al. (2020) and, more recently, by Noremylia et al. (2022), nanocelluloses can be extracted from native cellulose using top-down approaches, or obtained by bottom-up methodologies, producing nanostructures with distinct morphological features. According to their characteristics, three types of nanocellulose can be identified: 1) Cellulose nanocrystals (CNCs), also known as “nanocrystalline cellulose” or “cellulose nanowhiskers,” 2) Nanofibrillated cellulose (NFC), also commonly referred to as “microfibrillated cellulose” or “cellulose nanofibrils,” and 3) Bacterial nanocellulose (BNC), also denominated “microbial cellulose,” “biocellulose” or “biotech cellulose” (Klemm et al., 2018). As previously stated, this section will provide a brief rundown of the fabrication processes and attributes of NFC and BNC. Regardless, the reader is encouraged to examine the publications mentioned in each section, which provide a comprehensive overview of these topics.
Plant cellulose can be used to obtain nanofibrils (NFC) with a diameter of 5–60 nm and a length of a few micrometers, which contain both amorphous and crystalline regions (Figure 2A) (Nechyporchuk et al., 2016). Delamination of cellulose fibers is most frequently accomplished in an aqueous medium through intense mechanical treatments (e.g., high-pressure homogenization, grinding, and refining), resulting in suspensions of NFC with low solid content (<5 wt%) (Nechyporchuk et al., 2016; Noremylia et al., 2022). Despite the high energy consumption associated with these processes, this is the most scalable methodology and, as a result, the preferred option for industrial applications. Nonetheless, chemical and enzymatic pretreatments are also proposed to minimize the energy consumption in mechanical processing, while also increasing the degree of fibrillation of NFC, as these pretreatments disturb the hydrogen bond network (Pradhan et al., 2022; Squinca et al., 2022). Nevertheless, depending on the selection and combination of pretreatments and extraction processes, various grades of NFC with variable dimensions and properties (e.g., crystallinity, rheological behavior, surface chemistry) can be obtained (Nechyporchuk et al., 2016). Non-traditional processes, such as extrusion, ball milling, steam explosion, aqueous counter collision, cryocrushing, and ultrasonication, have been proposed to govern the fibrillation process yielding NFC with more predictable properties (Wang et al., 2021).
In contrast, BNC results from a bottom-up process in which non-pathogenic bacterial strains synthesize the nanofibrils through a sequence of biochemical reactions driven by specific enzymes and cofactors (Manan et al., 2022). Most common BNC-producing species belong to the Komagataeibacter genus (previously known as Gluconacetobacter (Trovatti et al., 2011a)), although the list may include organisms from the Agrobacterium, Rhizobium, and Pseudomonas genus. In practice, BNC is a three-dimensional nanofiber network resulting from a fermentation process taking place in a nutrient-enriched culture medium (Klemm et al., 2018). Depending on the culture conditions, this network can be produced with tailored shapes and sizes, ranging from planar hydrogel pellicles, formed at the air-water interface of the culture media in static cultures, to sphere-like particles in agitated cultures (Figure 2B) (Chandana et al., 2022). The use of bioreactor systems with complex geometries is known to improve BNC yield, enabling the production of BNC on a larger scale (Chandana et al., 2022). Compared to NFC, bacterial nanocellulose has a high degree of crystallinity because it is free of hemicelluloses, lignin, and other minor compounds found in plant-lignocellulosic biomass (Klemm et al., 2018). BNC also has an exceptional water retention ability since it contains nearly 99% water.
Overall, cellulosic nanofibrils have remarkable properties, such as high surface area, high aspect ratio, tailorable surface chemistry, high mechanical strength, rheological behavior, high water absorption capacity, non-cytotoxicity, non-genotoxicity, and inherent renewability (Chandana et al., 2022; Noremylia et al., 2022), which elevate this polysaccharide out of the shadows of the pervasive pulp and paper industries to produce sophisticated materials, with diverse types and functionalities. However, due to the presence of three hydroxyl groups per anhydroglucose unit, these nanofibers are extremely hydrophilic, which can be seen as a benefit in applications in which water compatibility is an advantage (e.g., most often in biomedical applications) or as a drawback when compatibilization with hydrophobic domains is essential, thus requiring adequate modifications towards their hydrophobization.
The following sections illustrate some of the most recent and relevant contributions about the use of NFC and BNC to assemble membranes, films, patches and other materials.
Membranes
The simplicity of creating membranes with tailored size and shape that do not disintegrate when exposed to water-rich environments is a particular advantage of BNC materials in several sectors. NFC-based membranes can also be produced by simple methodologies, like casting or filtration (Table 1). Moreover, cellulose nanofibrils have a high surface area and are simple to modify. As such, the properties of BNC and NFC can be customized to accomplish specific functions (Shen et al., 2020). For instance, the chemical grafting of amino moieties grants antibacterial activity to BNC fibrils (Fernandes et al., 2013; Chantereau et al., 2019a), and the in situ atom transfer radical polymerization reaction of acrylate monomers onto the BNC nanofibrils surface can improve the hydrophobicity of the membranes (Lacerda et al., 2013). Additionally, the in situ free radical polymerization of acrylate monomers inside the 3D-network of BNC can increase their water uptake capacity (Figueiredo et al., 2015a), depending on the starting monomers and conditions. To illustrate, the grafting of poly(methyl methacrylate) in the BNC network increased the water static contact angle of the membrane from 32° up to 134°, thus enhancing its hydrophobic behavior (Lacerda et al., 2013). Owing to the properties mentioned above, allied with their tunable porous structure, nanocellulose-based materials are receiving significant attention for the fabrication of high-performance membranes for environmental remediation purposes, with application as ion-selective separators for clean energy, in water purification, air filtration, and carbon dioxide sieving (Wang et al., 2022).
In the energy field, nanocellulose membranes are being exploited to design components of energy storage systems, in the development of electrodes via direct carbonization of the cellulose nanofibrils or incorporation of conductive polymers (e.g., polypyrrole), carbon (e.g., carbon nanotubes) and metal/metal oxides (e.g., silver, manganese dioxide) phases (Xiao et al., 2022). Nanocelluloses are also being explored as alternatives for components in energy generators like fuel cells, particularly as replacements for the ion-exchange membranes (Vilela et al., 2019e). Nanocelluloses do not naturally possess the ionic conductivity required for this application; however, this problem can be solved by incorporating ion-conducting phases either through the direct diffusion of ionomers, like Nafion®, into the BNC 3D-nanofibrillar structure (Gadim et al., 2016) or via the in situ free-radical polymerization under green conditions of adequate synthetic monomers to generate polyelectrolytes, such as poly(4-styrene sulfonic acid) (Gadim et al., 2014, 2017; Vilela et al., 2020a), poly(methacryloyloxyethyl phosphate) (Vilela et al., 2016), poly(methacroylcholine chloride) (Vilela et al., 2017) and poly(bis[2-(methacryloyloxy)ethyl] phosphate) (Vilela et al., 2018b). More recently, BNC and naturally derived macromolecules rich in ion-conducting functional groups have been combined to create fully biobased ion-exchange membranes. Fucoidan, an algal polysaccharide (Vilela et al., 2020c), and lignosulfonates, a by-product of the sulfite pulping process (Vilela et al., 2020b), are examples of this practice. Despite the ionic conductivity of some of these systems being two orders of magnitude lower than Nafion®, the benchmark for this application, these findings show the immense potential of cellulose nanofibrils for creating environmentally friendly separators.
Sorbents of modified nanocellulose membranes have been described to remove heavy metal ions, dyes, pesticides, pharmaceuticals, and other dissolved organic pollutants from contaminated waters (Muqeet et al., 2020; Abdelhamid and Mathew, 2021). Since cellulosic nanofibrils have low affinity for ionic species, it is necessary their combination with materials with high adsorption capacity (e.g., graphene oxide) (Abdelhamid and Mathew, 2021) or modification with adequate functional groups (e.g., carboxylate or quaternary ammonium moieties) to which metal ions, dyes or other contaminants (e.g., sulfate and fluoride anions) can bind to. Functional groups can also be imparted to cellulose nanofibers during the production stages, in the case of NFC, or by post-modification (Abdelhamid and Mathew, 2021). A common example is the introduction of carboxyl groups (COO⁻) via TEMPO-mediated oxidation of the C6 primary hydroxyl group, which is often used in the nanocellulose production stages. Cellulose nanofibrils with anionic functional groups (e.g., COO⁻) ensure high adsorption toward cationic contaminants. In contrast, nanofibrils bearing cationic groups can be effective sorbents for anionic compounds (e.g., fluoride) (Abdelhamid and Mathew, 2021).
However, the affinity towards both positively and negatively charged molecules is highly desirable in this field. To address that challenge, cellulose nanofibrils can be combined with zwitterionic polymers, such as the non-toxic poly(2-methacryloyloxyethyl phosphorylcholine), that contains a phosphate anion and a trimethylammonium cation (Vilela et al., 2019b). It was shown that this BNC/zwitterionic polymer membrane effectively collected cationic (methylene blue) and anionic (methylene orange) model dyes from contaminated water (ca. 4.4–4.5 mg g−1) and limited the growth of pathogens commonly observed in these environments (up to 4.3- and 1.8-log CFU reduction for Staphylococcus aureus and Escherichia coli, respectively), owing to the antimicrobial action of the polymer, highlighting the effective dual action of the biosorbents in the retrieval of contaminants (Figure 3). Overall, reusable nanocellulose membranes hold the potential for contaminant removal and salvage and repurposing of valuable waste matter, such as metals.
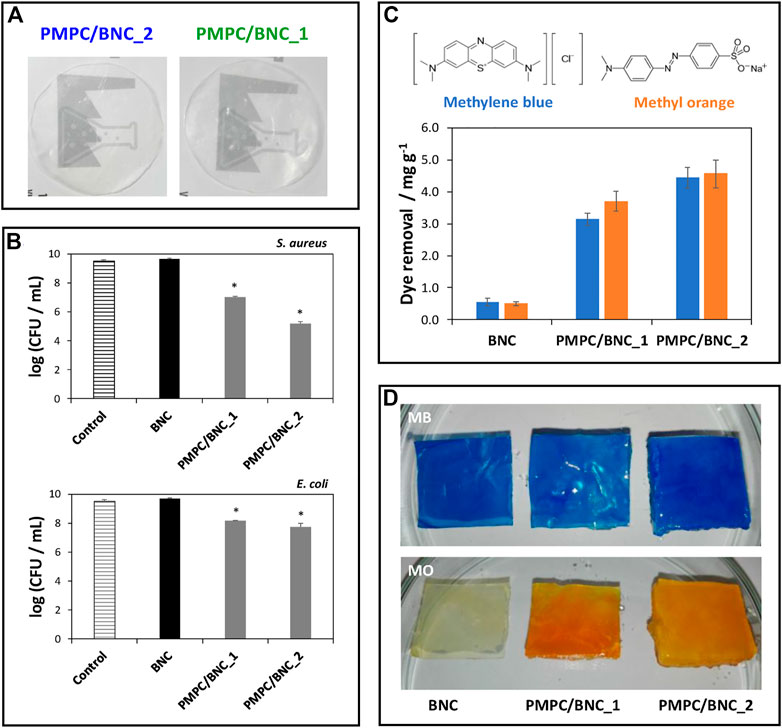
FIGURE 3. (A) Digital photographs of the zwitterionic membranes; (B) Graphical representation of the antibacterial activity of the membranes against S. aureus and E. coli after 24 h of exposure; (C) Chemical structures of the methylene blue (MB) and methylene orange (MO) dyes and graphical representation of the dye removal capacity of the BNC membranes with different compositions; (D) Digital photographs of the membranes after immersion in the aqueous dye solutions for 12 h. Reprinted with permission from (Vilela et al., 2019b). Copyright MDPI, 2019.
Films
The fundamental shift towards a more environmentally conscious society has increased the demand for packaging materials made from sustainable resources to reduce the volume and impact of the typical petroleum-based plastics we are accustomed to (Silva et al., 2021b) (Table 2). The inclusion of cellulose nanofibrils in polymeric films results in improved thermal and mechanical properties that are often vital for their target applications (Amara et al., 2021; Cazón and Vázquez, 2021). For instance, both BNC (Trovatti et al., 2012a) and NFC (Trovatti et al., 2012b) have been employed as reinforcing elements in homogeneous and translucent pullulan films via the simple solvent casting of aqueous suspensions, leading to nanocomposites films with improved thermal (increase in the maximum degradation temperature, compared to the neat pullulan films) and mechanical (e.g., a 22 times increase in the Young’s modulus value with the inclusion of 10% of BNC, compared to unfilled pullulan matrices) properties.
Films that also offer active and intelligent functions are becoming ever more relevant in the food industry sector, as packaging acts as both a method of transportation and a mean of preserving the food contained within (Vilela et al., 2018a; Carvalho et al., 2021a). In this sense, films that incorporate additives with antimicrobial (e.g., metal nanoparticles, chitosan), antioxidant (e.g., plant extracts, phenolic compounds), and gas scavenging (e.g., metal oxides) functions can extend the shelf life of packed products, resulting in less food spoilage and waste (Vilela et al., 2018a). As an illustrative example, NFC-based films containing arabinoxylans obtained from brewers’ spent grains and ferulic acid or feruloylated arabinoxylo-oligosaccharides displayed good antioxidant activity (ca. 90%, assessed by the DPPH radical scavenging activity), which is essential to control the oxidative reactions and maintain the sensory properties of the foodstuff (Moreirinha et al., 2020). Furthermore, these films showed antimicrobial activity toward common food pathogens, i.e., Gram-positive (S. aureus) and Gram-negative (E. coli) bacteria and fungi (Candida albicans). The inclusion of these active compounds also offers the opportunity to improve the UV-light properties (decrease in the transmittance values of the films in the UVC (100–280 nm), UVB (280–320 nm) and UVA (320–400 nm) regions) and gas barrier of the films, while retaining the mechanical strength (Young’s modulus up to 7.5 GPa) and flexibility characteristic of the cellulose nanofibrils (Moreirinha et al., 2020). NFC has also been combined with a mango leaf extract using conventional solvent-casting methodologies and alternatively by supercritical solvent impregnation (Bastante et al., 2021). The ensuing films displayed good antioxidant (ca. 84%, using the DPPH assay) and antimicrobial activity (ca. 37% and 91% growth inhibition of S. aureus and E. coli, correspondingly), which was more pronounced in the films prepared by the non-conventional supercritical solvent impregnation methodology, highlighting the potential of this eco-friendly technique in the production of bioactive films with improved functional properties.
Film packaging materials can house molecules that interact with internal (e.g., food) or external factors (e.g., temperature) and provide dynamic feedback regarding the condition of packed goods (Amin et al., 2022). These intelligent films can respond to environmental changes, such as variation in pH levels, gaseous composition, and microbial activity metabolites (e.g., hydrogen sulfide). The incorporation of these types of molecules in inherently biodegradable matrices (such as NFC and BNC) can be an appealing platform for the mitigation of the environmental footprint imposed by packaging goods. A case in point is the incorporation of poly(sulfobetaine methacrylate) in BNC films (Vilela et al., 2019c). This zwitterionic polymer endows antibacterial activity against S. aureus and E. coli, while also providing proton conductivity to the material (maximum of 1.5 mS cm−1), which can be advantageous for the implementation as sensors to monitor food humidity levels.
Patches
Nanostructured cellulose-based patches are attractive for a wide range of cosmetic and biomedical applications, including wound healing (Carvalho et al., 2019), drug delivery (Silvestre et al., 2014; Raghav et al., 2021), and skin care (Almeida et al., 2021), due to their inherent biodegradability, biocompatibility, capacity to avoid immunological reactions, and potential to be combined with other components, in particular, bioactive molecules and drugs (Table 3).
In the cosmetics industry, cellulose nanofibrils have been widely employed as stabilizers and thickening agents in cream and liquid formulations, as well as moisturizing agents and polymeric matrices (particularly BNC) in facial sheet masks and skin patches (Meftahi et al., 2022). In this latter application, BNC has emerged as an appealing substitute for cotton-based and synthetic polymer-based patches owing to its versatile production with tailored shapes and sizes and capacity to retain a broad range of compounds that can be delivered to the skin in a controlled and sustained manner (Almeida et al., 2021). As such, BNC can act as a carrier of skin-active substances such as caffeine (Silva et al., 2014b), commonly used in cellulite treatment, or plant-based extracts (Almeida et al., 2022), ionic liquids based on phenolic acids (Morais et al., 2019) and complex-B vitamins (Chantereau et al., 2020), which can be used in antiaging formulations. The formulation of these active principles as ionic liquids is aimed at modulating their water solubility and, thus, the resulting release profile. For instance, Chantereau et al. (2020) described an enhancement of the solubility of complex-B vitamins ionic liquids, composed of the vitamin anion and the cholinium cation, compared to the solubility of the original vitamins in water, thereby increasing their bioavailability (up to 30-fold). Moreover, including these active principles in BNC leads to a more complete and faster release of the compounds, which is attractive for short-term applications. BNC has also been reported as the backing layer of microneedles, i.e., arrays of micron-sized needles with 25–2000 μm in height (Fonseca et al., 2019), to support the incorporation of bioactive molecules (e.g., rutin) and delay the release of active pharmaceutical ingredients (APIs) to the skin (delayed cumulative release plateau from 3 to 6.5 h) (Fonseca et al., 2021).
NFC (Raghav et al., 2021) and BNC (Silvestre et al., 2014) based materials can be employed as oral, buccal, or topical drug delivery systems for hydrophilic and hydrophobic APIs. BNC displays its supremacy yet again by facilitating the manufacture of pharmacological patches or other carriers via the simple diffusion of APIs aqueous solutions across its three-dimensional porous network. Among the numerous works, we highlight the use of BNC in combination with lidocaine (Trovatti et al., 2011b; 2012c), ibuprofen (Trovatti et al., 2012c), diclofenac (Silva et al., 2014c; Saïdi et al., 2017), diclofenac/hyaluronic acid (Carvalho et al., 2020), and non-steroidal anti-inflammatory drugs (NSAIDs)-based ionic liquids (Chantereau et al., 2019b).
Even when subjected to accelerated testing settings at varying temperatures and relative humidity, BNC patches retain their morphological integrity and release profile (Silva et al., 2020c). In the cases mentioned above, the release of the drugs is essentially governed by their hydrophobic/hydrophilic character diffusion through the 3D network of BNC. However, the modulation of drug release is highly desirable in drug delivery. For instance, Saïdi et al. (2017) modified BNC, under green reaction conditions, with polymers containing amino acid pending moieties to produce patches with pH-responsive behavior.
In wound healing, BNC is once more the material of choice for most applications due to its high purity and similarity to the extracellular matrix (Carvalho et al., 2019). BNC-based wound dressings provide an adequate moist environment with selective oxygen and water permeability, while simultaneously protecting the injured area from the entry of pathogens and removing the exudates from the site (Carvalho et al., 2019). Additionally, they are simple to detach from the area without inflicting pain or dislodging the newly formed tissue. BNC patches can be functionalized with hemostatic and antimicrobial agents or other bioactive molecules that improve skin cell proliferation and accelerate wound healing. For instance, Fonseca et al. (2020) prepared BNC patches loaded with dexpanthenol, an API used in the treatment of dermatological conditions as a topical protectant and moisturizing agent, and spin-coated the patches with varying layers of alginate and chitosan (Figure 4). The multilayered patches presented antibacterial activity against S. aureus, mainly due to the presence of chitosan, a polysaccharide with well-known antimicrobial activity (Wang et al., 2020). They also promoted cell migration and wound closure (in vitro) by the inclusion of dexpanthenol in the non-sacrificial BNC matrix. Furthermore, the in vitro release profile of the API could be modulated by the number of layers of the polysaccharides, with an extended timeframe of drug delivery as the number of layers increased (Fonseca et al., 2020).
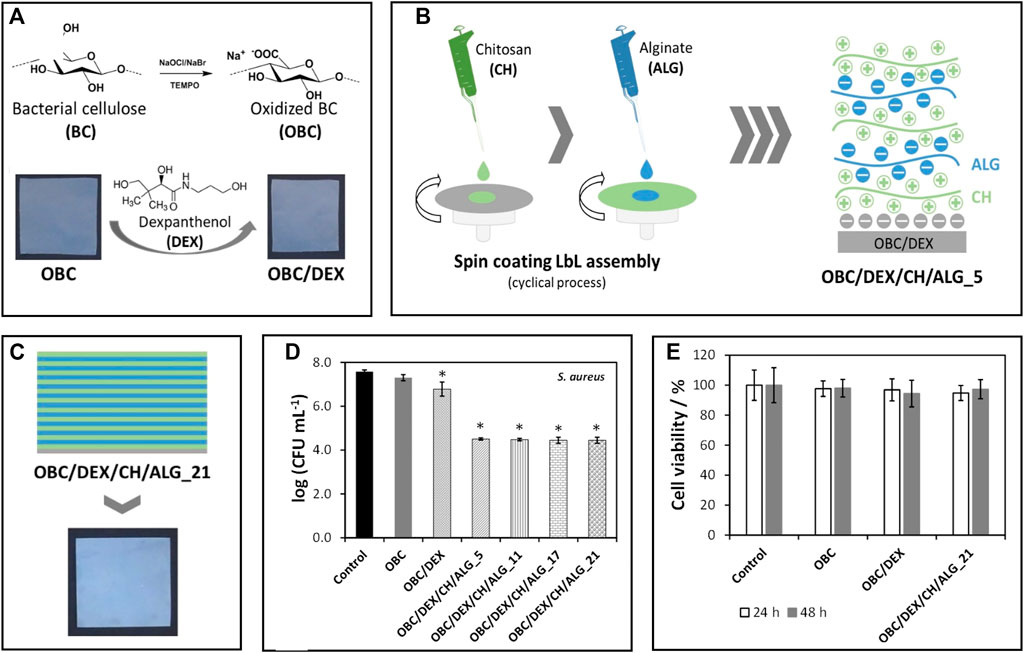
FIGURE 4. (A) Representation of the preparation of TEMPO-mediated oxidated BNC (OBC) and incorporation of dexpanthenol (DEX); (B) Scheme of the layer-by-layer spin coating assembly of the patches using alginate and chitosan, and (C) Digital photograph of the multilayered patches with 21 layers; Graphical representation of the (D) antibacterial activity of the patches against Staphylococcus aureus after 24 h of exposure and (E) cell viability of HaCaT cells after 24 and 48 h of exposure. Reprinted with permission from (Fonseca et al., 2020). Copyright MDPI, 2020.
Other materials
Over the years, researchers have been exploring the exceptional mechanical properties and thermal stability of cellulose nanofibrils for application as reinforcing agents in thermoplastic matrices to improve the properties of the ensuing composites (Shen et al., 2020) (Table 4). BNC has been used as a reinforcement of poly(lactic acid) (PLA) membranes with visible improvements on the mechanical (higher Young’s modulus, compared to neat PLA) and thermal (increase in the initial and maximum degradation temperatures, compared to neat PLA) properties of the materials (Tomé et al., 2011). Unmodified and modified NFC also was used to reinforce poly(ε-caprolactone) (PCL) matrices using a melt-mixing approach, wherein the nanofillers are directly dispersed in the melted polymer and extruded to produce the final nanocomposite materials (Vilela et al., 2019a). The inclusion of NFC modified with cationic latex nanoparticles had no noticeable impact on the composite’s thermal properties (thermal stability up to 335–340°C). However, it improved the mechanical properties (increase in Young’s modulus of the PCL matrices from 41.1 to 52.5 MPa, with the inclusion of only 7.5 wt% of modified NFC) and accelerated the rate of enzymatic degradation of the nanocomposites (from 1.40% for the pure PCL to 1.67–2.21% for the modified nanocomposites, after 10 weeks). This study demonstrated the application of environmentally friendly techniques that improve the compatibility of cellulose nanofibrils with hydrophobic matrices and accelerate the rate at which composites biodegrade. Following a different strategy, Figueiredo et al. (2015b) produced BNC/PCL composites through the addition of the powdered PCL to the culture media, followed by hot pressing of the ensuing membranes, to melt the PCL that was retained inside the porous cellulose network.
As shown in the previous sections, nanocelluloses can harbor molecules with known functional roles using simple and cost-effective techniques. Nanopapers with inherent conductivity can be prepared by the simple vacuum filtration of NFC suspensions containing copper nanowires (Pinto et al., 2020a), and nanocomposite foams with enhanced fire-retardancy and thermal energy storage capacity may be prepared by solvent casting and freeze-drying of disintegrated BNC nanofibrils, graphene oxide, and phase change materials (Pinto et al., 2020b). Cellulose-based functional coatings prepared via the functionalization of nanofibrils with antibacterial moieties [e.g., silver (Martins et al., 2012) and zinc oxide nanoparticles (Martins et al., 2013)] are desirable for many substrates, namely paper materials for packaging solutions. Highly porous nanocellulose materials (e.g., aerogels, cryogels, xerogels, foams, and sponges) are of great interest not only in environmental remediation and catalysis but also in the construction field as thermal and sound insulators (Sun et al., 2021).
Since BNC nanofibrils are analogous to the fibrillar component of the extracellular matrix, nanostructured implantable materials (e.g., injectable hydrogels, tubular grafts, and scaffolds) have been extensively reported in the field of tissue engineering to promote cell regeneration in damaged sites (Carvalho et al., 2019). BNC and NFC nanofibrils can be manipulated to fabricate biomimetic spherical microparticles or microcapsules for cell culture applications (Carvalho et al., 2021b). Cellulosic nanofibrils have also been described as reinforcement additives in the development of bioinks for the 3D printing of hydrogel scaffolds, with application in tissue engineering and 3D cell culture (Teixeira et al., 2022a).
Exploiting protein fibrils for the production of novel materials
Proteins (or polypeptides) are among the most prevalent organic macromolecules in living organisms, taking part in important structural and biological roles (Silva et al., 2014a). As illustrated in Figure 5A, they are comprised of a linear sequence of amino acids (primary structure) with a specific local conformation (secondary structure) and a three-dimensional spatial arrangement (tertiary structure). Some proteins can also display a quaternary structure, resulting from the non-covalent interaction between different tertiary structures. These macromolecules can self-assemble into highly ordered amyloid nanofibrils with diameters of 5–10 nm, lengths of several micrometers, and aligned cross-β structures connected by a strong network of hydrogen bonds (Ye et al., 2019). The fibrillation phenomenon is typically linked to the misfolding of soluble proteins and accumulation of agglomerated amyloid nanofibrils (i.e., amyloid plaques) present in neurodegenerative conditions like Parkinson’s and Alzheimer’s (Salahuddin et al., 2021). Several biological proteins are known to self-assemble both in vivo and in vitro into nanofibrils with non-toxic and functional properties, such as the chorion proteins that protect silkworm eggs and the Pmel17 that is involved in human melanin formation (Ye et al., 2019).
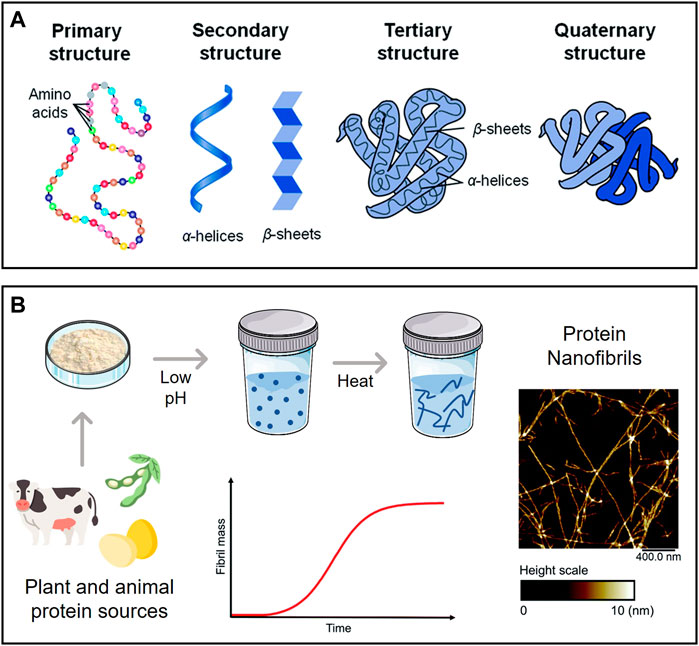
FIGURE 5. (A) Levels of protein organization; (B) Schematic representation of a typical process of protein nanofibril production using low pH and elevated temperature, with an illustrative example of their morphology (atomic force microscopy micrograph). Adapted and reprinted with permission from (Silva et al., 2014a). Copyright The Royal Society of Chemistry, 2014; and (Lendel and Solin, 2021). Copyright The Royal Society of Chemistry, 2021.
Amyloid nanofibrils can be produced in vitro from animal proteins, such as whey protein and hen egg white lysozyme, as study models to examine the principles and mechanisms governing protein self-assembly and fibrillation (Loveday et al., 2017). These protein nanofibrils are formed by partially or entirely unfolding the native structure of the protein, revealing potential amyloidogenic regions in the chain that can assemble into fibrils under specific conditions (Ye et al., 2019). Exposure to low pH (ca. 2) and high temperature is known to promote protein fibrillation (Figure 5B), as is the addition of certain chemicals (e.g., urea, alcohols) and enzymes (e.g., trypsin). The morphology and yield can be adjusted by varying the incubation time, temperature, pH, and ionic strength (Moayedzadeh et al., 2015). Furthermore, microwave irradiation (Carvalho et al., 2016) can shorten the time required to obtain the protein nanofibrils from days to a couple of hours. The use of alternative solvents with benign character as fibrillation agents (e.g., ionic liquids (Silva et al., 2018a) and deep eutectic solvents (Silva et al., 2018c)) is also able to reduce the fibrillation time.
Aside from providing new insights into the pathophysiology triggered by the formation of amyloid nanofibrils, the ability to obtain proteinaceous nanofibrils with exceptional mechanical and thermal stability from animal (e.g., whey protein, hen egg white lysozyme, milk casein) and plant-based (e.g., soy protein isolate, rice glutelin, α-zein) proteins offer an opportunity to use these renewable resources for the development of innovative materials with a high added value (Knowles and Mezzenga, 2016; Lendel and Solin, 2021; Meng et al., 2022). Furthermore, the discovery of functional nanofibrils in vivo opens the door to the laboratory synthesis of protein nanofibrils that preserve the inherent biological features of parent proteins, e.g., the antibacterial capabilities of lysozyme (Sarkar et al., 2020), which might be imparted onto the final materials (Table 5).
In the next sections, relevant examples of the development of new nanomaterials (films, patches and other materials) based on protein nanofibrils will be discussed in detail.
Films
Protein nanofibrils are incredibly robust, with mechanical strength akin to spider silk and far superior to most biological filaments, and interestingly, also show higher thermochemical stability than their native counterparts (Knowles and Mezzenga, 2016). Nevertheless, the inability of protein nanofibrils to form freestanding films, together with their distinct size and morphology, underline the unique role of protein nanofibrils as nanofillers or templates for the creation of innovative nanocomposite films (Ye et al., 2019). For instance, lysozyme nanofibrils (LNFs) can be combined with the filmogenic polysaccharide pullulan using a simple solvent casting technique (Silva et al., 2018b). The incorporation of LNFs imparted mechanical reinforcement abilities, as demonstrated by the increase in Young’s modulus from 1.69 to 2.50 GPa, and the reduction in the elongation at break from 6.63% to 1.34%, with the addition of 15 wt% of nanofibrils to the pullulan films. Moreover, the inclusion of LNFs endowed antioxidant (ca. 77%, using the DPPH assay) and antimicrobial properties (towards S. aureus) to the films, which are highly desirable for application in the food packaging sector.
Patches
Electrospinning approaches can be employed to obtain other nanomaterials with planar structures, such as patches, from protein suspensions (Lendel and Solin, 2021). For example, amyloid-like bovine serum albumin fibrils were combined with ampicillin to produce electrospun patches that enabled the controlled release of the hydrophilic drug (Kabay et al., 2017). Moreover, even after treatment with solvents prior to the electrospinning process and application of high voltages, the drug retained its antimicrobial action towards S. aureus and E. coli. In another work, gelatin/LNFs electrospun patches were designed for tissue regeneration, specifically as an implant for infarcted myocardium tissues (Carvalho et al., 2022). The patches displayed excellent mechanical properties (increase in Young’s modulus from 3 to 6 MPa, in wet state) and enhanced antioxidant activity (ca. 80%, using the DPPH assay), ascribed to the reinforcement with the protein nanofibrils. Furthermore, the materials had an increased bioresorbability rate compared to patches comprised only of gelatin (reduction in 10–15 days), highlighting the immense potential of LNFs as functional reinforcements.
Other materials
Owing to the increase in viscosity during fibrillation, which is related to the interfibrillar interactions facilitated by the high aspect ratio of the nanofibrils, protein nanofibril dispersions demonstrate impressive gelation capabilities, even at low concentrations (Lendel and Solin, 2021). This ability is responsible for the formation of hydro/aero-gels with high stability, which find applicability in the biomedical field as scaffolds for cell and tissue growth and bioink formulations used in 3D printing technology (Veiga et al., 2021). In this realm, composite hydrogels of LNFs and alginate were produced to formulate bioinks for extrusion-based 3D bioprinting (Figure 6A) (Teixeira et al., 2022b). The reinforcement of the alginate matrix with the protein nanofibrils was responsible for improving the shear-thinning behavior, which is crucial in the printing process (Figures 6B,C). As a proof of concept, the gels were loaded with immortalized human keratinocytes (HaCaT cells). After printing the scaffold structures, the suitability of the hydrogels was evaluated via cell viability. Not only were the structures able to maintain the cells alive during the studied time (7 days), but the structures printed with the bioinks containing LNFs showed enhanced cell proliferation (cell viability of ca. 88%) compared to the cell-laden hydrogels without the protein nanofibrils (cell viability of 75%), highlighting their bioactive role (Figure 6D).
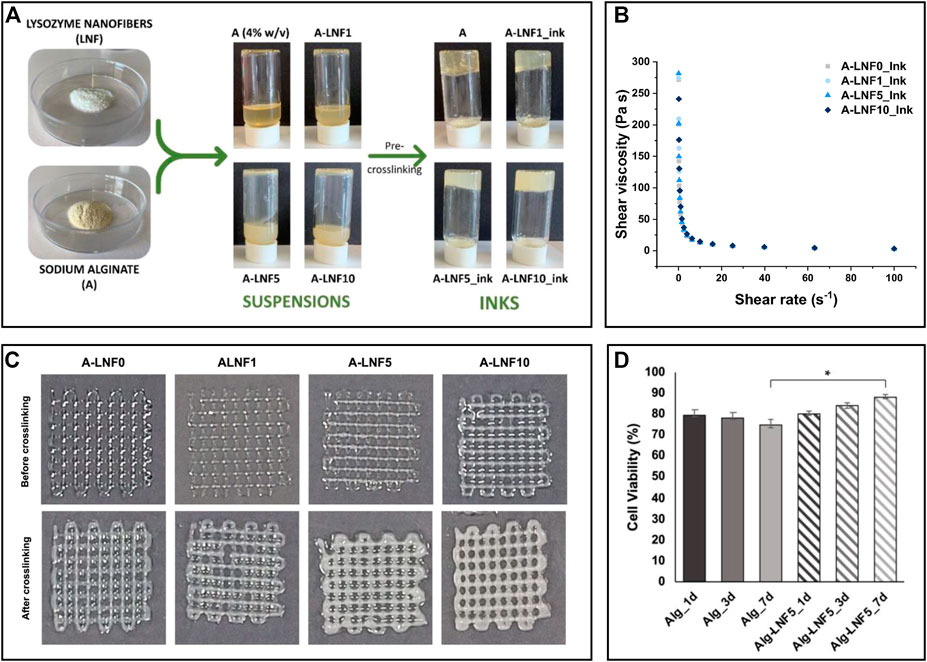
FIGURE 6. (A) Illustration of the preparation of the alginate and lysozyme nanofibrils (LNFs) bioinks; (B) Graphical representation of the shear viscosity of the inks with different contents of LNFs; (C) Optical micrographs of the printed scaffolds (2 layers) before and after crosslinking with CaCl2; (D) Graphical representation of the cell viability of HaCaT cells incorporated in the scaffolds after 1, 3 and 7 days of bioprinting. Reproduced with permission from (Teixeira et al., 2022b). Copyright MDPI, 2022.
The addition of protein nanofibrils is also known to alter the mechanical behavior of thermoplastic matrices. For instance, the blend of bovine insulin nanofibrils with poly(vinyl alcohol) resulted in increased stiffness of the films compared with the addition of the same amount (1 wt%) of non-fibrillar bovine insulin (Rao et al., 2012). The increase of other mechanical properties, such as elongation at break and elastic modulus, has also been reported with the blend of LNFs with poly (lactic acid) (Byrne et al., 2011) and a silicone elastomer (Oppenheim et al., 2010), respectively.
The partnership between nanocellulose and protein fibrils
The use of cellulose nanofibers or protein fibrils in combination with other (bio)polymers or bioactive molecules results in nanostructured materials with promising physicochemical properties and functionalities, which can take the form of membranes, films, patches, and other materials, as illustrated in the works mentioned above (Tables 1–5). Given the tremendous potential of cellulose and protein nanofibrils on the design of new functional materials, their assembly will certainly result in fibrillar materials with unique properties. Nonetheless, as far as we could gather, only three publications to date dealing with the joint use of nanocellulose and protein nanofibrils in advanced material production, namely in films and aerogels with application in water remediation (Silva et al., 2020a; Sorriaux et al., 2021) and patches for wound healing (Silva et al., 2020b), have been reported (Table 5).
Silva et al. (2020a) described for the first time the preparation of NFC/LNFs biobased films using a simple methodology of vacuum filtration of the water-based suspensions of nanocellulose (obtained from softwood) and protein nanofibrils (extracted from hen egg white). The dual nanofibrillar films exhibited superior mechanical properties compared to neat NFC films (which already have remarkable mechanical properties), namely an increase up to 2 GPa of the Young’s modulus and a concomitant decrease in the elongation at break. These results highlighted the structural reinforcement role of the lysozyme nanofibrils in the system, possibly due to the interactions between the hydroxyl and carboxyl groups of the nanocellulose fibrils and the amide groups of the LNFs. The adequacy of the NFC/LNFs films as biosorbents was evaluated in mercury-contaminated ultrapure and spring waters. After 24 h of contact at pH 11 (close to the isoelectric point of the lysozyme), the removal effectiveness reached a maximum of 99% and a residual concentration below the threshold value in waters intended for human consumption. The presence of amino acid side chains with multiple binding sites plays a crucial role in the adsorption of the Hg2+ ions.
In the same field, β-lactoglobulin nanofibrils were combined with polydopamine-coated cellulose nanofibrils (NFC) and crosslinked via periodate oxidation to produce biosorbent aerogels (Sorriaux et al., 2021). The adsorption capability of the aerogels was evaluated in water contaminated with an assortment of pollutants (e.g., dyes, pesticide/pharmaceutical agents, and heavy metal ions), with good efficiencies and fast adsorption rates in the removal of crystal violet dye (93%, 30 min), bisphenol A (92%, 5 min) and Pb2+ ions (95%, 5 min), specifically. In this case, the adsorption is facilitated by the presence of various functional groups (e.g., catechols, quinones amines, and aromatic moieties) in the polydopamine functional coating.
The combination of NFC and LNFs was also evaluated in the preparation of nanofibrillated patches for wound healing following two different approaches, viz., patches produced via vacuum filtration of the mixed NFC and LNF dispersions versus ones obtained through the sequential filtration of NFC and LNFs, respectively (Figure 7A) (Silva et al., 2020b). Scanning electron microscopy analysis revealed the excellent compatibility between NFC and LNF in the mixed nanofibrils patch and in the two distinct layers in the patch obtained by sequential deposition of NFC and LNF (Figure 7B). The mechanical properties differed due to the varying layouts. Compared to the pure NFC patch, the blended nanofibrils patch displayed an increase in Young’s modulus, as expected, from 4.4 to 6.7 GPa. This trend, however, was not mirrored in the layered nanofibrils patch, which exhibited a lower Young’s modulus than neat NFC (3.7 GPa), most likely due to the exclusive establishment of interfacial interactions between the functional groups of both nanofibrils. The inclusion of proteinaceous nanofibrils provided the patches with good UV-barrier properties, high antioxidant activity (up to 79.5%, using the DPPH assay), and antimicrobial activity against S. aureus (up to 3.5-log CFU mL−1 reduction), which was slightly higher in the layered patch due to the bacterium’s direct contact with the LNFs side (Figure 7C). Both NFC/LNFs patches were biocompatible toward the L929 fibroblast cell line and, in contrast to the pure NFC patch, promoted cell adhesion with high viability values (Figure 7C). The in vitro wound healing assay exhibited good migratory capacity of the cells on the surface of the patches, resulting in nearly complete occlusion of the simulated wound (Figure 7D). These findings point to the potential of the dual nanofibrils patches in wound healing improvement.
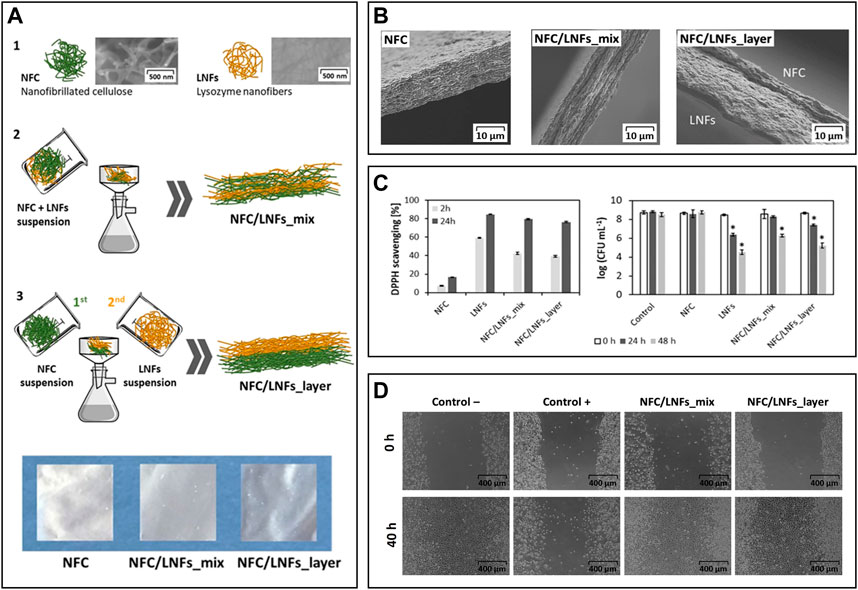
FIGURE 7. (A) Overview of the constituents (1) and the preparation of the nanocellulose (herein represented as NFC) and lysozyme nanofibrils (LNFs) patches via vacuum filtration by direct mixing of the suspensions (2) or using a layered approach (3), and digital photographs of the dry patches; (B) Scanning electron microscopy micrographs of the cross-section of the patches; (C) In vitro antioxidant activity (left) and antimicrobial activity (right) of the functional patches; (D) Optical micrographs of the scratch assay of the fibroblast cells after 40 h of exposure to both patches. Reproduced with permission from (Silva et al., 2020b). Copyright Elsevier, 2020.
Conclusion and future perspectives
In this review, we outlined the properties of cellulose and protein (amyloid) nanofibrils in the development of ecofriendly and sustainable advanced materials, namely membranes, films, and patches, among others (Tables 1–5). Owing to their excellent mechanical strength, these biobased nanofibrils are frequently used as reinforcement agents by compounding with thermoplastics such as PLA (Tomé et al., 2011) and PCL (Figueiredo et al., 2015b; Vilela et al., 2019a), or through combination with biopolymeric matrices like pullulan (Trovatti et al., 2012a; Trovatti et al., 2012b), resulting in materials with improved mechanical and thermal properties.
Apart from the reinforcement role, the remarkable physicochemical properties of nanofibrils (e.g., high water absorption capacity, high surface area, biocompatibility and biodegradability) are being exploited in the development of advanced functional materials (Tables 1–4). BNC is particularly interesting for some applications since its membranes are simple to obtain in tailored shapes and sizes (in situ moldability) to better suit the target application. Combining BNC with bioactive molecules or polymers with different functionalities can produce materials for widely different applications, which explains the deluge of works reported using this nanocellulosic form.
Overall, materials produced with BNC and NFC have received much attention in a variety of emerging fields, including fuel cell components (Gadim et al., 2014; Gadim et al., 2016; Vilela et al., 2016; Gadim et al., 2017; Vilela et al., 2017; Vilela et al., 2018b; Vilela et al., 2020a; Vilela et al., 2020c; Vilela et al., 2020b), water remediation (Vilela et al., 2019b), active food packaging (Vilela et al., 2019c; Moreirinha et al., 2020; Bastante et al., 2021), skin treatment (Silva et al., 2014b; Morais et al., 2019; Chantereau et al., 2020; Almeida et al., 2022), wound healing (Fonseca et al., 2020) and drug delivery (Trovatti et al., 2011b; Trovatti et al., 2012c; Silva et al., 2014c; Silva et al., 2020c; Saïdi et al., 2017; Chantereau et al., 2019b; Carvalho et al., 2020; Fonseca et al., 2021).
Contrary to cellulose nanofibrils, protein amyloid nanofibrils, apart from their excellent mechanical properties, biodegradability and biocompatibility, also carry the functional groups of their native amino acid chains, avoiding an additional step in modification. Given so, the ability to hold intrinsic functional properties, paired with their high surface area and capacity to mimic non-cellular components, is attracting tremendous interest in the use of protein nanofibrils as functional reinforcements for the development of films, patches and other materials (Table 5) with application in areas like active food packaging (Silva et al., 2018b), bioinks formulation (Teixeira et al., 2022b) and tissue regeneration (Carvalho et al., 2022).
Moreover, the design of dual-fibrillar systems comprising both nanocellulose and protein nanofibrils seems to be a promising approach, still in its infancy. Regardless, their successful partnership is notorious, particularly in the formulation of patches for biomedical treatments (Silva et al., 2020b) and in the development of films (Silva et al., 2020a) and aerogels (Sorriaux et al., 2021) for environmental remediation strategies. In these studies, only NFC and two protein nanofibrils (lysozyme and β-lactoglobulin) have been used; therefore, plenty of materials can be foreseen by the combination of other nanocelluloses with nanofibrils obtained from other protein sources.
Even though the transition to more sustainable biopolymeric nanofibrillated materials remains limited to the establishment of straightforward, economically viable, and speedier methods for nanofibril production, there is a clear potential for economic expansion in this field. The global nanocellulose market is estimated to reach USD 1,053.09 million by 2027, with a compound annual growth rate (CAGR) of 19.9% throughout the forecast period (2020–2027), demonstrating the clear interest in cellulosic nanofibrils (Fortune Business Insights, 2021). The functional protein industry is still primarily driven by uses for animal feed, nutraceuticals, and food and beverage supplementation (Markets and Markets, 2021). However, the growing interest in this research field over the past few years might be a driving factor towards the development of functional materials (Ye et al., 2019; Daniloski et al., 2021; Lendel and Solin, 2021; Peydayesh and Mezzenga, 2021; Vinayagam et al., 2022). Protein nanofibrils have significant advantageous characteristics and can be valuable building blocks in the creation of sophisticated nanostructured materials with intrinsic functional properties. Despite the significant progress that remains to be accomplished in this field, we foresee a growing interest in the use of nanocellulosic and protein nanofibrils, as well as their blends, as we begin to witness the merits of this partnership (Silva et al., 2020a; Silva et al., 2020b; Sorriaux et al., 2021).
Author contributions
Conceptualization, CSRF; writing–original draft preparation, ACQS; writing–review and editing, ACQS, AJDS, CV, CSRF; supervision, AJDS, CV, CSRF; funding acquisition, AJDS, CV, CSRF. All authors have read and agreed to the published version of the manuscript.
Funding
This work was developed within the scope of the project CICECO–Aveiro Institute of Materials, UIDB/50011/2020, UIDP/50011/2020 and LA/P/0006/2020, financed by national funds through the FCT/MCTES (PIDDAC) and project Cell4Janus: Engineering self-propelled cellulose-based Janus microrobots (PTDC/BII-BIO/1901/2021), financially supported by national funds (OE), through FCT/MCTES. FCT is also acknowledged for the doctoral grant to ACQS (SFRH/BD/140230/2018), and the research contracts under Scientific Employment Stimulus to CV (CEECIND/00263/2018 and 2021.01571.CEECIND) and CSRF (CEECIND/00464/2017).
Conflict of interest
The authors declare that the research was conducted in the absence of any commercial or financial relationships that could be construed as a potential conflict of interest.
Publisher’s note
All claims expressed in this article are solely those of the authors and do not necessarily represent those of their affiliated organizations, or those of the publisher, the editors and the reviewers. Any product that may be evaluated in this article, or claim that may be made by its manufacturer, is not guaranteed or endorsed by the publisher.
References
Abdelhamid, H. N., and Mathew, A. P. (2021). Cellulose-based materials for water remediation: Adsorption, catalysis, and antifouling. Front. Chem. Eng. 3, 790314. doi:10.3389/fceng.2021.790314
Abdul Khalil, H. P. S., Bhat, A. H., and Ireana Yusra, A. F. (2012). Green composites from sustainable cellulose nanofibrils: A review. Carbohydr. Polym. 87, 963–979. doi:10.1016/j.carbpol.2011.08.078
Almeida, I. F., Pereira, T., Silva, N. H. C. S., Gomes, F. P., Silvestre, A. J. D., Freire, C. S. R., et al. (2014). Bacterial cellulose membranes as drug delivery systems: An in vivo skin compatibility study. Eur. J. Pharm. Biopharm. 86, 332–336. doi:10.1016/j.ejpb.2013.08.008
Almeida, T., Moreira, P., Sousa, F. J., Pereira, C., Silvestre, A. J. D., Vilela, C., et al. (2022). Bioactive bacterial nanocellulose membranes enriched with Eucalyptus globulus labill. Leaves aqueous extract for anti-aging skin care applications. Materials 15, 1982. doi:10.3390/ma15051982
Almeida, T., Silvestre, A. J. D., Vilela, C., and Freire, C. S. R. (2021). Bacterial nanocellulose toward green cosmetics: Recent progresses and challenges. Int. J. Mol. Sci. 22, 2836. doi:10.3390/ijms22062836
Amara, C., El Mahdi, A., Medimagh, R., and Khwaldia, K. (2021). Nanocellulose-based composites for packaging applications. Curr. Opin. Green Sustain. Chem. 31, 100512. doi:10.1016/j.cogsc.2021.100512
Amin, U., Khan, M. K. I., Maan, A. A., Nazir, A., Riaz, S., Khan, M. U., et al. (2022). Biodegradable active, intelligent, and smart packaging materials for food applications. Food packag. Shelf Life 33, 100903. doi:10.1016/j.fpsl.2022.100903
Babitha, S., Rachita, L., Karthikeyan, K., Shoba, E., Janani, I., Poornima, B., et al. (2017). Electrospun protein nanofibers in healthcare: A review. Int. J. Pharm. X. 523, 52–90. doi:10.1016/j.ijpharm.2017.03.013
Barhoum, A., Pal, K., Rahier, H., Uludag, H., Kim, I. S., and Bechelany, M. (2019). Nanofibers as new-generation materials: From spinning and nano-spinning fabrication techniques to emerging applications. Appl. Mat. Today 17, 1–35. doi:10.1016/j.apmt.2019.06.015
Barhoum, A., Rasouli, R., Yousefzadeh, M., Rahier, H., and Bechelany, M. (2018). “Nanofiber technology: History and developments,” in Handbook of nanofibers. Editors H. Barhoum, M. Bechelany, and A. S. H. Makhlouf (Cham: Springer International Publishing), 1–42. doi:10.1007/978-3-319-42789-8_54-1
Bastante, C. C., Silva, N. H. C. S., Cardoso, L. C., Serrano, C. M., Martínez de la Ossa, E. J., Freire, C. S. R., et al. (2021). Biobased films of nanocellulose and mango leaf extract for active food packaging: Supercritical impregnation versus solvent casting. Food Hydrocoll. 117, 106709. doi:10.1016/j.foodhyd.2021.106709
Byrne, N., Hameed, N., Werzer, O., and Guo, Q. (2011). The preparation of novel nanofilled polymer composites using poly(l-lactic acid) and protein fibers. Eur. Polym. J. 47, 1279–1283. doi:10.1016/J.EURPOLYMJ.2010.12.002
Carvalho, J. P. F., Freire, C. S. R., and Vilela, C. (2021a). “Active packaging,” in Sustainable food processing and engineering challenges. Editor C. Galanakis (London: Academic Press, Elsevier), 315–341. doi:10.1016/B978-0-12-822714-5.00009-7
Carvalho, J. P. F., Silva, A. C. Q., Bastos, V., Oliveira, H., Pinto, R. J. B., Silvestre, A. J. D., et al. (2020). Nanocellulose-based patches loaded with hyaluronic acid and diclofenac towards aphthous stomatitis treatment. Nanomaterials 10, 628. doi:10.3390/nano10040628
Carvalho, J. P. F., Silva, A. C. Q., Silvestre, A. J. D., Freire, C. S. R., and Vilela, C. (2021b). Spherical cellulose micro and nanoparticles: A review of recent developments and applications. Nanomaterials 11, 2744. doi:10.3390/nano11102744
Carvalho, T., Ezazi, N. Z., Correia, A., Vilela, C., Santos, H. A., and Freire, C. S. R. (2022). Gelatin-lysozyme nanofibrils electrospun patches with improved mechanical, antioxidant, and bioresorbability properties for myocardial regeneration applications. Adv. Funct. Mat. 32, 2113390. doi:10.1002/adfm.202113390
Carvalho, T., Guedes, G., Sousa, F. L., Freire, C. S. R. R., and Santos, H. A. (2019). Latest advances on bacterial cellulose-based materials for wound healing, delivery systems, and tissue engineering. Biotechnol. J. 14, 1900059. doi:10.1002/biot.201900059
Carvalho, T., Pinto, R. J. B., Martins, M. A., Silvestre, A. J. D., and Freire, C. S. R. (2016). Timesaving microwave assisted synthesis of insulin amyloid fibrils with enhanced nanofiber aspect ratio. Int. J. Biol. Macromol. 92, 225–231. doi:10.1016/j.ijbiomac.2016.07.008
Cazón, P., and Vázquez, M. (2021). Bacterial cellulose as a biodegradable food packaging material: A review. Food Hydrocoll. 113, 106530. doi:10.1016/j.foodhyd.2020.106530
Chandana, A., Mallick, S. P., Dikshit, P. K., Singh, B. N., and Sahi, A. K. (2022). Recent developments in bacterial nanocellulose production and its biomedical applications. J. Polym. Environ. 30, 4040–4067. doi:10.1007/s10924-022-02507-0
Chantereau, G., Brown, N., Dourges, M.-A., Freire, C. S. R., Silvestre, A. J. D., Sebe, G., et al. (2019a). Silylation of bacterial cellulose to design membranes with intrinsic anti-bacterial properties. Carbohydr. Polym. 220, 71–78. doi:10.1016/j.carbpol.2019.05.009
Chantereau, G., Sharma, M., Abednejad, A., Neves, B. M., Sèbe, G., Coma, V., et al. (2019b). Design of nonsteroidal anti-inflammatory drug-based ionic liquids with improved water solubility and drug delivery. ACS Sustain. Chem. Eng. 7, 14126–14134. doi:10.1021/acssuschemeng.9b02797
Chantereau, G., Sharma, M., Abednejad, A., Vilela, C., Costa, E. M., Veiga, M., et al. (2020). Bacterial nanocellulose membranes loaded with vitamin B-based ionic liquids for dermal care applications. J. Mol. Liq. 302, 112547. doi:10.1016/j.molliq.2020.112547
Charreau, H., Cavallo, E., and Foresti, M. L. (2020). Patents involving nanocellulose: Analysis of their evolution since 2010. Carbohydr. Polym. 237, 116039. doi:10.1016/j.carbpol.2020.116039
Chen, C., Ding, W., Zhang, H., Zhang, L., Huang, Y., Fan, M., et al. (2022). Bacterial cellulose-based biomaterials: From fabrication to application. Carbohydr. Polym. 278, 118995. doi:10.1016/j.carbpol.2021.118995
Cherian, R. M., Tharayil, A., Varghese, R. T., Antony, T., Kargarzadeh, H., Chirayil, C. J., et al. (2022). A review on the emerging applications of nano-cellulose as advanced coatings. Carbohydr. Polym. 282, 119123. doi:10.1016/j.carbpol.2022.119123
Daniloski, D., Petkoska, A. T., Lee, N. A., Bekhit, A. E.-D., Carne, A., Vaskoska, R., et al. (2021). Active edible packaging based on milk proteins: A route to carry and deliver nutraceuticals. Trends Food Sci. Technol. 111, 688–705. doi:10.1016/j.tifs.2021.03.024
Du, H., Liu, W., Zhang, M., Si, C., Zhang, X., and Li, B. (2019). Cellulose nanocrystals and cellulose nanofibrils based hydrogels for biomedical applications. Carbohydr. Polym. 209, 130–144. doi:10.1016/j.carbpol.2019.01.020
Faria, M., Vilela, C., Mohammadkazemi, F., Silvestre, A. J. D., Freire, C. S. R., and Cordeiro, N. (2019a). Poly(glycidyl methacrylate)/bacterial cellulose nanocomposites: Preparation, characterization and post-modification. Int. J. Biol. Macromol. 127, 618–627. doi:10.1016/j.ijbiomac.2019.01.133
Faria, M., Vilela, C., Silvestre, A. J. D., Deepa, B., Resnik, M., Freire, C. S. R., et al. (2019b). Physicochemical surface properties of bacterial cellulose/polymethacrylate nanocomposites: An approach by inverse gas chromatography. Carbohydr. Polym. 206, 86–93. doi:10.1016/j.carbpol.2018.10.110
Fernandes, S. C. M., Sadocco, P., Alonso-Varona, A., Palomares, T., Eceiza, A., Silvestre, A. J. D., et al. (2013). Bioinspired antimicrobial and biocompatible bacterial cellulose membranes obtained by surface functionalization with aminoalkyl groups. ACS Appl. Mat. Interfaces 5, 3290–3297. doi:10.1021/am400338n
Figueiredo, A. R. P., Figueiredo, A. G. P. R., Silva, N. H. C. S., Barros-Timmons, A., Almeida, A., Silvestre, A. J. D., et al. (2015a). Antimicrobial bacterial cellulose nanocomposites prepared by in situ polymerization of 2-aminoethyl methacrylate. Carbohydr. Polym. 123, 443–453. doi:10.1016/j.carbpol.2015.01.063
Figueiredo, A. R. P. P., Vilela, C., Neto, C. P., Silvestre, A. J. D. D., and Freire, C. S. R. R. (2014). “Bacterial cellulose-based nanocomposites: Roadmap for innovative materials,” in Nanocellulose polymer nanocomposites. Editor V. K. Thakur (Hoboken, NJ, USA: John Wiley & Sons), 17–64. doi:10.1002/9781118872246.ch2
Figueiredo, A. R. P., Silvestre, A. J. D., Neto, C. P., and Freire, C. S. R. (2015b). In situ synthesis of bacterial cellulose/polycaprolactone blends for hot pressing nanocomposite films production. Carbohydr. Polym. 132, 400–408. doi:10.1016/j.carbpol.2015.06.001
Fonseca, D. F. S., Carvalho, J. P. F., Bastos, V., Oliveira, H., Moreirinha, C., Almeida, A., et al. (2020). Antibacterial multi-layered nanocellulose-based patches loaded with dexpanthenol for wound healing applications. Nanomaterials 10, 2469. doi:10.3390/nano10122469
Fonseca, D. F. S., Vilela, C., Pinto, R. J. B., Bastos, V., Oliveira, H., Catarino, J., et al. (2021). Bacterial nanocellulose-hyaluronic acid microneedle patches for skin applications: In vitro and in vivo evaluation. Mater. Sci. Eng. C 118, 111350. doi:10.1016/j.msec.2020.111350
Fonseca, D. F. S., Vilela, C., Silvestre, A. J. D., and Freire, C. S. R. (2019). A compendium of current developments on polysaccharide and protein-based microneedles. Int. J. Biol. Macromol. 136, 704–728. doi:10.1016/j.ijbiomac.2019.04.163
Fortune Business Insights (2021). Nanocellulose market size, growth and industry trends. Available at: https://www.fortunebusinessinsights.com/nanocellulose-market-104565 (Accessed June 13, 2022).
Frazão, C. J. R., Silva, N. H. C., Freire, C. S. R., Silvestre, A. J. D., Xavier, A. M. R. B., and Tavares, A. P. M. (2014). Bacterial cellulose as carrier for immobilization of laccase: Optimization and characterization. Eng. Life Sci. 14, 500–508. doi:10.1002/elsc.201400054
Freire, C. S. R., Fernandes, S. C. M., Silvestre, A. J. D., and Pascoal Neto, C. (2013). Novel cellulose-based composites based on nanofibrillated plant and bacterial cellulose: Recent advances at the university of Aveiro – a review. Holzforschung 67, 603–612. doi:10.1515/hf-2012-0127
Freire, C. S. R., and Vilela, C. (2022). Advanced nanocellulose-based materials: Production, properties, and applications. Nanomaterials 12, 431. doi:10.3390/nano12030431
Gadim, T. D. O., Figueiredo, A. G. P. R., Rosero-Navarro, N. C., Vilela, C., Gamelas, J. A. F., Barros-Timmons, A., et al. (2014). Nanostructured bacterial cellulose–poly(4-styrene sulfonic acid) composite membranes with high storage modulus and protonic conductivity. ACS Appl. Mat. Interfaces 6, 7864–7875. doi:10.1021/am501191t
Gadim, T. D. O., Loureiro, F. J. A., Vilela, C., Rosero-Navarro, N., Silvestre, A. J. D., Freire, C. S. R., et al. (2017). Protonic conductivity and fuel cell tests of nanocomposite membranes based on bacterial cellulose. Electrochim. Acta 233, 52–61. doi:10.1016/j.electacta.2017.02.145
Gadim, T. D. O., Vilela, C., Loureiro, F. J. A., Silvestre, A. J. D., Freire, C. S. R., and Figueiredo, F. M. L. (2016). Nafion ® and nanocellulose: A partnership for greener polymer electrolyte membranes. Ind. Crops Prod. 93, 212–218. doi:10.1016/j.indcrop.2016.01.028
Galiano, F., Briceño, K., Marino, T., Molino, A., Christensen, K. V., and Figoli, A. (2018). Advances in biopolymer-based membrane preparation and applications. J. Memb. Sci. 564, 562–586. doi:10.1016/j.memsci.2018.07.059
Hadidi, M., Jafarzadeh, S., Forough, M., Garavand, F., Alizadeh, S., Salehabadi, A., et al. (2022). Plant protein-based food packaging films; recent advances in fabrication, characterization, and applications. Trends Food Sci. Technol. 120, 154–173. doi:10.1016/j.tifs.2022.01.013
Hamimed, S., Abdeljelil, N., Landoulsi, A., Chatti, A., Aljabali, A. A. A., and Barhoum, A. (2022). “Bacterial cellulose nanofibers,” in Handbook of nanocelluloses (Cham: Springer International Publishing), 1–38. doi:10.1007/978-3-030-62976-2_15-1
Inácio, P. M. C., Medeiros, M. C. R., Carvalho, T., Félix, R. C., Mestre, A., Hubbard, P. C., et al. (2020). Ultra-low noise PEDOT:PSS electrodes on bacterial cellulose: A sensor to access bioelectrical signals in non-electrogenic cells. Org. Electron. 85, 105882. doi:10.1016/j.orgel.2020.105882
Kabay, G., Meydan, A. E., Kaleli Can, G., Demirci, C., and Mutlu, M. (2017). Controlled release of a hydrophilic drug from electrospun amyloid-like protein blend nanofibers. Mater. Sci. Eng. C 81, 271–279. doi:10.1016/J.MSEC.2017.08.003
Klemm, D., Cranston, E. D., Fischer, D., Gama, M., Kedzior, S. A., Kralisch, D., et al. (2018). Nanocellulose as a natural source for groundbreaking applications in materials science: Today’s state. Mat. TodayKidlingt. 21, 720–748. doi:10.1016/j.mattod.2018.02.001
Knowles, T. P. J., and Mezzenga, R. (2016). Amyloid fibrils as building blocks for natural and artificial functional materials. Adv. Mat. 28, 6546–6561. doi:10.1002/adma.201505961
Krishnaswamy, K., and Orsat, V. (2017). “Sustainable delivery systems through green nanotechnology,” in Nano- and microscale drug delivery systems (Amsterdam: Elsevier), 17–32. doi:10.1016/B978-0-323-52727-9.00002-9
Lacerda, P. S. S., Barros-Timmons, A. M. M. V., Freire, C. S. R., Silvestre, A. J. D., and Neto, C. P. (2013). Nanostructured composites obtained by ATRP sleeving of bacterial cellulose nanofibers with acrylate polymers. Biomacromolecules 14, 2063–2073. doi:10.1021/bm400432b
Lendel, C., and Solin, N. (2021). Protein nanofibrils and their use as building blocks of sustainable materials. RSC Adv. 11, 39188–39215. doi:10.1039/D1RA06878D
Ling, S., Chen, W., Fan, Y., Zheng, K., Jin, K., Yu, H., et al. (2018a). Biopolymer nanofibrils: Structure, modeling, preparation, and applications. Prog. Polym. Sci. 85, 1–56. doi:10.1016/j.progpolymsci.2018.06.004
Ling, S., Kaplan, D. L., and Buehler, M. J. (2018b). Nanofibrils in nature and materials engineering. Nat. Rev. Mat. 3, 18016. doi:10.1038/natrevmats.2018.16
Long, W., Ouyang, H., Hu, X., Liu, M., Zhang, X., Feng, Y., et al. (2021). State-of-art review on preparation, surface functionalization and biomedical applications of cellulose nanocrystals-based materials. Int. J. Biol. Macromol. 186, 591–615. doi:10.1016/j.ijbiomac.2021.07.066
Loveday, S. M., Anema, S. G., and Singh, H. (2017). β-Lactoglobulin nanofibrils: The long and the short of it. Int. Dairy J. 67, 35–45. doi:10.1016/j.idairyj.2016.09.011
Manan, S., Ullah, M. W., Ul-Islam, M., Shi, Z., Gauthier, M., and Yang, G. (2022). Bacterial cellulose: Molecular regulation of biosynthesis, supramolecular assembly, and tailored structural and functional properties. Prog. Mat. Sci. 129, 100972. doi:10.1016/j.pmatsci.2022.100972
Markets and Markets (2021). Functional proteins market [2020-2025]. Available at: https://www.marketsandmarkets.com/Market-Reports/functional-protein-market-140299581.html (Accessed July 21, 2022).
Martins, N. C. T., Freire, C. S. R., Neto, C. P., Silvestre, A. J. D., Causio, J., Baldi, G., et al. (2013). Antibacterial paper based on composite coatings of nanofibrillated cellulose and ZnO. Colloids Surfaces A Physicochem. Eng. Aspects 417, 111–119. doi:10.1016/j.colsurfa.2012.10.042
Martins, N. C. T., Freire, C. S. R., Pinto, R. J. B., Fernandes, S. C. M., Pascoal Neto, C., Silvestre, A. J. D., et al. (2012). Electrostatic assembly of Ag nanoparticles onto nanofibrillated cellulose for antibacterial paper products. Cellulose 19, 1425–1436. doi:10.1007/s10570-012-9713-5
Meftahi, A., Momeni Heravi, M. E., Baroum, A., Samyn, P., Najarzadeh, H., and Alibakhshi, S. (2021). “Cellulose nanofibers,” in Handbook of nanocelluloses. Editor A. Barhoum (Cham: Springer International Publishing), 1–30. doi:10.1007/978-3-030-62976-2_13-1
Meftahi, A., Samyn, P., Geravand, S. A., Khajavi, R., Alibkhshi, S., Bechelany, M., et al. (2022). Nanocelluloses as skin biocompatible materials for skincare, cosmetics, and healthcare: Formulations, regulations, and emerging applications. Carbohydr. Polym. 278, 118956. doi:10.1016/j.carbpol.2021.118956
Meng, Y., Wei, Z., and Xue, C. (2022). Protein fibrils from different food sources: A review of fibrillation conditions, properties, applications and research trends. Trends Food Sci. Technol. 121, 59–75. doi:10.1016/j.tifs.2022.01.031
Miyashiro, D., Hamano, R., and Umemura, K. (2020). A review of applications using mixed materials of cellulose, nanocellulose and carbon nanotubes. Nanomaterials 10, 186. doi:10.3390/nano10020186
Moayedzadeh, S., Madadlou, A., and Khosrowshahi asl, A. (2015). Formation mechanisms, handling and digestibility of food protein nanofibrils. Trends Food Sci. Technol. 45, 50–59. doi:10.1016/j.tifs.2015.05.005
Mohammadian, M., and Madadlou, A. (2018). Technological functionality and biological properties of food protein nanofibrils formed by heating at acidic condition. Trends Food Sci. Technol. 75, 115–128. doi:10.1016/j.tifs.2018.03.013
Mohammadian, M., Waly, M. I., Moghadam, M., Emam-Djomeh, Z., Salami, M., and Moosavi-Movahedi, A. A. (2020). Nanostructured food proteins as efficient systems for the encapsulation of bioactive compounds. Food Sci. Hum. Wellness 9, 199–213. doi:10.1016/j.fshw.2020.04.009
Morais, E. S., Silva, N. H. C. S., Sintra, T. E., Santos, S. A. O., Neves, B. M., Almeida, I. F., et al. (2019). Anti-inflammatory and antioxidant nanostructured cellulose membranes loaded with phenolic-based ionic liquids for cutaneous application. Carbohydr. Polym. 206, 187–197. doi:10.1016/j.carbpol.2018.10.051
Moreirinha, C., Vilela, C., Silva, N. H. C. S., Pinto, R. J. B., Almeida, A., Rocha, M. A. M., et al. (2020). Antioxidant and antimicrobial films based on brewers spent grain arabinoxylans, nanocellulose and feruloylated compounds for active packaging. Food Hydrocoll. 108, 105836. doi:10.1016/j.foodhyd.2020.105836
Muqeet, M., Mahar, R. B., Gadhi, T. A., and Ben Halima, N. (2020). Insight into cellulose-based-nanomaterials - a pursuit of environmental remedies. Int. J. Biol. Macromol. 163, 1480–1486. doi:10.1016/j.ijbiomac.2020.08.050
Nazrin, A., Sapuan, S. M., Zuhri, M. Y. M., Ilyas, R. A., Syafiq, R., and Sherwani, S. F. K. (2020). Nanocellulose reinforced thermoplastic starch (TPS), polylactic acid (PLA), and polybutylene succinate (PBS) for food packaging applications. Front. Chem. 8, 213. doi:10.3389/fchem.2020.00213
Nechyporchuk, O., Belgacem, M. N., and Bras, J. (2016). Production of cellulose nanofibrils: A review of recent advances. Ind. Crops Prod. 93, 2–25. doi:10.1016/j.indcrop.2016.02.016
Noremylia, M. B., Hassan, M. Z., and Ismail, Z. (2022). Recent advancement in isolation, processing, characterization and applications of emerging nanocellulose: A review. Int. J. Biol. Macromol. 206, 954–976. doi:10.1016/j.ijbiomac.2022.03.064
Oppenheim, T., Knowles, T. P. J., Lacour, S. P., and Welland, M. E. (2010). Fabrication and characterisation of protein fibril–elastomer composites. Acta Biomater. 6, 1337–1341. doi:10.1016/J.ACTBIO.2009.10.013
Owolabi, F. A. T., Deepu, A. G., Thomas, S., Shima, J., Rizal, S., Sri Aprilia, N. A., et al. (2020). “Green composites from sustainable cellulose nanofibrils,” in Encyclopedia of renewable and sustainable materials (Amsterdam: Elsevier), 81–94. doi:10.1016/B978-0-12-803581-8.11422-5
Peydayesh, M., and Mezzenga, R. (2021). Protein nanofibrils for next generation sustainable water purification. Nat. Commun. 12, 3248. doi:10.1038/s41467-021-23388-2
Pinto, R. J. B., Martins, M. A., Lucas, J. M. F., Vilela, C., Sales, A. J. M., Costa, L. C., et al. (2020a). Highly electroconductive nanopapers based on nanocellulose and copper nanowires: A new generation of flexible and sustainable electrical materials. ACS Appl. Mat. Interfaces 12, 34208–34216. doi:10.1021/acsami.0c09257
Pinto, S. C., Silva, N. H. C. S., Pinto, R. J. B., Freire, C. S. R., Duarte, I., Vicente, R., et al. (2020b). Multifunctional hybrid structures made of open-cell aluminum foam impregnated with cellulose/graphene nanocomposites. Carbohydr. Polym. 238, 116197. doi:10.1016/j.carbpol.2020.116197
Pradhan, D., Jaiswal, A. K., and Jaiswal, S. (2022). Emerging technologies for the production of nanocellulose from lignocellulosic biomass. Carbohydr. Polym. 285, 119258. doi:10.1016/j.carbpol.2022.119258
Qiu, J., Li, M., Ding, M., and Yao, J. (2022). Cellulose tailored semiconductors for advanced photocatalysis. Renew. Sustain. Energy Rev. 154, 111820. doi:10.1016/j.rser.2021.111820
Raghav, N., Sharma, M. R., and Kennedy, J. F. (2021). Nanocellulose: A mini-review on types and use in drug delivery systems. Carbohydr. Polym. Technol. Appl. 2, 100031. doi:10.1016/j.carpta.2020.100031
Rana, A. K., Frollini, E., and Thakur, V. K. (2021). Cellulose nanocrystals: Pretreatments, preparation strategies, and surface functionalization. Int. J. Biol. Macromol. 182, 1554–1581. doi:10.1016/j.ijbiomac.2021.05.119
Rao, S. P., Meade, S. J., Healy, J. P., Sutton, K. H., Larsen, N. G., Staiger, M. P., et al. (2012). Amyloid fibrils as functionalizable components of nanocomposite materials. Biotechnol. Prog. 28, 248–256. doi:10.1002/btpr.726
Raza, Z. A., Munim, S. A., and Ayub, A. (2021). Recent developments in polysaccharide-based electrospun nanofibers for environmental applications. Carbohydr. Res. 510, 108443. doi:10.1016/j.carres.2021.108443
Saïdi, L., Vilela, C., Oliveira, H., Silvestre, A. J. D., and Freire, C. S. R. (2017). Poly(N-methacryloyl glycine)/nanocellulose composites as pH-sensitive systems for controlled release of diclofenac. Carbohydr. Polym. 169, 357–365. doi:10.1016/j.carbpol.2017.04.030
Salahuddin, P., Fatima, M. T., Uversky, V. N., Khan, R. H., Islam, Z., and Furkan, M. (2021). The role of amyloids in Alzheimer’s and Parkinson’s diseases. Int. J. Biol. Macromol. 190, 44–55. doi:10.1016/j.ijbiomac.2021.08.197
Santos, R. F., Ribeiro, J. C. L., Franco de Carvalho, J. M., Magalhães, W. L. E., Pedroti, L. G., Nalon, G. H., et al. (2021). Nanofibrillated cellulose and its applications in cement-based composites: A review. Constr. Build. Mat. 288, 123122. doi:10.1016/j.conbuildmat.2021.123122
Sarkar, S., Gulati, K., Mishra, A., and Poluri, K. M. (2020). Protein nanocomposites: Special inferences to lysozyme based nanomaterials. Int. J. Biol. Macromol. 151, 467–482. doi:10.1016/j.ijbiomac.2020.02.179
Sayyed, A. J., Pinjari, D. V., Sonawane, S. H., Bhanvase, B. A., Sheikh, J., and Sillanpää, M. (2021). Cellulose-based nanomaterials for water and wastewater treatments: A review. J. Environ. Chem. Eng. 9, 106626. doi:10.1016/j.jece.2021.106626
Shaghaleh, H., Xu, X., and Wang, S. (2018). Current progress in production of biopolymeric materials based on cellulose, cellulose nanofibers, and cellulose derivatives. RSC Adv. 8, 825–842. doi:10.1039/C7RA11157F
Shen, R., Xue, S., Xu, Y., Liu, Q., Feng, Z., Ren, H., et al. (2020). Research progress and development demand of nanocellulose reinforced polymer composites. Polymers 12, 2113. doi:10.3390/polym12092113
Shen, Y., Levin, A., Kamada, A., Toprakcioglu, Z., Rodriguez-Garcia, M., Xu, Y., et al. (2021). From protein building blocks to functional materials. ACS Nano 15, 5819–5837. doi:10.1021/acsnano.0c08510
Shojaeiarani, J., Bajwa, D. S., and Chanda, S. (2021). Cellulose nanocrystal based composites: A review. Compos. Part C. Open Access 5, 100164. doi:10.1016/j.jcomc.2021.100164
Silva, A. C. Q., Silvestre, A. J. D., Freire, C. S. R., and Vilela, C. (2021a). in Fundamentals of natural Fibres and textiles. Editor M. I. H. Mondal (Cambridge: Woodhead Publishing, Elsevier). doi:10.1016/C2019-0-03400-0
Silva, A. C. Q., Silvestre, A. J. D., Vilela, C., and Freire, C. S. R. (2021b). Natural polymers-based materials: A contribution to a greener future. Molecules 27, 94. doi:10.3390/molecules27010094
Silva, N. H. C. S. C. S., Vilela, C., Marrucho, I. M., Freire, C. S. R. R., Pascoal Neto, C., and Silvestre, A. J. D. D. (2014a). Protein-based materials: From sources to innovative sustainable materials for biomedical applications. J. Mat. Chem. B 2, 3715. doi:10.1039/c4tb00168k
Silva, N. H. C. S., Drumond, I., Almeida, I. F., Costa, P., Rosado, C. F., Neto, C. P., et al. (2014b). Topical caffeine delivery using biocellulose membranes: A potential innovative system for cellulite treatment. Cellulose 21, 665–674. doi:10.1007/s10570-013-0114-1
Silva, N. H. C. S., Figueira, P., Fabre, E., Pinto, R. J. B., Pereira, M. E., Silvestre, A. J. D., et al. (2020a). Dual nanofibrillar-based bio-sorbent films composed of nanocellulose and lysozyme nanofibrils for mercury removal from spring waters. Carbohydr. Polym. 238, 116210. doi:10.1016/j.carbpol.2020.116210
Silva, N. H. C. S., Garrido-Pascual, P., Moreirinha, C., Almeida, A., Palomares, T., Alonso-Varona, A., et al. (2020b). Multifunctional nanofibrous patches composed of nanocellulose and lysozyme nanofibers for cutaneous wound healing. Int. J. Biol. Macromol. 165, 1198–1210. doi:10.1016/j.ijbiomac.2020.09.249
Silva, N. H. C. S., Mota, J. P., Santos de Almeida, T., Carvalho, J. P. F., Silvestre, A. J. D., Vilela, C., et al. (2020c). Topical drug delivery systems based on bacterial nanocellulose: Accelerated stability testing. Int. J. Mol. Sci. 21, 1262. doi:10.3390/ijms21041262
Silva, N. H. C. S., Pinto, R. J. B., Martins, M. A., Ferreira, R., Correia, I., Freire, C. S. R., et al. (2018a). Ionic liquids as promoters of fast lysozyme fibrillation. J. Mol. Liq. 272, 456–467. doi:10.1016/j.molliq.2018.08.064
Silva, N. H. C. S., Rodrigues, A. F., Almeida, I. F., Costa, P. C., Rosado, C., Neto, C. P., et al. (2014c). Bacterial cellulose membranes as transdermal delivery systems for diclofenac: In vitro dissolution and permeation studies. Carbohydr. Polym. 106, 264–269. doi:10.1016/j.carbpol.2014.02.014
Silva, N. H. C. S., Vilela, C., Almeida, A., Marrucho, I. M., and Freire, C. S. R. (2018b). Pullulan-based nanocomposite films for functional food packaging: Exploiting lysozyme nanofibers as antibacterial and antioxidant reinforcing additives. Food Hydrocoll. 77, 921–930. doi:10.1016/j.foodhyd.2017.11.039
Silva, N. H. C. S., Vilela, C., Pinto, R. J. B., Martins, M. A., Marrucho, I. M., and Freire, C. S. R. (2018c). Tuning lysozyme nanofibers dimensions using deep eutectic solvents for improved reinforcement ability. Int. J. Biol. Macromol. 115, 518–527. doi:10.1016/j.ijbiomac.2018.03.150
Silvestre, A. J. D., Freire, C. S. R., and Neto, C. P. (2014). Do bacterial cellulose membranes have potential in drug-delivery systems? Expert Opin. Drug Deliv. 11, 1113–1124. doi:10.1517/17425247.2014.920819
Soroush, E., Mohammadpour, Z., Kharaziha, M., Bakhsheshi-Rad, H. R., and Berto, F. (2022). Polysaccharides-based nanofibrils: From tissue engineering to biosensor applications. Carbohydr. Polym. 291, 119670. doi:10.1016/j.carbpol.2022.119670
Sorriaux, M., Sorieul, M., and Chen, Y. (2021). Bio-based and robust polydopamine coated nanocellulose/amyloid composite aerogel for fast and wide-spectrum water purification. Polymers 13, 3442. doi:10.3390/polym13193442
Squinca, P., Bilatto, S., Badino, A. C., and Farinas, C. S. (2022). The use of enzymes to isolate cellulose nanomaterials: A systematic map review. Carbohydr. Polym. Technol. Appl. 3, 100212. doi:10.1016/j.carpta.2022.100212
Subhedar, A., Bhadauria, S., Ahankari, S., and Kargarzadeh, H. (2021). Nanocellulose in biomedical and biosensing applications: A review. Int. J. Biol. Macromol. 166, 587–600. doi:10.1016/j.ijbiomac.2020.10.217
Sun, Y., Chu, Y., Wu, W., and Xiao, H. (2021). Nanocellulose-based lightweight porous materials: A review. Carbohydr. Polym. 255, 117489. doi:10.1016/j.carbpol.2020.117489
Teixeira, M. C., Lameirinhas, N. S., Carvalho, J. P. F., Silvestre, A. J. D., Vilela, C., and Freire, C. S. R. (2022a). A guide to polysaccharide-based hydrogel bioinks for 3D bioprinting applications. Int. J. Mol. Sci. 23, 6564. doi:10.3390/ijms23126564
Teixeira, M. C., Lameirinhas, N. S., Carvalho, J. P. F., Valente, B. F. A., Luís, J., Pires, L., et al. (2022b). Alginate-lysozyme nanofibers hydrogels with improved rheological behavior, printability and biological properties for 3D bioprinting applications. Nanomaterials 12, 2190. doi:10.3390/nano12132190
Thomas, P., Duolikun, T., Rumjit, N. P., Moosavi, S., Lai, C. W., Bin Johan, M. R., et al. (2020). Comprehensive review on nanocellulose: Recent developments, challenges and future prospects. J. Mech. Behav. Biomed. Mat. 110, 103884. doi:10.1016/j.jmbbm.2020.103884
Tomé, L. C., Pinto, R. J. B., Trovatti, E., Freire, C. S. R., Silvestre, A. J. D., Neto, C. P., et al. (2011). Transparent bionanocomposites with improved properties prepared from acetylated bacterial cellulose and poly(lactic acid) through a simple approach. Green Chem. 13, 419. doi:10.1039/c0gc00545b
Trache, D., Tarchoun, A. F., Derradji, M., Hamidon, T. S., Masruchin, N., Brosse, N., et al. (2020). Nanocellulose: From fundamentals to advanced applications. Front. Chem. 8, 392. doi:10.3389/fchem.2020.00392
Trovatti, E., Fernandes, S. C. M., Rubatat, L., Freire, C. S. R., Silvestre, A. J. D., and Neto, C. P. (2012a). Sustainable nanocomposite films based on bacterial cellulose and pullulan. Cellulose 19, 729–737. doi:10.1007/s10570-012-9673-9
Trovatti, E., Fernandes, S. C. M., Rubatat, L., Perez, D., da, S., Freire, C. S. R., et al. (2012b). Pullulan–nanofibrillated cellulose composite films with improved thermal and mechanical properties. Compos. Sci. Technol. 72, 1556–1561. doi:10.1016/j.compscitech.2012.06.003
Trovatti, E., Freire, C. S. R., Pinto, P. C., Almeida, I. F., Costa, P., Silvestre, A. J. D., et al. (2012c). Bacterial cellulose membranes applied in topical and transdermal delivery of lidocaine hydrochloride and ibuprofen: In vitro diffusion studies. Int. J. Pharm. X. 435, 83–87. doi:10.1016/j.ijpharm.2012.01.002
Trovatti, E., Serafim, L. S., Freire, C. S. R., Silvestre, A. J. D., and Neto, C. P. (2011a). Gluconacetobacter sacchari: An efficient bacterial cellulose cell-factory. Carbohydr. Polym. 86, 1417–1420. doi:10.1016/j.carbpol.2011.06.046
Trovatti, E., Silva, N. H. C. S., Duarte, I. F., Rosado, C. F., Almeida, I. F., Costa, P., et al. (2011b). Biocellulose membranes as supports for dermal release of lidocaine. Biomacromolecules 12, 4162–4168. doi:10.1021/bm201303r
United Nations (2015). Transforming our world: The 2030 Agenda for sustainable development. U. N. Gen. Assem 1, 529–567. doi:10.1891/9780826190123.ap02
Veiga, A., Silva, I. V., Duarte, M. M., and Oliveira, A. L. (2021). Current trends on protein driven bioinks for 3D printing. Pharmaceutics 13, 1444. doi:10.3390/pharmaceutics13091444
Vilela, C., Cordeiro, D. M., Boas, J. V., Barbosa, P., Nolasco, M., Vaz, P. D., et al. (2020a). Poly(4-styrene sulfonic acid)/bacterial cellulose membranes: Electrochemical performance in a single-chamber microbial fuel cell. Bioresour. Technol. Rep. 9, 100376. doi:10.1016/j.biteb.2019.100376
Vilela, C., Engström, J., Valente, B. F. A., Jawerth, M., Carlmark, A., and Freire, C. S. R. (2019a). Exploiting poly( ɛ -caprolactone) and cellulose nanofibrils modified with latex nanoparticles for the development of biodegradable nanocomposites. Polym. Compos. 40, 1342–1353. doi:10.1002/pc.24865
Vilela, C., Gadim, T. D. O., Silvestre, A. J. D., Freire, C. S. R., and Figueiredo, F. M. L. (2016). Nanocellulose/poly(methacryloyloxyethyl phosphate) composites as proton separator materials. Cellulose 23, 3677–3689. doi:10.1007/s10570-016-1050-7
Vilela, C., Kurek, M., Hayouka, Z., Röcker, B., Yildirim, S., Antunes, M. D. C., et al. (2018a). A concise guide to active agents for active food packaging. Trends Food Sci. Technol. 80, 212–222. doi:10.1016/j.tifs.2018.08.006
Vilela, C., Martins, A., Sousa, N., Silvestre, A., Figueiredo, F., and Freire, C. (2018b). Poly(bis[2-(methacryloyloxy)ethyl] phosphate)/bacterial cellulose nanocomposites: Preparation, characterization and application as polymer electrolyte membranes. Appl. Sci. (Basel). 8, 1145. doi:10.3390/app8071145
Vilela, C., Morais, J. D., Silva, A. C. Q., Muñoz-Gil, D., Figueiredo, F. M. L. L., Silvestre, A. J. D. D., et al. (2020b). Flexible nanocellulose/lignosulfonates ion-conducting separators for polymer electrolyte fuel cells. Nanomaterials 10, 1713. doi:10.3390/nano10091713
Vilela, C., Moreirinha, C., Almeida, A., Silvestre, A. J. D., and Freire, C. S. R. (2019b). Zwitterionic nanocellulose-based membranes for organic dye removal. Materials 12, 1404. doi:10.3390/ma12091404
Vilela, C., Moreirinha, C., Domingues, E. M., Figueiredo, F. M. L., Almeida, A., and Freire, C. S. R. (2019c). Antimicrobial and conductive nanocellulose-based films for active and intelligent food packaging. Nanomaterials 9, 980. doi:10.3390/nano9070980
Vilela, C., Oliveira, H., Almeida, A., Silvestre, A. J. D., and Freire, C. S. R. (2019d). Nanocellulose-based antifungal nanocomposites against the polymorphic fungus Candida albicans. Carbohydr. Polym. 217, 207–216. doi:10.1016/j.carbpol.2019.04.046
Vilela, C., Silva, A. C. Q., Domingues, E. M., Gonçalves, G., Martins, M. A., Figueiredo, F. M. L., et al. (2020c). Conductive polysaccharides-based proton-exchange membranes for fuel cell applications: The case of bacterial cellulose and fucoidan. Carbohydr. Polym. 230, 115604. doi:10.1016/j.carbpol.2019.115604
Vilela, C., Silvestre, A. J. D., Figueiredo, F. M. L., and Freire, C. S. R. (2019e). Nanocellulose-based materials as components of polymer electrolyte fuel cells. J. Mat. Chem. A 7, 20045–20074. doi:10.1039/C9TA07466J
Vilela, C., Sousa, N., Pinto, R. J. B., Silvestre, A. J. D., Figueiredo, F. M. L., and Freire, C. S. R. (2017). Exploiting poly(ionic liquids) and nanocellulose for the development of bio-based anion-exchange membranes. Biomass Bioenergy 100, 116–125. doi:10.1016/j.biombioe.2017.03.016
Vinayagam, V., Murugan, S., Kumaresan, R., Narayanan, M., Sillanpää, M., Vo, D.-V. N., et al. (2022). Protein nanofibrils as versatile and sustainable adsorbents for an effective removal of heavy metals from wastewater: A review. Chemosphere 301, 134635. doi:10.1016/j.chemosphere.2022.134635
Wang, L., Li, K., Copenhaver, K., Mackay, S., Lamm, M. E., Zhao, X., et al. (2021). Review on nonconventional fibrillation methods of producing cellulose nanofibrils and their applications. Biomacromolecules 22, 4037–4059. doi:10.1021/acs.biomac.1c00640
Wang, Q., Liu, S., Liu, J., Sun, J., Zhang, Z., and Zhu, Q. (2022). Sustainable cellulose nanomaterials for environmental remediation - achieving clean air, water, and energy: A review. Carbohydr. Polym. 285, 119251. doi:10.1016/j.carbpol.2022.119251
Wang, W., Xue, C., and Mao, X. (2020a). Chitosan: Structural modification, biological activity and application. Int. J. Biol. Macromol. 164, 4532–4546. doi:10.1016/j.ijbiomac.2020.09.042
Wang, X., Wang, Q., and Xu, C. (2020b). Nanocellulose-based inks for 3D bioprinting: Key aspects in research development and challenging perspectives in applications—a mini review. Bioengineering 7, 40. doi:10.3390/bioengineering7020040
Xiao, J., Li, H., Zhang, H., He, S., Zhang, Q., Liu, K., et al. (2022). Nanocellulose and its derived composite electrodes toward supercapacitors: Fabrication, properties, and challenges. J. Bioresour. Bioprod. 7, 245–269. doi:10.1016/j.jobab.2022.05.003
Ye, X., Lendel, C., Langton, M., Olsson, R. T., and Hedenqvist, M. S. (2019). “Protein nanofibrils: Preparation, properties, and possible applications in industrial nanomaterials,” in Industrial applications of nanomaterials. Editors S. Thomas, Y. Grohens, and Y. B. Pottathara (Amsterdam: Elsevier), 29–63. doi:10.1016/B978-0-12-815749-7.00002-5
Keywords: cellulose, proteins, nanofibrils, nanostructured materials, advanced materials, sustainability
Citation: Silva ACQ, Silvestre AJD, Vilela C and Freire CSR (2022) Cellulose and protein nanofibrils: Singular biobased nanostructures for the design of sustainable advanced materials. Front. Bioeng. Biotechnol. 10:1059097. doi: 10.3389/fbioe.2022.1059097
Received: 30 September 2022; Accepted: 25 November 2022;
Published: 13 December 2022.
Edited by:
Sidney JL. Ribeiro, São Paulo State University, BrazilReviewed by:
Nuno Gonçalo Azoia, Aquitex S.A., PortugalTheo G.M. Van De Ven, McGill University, Canada
Copyright © 2022 Silva, Silvestre, Vilela and Freire. This is an open-access article distributed under the terms of the Creative Commons Attribution License (CC BY). The use, distribution or reproduction in other forums is permitted, provided the original author(s) and the copyright owner(s) are credited and that the original publication in this journal is cited, in accordance with accepted academic practice. No use, distribution or reproduction is permitted which does not comply with these terms.
*Correspondence: Carmen S. R. Freire, Y2ZyZWlyZUB1YS5wdA==