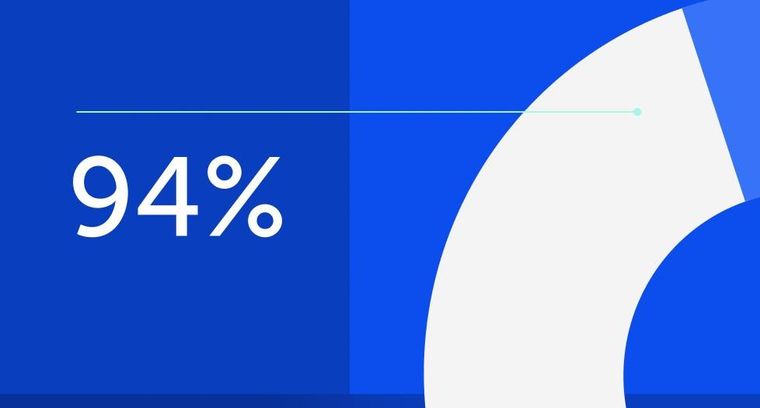
94% of researchers rate our articles as excellent or good
Learn more about the work of our research integrity team to safeguard the quality of each article we publish.
Find out more
REVIEW article
Front. Bioeng. Biotechnol., 14 November 2022
Sec. Biomaterials
Volume 10 - 2022 | https://doi.org/10.3389/fbioe.2022.1058251
This article is part of the Research TopicBiomaterials In AsiaView all 36 articles
Intervertebral disc (IVD) degeneration (IDD) is the most universal pathogenesis of low back pain (LBP), a prevalent and costly medical problem across the world. Persistent low back pain can seriously affect a patient’s quality of life and even lead to disability. Furthermore, the corresponding medical expenses create a serious economic burden to both individuals and society. Intervertebral disc degeneration is commonly thought to be related to age, injury, obesity, genetic susceptibility, and other risk factors. Nonetheless, its specific pathological process has not been completely elucidated; the current mainstream view considers that this condition arises from the interaction of multiple mechanisms. With the development of medical concepts and technology, clinicians and scientists tend to intervene in the early or middle stages of intervertebral disc degeneration to avoid further aggravation. However, with the aid of modern delivery systems, it is now possible to intervene in the process of intervertebral disc at the cellular and molecular levels. This review aims to provide an overview of the main mechanisms associated with intervertebral disc degeneration and the delivery systems that can help us to improve the efficacy of intervertebral disc degeneration treatment.
Low back pain (LBP) is a widespread health problem characterized by a long duration and high disability rate that is a cause of widespread concern (Katz, 2006). LBP seriously affects the quality of life and is one of the top three causes of disability in developed countries (Murray et al., 2012; Hartvigsen et al., 2018). According to past statistics, it is estimated that LBP affects almost 700 million people worldwide, although this number is increasing (GBD 2017 Disease and Injury Incidence and Prevalence Collaborators, 2017; Jin et al., 2020). LBP also imposes a significant burden on society and the economy. LBP is responsible for more than 30% of work absences and its directly related costs are as high as $90 billion annually in the United States alone (Dieleman et al., 2016; Hartvigsen et al., 2018).
The etiology of LBP is complex and has yet to be fully elucidated. The current consensus is that LBP is caused by multiple factors, including biological, psychological and social factors (Vlaeyen et al., 2018). Despite many cognitive limitations, Intervertebral disc (IVD) degeneration (IDD) is definitely an important cause of LBP (Cheung et al., 2009; Mohd Isa et al., 2022). Interestingly, not all patients with IDD develop LBP. A previous study estimated that between 26% and 42% of IDD patients experience significant LBP (Peng, 2013; Mohd Isa et al., 2022). There are two main possible reasons for such pain, one is the sagittal imbalance of the spine due to disc height reduction which may lead to soreness from exertion of the surrounding muscles; the other reason is the inflammation caused by damage to the disc and its adjacent tissues.
The three-joint structure between adjacent vertebral bodies plays an important role in motion, weight-bearing, maintaining flexibility and protecting vulnerable tissues. IVDs are a crucial fibrocartilage component of this structure and regulate the amounts of bound water to ensure that the intrinsic intradiscal pressure is within the appropriate range (Urban and McMullin, 1988; Vergroesen et al., 2014). The intradiscal pressure is approximately 0.1 MPa–2.3 MPa according to its loading (Sato et al., 1999; Wilke et al., 1999). The ability to retain water under stress decreases in degenerating IVDs; this is reflected by the reduction of disc high and intradiscal pressure (Iatridis et al., 2013; Lee et al., 2013; Vergroesen et al., 2014). At the same time, the reduced intradiscal pressure increases the shear stress concentrations in IVDs, thus leading to subsequent complications such as herniation (Adams et al., 1996; Andersson et al., 2006). The main anatomical structure of an IVD consists of three parts, the nucleus pulposus (NP), the annulus fibrosus (AF) and the cartilaginous endplates (CEP). The healthy NP is a highly hydrated tissue that is rich in proteoglycans and generates an intradiscal pressure and evenly distributes pressure on the adjacent endplates (Iatridis et al., 2013; Vergroesen et al., 2014). The AF is composed of collagen fibers, which together with lamellae and proteoglycans, form a multilayered concentric structure (Nerurkar et al., 2010). The AF is tensioned by intradiscal pressure and also plays a role in protecting and constraining the NP inside it (Iatridis et al., 2013). It is worth noting that the majority of the AF is avascular and nerve-free, thus making its renewal and healing highly dependent on sparsely distributed cell populations. At the same time, fragile blood supply and nutritional support mean that once damaged, the AF experiences difficulty in repairing itself (Holm et al., 1981). In a healthy state, the endplate is composed of homogeneous hyaline cartilage of uniform thickness (Rutges et al., 2013). Almost all nutrients are transported to the IVD via the CEPs (Malandrino et al., 2014; Bowles and Setton, 2017). Cartilage damage is an important characteristic of IDD, and is a similar condition to osteoarthritis (Wang et al., 2012; Zhu et al., 2022b; Francisco et al., 2022). Mineralized endplates may hinder nutrient transport to the IVD, and insufficient nutrient supply has been considered as one of the causes of IDD (Roberts et al., 1996; Benneker et al., 2014; Huang et al., 2014; Malandrino et al., 2014). Collectively, the IVD components can affect each other and any single site of injury or degeneration can lead to overall IDD.
In clinical work, magnetic resonance imaging (MRI) of the spine is the most commonly used method to detect IDD. There are some IDD classification methods based on T2-weighted MRI. Pfirrmann divided IDD into five grades (grade I to grade V) according to structural heterogeneity, the degree of differentiation between the NP and AF, disc height and signal density (Pfirrmann et al., 2001). In 2007, Griffith made an adjustment based on the previous classification by classifying IDD into eight grades (grade I to VIII). Unfortunately, T2-weighted MRI has irreparable limitations in detecting the early stage of IDD. Bruno et al. (Zobel et al., 2012) used T1ρ to identify early IDD and found that this system achieved good discrimination performance. In addition, more advanced equipment, along with the development of artificial intelligence (AI) technology, provide new directions for the detection of IDD (Sher et al., 2019; Gao et al., 2021). At present, there are three main approaches to treating LBP caused by IDD, including pharmacological therapies, physical and psychological therapies, and surgical interventions (Foster et al., 2018). Nevertheless, all of these approaches are remedial for patients with IDD who already have obvious symptoms. Blocking disease progression at an early stage can further reduce symptoms and complications and improve quality of life. Benefiting from the rapid development of modern biotechnology, an increasing number of biomarkers have been detected, thus making it possible for us to intervene in the development of IDD. However, an inevitable problem is how to deliver the required substances to the appropriate location to achieve effective intervention. Therefore, in the present study, we review the mainstream understanding of IDD-related pathological mechanisms and summarize the delivery systems that could effectively intervene in these mechanisms. Furthermore, we analyzed the classification, application and clinical application prospects of these systems.
The pathophysiology of IDD is intricate and has yet to be explained fully. Several pathological changes of IVD are thought to be related to IDD. These pathological mechanisms involve almost all levels of the IVD, from the microscopic level such as gene and cytokine expression to the macroscopic level such as structural loading and composition changes. These changes are interconnected and create a vicious cycle (Figure 1). Enhancing our understanding of the pathological processes involved will provide new opportunities and challenges for the treatment of IDD.
FIGURE 1. Several major pathological mechanisms in IDD are interconnected (The Figure was partly generated using Servier Medical Art, provided by Servier, licensed under a Creative Commons Attribution 3.0 unported license).
The instability of the mechanical structure of the IVD is an important cause of complications such as annulus rupture, herniation and nerve extrusion (Adams and Roughley, 2006; Vergroesen et al., 2014; Desmoulin et al., 2020). In recent years, biomechanical changes have been progressively considered as one of the pathological processes of IDD; this is closely related to metabolism, cell renewal and the inflammatory response of IVD tissues (Chan et al., 2011; Neidlinger-Wilke et al., 2012; Desmoulin et al., 2020). Because the IVD is avascular, diffusion is the main transport mechanism used to move nutrients and oxygen (Desmoulin et al., 2020). In addition, due to low oxygen content, IVD cells perform anaerobic respiration which consumes more glucose and produces more metabolic toxicity than aerobic respiration (Bartels et al., 1998). Previous studies have confirmed that excessive loading not only obstructs the diffusion of nutrients and oxygen but also leads to lactic acid accumulation in the NP and AF regions, accompanied by local PH reduction (Huang and Gu, 2008; Wang et al., 2013). Two in vitro experiments using human tissues revealed that the diffusion of glucose by AF and CEP diminished with increasing loading (Jackson et al., 2012; Wu et al., 2016). This may be related to the narrowing of the microscopic pore size due to compression. Unfortunately, these results have not yet been verified with in vivo data due to a lack of in vivo measurement techniques (Desmoulin et al., 2020; Shalash et al., 2021; McDonnell and Buckley, 2022).
Biomechanics also affect cells and their extracellular matrix (ECM). Biomechanical changes affect cellular homeostasis by regulating the expression of several substances, such as matrix metalloproteinases (MMP), cytokines and growth factors (Phillips et al., 2013a; Vo et al., 2013; Neidlinger-Wilke et al., 2014). These substances are bound up with the degradation of the ECM, activation of the inflammatory response and cell loss. Previous studies have shown that tensile strain can cause IVD cells to reduce the expression of proteoglycan and collagen II; these are important for maintaining normal disc performance (Court et al., 2001; Hutton et al., 2002). Svenja et al. (Illien-Jünger et al., 2010) reported that high-frequency loading resulted in cell death and an increase in MMP-13 expression. An in vivo study in a mouse model indicated that excessive load exposure resulted in a reduction in tissue inhibitor of matrix metalloproteinase-3 (TIMP-3) and an increase in a disintegrin and a metalloproteinase with thrombospondin motifs-4 (ADAMTS-4); this could relate to an early stage of IDD (Wuertz et al., 2009). It is important to note that a moderate dynamic compressive load is beneficial for IVDs (Walsh and Lotz, 2004; Wang et al., 2007; Paul et al., 2012). In addition, a distinct compressive force on the IVD can cause inflammatory responses; this has been verified in both in vivo and in vitro models (Wang et al., 2007; Paul et al., 2013; Gawri et al., 2014). Structural deficits in IVDs, such as herniation, can cause immune cell activation and infiltration; these cells include macrophages, neutrophils and T cells (Kokubo et al., 2008; Risbud and Shapiro, 2014). Meanwhile, the loss of intradiscal pressure significantly elevates the release of inflammatory cytokines such as interleukin (IL)-1 and-6 (Dudli et al., 2014). Interestingly, the migration of immune cells into the disc is accompanied by the appearance of nerve fibers arising from the dorsal root ganglion (DRG) (Martínez-Lavín, 2021). These changes are thought to contribute to the sensation of pain. Of note, almost all astronauts experience LBP when the gravity environment changes dramatically, again demonstrating the importance of biomechanics in IVD (Bailey et al., 2018; Lazzari et al., 2021).
Degradation, rebuilding and composition changes are crucial characteristics of IDD pathology. In fact, it is quite common to induce IDD animal models by damaging the ECM, thus demonstrating the importance of the ECM in maintaining IVD stability. The normal ECM consists of two main substances. One is proteoglycans; aggrecan is the most abundant proteoglycan in the NP (Roughley et al., 2006). The polyanionic proteoglycans provide the normal physiological osmotic pressure within the NP, which is crucial in maintaining tissue hydration and buffering compressive forces during daily activities. The water content of the IVD increases from the outer layer of AF to the NP located in the center, as does the concentration of proteoglycans (Feng et al., 2006). In IDD, aggrecan is cleaved from the hyaluronic acid backbone; this reduces its ability to bind water (Yurube et al., 2012; latridis et al., 2011). Another main substance in ECM is collagen. Type I and II collagen are the most abundant collagens in the disc (approximately 80%). The collagen framework of the disc is crucial for preserving normal architecture and function (Roughley et al., 2006). AF cells possess mostly type I while NP cells possess mostly type II collagen. From an anatomical structure viewpoint, from the inside to the outside of the IVD, the content of type II collagen gradually decreases and the content of type I collagen increases (Scott et al., 1994). Previous studies have observed the gradual transformation of notochordal cells into chondrocyte-like cells with increasing age; this reduces the expression of type II collagen and proteoglycans (Scott et al., 1994; Antoniou et al., 1996; Boos et al., 2002; Zhao et al., 2007). Type II collagen has better mechanical compliance than type I; thus, a reduction in type II collagen results in an uneven distribution of stress across the IVD. In addition, the loss of water-binding potential is reflected in the reduction of IVD height (Iatridis et al., 2013; Vergroesen et al., 2014). Collectively, these changes further deteriorate the mechanical environment of the IVD.
Like other substances in the body, normal ECM is in a delicate balance between anabolism and catabolism. Disruption of this balance will undoubtedly lead to the impairment of function. NP cells perform a central role in regulating the anabolism and catabolism of the ECM by regulating the MMP and ADAMTS families of enzymes (Weiler et al., 2002; Vo et al., 2013). Both the excessive activation of MMP and ADAMTS are mainly reflected in the catabolism of ECM. A previous study included 41 specimens of patients who had undergone operations for lumbar disc herniation and analyzed the relationship between the degree of degeneration and the expression of MMP-1 (Xu et al., 2014a). As the degree of degeneration increased, the expression of MMP-1 increased notably. Tang et al. (Tang et al., 2014) used microarray analysis to show that MMP-2 was overexpressed in IDD tissue. The increased expression of MMP-3 was also reported in different animal models (Sobajima et al., 2005; Wei et al., 2014). Furthermore, the upregulation of MMP-7, -8, -10, -12, -13 and-14 were also reported in previous studies (Bachmeier et al., 2009; Richardson et al., 2009; Phillips et al., 2013b; Gruber et al., 2014b; Xu et al., 2014b; Iwata et al., 2015). Similar to MMP, the upregulation of several types of ADAMTS has been reported in degenerated discs (Pockert et al., 2009; Zhang et al., 2012). Of note, Patel et al. (Patel et al., 2007) reported that the amount of ADAMTS-5 in the NP and AF shows no significant difference in different degeneration grades. However, Chen et al. (Chen et al., 2014) reported the upregulation of ADAMTS-5 in the CEPs of IDD patients. Interestingly, ADAMTS-3 and ADAMTS-10 were found to be significantly downregulated in degenerated discs (Gruber et al., 2011). Thus, different tissues may tend to express ADAMTS differently. In addition, the upregulation of inflammatory cytokines, such as IL-1 and tumor necrosis factor (TNF)-α, is closely related to the regulation of MMP and ADAMTS. The upregulation of a variety of catabolic mediators by cytokines, including ADAMTS −4/5, MMP −1, −2, −3, −13 and −14 has been reported in many previous studies (Le Maitre et al., 2005; Séguin et al., 2005; Wang et al., 2011; Shi et al., 2019). The emergence of so many biomarkers makes it possible to better explain the pathological process of IDD and block its pathological progress at an early stage.
The inflammatory response of IVD tissue and the corresponding release of proinflammatory cytokines are considered important hallmarks of IDD. A prominent feature is the IL-1β and TNF-α secreted by the disc cells (Kadow et al., 2015). These cytokines trigger a range of pathogenic responses, such as amplifying the inflammatory cascade, apoptosis, cellular senescence, pyroptosis and degradation of ECM. Kepler et al. (Kepler et al., 2013b) showed that exposing human NP and AF cells to IL-1β or TNF-α can upregulate the expression of substance P. The contents of other inflammatory factors such as IL-6 and IL-8 were further significantly increased by substance P. In addition, the expression of some other inflammatory molecules was also upregulated by exposure to IL-1β, such as nitric oxide (NO), cyclooxygenase (COX)-2 and prostaglandin E (PGE)-2 (Wang et al., 2019b; Jin et al., 2019). Similarly, the upregulation of IL-17, NO, and PGE-2 in NP and AF cells exposed to TNF-α have also been reported in previous studies (Gabr et al., 2011; Gruber et al., 2013). IL-1β and TNF-α can also regulate chemokine expression in IVD. In a previous study using IVD samples from LBP patients, the expression of C-C motif ligand (CCL)-5 and IL-1β was significantly enhanced in painful IVDs; furthermore, the correlated expression of CCL-5 with IL-1β expression was also indicated (Kepler et al., 2013a). In subsequent in vitro experiments, disc cells exposed to both IL-1β and TNF-α upregulated CCL-5 expression, and interestingly, TNF-α promoted higher levels of CCL-5 (Gruber et al., 2014a). Phillips et al. (Phillips et al., 2015) reported that the expression of CCL −2, −3, −4, −5, −6, −7 and C-X-C motif chemokine ligand (CXCL) −1, −8, −9 and −10 was increased by the stimulation of IL-1β. A bioinformatics study reported that the gene expression levels of CCL −3, −20, CXCL −2 and −5 were increased in disc cells under TNF-α stimulation. In addition, IL-1β and TNF-α are closely related to mechanical loading (Elfervig et al., 2001; Wang et al., 2007).
IL-1β and TNF-α also play crucial roles in the regulation of the cell cycle. Yang et al. (Yang et al., 2019) reported that IL-1β stimulated the expression of senescence associated-β-galactosidase (SA-β-Gal), which is considered as a dependable marker of cell senescence. Chen et al. (Chen et al., 2020b) subsequently indicated that IL-1β promoted the progression of IDD, with significantly increased expression of type I collagen, p16, p53 and SA-β-Gal, as well as the reduced expression of type II collagen and aggrecan. Similarly, a previous study from Xie et al. (Xie et al., 2018) indicated that TNF-α could also promote NP cell senescence, reflected by the elevation of SA-β-Gal, p16 and p53 expression. Although apoptosis is important for maintaining cell renewal, once out of balance, excessive apoptosis can lead to the depletion of IVD cells and result in IDD. Jiang et al. (Jiang et al., 2019) demonstrated that IL-1β significantly increased caspase-3 activity, NP cell apoptosis ratio and the expression of Bax, caspase-3, cleaved caspase-3 and cleaved PARP. Similarly, Yu et al. (Yu et al., 2018) reported that TNF-α stimulation markedly improved cell apoptosis ratio, caspase-3 activity, expression of Bcl-2, Bax, and caspase-3, reactive oxygen species (ROS) content, and the activity of the NF-κB pathway.
Pyroptosis is a newfound type of cell death mediated by inflammation. The pyroptosis of cells is often accompanied by an increased number of the NLRP3 inflammasome (He et al., 2015; He et al., 2016). Zhao et al. (Zhao et al., 2021b) reported that an increase in pyroptosis was accompanied by an increase in IL-1β and NLRP3 inflammasome activation. In addition, the disc becomes vascularized and innervated; this has been recognized as an important pathological change of IDD (Karamouzian et al., 2010; Miyagi et al., 2014). The overexpression of vascular endothelial growth factor (VGEF) and neurotrophic factors, such as nerve growth factor (NGF) and brain-derived neurotrophic factor (BDNF), result in vascularization and innervation of the IVD (Liu et al., 2013; Moon et al., 2014; Zhu et al., 2018). Lee et al. (Lee et al., 2011) indicated that IL-1β and TNF-α stimulated the gene expression of VGEF, NGF and BDNF in NP cells; furthermore, they also indicated a positive inter-relationship between IL-1β and VEGF/NGF/BDNF expression using the immunohistochemical method. A deeper understanding of the inflammatory mechanism in IDD will undoubtedly help us to block pathological processes in a timelier manner.
A sufficient number of cells is undoubtedly necessary to maintain material renewal and the functional maintenance of IVD. Degenerative discs show higher rates of senescence, apoptosis and pyroptosis, thus leading to fewer functioning cells. The most prominent feature of cellular senescence is the irreversible cessation of cell proliferation, which can be identified by the expression of a senescence-associated secretory phenotype (SASP) (McCarthy et al., 2013; Childs et al., 2015; Su et al., 2019). Although multiple molecular signaling pathways are involved in cellular senescence, these all converge on the p53/p21/retinoblastoma (RB) and p16/RB pathways (Chicas et al., 2010; Muñoz-Espín and Serrano, 2014). Because IVD is avascular, this limits the clearance of metabolic waste and immune mediation to cause abnormal accumulation (de Magalhães and Passos, 2018). Previous studies indicated that oxidative stress (OS) and epigenomic perturbations accelerated the cellular senescence of IDD (Campisi et al., 2019). The use of antioxidant drugs to reduce cellular senescence in IDD has been reported in previous studies (Larrañaga et al., 2018; Liu et al., 2018). In addition, genome-wide analysis of DNA methylation profiles revealed significant differences in DNA methylation profiles of NP cells at different stages of IDD. Ikuno et al. (Ikuno et al., 2019) reported 220 differently methylated loci. Unfortunately, differential methylation was found mainly in genes located upstream, which hindered their further clinical application (Ikuno et al., 2019). As key regulators of gene expression, miRNAs have also attracted attention in recent years. Notably, some of these, such as miRNA-338-3p and miRNA-24-3p, are reported to be upregulated in degenerative NP cells (Chen et al., 2020a; Jiang et al., 2021).
Apoptosis and pyroptosis are common modes of programmed cell death and play critical roles in immune regulation. An imperative characteristic of apoptosis is the release of cytochrome-c from mitochondria (Fraser and Evan, 1996). At present, it is commonly accepted that apoptosis mainly includes intrinsic and extrinsic pathways. The dysregulation of intracellular homeostasis by toxic agents or DNA damage triggers the intrinsic pathway; this is characterized by mitochondrial outer membrane permeabilization (MOMP). Under the joint action of MOMP and cytochrome-c, the activity of caspase-3 is upregulated; this is broadly considered the point of no return in apoptotic death (Liu et al., 1996). The activation of cell surface receptors, such as the TNF-related apoptosis-including ligand receptors R1 and R2 triggers the extrinsic pathway (Schneider et al., 1997). Upon activation by their ligands, the intrinsic pathway is finally activated through a complex signaling network (Bertheloot et al., 2021). In the simplified pathway of pyroptosis, caspase-1 is activated by inflammasomes; then, gasdermin-D (GSDMD) is cleaved to GSDMD C-terminal and GSDMD N-terminal. The GSDMD N-terminal can puncture the cell membrane, thus resulting in pyroptosis (Boucher et al., 2018; Wang et al., 2020b). Recent studies demonstrated that (P. acnes) is a microbial pathogenic factor of IDD (Berjano et al., 2019). He et al. (He et al., 2020) reported that the expression of NLRP3, caspase -1, caspase -5, and IL-1β were upregulated in NP cells co-cultured with P. acnes, thus suggesting the activation of pyroptosis. Tang et al. (Tang et al., 2021a) demonstrated that P. acnes activated the pyroptosis of NP cells via the ROS-NLRP3 pathway. The complex relationship between cell loss and other pathological factors makes our understanding of IDD even more challenging, but also provides more directions for subsequent interventions.
Our increasing understanding of IDD pathology has led to more therapeutic approaches. Current treatment covers almost all scales of disc tissue from macro to micro levels, such as gene therapy, cell replenishment and surgical treatment (Debono et al., 2018; Li et al., 2021; Roh et al., 2021). Limited by many factors such as ethics, biocompatibility and persistence, IDD therapy, which regulates the metabolism and repairment of cells and tissues, appears to be more popular. Many previous studies have focused on IDD-related drugs, regulatory molecules and gene targets (Chao-Yang et al., 2021; Kamali et al., 2021). Because most of these biologics are fragile and easily decomposed, it is very important to select suitable delivery systems. Nevertheless, comparatively few studies have focused on delivery systems. Here, we exemplify the design principles and application scenarios of delivery systems on different scales.
The continuous discovery of IDD-related gene targets makes it possible to intervene in the pathological progress of IDD at the gene level (Cazzanelli and Wuertz-Kozak, 2020). In addition, due to its avascular structure, which makes drug delivery through the blood more difficult, the targeted therapy of IVD cells is a more promising treatment method. As a type of targeted therapy, gene therapy can delay or even reverse IDD by regulating the expression of IDD-related substances in cells (Roh et al., 2021). The method of delivering the proper gene to the target cells is the first issue to be considered. Because of the natural advantages of viruses in delivering genetic information to host cells, viral vectors have attracted extensive attention.
Retroviruses were the first to be considered. Wehling et al. (Wehling et al., 1997) successfully transfected a target gene by retroviruses into chondrocytes from bovine CEP in vitro. Unfortunately, the effect of retroviruses on cells with a low-dividing rate is restricted, thus limiting the use of retroviruses in IDD (Bartels et al., 1998; Desmoulin et al., 2020). Subsequently, researchers turned to adenoviruses because of their higher transduction efficiency, notably in quiescent or slow-dividing cells (Moon et al., 2000). Many previous studies have demonstrated that adenovirus delivery systems play a significant role in regulating inflammation and improving ECM metabolism (Liang et al., 2010; Luo et al., 2016; Zheng et al., 2018). However, the potential infectivity and immunogenicity of adenoviral vectors cannot be ignored. In 1999, the first case of lethal systemic inflammation after the intra-arterial injection of an adenovirus vector was reported. This has forced researchers to stop the rapid development of adenoviral vectors and reassess their safety. In a safety assessment of gene transfer for the treatment of IVD, Wallach et al. (Wallach et al., 2006) indicated that improperly dosed or direct gene delivery to disc tissue may result in adverse complications. Driesse et al. (Driesse et al., 2000) reported that recombinant adenovirus may cause infection of the central nervous system. In fact, there has been a significant decline in the number of articles investigating adenoviral vectors over recent years; these have been replaced by adeno-associated viruses (AAVs). Compared with adenoviruses, AAVs have lower immunogenicity and safety has been confirmed (Levicoff et al., 2008; Mern et al., 2017). In 2015, Mern et al. (Mern and Thomé, 2015) identified NP cell-specific AAV serotypes by transfecting human NP cells with diverse AAV serotypes. This study makes AAVs more promising in the treatment of IDD. Kim et al. (Kim et al., 2022) reported that AAV6 could provide superb transduction efficiency with limited cytotoxicity in vivo (Figure 2). Although some AAV-based therapeutics gained regulatory approval in Europe or the United States, their use in IDD has been limited by their high price and difficulties in guaranteeing homogeneity between different batches (Wang et al., 2019a). Lentiviruses have become popular vectors in recent years, mainly due to their ability to transduce non-dividing cells. Wu et al. (Wu et al., 2014) reported that lentiviral vector-mediated gene expression lasted nearly twice as long as AAV in human NP cells. The regulation of IDD-related factors such as inflammation, ECM and apoptosis by lentiviral vectors has been reported (Farhang et al., 2019; Lin et al., 2021; Lu et al., 2021; Lu and Lin, 2021).
FIGURE 2. Complete mice caudal spines obtained using the PEGASOS method (A). The images of the caudal tissues transferred by AAV6 alone (B) and AAV6+cell-permeable peptide (C) are viewed using 3D lightsheet microscopy, pink represents cells that transduced successfully, and cyan represents cells that transduced failed. Statistical analysis of the number of NP cells successfully transduced under each condition (D). The representative image of IVD tissue without punctured (E). Error bars show SEM. ‘∗’ means p < 0.05, indicating a statistical difference. Reprinted permission by CC BY license (Kim et al., 2022). https://creativecommons.org/licenses/by-nc-nd/4.0/.
Although virus vectors have great potential, the high development cost, the need for rigorous toxicology tests, safety verification, the lack of standardization, and inherent batch-to-batch differences are all difficulties that need to be overcome.
Rather than using a virus as a transit point, physically delivering the target material into a cell seems to be a more straightforward solution. The main options at present are ultrasound exposure and electroporation. In 2006, Nishida et al. (Nishida et al., 2006) established a novel gene therapy technique for IVD cells using ultrasound. The target gene was wrapped in microbubbles, and these microbubbles were injected into IVDs. Immediately after injection, ultrasound was irradiated on the surface of the injected discs, thus resulting in the breaking of the microbubbles and transient holes in the cell surface (Nishida et al., 2006). Electroporation is a method that uses short electrical pulses to disturb cell membranes and momentarily creates holes in the membranes that allow genetic material to pass through. Once the optimal conditions for electroporation are determined, it is possible to transfer many cells in a short period. May et al. (May et al., 2017) indicated that two pulses of 1400 V for 20 ms resulted in favorable and reproducible results for both human and bovine IVD cells. Tang et al. (Tang et al., 2021c) used the electroporation method to deliver forkhead-box F1 mRNA to degenerated NP cells in human disc tissue and significantly reduced the secretion of IL-1β and MMP-13 (Figure 3). In addition, laser-mediated transfection and magnetofection are also used in other diseases (Antkowiak et al., 2013; Sizikov et al., 2021). Despite physical methods that can deliver genetic materials directly into target cells, there are non-negligible drawbacks, such as lower transfection rates, potential cell damage, and difficulty in determining the appropriate stimulation intensity.
FIGURE 3. Representative 4 × (top) and 10 × (bottom) stained images (scale bar: 500 μm) of gel sagittal frozen sections containing human NP cells at day 0, week 2 and 4 weeks in culture (A); green represents cells that survived after electroporation transduction, and red represents cells that died after electroporation. The live rate of cells transfected with FOXF1 and pCMV6 by electroporation in the autopsy group and surgery group were quantified (B). Reprinted permission by CC BY license (Tang et al., 2021c). https://creativecommons.org/licenses/by-sa/4.0/.
Hydrogel is a three-dimensional mesh polymer with a hydrophilic structure that is able to bound large amounts of water. A microenvironment with ideal water content is beneficial to maintain the metabolism of IVD tissue and promote regeneration. Besides water, hydrogels can also provide mechanical support for cell proliferation. Hydrogels are highly manipulable because their mechanical strength, degradability, gelation time/temperature and other characteristics can be modified. Due to these advantages, hydrogels have been widely used to intervene with the pathological development of IDD.
There are various methods to arrange hydrogels into different subclasses. At the most fundamental level, natural hydrogels and synthetic hydrogels are classified according to their source composition. Natural hydrogels such as cellulose, alginate and hyaluronic acid always have favorable biocompatibility and low toxicity. However, most natural hydrogels can barely meet the mechanical stress requirements needed in human IVD tissue, thus severely limiting their application (Growney Kalaf et al., 2017; Alinejad et al., 2019). Compared with natural hydrogels, synthetic hydrogels generally have less variability and greater flexibility in mechanical properties, although their potential toxicity to cells cannot be ignored (Klein et al., 2009). As the name suggests, composite hydrogels are composed of at least two components and seem to overcome defects caused by a traditional single component. According to the cross-linking form, composite hydrogels can be classified into chemical and physical hydrogels. Chemical hydrogels are linked by covalent bonds, making the cross-linking irreversible, while physical hydrogels are connected by molecular entanglement and secondary forces and the transformations are reversible (Hoffman, 2002). Nevertheless, in the clinical transformation of hydrogels, it is difficult to implant gelatiniform hydrogels in vivo due to the deep target location and complex anatomy.
It is worth noting that traditional in situ-forming bulk hydrogels have relatively low efficiency in exchanging substances with tissues. In fact, exchanges with the target microenvironment are limited to the hydrogel/tissue interface. In recent years, stimulus-responsive composite hydrogels have been developed rapidly. Because stimulus-responsive composite hydrogels can solidify rapidly only after receiving the corresponding stimulus, they can be injected into the damaged area in a sol-gel state, thus making the hydrogels fit the tissue well. There are various types of stimuli, such as physical, chemical and biological (Koetting et al., 2015; Choi et al., 2019). Considering the changes in the pH microenvironment caused by inflammation and dysregulation of cellular metabolism, pH-sensitive composite hydrogels seem to have ideal prospects. The ionizable pendant groups in the backbone of the hydrogel are responsible for its pH-sensitive behavior (Gupta et al., 2002). According to pendant group ionization, pH-sensitive composite hydrogels can be classified into anionic hydrogels and cationic hydrogels. The generation of an electrostatic repulsive force leads to the swelling and deswelling of the hydrogel; this is accompanied by the absorption and expulsion of water (Gupta et al., 2002). Nguyen et al. (Nguyen et al., 2019) reported the synthesis of a type of poly (ethylene glycol) (PEG)-based hydrogel with adjustable mechanical strength via ring-opening reactions; cytotoxicity tests showed that human NP cells remained a high survival rate after 8 days. In another previous study, Zhang et al. (Zhang et al., 2022b) adjusted the ratio between components to reach a balance of histocompatibility and mechanical stability of poly (acrylic acid) (PAA) hydrogels. Collectively, the most common monomers that can be used to introduce pH-sensitive properties include methacrylic acid (MMA), acrylic acid (AA) and dimethylaminoethyl methacrylate (DMAEMA) (Koetting et al., 2015). In addition, some natural hydrogels can also exhibit pH-sensitive properties, such as albumin and alginate (Zheng and Du, 2021). Considering the constant temperature inside the body compared to outside, temperature-sensitive composite hydrogels have also attracted the attention of researchers. Temperature-sensitive composite hydrogels can be divided into two fundamental subtypes: positively and negatively responsive hydrogels. Common monomers with temperature sensitivity include cellulose derivatives, chitosan, PEG and poly (N-isopropyl acrylamide) (pNiPAAm) (Klouda and Mikos, 2008). Schmitt et al. (Schmitt et al., 2021) reported the long-term evaluation of an injectable chitosan carboxymethyl cellulose hydrogel in an ovine model. Compared with the untreated group, the intervertebral disc was stabilized, and the progression of degeneration was significantly slower in the treated group after 12 months. Furthermore, due to its low cytotoxicity and excellent biocompatibility, PEG has been widely used in medical applications such as promoting the healing of surgical incisions or drug delivery (Tan et al., 2019; Chen et al., 2022).
A significant advantage of photosensitive composite hydrogels is easy to access to light stimulation and the precise control of stimulation. Thus far, three types of photosensitive monomers have attracted a lot of attention, including gelatin methacrylate (GelMA), photopolymerized polyethylene glycol diacrylate (PEGDA) and hyaluronic acid methacrylate (HAMA) (Burdick and Prestwich, 2011; Clark et al., 2017; Kurian et al., 2022). Xu et al. (Xu et al., 2021) combined collagen hydrolysate and GelMA to make a photosensitive composite hydrogel that could encapsulate NP cells in vitro (Figure 4). Unfortunately, the debilitating effect of IVD tissue on light stimulation prevents the application of photosensitive composite hydrogels in vivo. Considering that there are some upregulated enzymes in IDD, such as MMP, some enzyme-sensitive composite hydrogels focusing on IDD-related enzymes have been developed in recent years, although more in vivo experiments are needed (Skaalure et al., 2015; Amer and Bryant, 2016; Schneider et al., 2020). Due to the special mechanical and metabolic environment of the degenerated disc, pressure-sensitive composite hydrogels and ion-sensitive composite hydrogels are expected to play a role in IDD treatment; however, few relevant studies have been reported.
FIGURE 4. Schematic diagram of synthetic molecules of GelMA hydrogels (A). Scanning electron microscopy was used to observe the microstructure of GelMA hydrogels with different concentrations (B–D). Comparison of the pore size of hydrogels with different concentrations (E). Comparison of the appearance of three different concentrations of hydrogels (F). Comparison of mechanical properties of three different concentrations of hydrogels (G). Degradation of hydrogels with different concentrations over time in vitro (H). The appearance of hydrogel after 2 h in vitro degradation (I). Comparison of water binding capacity of hydrogels with different concentrations (J). Comparison of water content angle of hydrogels with different concentrations (K). (*p < 0.05, **p < 0.01). Reproduced with permission from (Xu et al., 2021).
There has been promising progress in stimulus-responsive composite hydrogels, although the advances in biological material and tissue engineering technologies have led researchers to focus on more microscopic structures. A better understanding of microstructure would allow us to better manipulate the properties of materials.
Just as stimulus-responsive composite hydrogels solve the problem of tissue fitting when compared to in situ gels, injectable microsphere-based delivery systems can provide superior substance exchange efficiency. Microspheres are a class of three-dimensional spherical structures with a mean particle diameter of 1–1,000 μm (Gupta et al., 2017). According to the distribution of therapeutic substances in microspheres, microspheres can be divided into microcapsules and micromatrices. Microcapsules take the therapeutic substance as the core, while micromatrices are formed when the therapeutic substance is dispersed or embedded in the entire material matrix (Kulchar et al., 2021). Due to its good delivery capacity and biocompatibility, the microsphere system has significant application potential as a cell, drug and biological factor delivery system in IVD therapy. Common natural ingredients include collagen, chitosan, gelatin and alginate. Indeed, gelatin- and collagen-based microsphere systems are already commercially available (Chen et al., 2013).
Poly (lactic-co-glycolic acid) (PLGA) copolymer is one of the most widely used biomaterials at present due to its excellent biodegradation and biocompatibility (Zhao et al., 2021a; Zhu et al., 2022a). PLGA is synthesized by lactic acid (LA) and glycolic (GA) through random polymerization (Su et al., 2021). Hodgkinson et al. (Hodgkinson et al., 2019) reported that recombinant human growth difference factor (rhGDF-6) was loaded in PLGA-PEG-PLGA microspheres and induced the differentiation of adipose stem cells (ASCs) to NP cells and aggrecan production (Figure 5). Huang et al. (Huang et al., 2021) used PEG-PLGA-PGE as a drug carrier and showed that this microsphere system had ideal biocompatibility and low cytotoxicity. In a recent study, Lim et al. (Lim et al., 2022) encapsulated ABT263, a senolytic drug, in PLGA microspheres which were intradiscally administered into injury-induced IDD rat models. Analysis showed the selective clearance of senescent cells from degenerative IVD tissue, the downregulated expression of proinflammatory cytokines and the restoration of the IVD structure. However, considering the more complex anatomical structure and mechanical environment of the human spine, the application of PLGA microspheres in human IVD still needs to be demonstrated in further experiments.
FIGURE 5. The binding ability of different concentrations of small (A) and large (B) microspheres to collagen gel under scanning electron microscopy. After 14 days of culture, the microspheres containing rhGDF-6 showed more ideal target substances expression in both histological and immunohistochemical staining (C). Scale bars = 300 μm. Reproduced with permission from (Hodgkinson et al., 2019).
Poly (ε-caprolactone) (PCL) is a polymer with very low glasses transition temperature and melting point. Due to its good biocompatibility, PCL has been widely used in medicine; for example, as surgical sutures (Wei et al., 2009). Jang et al. (Jang et al., 2020) embedded hyaluronic acid (HA) in PCL/HA hybrid microspheres, and the results indicated that the PCL/HA hybrid microspheres provided a proper environment for the proliferation and differentiation of human periosteum-derived cells in vitro. Zhou et al. (Zhou et al., 2020) presented a simple method that combined poly (vinyl alcohol) (PVA) hydrogels with PCL and reflected a new type of microsphere with a PVA core and PCL shell. Unfortunately, due to its slow decomposition in vivo, PCL is mainly used as a scaffold material to repair AF or osteonecrosis, and its use as a microsphere-based delivery system in IDD has been rarely reported (Shamsah et al., 2019; Zhang et al., 2022a).
Since its release properties can be modified by adjusting physical and chemical parameters, researchers can better regulate the release of therapeutic substances by microsphere hydrogels. Chang et al. (Chang et al., 2022b) reported a circRNA silencing-hydrogel microsphere by grafting silencing genes loaded on HAMA microspheres. The results indicated that HAMA microspheres had ideal degradability and that silencing genes can be significantly released for 27 days. Platelet-rich plasma (PRP) is the product of whole blood centrifugation and can relieve IDD symptoms in humans (Tuakli-Wosornu et al., 2016). In another previous study, Miran et al. (Choi et al., 2020) reported that loading powdered PRP into PEG microspheres could significantly prolong the degradation and protein release time of microspheres. In short, the development of microsphere hydrogels brings us one step closer to the single injection of drugs that could provide long-term intervention for IDD processes.
As a common delivery system, the microsphere-based delivery system has a broad clinical application prospect for IDD. However, rigorous safety audits must be carried out and the performance degradation caused by the complex physical and chemical environment in the human body must be considered. In addition, the parameters that are more suitable for IVD tissue also require extensive preliminary experiments.
Besides microspheres, many nanomaterials are also used for drug delivery because they are more easily taken up by cells. Therapeutic substances can be immobilized on or encapsulated by such nanomaterials. Nanomaterials that can be degraded in situ are generally considered to have excellent biocompatibility and low immunogenicity (Mitchell et al., 2021). Common nanomaterials used for nanocarriers include lipids, polymers and inorganic compounds.
Lipid-based delivery systems have many inherent advantages, such as simple composition, good biocompatibility, low toxicity and self-assembly (Sercombe et al., 2015). Liposomes are the most well know lipid-based delivery systems. In addition, some artificial liposomes also show promising therapeutic prospects. Banala et al. (Banala et al., 2019) were able to reduce the expression of caspase-3 and ADAMTS-5 via liposomes loaded with siDNA. In 2020, Wang et al. (Wang et al., 2020a) used liposomes to deliver oxymatrine and found that the degeneration of IDD was slowed down both in vitro and in vivo (Figure 6). It is worth noting that liposomes are susceptible to the interference of complex environments in vivo, thus limiting their clinical application. Extracellular vesicles (EVs) can break through the limitations of mesenchymal stem cells (MSCs) in the treatment of IDD, of which exosomes have received widespread attention over recent years. Kang et al. (Lu et al., 2017) reported that exosomes act as a critical transport agent in information communication between MSCs and NP cells. Luo et al. (Luo et al., 2021) confirmed that CEP delayed the progression of IDD via exosomes. Similarly, Sun et al. (Sun et al., 2021b) reported that the endothelial cell migration and inflammation response could be controlled by AF cell-derived exosomes. EV-based therapies are also developing rapidly. Liao et al. (Liao et al., 2021) achieved a high uptake of EVs loaded with antioxidant proteins in TNF-α-treated NP cells by gene-editing parental MSCs; this resulted in reduced cell death and lower progression of IDD. In a subsequent study, Qian et al. (Qian et al., 2022) reported that the application of PRP-derived exosomes could alleviate IDD-associated inflammation. At present, the optimal dose and mode of administration of exosomes are not clear, and due to their cell origin, they may receive a more stringent ethical review.
FIGURE 6. Image of oxymatrine liposome staining under the transmission electron microscope (A). The particle size distribution of oxymatrine liposome (B). The particle size changes of oxymatrine liposome during storage for 7°days (C) and 3°months (D) at 4°C. The curve of oxymatrine liposome releasing oxymatrine in vitro (E). The NIFR images of the liposome delivery system biodistribution in tails (F) and whole bodies (G) at different timepoints (2, 8, 24, and 36 h). Red arrows indicate puncture sites. Statistical analysis of fluorescence intensity at the degenerative discs (H). (**p < 0.01). Reproduced with permission from (207). https://creativecommons.org/licenses/by-nc/3.0/.
Polymeric nanoparticles can be classified into three types: polymersomes, micelles and dendrimers. Similar to liposomes, polymersomes have membranes made by amphiphilic block copolymers. Polymersomes are generally more stable and less permeable than liposomes, but many factors can affect these properties, such as the diameter of the polymeric vesicle and the inherent properties of the material (Rideau et al., 2018). Polymersomes have been extensively studied in the fields of anti-inflammation and tumors and their application in IDD is worth considering. Polymeric micelles have a hydrophilic core and a hydrophobic coating and have been used in the clinical treatment of ovarian cancer (Lee et al., 2018). Zhang et al. (Chang et al., 2022a) used polymeric micelles loaded with mRNA to improve the synthesis of ECM. Dendrimers are branched polymers, and their size, mass, and shape can be tightly controlled (Mitchell et al., 2021). Dendrimers have been widely used in many clinical applications, such as contrast media and topical gels (Kannan et al., 2014; Palmerston Mendes et al., 2017). However, the further use of polymeric nanoparticles in human bodies requires strict safety reviews.
Inorganic materials such as carbon and silicon can also be used to establish nano-scale delivery systems. Carbon nanotubes are hollow graphitic structures made of a layer of graphene; therefore, they have a high surface area and excellent electrical conductivity (Negri et al., 2020). Carbon nanotubes have an excellent ability to penetrate cell membranes and bind therapeutic substances. Carbon nanotubes have significant potential in the diagnosis and treatment of cancer and neurodegeneration (John et al., 2015; Tang et al., 2021b). In addition, carbon nanotubes modified with hydrogels have better mechanical, physical and biological features (Vashist et al., 2018). All of these findings provide a reference for the application of carbon nanotubes in IDD. However, the clinical application of carbon nanotubes still is still associated with difficulties that need to be overcome, such as poor water solubility, low biodegradation and dispersion. There have been a number of studies focusing on the use of mesoporous silica nanoparticles in cancer-related drug systems (Li et al., 2019). Mesoporous silica nanoparticles exhibit many advantages such as a large surface area, a high pore volume, tunable pore size and excellent stability. Wang et al. (Wang et al., 2020c) reported the synthesis of mesoporous silica nanoparticles with a cell-targeting function. Although mesoporous silica nanoparticles have broad application prospects, it is necessary to adjust parameters such as hollow structure, surface chemistry and pore size based on IVD tissue and corresponding drug properties; this requires a large amount of in vivo and in vitro experiments.
The application of nanotechnology provides a new solution for the treatment of IDD but still needs to be personalized and adjusted according to the local complex microenvironment in the spine. In addition, the potential cytotoxicity requires further attention.
With the rapid development of new technologies such as gene programming and bio-3D printing, the boundaries between biology, materials science, pharmacy and other related disciplines are gradually becoming blurred (Cui et al., 2020; Zhu et al., 2020). Multi-disciplinary and multi-scale technology exchanges make up for the shortcomings of a traditional single technology, which undoubtedly brings new hope for the treatment of IDD. Multi-scale delivery systems do not only include the multiscale aspect; here, we prefer to extend this concept to the temporal scale in which this type of system could show difference performance in different disease stages.
The pathological process of IDD is a multi-scale process from the gene to the local anatomical structure, which makes it difficult to obtain satisfactory results by intervening with a single factor; therefore, the establishment of a multi-scale delivery system has good prospects. Ligorio et al. (Ligorio et al., 2019) reported a new hybrid injectable 3D-scaffold that used graphene oxide (GO) as a nanofiller; results indicated that the 3D-scaffold had similar mechanical properties as health NP tissue and promotes metabolic activity. In addition to its potential to deliver NP cells, researchers explored the possibility of using the 3D-scaffold as a transporter that modulated the sequestration and delivery of transforming growth factor beta-3 (TGF-β3) (Ligorio et al., 2021). In their latest study, these authors extended the use of the 3D-scaffold as a versatile pH-tunable platform for cell encapsulation (Ligorio et al., 2022). Instead of using one system to interfere with pathological processes at different levels, it seems more convenient to combine two systems that work at different levels as one. Due to the instability of exosomes in vivo, their clinical application has stalled. Zhou et al. (Xing et al., 2021) reported the combination of thermosensitive acellular ECM hydrogels and adipose-derived MSC exosomes. This hydrogel system can provide both gelation for ECM leakage and a suitable environment for the growth of NP cells (Figure 7). In addition, sustained released exosomes significantly downregulated the expression of MMP and mitigated the inflammatory response in vitro.
FIGURE 7. Schematic diagram of the mechanisms of one kind of multiscale drug delivery system combining thermosensitive acellular ECM hydrogels and adipose-derived MSC exosomes for IVD treatment. Reproduced with permission from (Xing et al., 2021).
Bio-3D printing is an emerging technology platform that uses computational methods to precisely manipulate the distribution of biomaterials, biological factors and cells. Due to its flexible, precise, and personalized features, bio-3D printing can meet people’s needs more accurately than traditional manufacturing methods (Kang et al., 2016). Because printing different materials requires different parameters, it is difficult to integrate biomaterials, biological factors and cells into one system. Sun et al. (Sun et al., 2021a) designed a new scaffold with biomaterials, cells and biological factors. The replica NP component was printed from MSCs and TGF-β3 hydrogel while the replica AF part was printed by MSCs and connective tissue growth factor hydrogel. In addition, the framework of the whole scaffold was printed by PCL to provide necessary mechanical support (Sun et al., 2021a). Zhu et al. (Zhu et al., 2021) conceived another method to solve this problem by using poly lactide (PLA) to print an IVD frame structure; AF and NP are modeled by oriented porous poly (l-lactide)/octa-armed polyhedral oligomeric silsesquioxanes fiber bundles and gellan gum/PEGDA hydrogel loaded with MSCs, respectively. Both scaffolds exhibited good mechanical properties in vitro, maintained high cell activity and produced new ECM in vivo.
A sequential delivery system that can deliver corresponding drugs at different pathological stages in the temporal dimension is also remarkable. Bayer et al. (Bayer et al., 2017) established a delivery system that achieved the sequential release of platelet-derived growth factor (PDGF) and bone morphogenetic protein-2 (BMP-2) by combing alginate matrices with calcium phosphate scaffolding. In a subsequent study, Leslie et al. (Frapin et al., 2020) developed a delivery system based on pullulan microbeads. This sequential delivery system first released CCL-5 to recruit stem cells to NP, followed by the release of TGF-β1 and growth/differentiation factor (GDF)-5 to upregulate the expression of type II collagen and aggrecan.
New technologies and multidisciplinary communication have accelerated the development of multi-scale delivery systems, but long-term safety assessment and adequate in vivo experiments on large mammals still need to be carried out. In addition, the clinical experience and the need for detailed evaluation also need to be considered.
IDD is an important health problem that has a significant effect on a patient’s quality of life. In today’s aging society, this problem should be given increased levels of attention. Although we are still exploring the detailed pathological process of IDD, we have reviewed several widely accepted mechanisms in the development of IDD; these mechanisms are interconnected and form a vicious loop. Treatment methods for these mechanisms are also developing rapidly, covering almost every time stage and physical scale of IDD development. Although significant levels of attention have been paid to specific targets and effective drugs, an equally important question tends to be overlooked: how do we get these therapeutic substances to where they should be and in the way that people expect them to be? In this review, we summarized the pathological mechanisms associated with IDD and described the delivery systems at various scales. We emphasized the multi-scale delivery systems brought about by multidisciplinary communication as a new direction for delivery systems. However, there are still many difficulties to be overcome in the selection of suitable carriers. Cytotoxicity, degradability, and biocompatibility are unavoidable problems for all implants. In addition, the technical difficulty and cost of materials are also practical problems that need to be considered. The choice is often a trade-off between various performances, and it is difficult to meet all the expectations at the beginning of the design.
When the delivery system is considered for clinical application, it is necessary to discuss the practical aspects; various delivery systems are briefly evaluated in Table 1. The mechanisms responsible for IDD and LBP remain unclear and some IDD patients do not receive special examinations before the onset of symptoms. How we screen early-stage IDD patients and persuade them to accept treatment is the first problem that clinicians need to address. The next problem is the personalization of therapeutic strategies and how to select the appropriate system according to the specific condition of patients; this requires more research. Finally, any material implanted into the body should undergo rigorous safety assessments and pre-clinical validation.
In conclusion, we should design delivery systems with better theoretical performance through multidisciplinary and advanced technology. Furthermore, we should adjust the parameters and components of these systems according to the specific situation of patients. Although a significant amount of research is still needed, we still expect that multi-scale delivery systems will bring new opportunities for IDD treatment.
ZL: Conception of the work; Drafted the manuscript; Revision of the manuscript. CF: Supervised the direction of manuscript; Design of the work; Revision of the manuscript.
The authors would like to express their gratitude to EditSprings (https://www.editsprings.cn) for the expert linguistic services provided.
The authors declare that the research was conducted in the absence of any commercial or financial relationships that could be construed as a potential conflict of interest.
All claims expressed in this article are solely those of the authors and do not necessarily represent those of their affiliated organizations, or those of the publisher, the editors and the reviewers. Any product that may be evaluated in this article, or claim that may be made by its manufacturer, is not guaranteed or endorsed by the publisher.
Adams, M. A., Mcmillan, D. W., Green, T. P., and Dolan, P. (1996). Sustained loading generates stress concentrations in lumbar intervertebral discs. Spine (Phila Pa 1976) 21, 434–438. doi:10.1097/00007632-199602150-00006
Adams, M. A., and Roughley, P. J. (2006). What is intervertebral disc degeneration, and what causes it? Spine (Phila Pa 1976) 31, 2151–2161. doi:10.1097/01.brs.0000231761.73859.2c
Alinejad, Y., Adoungotchodo, A., Grant, M. P., Epure, L. M., Antoniou, J., Mwale, F., et al. (2019). Injectable chitosan hydrogels with enhanced mechanical properties for nucleus pulposus regeneration. Tissue Eng. Part A 25, 303–313. doi:10.1089/ten.tea.2018.0170
Amer, L. D., and Bryant, S. J. (2016). The in vitro and in vivo response to MMP-sensitive poly(ethylene glycol) hydrogels. Ann. Biomed. Eng. 44, 1959–1969. doi:10.1007/s10439-016-1608-4
Andersson, G. B., An, H. S., Oegema, T. R., and Setton, L. A. (2006). Directions for future research. J. Bone Jt. Surg. 88 (2), 110–114. doi:10.2106/jbjs.f.00030
Antkowiak, M., Torres-Mapa, M. L., Stevenson, D. J., Dholakia, K., and Gunn-Moore, F. J. (2013). Femtosecond optical transfection of individual mammalian cells. Nat. Protoc. 8, 1216–1233. doi:10.1038/nprot.2013.071
Antoniou, J., Steffen, T., Nelson, F., Winterbottom, N., Hollander, A. P., Poole, R. A., et al. (1996). The human lumbar intervertebral disc: Evidence for changes in the biosynthesis and denaturation of the extracellular matrix with growth, maturation, ageing, and degeneration. J. Clin. Invest. 98, 996–1003. doi:10.1172/jci118884
Bachmeier, B. E., Nerlich, A., Mittermaier, N., Weiler, C., Lumenta, C., Wuertz, K., et al. (2009). Matrix metalloproteinase expression levels suggest distinct enzyme roles during lumbar disc herniation and degeneration. Eur. Spine J. 18, 1573–1586. doi:10.1007/s00586-009-1031-8
Bailey, J. F., Miller, S. L., Khieu, K., O'Neill, C. W., Healey, R. M., Coughlin, D. G., et al. (2018). From the international space station to the clinic: How prolonged unloading may disrupt lumbar spine stability. Spine J. 18, 7–14. doi:10.1016/j.spinee.2017.08.261
Banala, R. R., Vemuri, S. K., Dar, G. H., Palanisamy, V., Penkulinti, M., Surekha, M. V., et al. (2019). Efficiency of dual siRNA-mediated gene therapy for intervertebral disc degeneration (IVDD). Spine J. 19, 896–904. doi:10.1016/j.spinee.2018.10.016
Bartels, E. M., Fairbank, J. C., Winlove, C. P., and Urban, J. P. (1998). Oxygen and lactate concentrations measured in vivo in the intervertebral discs of patients with scoliosis and back pain. Spine (Phila Pa 1976) 23, 1–7. discussion 8. doi:10.1097/00007632-199801010-00001
Bayer, E. A., Jordan, J., Roy, A., Gottardi, R., Fedorchak, M. V., Kumta, P. N., et al. (2017). <sup/>Programmed platelet-derived growth factor-BB and bone morphogenetic protein-2 delivery from a hybrid calcium phosphate/alginate scaffold. Tissue Eng. Part A 23, 1382–1393. doi:10.1089/ten.tea.2017.0027
Benneker, L. M., Andersson, G., Iatridis, J. C., Sakai, D., HäRTL, R., Ito, K., et al. (2014). Cell therapy for intervertebral disc repair: Advancing cell therapy from bench to clinics. Eur. Cell. Mat. 27, 5–11. doi:10.22203/ecm.v027sa02
Berjano, P., VillafañE, J. H., Lo Re, D., Ismael, M., Damilano, M., Bertozzi, L., et al. (2019). Is Propionibacterium acnes related to disc degeneration in adults? A systematic review. J. Neurosurg. Sci. 63, 216–223. doi:10.23736/s0390-5616.16.03842-x
Bertheloot, D., Latz, E., and Franklin, B. S. (2021). Necroptosis, pyroptosis and apoptosis: An intricate game of cell death. Cell. Mol. Immunol. 18, 1106–1121. doi:10.1038/s41423-020-00630-3
Boos, N., Weissbach, S., Rohrbach, H., Weiler, C., Spratt, K. F., and Nerlich, A. G. (2002). Classification of age-related changes in lumbar intervertebral discs: 2002 volvo award in basic science. Spine (Phila Pa 1976) 27, 2631–2644. doi:10.1097/00007632-200212010-00002
Boucher, D., Monteleone, M., Coll, R. C., Chen, K. W., Ross, C. M., Teo, J. L., et al. (2018). Caspase-1 self-cleavage is an intrinsic mechanism to terminate inflammasome activity. J. Exp. Med. 215, 827–840. doi:10.1084/jem.20172222
Bowles, R. D., and Setton, L. A. (2017). Biomaterials for intervertebral disc regeneration and repair. Biomaterials 129, 54–67. doi:10.1016/j.biomaterials.2017.03.013
Burdick, J. A., and Prestwich, G. D. (2011). Hyaluronic acid hydrogels for biomedical applications. Adv. Mat. 23, H41–H56. doi:10.1002/adma.201003963
Campisi, J., Kapahi, P., Lithgow, G. J., Melov, S., Newman, J. C., and Verdin, E. (2019). From discoveries in ageing research to therapeutics for healthy ageing. Nature 571, 183–192. doi:10.1038/s41586-019-1365-2
Cazzanelli, P., and Wuertz-Kozak, K. (2020). MicroRNAs in intervertebral disc degeneration, apoptosis, inflammation, and mechanobiology. Int. J. Mol. Sci. 21, 3601. doi:10.3390/ijms21103601
Chan, S. C., Ferguson, S. J., and Gantenbein-Ritter, B. (2011). The effects of dynamic loading on the intervertebral disc. Eur. Spine J. 20, 1796–1812. doi:10.1007/s00586-011-1827-1
Chang, C. C., Tsou, H. K., Chang, H. H., Chan, L. Y., Zhuo, G. Y., Maeda, T., et al. (2022a). Runx1 messenger RNA delivered by polyplex nanomicelles alleviate spinal disc hydration loss in a rat disc degeneration model. Int. J. Mol. Sci. 23, 565. doi:10.3390/ijms23010565
Chang, H., Cai, F., Zhang, Y., Jiang, M., Yang, X., Qi, J., et al. (2022b). Silencing gene-engineered injectable hydrogel microsphere for regulation of extracellular matrix metabolism balance. Small Methods 6, e2101201. doi:10.1002/smtd.202101201
Chao-Yang, G., Peng, C., and Hai-Hong, Z. (2021). Roles of NLRP3 inflammasome in intervertebral disc degeneration. Osteoarthr. Cartil. 29, 793–801. doi:10.1016/j.joca.2021.02.204
Chen, A. K., Reuveny, S., and Oh, S. K. (2013). Application of human mesenchymal and pluripotent stem cell microcarrier cultures in cellular therapy: Achievements and future direction. Biotechnol. Adv. 31, 1032–1046. doi:10.1016/j.biotechadv.2013.03.006
Chen, S., Huang, Y., Zhou, Z. J., Hu, Z. J., Wang, J. Y., Xu, W. B., et al. (2014). Upregulation of tumor necrosis factor α and ADAMTS-5, but not ADAMTS-4, in human intervertebral cartilage endplate with modic changes. Spine (Phila Pa 1976) 39, E817–E825. doi:10.1097/brs.0000000000000362
Chen, T., Wang, Y., Xie, J., Qu, X., and Liu, C. (2022). Lysozyme amyloid fibril-integrated PEG injectable hydrogel adhesive with improved antiswelling and antibacterial capabilities. Biomacromolecules 23, 1376–1391. doi:10.1021/acs.biomac.1c01597
Chen, Z. B., Yu, Y. B., Wa, Q. B., Zhou, J. W., He, M., and Cen, Y. (2020b). The role of quinazoline in ameliorating intervertebral disc degeneration by inhibiting oxidative stress and anti-inflammation via NF-κB/MAPKs signaling pathway. Eur. Rev. Med. Pharmacol. Sci. 24, 2077–2086. doi:10.26355/eurrev_202002_20387
Chen, Z., Liu, M., Zhang, W., Deng, M., Zhou, Y., and Li, Y. (2020a). miR-24-3p induces human intervertebral disc degeneration by targeting insulin-like growth factor binding protein 5 and the ERK signaling pathway. Life Sci. 243, 117288. doi:10.1016/j.lfs.2020.117288
Cheung, K. M., Karppinen, J., Chan, D., Ho, D. W., Song, Y. Q., Sham, P., et al. (2009). Prevalence and pattern of lumbar magnetic resonance imaging changes in a population study of one thousand forty-three individuals. Spine (Phila Pa 1976) 34, 934–940. doi:10.1097/brs.0b013e3181a01b3f
Chicas, A., Wang, X., Zhang, C., Mccurrach, M., Zhao, Z., Mert, O., et al. (2010). Dissecting the unique role of the retinoblastoma tumor suppressor during cellular senescence. Cancer Cell 17, 376–387. doi:10.1016/j.ccr.2010.01.023
Childs, B. G., Durik, M., Baker, D. J., and Van Deursen, J. M. (2015). Cellular senescence in aging and age-related disease: From mechanisms to therapy. Nat. Med. 21, 1424–1435. doi:10.1038/nm.4000
Choi, J. R., Yong, K. W., Choi, J. Y., and Cowie, A. C. (2019). Recent advances in photo-crosslinkable hydrogels for biomedical applications. Biotechniques 66, 40–53. doi:10.2144/btn-2018-0083
Choi, M. H., Blanco, A., Stealey, S., Duan, X., Case, N., Sell, S. A., et al. (2020). Micro-clotting of platelet-rich plasma upon loading in hydrogel microspheres leads to prolonged protein release and slower microsphere degradation. Basel: Polymers, 12.
Clark, E. A., Alexander, M. R., Irvine, D. J., Roberts, C. J., Wallace, M. J., Sharpe, S., et al. (2017). 3D printing of tablets using inkjet with UV photoinitiation. Int. J. Pharm. X. 529, 523–530. doi:10.1016/j.ijpharm.2017.06.085
Court, C., Colliou, O. K., Chin, J. R., Liebenberg, E., Bradford, D. S., and Lotz, J. C. (2001). The effect of static in vivo bending on the murine intervertebral disc. Spine J. 1, 239–245. doi:10.1016/s1529-9430(01)00056-0
Cui, L., Zhang, J., Zou, J., Yang, X., Guo, H., Tian, H., et al. (2020). Electroactive composite scaffold with locally expressed osteoinductive factor for synergistic bone repair upon electrical stimulation. Biomaterials 230, 119617. doi:10.1016/j.biomaterials.2019.119617
De MagalhãES, J. P., and Passos, J. F. (2018). Stress, cell senescence and organismal ageing. Mech. Ageing Dev. 170, 2–9. doi:10.1016/j.mad.2017.07.001
Debono, B., Lonjon, G., Galovich, L. A., Kerever, S., Guiot, B., Eicker, S. O., et al. (2018). Indication variability in degenerative lumbar spine surgery: A four-nation survey. Spine (Phila Pa 1976) 43, 185–192. doi:10.1097/brs.0000000000002272
Desmoulin, G. T., Pradhan, V., and Milner, T. E. (2020). Mechanical aspects of intervertebral disc injury and implications on biomechanics. Spine (Phila Pa 1976) 45, E457–e464. doi:10.1097/brs.0000000000003291
Dieleman, J. L., Baral, R., Birger, M., Bui, A. L., Bulchis, A., Chapin, A., et al. (2016). US spending on personal health care and public health. Jama 316, 2627–2646. doi:10.1001/jama.2016.16885
Driesse, M. J., Esandi, M. C., Kros, J. M., Avezaat, C. J., Vecht, C., Zurcher, C., et al. (2000). Intra-CSF administered recombinant adenovirus causes an immune response-mediated toxicity. Gene Ther. 7, 1401–1409. doi:10.1038/sj.gt.3301250
Dudli, S., Ferguson, S. J., and Haschtmann, D. (2014). Severity and pattern of post-traumatic intervertebral disc degeneration depend on the type of injury. Spine J. 14, 1256–1264. doi:10.1016/j.spinee.2013.07.488
Elfervig, M. K., Minchew, J. T., Francke, E., Tsuzaki, M., and Banes, A. J. (2001). IL-1beta sensitizes intervertebral disc annulus cells to fluid-induced shear stress. J. Cell. Biochem. 82, 290–298. doi:10.1002/jcb.1153
Farhang, N., Ginley-Hidinger, M., Berrett, K. C., Gertz, J., Lawrence, B., and Bowles, R. D. (2019). Lentiviral CRISPR epigenome editing of inflammatory receptors as a gene therapy strategy for disc degeneration. Hum. Gene Ther. 30, 1161–1175. doi:10.1089/hum.2019.005
Feng, H., Danfelter, M., StröMQVIST, B., and HeinegåRD, D. (2006). Extracellular matrix in disc degeneration. J. Bone Jt. Surg. 88 (2), 25–29. doi:10.2106/jbjs.e.01341
Foster, N. E., Anema, J. R., Cherkin, D., Chou, R., Cohen, S. P., Gross, D. P., et al. (2018). Prevention and treatment of low back pain: Evidence, challenges, and promising directions. Lancet 391, 2368–2383. doi:10.1016/s0140-6736(18)30489-6
Francisco, V., Pino, J., GonzáLEZ-Gay, M., Lago, F., Karppinen, J., Tervonen, O., et al. (2022). A new immunometabolic perspective of intervertebral disc degeneration. Nat. Rev. Rheumatol. 18, 47–60. doi:10.1038/s41584-021-00713-z
Frapin, L., Clouet, J., ChéDEVILLE, C., Moraru, C., Samarut, E., Henry, N., et al. (2020). Controlled release of biological factors for endogenous progenitor cell migration and intervertebral disc extracellular matrix remodelling. Biomaterials 253, 120107. doi:10.1016/j.biomaterials.2020.120107
Fraser, A., and Evan, G. (1996). A license to kill. Cell 85, 781–784. doi:10.1016/s0092-8674(00)81005-3
Gabr, M. A., Jing, L., Helbling, A. R., Sinclair, S. M., Allen, K. D., Shamji, M. F., et al. (2011). Interleukin-17 synergizes with IFNγ or TNFα to promote inflammatory mediator release and intercellular adhesion molecule-1 (ICAM-1) expression in human intervertebral disc cells. J. Orthop. Res. 29, 1–7. doi:10.1002/jor.21206
Gao, F., Liu, S., Zhang, X., Wang, X., and Zhang, J. (2021). Automated grading of lumbar disc degeneration using a push-pull regularization network based on MRI. J. Magn. Reson. Imaging 53, 799–806. doi:10.1002/jmri.27400
Gawri, R., Rosenzweig, D. H., Krock, E., Ouellet, J. A., Stone, L. S., Quinn, T. M., et al. (2014). High mechanical strain of primary intervertebral disc cells promotes secretion of inflammatory factors associated with disc degeneration and pain. Arthritis Res. Ther. 16, R21. doi:10.1186/ar4449
GBD 2017 Disease and Injury Incidence and Prevalence Collaborators (2017). Global, regional, and national incidence, prevalence, and years lived with disability for 354 diseases and injuries for 195 countries and territories, 1990-2017: A systematic analysis for the global burden of disease study 2017. Lancet 392, 1789–1858. doi:10.1016/S0140-6736(18)32279-7
Growney Kalaf, E. A., Pendyala, M., Bledsoe, J. G., and Sell, S. A. (2017). Characterization and restoration of degenerated IVD function with an injectable, in situ gelling alginate hydrogel: An in vitro and ex vivo study. J. Mech. Behav. Biomed. Mat. 72, 229–240. doi:10.1016/j.jmbbm.2017.05.014
Gruber, H. E., Hoelscher, G. L., Ingram, J. A., Bethea, S., Norton, H. J., and Hanley, E. N. (2014a). Production and expression of RANTES (CCL5) by human disc cells and modulation by IL-1-β and TNF-α in 3D culture. Exp. Mol. Pathol. 96, 133–138. doi:10.1016/j.yexmp.2014.01.002
Gruber, H. E., Hoelscher, G. L., Ingram, J. A., Bethea, S., Zinchenko, N., and Hanley, E. N. (2011). Variations in aggrecan localization and gene expression patterns characterize increasing stages of human intervertebral disk degeneration. Exp. Mol. Pathol. 91, 534–539. doi:10.1016/j.yexmp.2011.06.001
Gruber, H. E., Hoelscher, G. L., Ingram, J. A., Norton, H. J., and Hanley, E. N. (2013). Increased IL-17 expression in degenerated human discs and increased production in cultured annulus cells exposed to IL-1ß and TNF-α. Biotech. Histochem. 88, 302–310. doi:10.3109/10520295.2013.783235
Gruber, H. E., Ingram, J. A., Cox, M. D., and Hanley, E. N. (2014b). Matrix metalloproteinase-12 immunolocalization in the degenerating human intervertebral disc and sand rat spine: Biologic implications. Exp. Mol. Pathol. 97, 1–5. doi:10.1016/j.yexmp.2014.04.007
Gupta, P., Vermani, K., and Garg, S. (2002). Hydrogels: From controlled release to pH-responsive drug delivery. Drug Discov. Today 7, 569–579. doi:10.1016/s1359-6446(02)02255-9
Gupta, V., Khan, Y., Berkland, C. J., Laurencin, C. T., and Detamore, M. S. (2017). Microsphere-based scaffolds in regenerative engineering. Annu. Rev. Biomed. Eng. 19, 135–161. doi:10.1146/annurev-bioeng-071516-044712
Hartvigsen, J., Hancock, M. J., Kongsted, A., Louw, Q., Ferreira, M. L., Genevay, S., et al. (2018). What low back pain is and why we need to pay attention. Lancet 391, 2356–2367. doi:10.1016/s0140-6736(18)30480-x
He, D., Zhou, M., Bai, Z., Wen, Y., Shen, J., and Hu, Z. (2020). Propionibacterium acnes induces intervertebral disc degeneration by promoting nucleus pulposus cell pyroptosis via NLRP3-dependent pathway. Biochem. Biophys. Res. Commun. 526, 772–779. doi:10.1016/j.bbrc.2020.03.161
He, W. T., Wan, H., Hu, L., Chen, P., Wang, X., Huang, Z., et al. (2015). Gasdermin D is an executor of pyroptosis and required for interleukin-1β secretion. Cell Res. 25, 1285–1298. doi:10.1038/cr.2015.139
He, Y., Hara, H., and NúñEZ, G. (2016). Mechanism and regulation of NLRP3 inflammasome activation. Trends biochem. Sci. 41, 1012–1021. doi:10.1016/j.tibs.2016.09.002
Hodgkinson, T., Stening, J. Z., White, L. J., Shakesheff, K. M., Hoyland, J. A., and Richardson, S. M. (2019). Microparticles for controlled growth differentiation factor 6 delivery to direct adipose stem cell-based nucleus pulposus regeneration. J. Tissue Eng. Regen. Med. 13, 1406–1417. doi:10.1002/term.2882
Hoffman, A. S. (2002). Hydrogels for biomedical applications. Adv. Drug Deliv. Rev. 54, 3–12. doi:10.1016/s0169-409x(01)00239-3
Holm, S., Maroudas, A., Urban, J. P., Selstam, G., and Nachemson, A. (1981). Nutrition of the intervertebral disc: Solute transport and metabolism. Connect. Tissue Res. 8, 101–119. doi:10.3109/03008208109152130
Huang, C. Y., and Gu, W. Y. (2008). Effects of mechanical compression on metabolism and distribution of oxygen and lactate in intervertebral disc. J. Biomech. 41, 1184–1196. doi:10.1016/j.jbiomech.2008.02.002
Huang, X., He, W., Wang, W., Fan, Q., Ye, X., Wu, Z., et al. (2021). Toxicology and pharmacokinetics study of intradiscal injection of simvastatin in rabbits. Front. Pharmacol. 12, 582309. doi:10.3389/fphar.2021.582309
Huang, Y. C., Urban, J. P., and Luk, K. D. (2014). Intervertebral disc regeneration: Do nutrients lead the way? Nat. Rev. Rheumatol. 10, 561–566. doi:10.1038/nrrheum.2014.91
Hutton, W. C., Yoon, S. T., Elmer, W. A., Li, J., Murakami, H., Minamide, A., et al. (2002). Effect of tail suspension (or simulated weightlessness) on the lumbar intervertebral disc: Study of proteoglycans and collagen. Spine (Phila Pa 1976) 27, 1286–1290. doi:10.1097/00007632-200206150-00008
Iatridis, J. C., Nicoll, S. B., Michalek, A. J., Walter, B. A., and Gupta, M. S. (2013). Role of biomechanics in intervertebral disc degeneration and regenerative therapies: What needs repairing in the disc and what are promising biomaterials for its repair? Spine J. 13, 243–262. doi:10.1016/j.spinee.2012.12.002
Ikuno, A., Akeda, K., Takebayashi, S. I., Shimaoka, M., Okumura, K., and Sudo, A. (2019). Genome-wide analysis of DNA methylation profile identifies differentially methylated loci associated with human intervertebral disc degeneration. PLoS One 14, e0222188. doi:10.1371/journal.pone.0222188
Illien-JüNGER, S., Gantenbein-Ritter, B., Grad, S., Lezuo, P., Ferguson, S. J., Alini, M., et al. (2010). The combined effects of limited nutrition and high-frequency loading on intervertebral discs with endplates. Spine (Phila Pa 1976) 35, 1744–1752. doi:10.1097/brs.0b013e3181c48019
Iwata, M., Aikawa, T., Hakozaki, T., Arai, K., Ochi, H., Haro, H., et al. (2015). Enhancement of Runx2 expression is potentially linked to β-catenin accumulation in canine intervertebral disc degeneration. J. Cell. Physiol. 230, 180–190. doi:10.1002/jcp.24697
Jackson, A. R., Yuan, T. Y., Huang, C. Y., Brown, M. D., and Gu, W. Y. (2012). Nutrient transport in human annulus fibrosus is affected by compressive strain and anisotropy. Ann. Biomed. Eng. 40, 2551–2558. doi:10.1007/s10439-012-0606-4
Jang, H. Y., Shin, J. Y., Oh, S. H., Byun, J. H., and Lee, J. H. (2020). PCL/HA hybrid microspheres for effective osteogenic differentiation and bone regeneration. ACS Biomater. Sci. Eng. 6, 5172–5180. doi:10.1021/acsbiomaterials.0c00550
Jiang, H., Moro, A., Wang, J., Meng, D., Zhan, X., and Wei, Q. (2021). MicroRNA-338-3p as a novel therapeutic target for intervertebral disc degeneration. Exp. Mol. Med. 53, 1356–1365. doi:10.1038/s12276-021-00662-3
Jiang, Y., Xie, Z., Yu, J., and Fu, L. (2019). Resveratrol inhibits IL-1β-mediated nucleus pulposus cell apoptosis through regulating the PI3K/Akt pathway. Biosci. Rep. 39, BSR20190043. doi:10.1042/bsr20190043
Jin, H., Wang, Q., Wu, J., Han, X., Qian, T., Zhang, Z., et al. (2019). Baicalein inhibits the IL-1β-induced inflammatory response in nucleus pulposus cells and attenuates disc degeneration in vivo. Inflammation 42, 1032–1044. doi:10.1007/s10753-019-00965-8
Jin, Z., Wang, D., Zhang, H., Liang, J., Feng, X., Zhao, J., et al. (2020). Incidence trend of five common musculoskeletal disorders from 1990 to 2017 at the global, regional and national level: Results from the global burden of disease study 2017. Ann. Rheum. Dis. 79, 1014–1022. doi:10.1136/annrheumdis-2020-217050
John, A. A., Subramanian, A. P., Vellayappan, M. V., Balaji, A., Mohandas, H., and Jaganathan, S. K. (2015). Carbon nanotubes and graphene as emerging candidates in neuroregeneration and neurodrug delivery. Int. J. Nanomedicine 10, 4267–4277. doi:10.2147/ijn.s83777
Kadow, T., Sowa, G., Vo, N., and Kang, J. D. (2015). Molecular basis of intervertebral disc degeneration and herniations: What are the important translational questions? Clin. Orthop. Relat. Res. 473, 1903–1912. doi:10.1007/s11999-014-3774-8
Kamali, A., Ziadlou, R., Lang, G., Pfannkuche, J., Cui, S., Li, Z., et al. (2021). Small molecule-based treatment approaches for intervertebral disc degeneration: Current options and future directions. Theranostics 11, 27–47. doi:10.7150/thno.48987
Kang, H. W., Lee, S. J., Ko, I. K., Kengla, C., Yoo, J. J., and Atala, A. (2016). A 3D bioprinting system to produce human-scale tissue constructs with structural integrity. Nat. Biotechnol. 34, 312–319. doi:10.1038/nbt.3413
Kannan, R. M., Nance, E., Kannan, S., and Tomalia, D. A. (2014). Emerging concepts in dendrimer-based nanomedicine: From design principles to clinical applications. J. Intern. Med. 276, 579–617. doi:10.1111/joim.12280
Karamouzian, S., Eskandary, H., Faramarzee, M., Saba, M., Safizade, H., Ghadipasha, M., et al. (2010). Frequency of lumbar intervertebral disc calcification and angiogenesis, and their correlation with clinical, surgical, and magnetic resonance imaging findings. Spine (Phila Pa 1976) 35, 881–886. doi:10.1097/brs.0b013e3181b9c986
Katz, J. N. (2006). Lumbar disc disorders and low-back pain: Socioeconomic factors and consequences. J. Bone Jt. Surg. 88 (2), 21–24. doi:10.2106/jbjs.e.01273
Kepler, C. K., Markova, D. Z., Dibra, F., Yadla, S., Vaccaro, A. R., Risbud, M. V., et al. (2013a). Expression and relationship of proinflammatory chemokine RANTES/CCL5 and cytokine IL-1β in painful human intervertebral discs. Spine (Phila Pa 1976) 38, 873–880. doi:10.1097/brs.0b013e318285ae08
Kepler, C. K., Markova, D. Z., Hilibrand, A. S., Vaccaro, A. R., Risbud, M. V., Albert, T. J., et al. (2013b). Substance P stimulates production of inflammatory cytokines in human disc cells. Spine (Phila Pa 1976) 38, E1291–E1299. doi:10.1097/brs.0b013e3182a42bc2
Kim, C. H., Oliver, C., Dar, H., Drissi, H., and Presciutti, S. M. (2022). AAV6 as an effective gene delivery vector for prolonged transgene expression in intervertebral disc cells in vivo. Genes Dis. 9, 1074–1085. doi:10.1016/j.gendis.2020.12.009
Klein, T. J., Malda, J., Sah, R. L., and Hutmacher, D. W. (2009). Tissue engineering of articular cartilage with biomimetic zones. Tissue Eng. Part B Rev. 15, 143–157. doi:10.1089/ten.teb.2008.0563
Klouda, L., and Mikos, A. G. (2008). Thermoresponsive hydrogels in biomedical applications. Eur. J. Pharm. Biopharm. 68, 34–45. doi:10.1016/j.ejpb.2007.02.025
Koetting, M. C., Peters, J. T., Steichen, S. D., and Peppas, N. A. (2015). Stimulus-responsive hydrogels: Theory, modern advances, and applications. Mater. Sci. Eng. R Rep. 93, 1–49. doi:10.1016/j.mser.2015.04.001
Kokubo, Y., Uchida, K., Kobayashi, S., Yayama, T., Sato, R., Nakajima, H., et al. (2008). Herniated and spondylotic intervertebral discs of the human cervical spine: Histological and immunohistological findings in 500 en bloc surgical samples. Laboratory investigation. J. Neurosurg. Spine 9, 285–295. doi:10.3171/spi/2008/9/9/285
Kulchar, R. J., Denzer, B. R., Chavre, B. M., Takegami, M., and Patterson, J. (2021). A review of the use of microparticles for cartilage tissue engineering. Int. J. Mol. Sci. 22, 10292. doi:10.3390/ijms221910292
Kurian, A. G., Singh, R. K., Patel, K. D., Lee, J. H., and Kim, H. W. (2022). Multifunctional GelMA platforms with nanomaterials for advanced tissue therapeutics. Bioact. Mat. 8, 267–295. doi:10.1016/j.bioactmat.2021.06.027
LarrañAGA, A., Isa, I. L. M., Patil, V., Thamboo, S., Lomora, M., FernáNDEZ-Yague, M. A., et al. (2018). Antioxidant functionalized polymer capsules to prevent oxidative stress. Acta Biomater. 67, 21–31. doi:10.1016/j.actbio.2017.12.014
Latridis, J. C., Godburn, K., Wuertz, K., Alini, M., and Roughley, P. J. (2011). Region-dependent aggrecan degradation patterns in the rat intervertebral disc are affected by mechanical loading in vivo. Spine (Phila Pa 1976) 36, 203–209. doi:10.1097/brs.0b013e3181cec247
Lazzari, Z. T., Aria, K. M., and Menger, R. (2021). Neurosurgery and spinal adaptations in spaceflight: A literature review. Clin. Neurol. Neurosurg. 207, 106755. doi:10.1016/j.clineuro.2021.106755
Le Maitre, C. L., Freemont, A. J., and Hoyland, J. A. (2005). The role of interleukin-1 in the pathogenesis of human intervertebral disc degeneration. Arthritis Res. Ther. 7, R732–R745. doi:10.1186/ar1732
Lee, H. Y., Han, L., Roughley, P. J., Grodzinsky, A. J., and Ortiz, C. (2013). Age-related nanostructural and nanomechanical changes of individual human cartilage aggrecan monomers and their glycosaminoglycan side chains. J. Struct. Biol. X. 181, 264–273. doi:10.1016/j.jsb.2012.12.008
Lee, J. M., Song, J. Y., Baek, M., Jung, H. Y., Kang, H., Han, I. B., et al. (2011). Interleukin-1β induces angiogenesis and innervation in human intervertebral disc degeneration. J. Orthop. Res. 29, 265–269. doi:10.1002/jor.21210
Lee, S. W., Kim, Y. M., Cho, C. H., Kim, Y. T., Kim, S. M., Hur, S. Y., et al. (2018). An open-label, randomized, parallel, phase II trial to evaluate the efficacy and safety of a cremophor-free polymeric micelle formulation of paclitaxel as first-line treatment for ovarian cancer: A Korean gynecologic oncology group study (KGOG-3021). Cancer Res. Treat. 50, 195–203. doi:10.4143/crt.2016.376
Levicoff, E. A., Kim, J. S., Sobajima, S., Wallach, C. J., Larson, J. W., 3R. D., Robbins, P. D., et al. (2008). Safety assessment of intradiscal gene therapy II: Effect of dosing and vector choice. Spine (Phila Pa 1976) 33, 1509–1516. doi:10.1097/brs.0b013e318178866c
Li, B., Yang, Y., Wang, L., and Liu, G. (2021). Stem cell therapy and exercise for treatment of intervertebral disc degeneration. Stem Cells Int. 2021, 1–10. doi:10.1155/2021/7982333
Li, T., Shi, S., Goel, S., Shen, X., Xie, X., Chen, Z., et al. (2019). Recent advancements in mesoporous silica nanoparticles towards therapeutic applications for cancer. Acta Biomater. 89, 1–13. doi:10.1016/j.actbio.2019.02.031
Liang, H., Ma, S. Y., Feng, G., Shen, F. H., and Joshua Li, X. (2010). Therapeutic effects of adenovirus-mediated growth and differentiation factor-5 in a mice disc degeneration model induced by annulus needle puncture. Spine J. 10, 32–41. doi:10.1016/j.spinee.2009.10.006
Liao, Z., Liu, H., Ma, L., Lei, J., Tong, B., Li, G., et al. (2021). Engineering extracellular vesicles restore the impaired cellular uptake and attenuate intervertebral disc degeneration. ACS Nano 15, 14709–14724. doi:10.1021/acsnano.1c04514
Ligorio, C., O'Brien, M., Hodson, N. W., Mironov, A., Iliut, M., Miller, A. F., et al. (2021). TGF-β3-loaded graphene oxide - self-assembling peptide hybrid hydrogels as functional 3D scaffolds for the regeneration of the nucleus pulposus. Acta Biomater. 127, 116–130. doi:10.1016/j.actbio.2021.03.077
Ligorio, C., Vijayaraghavan, A., Hoyland, J. A., and Saiani, A. (2022). Acidic and basic self-assembling peptide and peptide-graphene oxide hydrogels: Characterisation and effect on encapsulated nucleus pulposus cells. Acta Biomater. 143, 145–158. doi:10.1016/j.actbio.2022.02.022
Ligorio, C., Zhou, M., Wychowaniec, J. K., Zhu, X., Bartlam, C., Miller, A. F., et al. (2019). Graphene oxide containing self-assembling peptide hybrid hydrogels as a potential 3D injectable cell delivery platform for intervertebral disc repair applications. Acta Biomater. 92, 92–103. doi:10.1016/j.actbio.2019.05.004
Lim, S., An, S. B., Jung, M., Joshi, H. P., Kumar, H., Kim, C., et al. (2022). Local delivery of senolytic drug inhibits intervertebral disc degeneration and restores intervertebral disc structure. Adv. Healthc. Mat. 11, e2101483. doi:10.1002/adhm.202101483
Lin, J., Zheng, X., Zhang, Z., Zhuge, J., Shao, Z., Huang, C., et al. (2021). Inhibition of LRRK2 restores parkin-mediated mitophagy and attenuates intervertebral disc degeneration. Osteoarthr. Cartil. 29, 579–591. doi:10.1016/j.joca.2021.01.002
Liu, H., Kang, H., Song, C., Lei, Z., Li, L., Guo, J., et al. (2018). Urolithin A inhibits the catabolic effect of TNFα on nucleus pulposus cell and alleviates intervertebral disc degeneration in vivo. Front. Pharmacol. 9, 1043. doi:10.3389/fphar.2018.01043
Liu, X., Kim, C. N., Yang, J., Jemmerson, R., and Wang, X. (1996). Induction of apoptotic program in cell-free extracts: Requirement for dATP and cytochrome c. Cell 86, 147–157. doi:10.1016/s0092-8674(00)80085-9
Liu, X. W., Kang, J., Fan, X. D., and Sun, L. F. (2013). Expression and significance of VEGF and p53 in rat degenerated intervertebral disc tissues. Asian pac. J. Trop. Med. 6, 404–406. doi:10.1016/s1995-7645(13)60047-4
Lu, K., Li, H. Y., Yang, K., Wu, J. L., Cai, X. W., Zhou, Y., et al. (2017). Exosomes as potential alternatives to stem cell therapy for intervertebral disc degeneration: In-vitro study on exosomes in interaction of nucleus pulposus cells and bone marrow mesenchymal stem cells. Stem Cell Res. Ther. 8, 108. doi:10.1186/s13287-017-0563-9
Lu, S., and Lin, C. W. (2021). Lentivirus-mediated transfer of gene encoding fibroblast growth factor-18 inhibits intervertebral disc degeneration. Exp. Ther. Med. 22, 856. doi:10.3892/etm.2021.10288
Lu, S., Song, Y., Luo, R., Li, S., Li, G., Wang, K., et al. (2021). Ferroportin-dependent iron homeostasis protects against oxidative stress-induced nucleus pulposus cell ferroptosis and ameliorates intervertebral disc degeneration in vivo. Oxid. Med. Cell. Longev. 2021, 1–18. doi:10.1155/2021/6670497
Luo, L., Jian, X., Sun, H., Qin, J., Wang, Y., Zhang, J., et al. (2021). Cartilage endplate stem cells inhibit intervertebral disc degeneration by releasing exosomes to nucleus pulposus cells to activate Akt/autophagy. Stem Cells 39, 467–481. doi:10.1002/stem.3322
Luo, X. W., Liu, K., Chen, Z., Zhao, M., Han, X. W., Bai, Y. G., et al. (2016). Adenovirus-mediated GDF-5 promotes the extracellular matrix expression in degenerative nucleus pulposus cells. J. Zhejiang Univ. Sci. B 17, 30–42. doi:10.1631/jzus.b1500182
Malandrino, A., Lacroix, D., Hellmich, C., Ito, K., Ferguson, S. J., and Noailly, J. (2014). The role of endplate poromechanical properties on the nutrient availability in the intervertebral disc. Osteoarthr. Cartil. 22, 1053–1060. doi:10.1016/j.joca.2014.05.005
MartíNEZ-LavíN, M. (2021). Dorsal root ganglia: Fibromyalgia pain factory? Clin. Rheumatol. 40, 783–787. doi:10.1007/s10067-020-05528-z
May, R. D., Tekari, A., Frauchiger, D. A., Krismer, A., Benneker, L. M., and Gantenbein, B. (2017). Efficient nonviral transfection of primary intervertebral disc cells by electroporation for tissue engineering application. Tissue Eng. Part C. Methods 23, 30–37. doi:10.1089/ten.tec.2016.0355
Mccarthy, D. A., Clark, R. R., Bartling, T. R., Trebak, M., and Melendez, J. A. (2013). Redox control of the senescence regulator interleukin-1α and the secretory phenotype. J. Biol. Chem. 288, 32149–32159. doi:10.1074/jbc.m113.493841
Mcdonnell, E. E., and Buckley, C. T. (2022). Consolidating and re-evaluating the human disc nutrient microenvironment. JOR Spine 5, e1192. doi:10.1002/jsp2.1192
Mern, D. S., and Thomé, C. (2015). Identification and characterization of human nucleus pulposus cell specific serotypes of adeno-associated virus for gene therapeutic approaches of intervertebral disc disorders. BMC Musculoskelet. Disord. 16, 341. doi:10.1186/s12891-015-0799-4
Mern, D. S., Tschugg, A., Hartmann, S., and Thomé, C. (2017). Self-complementary adeno-associated virus serotype 6 mediated knockdown of ADAMTS4 induces long-term and effective enhancement of aggrecan in degenerative human nucleus pulposus cells: A new therapeutic approach for intervertebral disc disorders. PLoS One 12, e0172181. doi:10.1371/journal.pone.0172181
Mitchell, M. J., Billingsley, M. M., Haley, R. M., Wechsler, M. E., Peppas, N. A., and Langer, R. (2021). Engineering precision nanoparticles for drug delivery. Nat. Rev. Drug Discov. 20, 101–124. doi:10.1038/s41573-020-0090-8
Miyagi, M., Millecamps, M., Danco, A. T., Ohtori, S., Takahashi, K., and Stone, L. S. (2014). ISSLS Prize winner: Increased innervation and sensory nervous system plasticity in a mouse model of low back pain due to intervertebral disc degeneration. Spine (Phila Pa 1976) 39, 1345–1354. doi:10.1097/brs.0000000000000334
Mohd Isa, I. L., Mokhtar, S. A., Abbah, S. A., Fauzi, M. B., Devitt, A., and Pandit, A. (2022). Intervertebral disc degeneration: Biomaterials and tissue engineering strategies toward precision medicine. Adv. Healthc. Mat. 11, e2102530. doi:10.1002/adhm.202102530
Moon, H. J., Yurube, T., Lozito, T. P., Pohl, P., Hartman, R. A., Sowa, G. A., et al. (2014). Effects of secreted factors in culture medium of annulus fibrosus cells on microvascular endothelial cells: Elucidating the possible pathomechanisms of matrix degradation and nerve in-growth in disc degeneration. Osteoarthr. Cartil. 22, 344–354. doi:10.1016/j.joca.2013.12.008
Moon, S. H., Gilbertson, L. G., Nishida, K., Knaub, M., Muzzonigro, T., Robbins, P. D., et al. (2000). Human intervertebral disc cells are genetically modifiable by adenovirus-mediated gene transfer: Implications for the clinical management of intervertebral disc disorders. Spine (Phila Pa 1976) 25, 2573–2579. doi:10.1097/00007632-200010150-00006
MuñOZ-EspíN, D., and Serrano, M. (2014). Cellular senescence: From physiology to pathology. Nat. Rev. Mol. Cell Biol. 15, 482–496. doi:10.1038/nrm3823
Murray, C. J., Vos, T., Lozano, R., Naghavi, M., Flaxman, A. D., Michaud, C., et al. (2012). Disability-adjusted life years (DALYs) for 291 diseases and injuries in 21 regions, 1990-2010: A systematic analysis for the global burden of disease study 2010. Lancet 380, 2197–2223. doi:10.1016/s0140-6736(12)61689-4
Negri, V., Pacheco-Torres, J., Calle, D., and LóPEZ-Larrubia, P. (2020). Carbon nanotubes in biomedicine. Top. Curr. Chem. (Cham). 378, 15. doi:10.1007/s41061-019-0278-8
Neidlinger-Wilke, C., Galbusera, F., Pratsinis, H., Mavrogonatou, E., Mietsch, A., Kletsas, D., et al. (2014). Mechanical loading of the intervertebral disc: From the macroscopic to the cellular level. Eur. Spine J. 23 (3), S333–S343. doi:10.1007/s00586-013-2855-9
Neidlinger-Wilke, C., Mietsch, A., Rinkler, C., Wilke, H. J., Ignatius, A., and Urban, J. (2012). Interactions of environmental conditions and mechanical loads have influence on matrix turnover by nucleus pulposus cells. J. Orthop. Res. 30, 112–121. doi:10.1002/jor.21481
Nerurkar, N. L., Elliott, D. M., and Mauck, R. L. (2010). Mechanical design criteria for intervertebral disc tissue engineering. J. Biomech. 43, 1017–1030. doi:10.1016/j.jbiomech.2009.12.001
Nguyen, N. T., Milani, A. H., Jennings, J., Adlam, D. J., Freemont, A. J., Hoyland, J. A., et al. (2019). Highly compressive and stretchable poly(ethylene glycol) based hydrogels synthesised using pH-responsive nanogels without free-radical chemistry. Nanoscale 11, 7921–7930. doi:10.1039/c9nr01535c
Nishida, K., Doita, M., Takada, T., Kakutani, K., Miyamoto, H., Shimomura, T., et al. (2006). Sustained transgene expression in intervertebral disc cells in vivo mediated by microbubble-enhanced ultrasound gene therapy. Spine (Phila Pa 1976) 31, 1415–1419. doi:10.1097/01.brs.0000219945.70675.dd
Palmerston Mendes, L., Pan, J., and Torchilin, V. P. (2017). Dendrimers as nanocarriers for nucleic acid and drug delivery in cancer therapy. Molecules 22, 1401. doi:10.3390/molecules22091401
Patel, K. P., Sandy, J. D., Akeda, K., Miyamoto, K., Chujo, T., An, H. S., et al. (2007). Aggrecanases and aggrecanase-generated fragments in the human intervertebral disc at early and advanced stages of disc degeneration. Spine (Phila Pa 1976) 32, 2596–2603. doi:10.1097/brs.0b013e318158cb85
Paul, C. P., Schoorl, T., Zuiderbaan, H. A., Zandieh Doulabi, B., Van Der Veen, A. J., Van De Ven, P. M., et al. (2013). Dynamic and static overloading induce early degenerative processes in caprine lumbar intervertebral discs. PLoS One 8, e62411. doi:10.1371/journal.pone.0062411
Paul, C. P., Zuiderbaan, H. A., Zandieh Doulabi, B., Van Der Veen, A. J., Van De Ven, P. M., Smit, T. H., et al. (2012). Simulated-physiological loading conditions preserve biological and mechanical properties of caprine lumbar intervertebral discs in ex vivo culture. PLoS One 7, e33147. doi:10.1371/journal.pone.0033147
Peng, B. G. (2013). Pathophysiology, diagnosis, and treatment of discogenic low back pain. World J. Orthop. 4, 42–52. doi:10.5312/wjo.v4.i2.42
Pfirrmann, C. W., Metzdorf, A., Zanetti, M., Hodler, J., and Boos, N. (2001). Magnetic resonance classification of lumbar intervertebral disc degeneration. Spine (Phila Pa 1976) 26, 1873–1878. doi:10.1097/00007632-200109010-00011
Phillips, K. L., Chiverton, N., Michael, A. L., Cole, A. A., Breakwell, L. M., Haddock, G., et al. (2013a). The cytokine and chemokine expression profile of nucleus pulposus cells: Implications for degeneration and regeneration of the intervertebral disc. Arthritis Res. Ther. 15, R213. doi:10.1186/ar4408
Phillips, K. L., Cullen, K., Chiverton, N., Michael, A. L., Cole, A. A., Breakwell, L. M., et al. (2015). Potential roles of cytokines and chemokines in human intervertebral disc degeneration: interleukin-1 is a master regulator of catabolic processes. Osteoarthr. Cartil. 23, 1165–1177. doi:10.1016/j.joca.2015.02.017
Phillips, K. L., Jordan-Mahy, N., Nicklin, M. J., and Le Maitre, C. L. (2013b). Interleukin-1 receptor antagonist deficient mice provide insights into pathogenesis of human intervertebral disc degeneration. Ann. Rheum. Dis. 72, 1860–1867. doi:10.1136/annrheumdis-2012-202266
Pockert, A. J., Richardson, S. M., Le Maitre, C. L., Lyon, M., Deakin, J. A., Buttle, D. J., et al. (2009). Modified expression of the ADAMTS enzymes and tissue inhibitor of metalloproteinases 3 during human intervertebral disc degeneration. Arthritis Rheum. 60, 482–491. doi:10.1002/art.24291
Qian, J., Wang, X., Su, G., Shu, X., Huang, Z., Jiang, H., et al. (2022). Platelet-rich plasma-derived exosomes attenuate intervertebral disc degeneration by promoting NLRP3 autophagic degradation in macrophages. Int. Immunopharmacol. 110, 108962. doi:10.1016/j.intimp.2022.108962
Richardson, S. M., Doyle, P., Minogue, B. M., Gnanalingham, K., and Hoyland, J. A. (2009). Increased expression of matrix metalloproteinase-10, nerve growth factor and substance P in the painful degenerate intervertebral disc. Arthritis Res. Ther. 11, R126. doi:10.1186/ar2793
Rideau, E., Dimova, R., Schwille, P., Wurm, F. R., and Landfester, K. (2018). Liposomes and polymersomes: A comparative review towards cell mimicking. Chem. Soc. Rev. 47, 8572–8610. doi:10.1039/c8cs00162f
Risbud, M. V., and Shapiro, I. M. (2014). Role of cytokines in intervertebral disc degeneration: Pain and disc content. Nat. Rev. Rheumatol. 10, 44–56. doi:10.1038/nrrheum.2013.160
Roberts, S., Urban, J. P., Evans, H., and Eisenstein, S. M. (1996). Transport properties of the human cartilage endplate in relation to its composition and calcification. Spine (Phila Pa 1976) 21, 415–420. doi:10.1097/00007632-199602150-00003
Roh, E. J., Darai, A., Kyung, J. W., Choi, H., Kwon, S. Y., Bhujel, B., et al. (2021). Genetic therapy for intervertebral disc degeneration. Int. J. Mol. Sci. 22, 1579. doi:10.3390/ijms22041579
Roughley, P. J., Melching, L. I., Heathfield, T. F., Pearce, R. H., and Mort, J. S. (2006). The structure and degradation of aggrecan in human intervertebral disc. Eur. Spine J. 15 (3), S326–S332. doi:10.1007/s00586-006-0127-7
Rutges, J. P., Duit, R. A., Kummer, J. A., Bekkers, J. E., Oner, F. C., Castelein, R. M., et al. (2013). A validated new histological classification for intervertebral disc degeneration. Osteoarthr. Cartil. 21, 2039–2047. doi:10.1016/j.joca.2013.10.001
Sato, K., Kikuchi, S., and Yonezawa, T. (1999). In vivo intradiscal pressure measurement in healthy individuals and in patients with ongoing back problems. Spine (Phila Pa 1976) 24, 2468–2474. doi:10.1097/00007632-199912010-00008
Schmitt, C., Radetzki, F., Stirnweiss, A., Mendel, T., Ludtka, C., Friedmann, A., et al. (2021). Long-term pre-clinical evaluation of an injectable chitosan nanocellulose hydrogel with encapsulated adipose-derived stem cells in an ovine model for IVD regeneration. J. Tissue Eng. Regen. Med. 15, 660–673. doi:10.1002/term.3216
Schneider, M. C., Lalitha Sridhar, S., Vernerey, F. J., and Bryant, S. J. (2020). Spatiotemporal neocartilage growth in matrix-metalloproteinase-sensitive poly(ethylene glycol) hydrogels under dynamic compressive loading: An experimental and computational approach. J. Mat. Chem. B 8, 2775–2791. doi:10.1039/c9tb02963j
Schneider, P., Thome, M., Burns, K., Bodmer, J. L., Hofmann, K., Kataoka, T., et al. (1997). TRAIL receptors 1 (DR4) and 2 (DR5) signal FADD-dependent apoptosis and activate NF-κB. Immunity 7, 831–836. doi:10.1016/s1074-7613(00)80401-x
Scott, J. E., Bosworth, T. R., Cribb, A. M., and Taylor, J. R. (1994). The chemical morphology of age-related changes in human intervertebral disc glycosaminoglycans from cervical, thoracic and lumbar nucleus pulposus and annulus fibrosus. J. Anat. 184 (1), 73–82.
SéGUIN, C. A., Pilliar, R. M., Roughley, P. J., and Kandel, R. A. (2005). Tumor necrosis factor-alpha modulates matrix production and catabolism in nucleus pulposus tissue. Spine (Phila Pa 1976) 30, 1940–1948. doi:10.1097/01.brs.0000176188.40263.f9
Sercombe, L., Veerati, T., Moheimani, F., Wu, S. Y., Sood, A. K., and Hua, S. (2015). Advances and challenges of liposome assisted drug delivery. Front. Pharmacol. 6, 286. doi:10.3389/fphar.2015.00286
Shalash, W., Ahrens, S. R., Bardonova, L. A., Byvaltsev, V. A., and Giers, M. B. (2021). Patient-specific apparent diffusion maps used to model nutrient availability in degenerated intervertebral discs. JOR Spine 4, e1179. doi:10.1002/jsp2.1179
Shamsah, A. H., Cartmell, S. H., Richardson, S. M., and Bosworth, L. A. (2019). Mimicking the annulus fibrosus using electrospun polyester blended scaffolds. Nanomater. (Basel) 9, 537. doi:10.3390/nano9040537
Sher, I., Daly, C., Oehme, D., Chandra, R. V., Sher, M., Ghosh, P., et al. (2019). Novel application of the pfirrmann disc degeneration grading system to 9.4T MRI: Higher reliability compared to 3T MRI. Spine (Phila Pa 1976) 44, E766–e773. doi:10.1097/brs.0000000000002967
Shi, C., Wu, L., Lin, W., Cai, Y., Zhang, Y., Hu, B., et al. (2019). MiR-202-3p regulates interleukin-1β-induced expression of matrix metalloproteinase 1 in human nucleus pulposus. Gene 687, 156–165. doi:10.1016/j.gene.2018.11.056
Sizikov, A. A., Kharlamova, M. V., Nikitin, M. P., Nikitin, P. I., and Kolychev, E. L. (2021). Nonviral locally injected magnetic vectors for in vivo gene delivery: A review of studies on magnetofection. Nanomater. (Basel) 11 (5), 1078. doi:10.3390/nano11051078
Skaalure, S. C., Chu, S., and Bryant, S. J. (2015). An enzyme-sensitive PEG hydrogel based on aggrecan catabolism for cartilage tissue engineering. Adv. Healthc. Mat. 4, 420–431. doi:10.1002/adhm.201400277
Sobajima, S., Shimer, A. L., Chadderdon, R. C., Kompel, J. F., Kim, J. S., Gilbertson, L. G., et al. (2005). Quantitative analysis of gene expression in a rabbit model of intervertebral disc degeneration by real-time polymerase chain reaction. Spine J. 5, 14–23. doi:10.1016/j.spinee.2004.05.251
Su, Y., Xu, C., Sun, Z., Liang, Y., Li, G., Tong, T., et al. (2019). S100A13 promotes senescence-associated secretory phenotype and cellular senescence via modulation of non-classical secretion of IL-1α. Aging (Albany NY) 11, 549–572. doi:10.18632/aging.101760
Su, Y., Zhang, B., Sun, R., Liu, W., Zhu, Q., Zhang, X., et al. (2021). PLGA-Based biodegradable microspheres in drug delivery: Recent advances in research and application. Drug Deliv. (Lond). 28, 1397–1418. doi:10.1080/10717544.2021.1938756
Sun, B., Lian, M., Han, Y., Mo, X., Jiang, W., Qiao, Z., et al. (2021a). A 3D-Bioprinted dual growth factor-releasing intervertebral disc scaffold induces nucleus pulposus and annulus fibrosus reconstruction. Bioact. Mat. 6, 179–190. doi:10.1016/j.bioactmat.2020.06.022
Sun, Z., Zhao, H., Liu, B., Gao, Y., Tang, W. H., Liu, Z. H., et al. (2021b). AF cell derived exosomes regulate endothelial cell migration and inflammation: Implications for vascularization in intervertebral disc degeneration. Life Sci. 265, 118778. doi:10.1016/j.lfs.2020.118778
Tan, H., Jin, D., Qu, X., Liu, H., Chen, X., Yin, M., et al. (2019). A PEG-Lysozyme hydrogel harvests multiple functions as a fit-to-shape tissue sealant for internal-use of body. Biomaterials 192, 392–404. doi:10.1016/j.biomaterials.2018.10.047
Tang, G., Han, X., Lin, Z., Qian, H., Chen, B., Zhou, C., et al. (2021a). Propionibacterium acnes accelerates intervertebral disc degeneration by inducing pyroptosis of nucleus pulposus cells via the ROS-NLRP3 pathway. Oxid. Med. Cell. Longev. 2021, 1–12. doi:10.1155/2021/4657014
Tang, L., Xiao, Q., Mei, Y., He, S., Zhang, Z., Wang, R., et al. (2021b). Insights on functionalized carbon nanotubes for cancer theranostics. J. Nanobiotechnology 19, 423. doi:10.1186/s12951-021-01174-y
Tang, S., Salazar-Puerta, A., Richards, J., Khan, S., Hoyland, J. A., Gallego-Perez, D., et al. (2021c). Non-viral reprogramming of human nucleus pulposus cells with FOXF1 via extracellular vesicle delivery: An in vitro and in vivo study. Eur. Cell. Mat. 41, 90–107. doi:10.22203/ecm.v041a07
Tang, Y., Wang, S., Liu, Y., and Wang, X. (2014). Microarray analysis of genes and gene functions in disc degeneration. Exp. Ther. Med. 7, 343–348. doi:10.3892/etm.2013.1421
Tuakli-Wosornu, Y. A., Terry, A., Boachie-Adjei, K., Harrison, J. R., Gribbin, C. K., Lasalle, E. E., et al. (2016). Lumbar intradiskal platelet-rich plasma (PRP) injections: A prospective, double-blind, randomized controlled study. PM&R. 8, 1–10. quiz 10. doi:10.1016/j.pmrj.2015.08.010
Urban, J. P., and Mcmullin, J. F. (1988). Swelling pressure of the lumbar intervertebral discs: Influence of age, spinal level, composition, and degeneration. Spine (Phila Pa 1976) 13, 179–187. doi:10.1097/00007632-198802000-00009
Vashist, A., Kaushik, A., Vashist, A., Sagar, V., Ghosal, A., Gupta, Y. K., et al. (2018). Advances in carbon nanotubes-hydrogel hybrids in nanomedicine for therapeutics. Adv. Healthc. Mat. 7, e1701213. doi:10.1002/adhm.201701213
Vergroesen, P. P., Van Der Veen, A. J., Van Royen, B. J., Kingma, I., and Smit, T. H. (2014). Intradiscal pressure depends on recent loading and correlates with disc height and compressive stiffness. Eur. Spine J. 23, 2359–2368. doi:10.1007/s00586-014-3450-4
Vlaeyen, J. W. S., Maher, C. G., Wiech, K., Van Zundert, J., Meloto, C. B., Diatchenko, L., et al. (2018). Low back pain. Nat. Rev. Dis. Prim. 4, 52. doi:10.1038/s41572-018-0052-1
Vo, N. V., Hartman, R. A., Yurube, T., Jacobs, L. J., Sowa, G. A., and Kang, J. D. (2013). Expression and regulation of metalloproteinases and their inhibitors in intervertebral disc aging and degeneration. Spine J. 13, 331–341. doi:10.1016/j.spinee.2012.02.027
Wallach, C. J., Kim, J. S., Sobajima, S., Lattermann, C., Oxner, W. M., Mcfadden, K., et al. (2006). Safety assessment of intradiscal gene transfer: A pilot study. Spine J. 6, 107–112. doi:10.1016/j.spinee.2005.05.002
Walsh, A. J., and Lotz, J. C. (2004). Biological response of the intervertebral disc to dynamic loading. J. Biomech. 37, 329–337. doi:10.1016/s0021-9290(03)00290-2
Wang, C., Gonzales, S., Levene, H., Gu, W., and Huang, C. Y. (2013). Energy metabolism of intervertebral disc under mechanical loading. J. Orthop. Res. 31, 1733–1738. doi:10.1002/jor.22436
Wang, D. L., Jiang, S. D., and Dai, L. Y. (2007). Biologic response of the intervertebral disc to static and dynamic compression in vitro. Spine (Phila Pa 1976) 32, 2521–2528. doi:10.1097/brs.0b013e318158cb61
Wang, D., Tai, P. W. L., and Gao, G. (2019a). Adeno-associated virus vector as a platform for gene therapy delivery. Nat. Rev. Drug Discov. 18, 358–378. doi:10.1038/s41573-019-0012-9
Wang, H., Ding, Y., Zhang, W., Wei, K., Pei, Y., Zou, C., et al. (2020a). <p>Oxymatrine liposomes for intervertebral disc treatment: Formulation, in vitro and vivo assessments</p>. Drug Des. devel. Ther. 14, 921–931. doi:10.2147/dddt.s242493
Wang, J., Markova, D., Anderson, D. G., Zheng, Z., Shapiro, I. M., and Risbud, M. V. (2011). TNF-α and IL-1β promote a disintegrin-like and metalloprotease with thrombospondin type I motif-5-mediated aggrecan degradation through syndecan-4 in intervertebral disc. J. Biol. Chem. 286, 39738–39749. doi:10.1074/jbc.m111.264549
Wang, K., Chen, T., Ying, X., Zhang, Z., Shao, Z., Lin, J., et al. (2019b). Ligustilide alleviated IL-1β induced apoptosis and extracellular matrix degradation of nucleus pulposus cells and attenuates intervertebral disc degeneration in vivo. Int. Immunopharmacol. 69, 398–407. doi:10.1016/j.intimp.2019.01.004
Wang, K., Sun, Q., Zhong, X., Zeng, M., Zeng, H., Shi, X., et al. (2020b). Structural mechanism for GSDMD targeting by autoprocessed caspases in pyroptosis. Cell 180, 941–955.e20. e20. doi:10.1016/j.cell.2020.02.002
Wang, L., Niu, X., Song, Q., Jia, J., Hao, Y., Zheng, C., et al. (2020c). A two-step precise targeting nanoplatform for tumor therapy via the alkyl radicals activated by the microenvironment of organelles. J. Control. Release 318, 197–209. doi:10.1016/j.jconrel.2019.10.017
Wang, Y., Videman, T., and Battié, M. C. (2012). Lumbar vertebral endplate lesions: Prevalence, classification, and association with age. Spine (Phila Pa 1976) 37, 1432–1439. doi:10.1097/brs.0b013e31824dd20a
Wehling, P., Schulitz, K. P., Robbins, P. D., Evans, C. H., and Reinecke, J. A. (1997). Transfer of genes to chondrocytic cells of the lumbar spine. Proposal for a treatment strategy of spinal disorders by local gene therapy. Spine (Phila Pa 1976) 22, 1092–1097. doi:10.1097/00007632-199705150-00008
Wei, F., Zhong, R., Zhou, Z., Wang, L., Pan, X., Cui, S., et al. (2014). In vivo experimental intervertebral disc degeneration induced by bleomycin in the rhesus monkey. BMC Musculoskelet. Disord. 15, 340. doi:10.1186/1471-2474-15-340
Wei, X., Gong, C., Gou, M., Fu, S., Guo, Q., Shi, S., et al. (2009). Biodegradable poly(ɛ-caprolactone)–poly(ethylene glycol) copolymers as drug delivery system. Int. J. Pharm. X. 381, 1–18. doi:10.1016/j.ijpharm.2009.07.033
Weiler, C., Nerlich, A. G., Zipperer, J., Bachmeier, B. E., and Boos, N. (2002). 2002 SSE award competition in basic science: Expression of major matrix metalloproteinases is associated with intervertebral disc degradation and resorption. Eur. Spine J. 11, 308–320. doi:10.1007/s00586-002-0472-0
Wilke, H. J., Neef, P., Caimi, M., Hoogland, T., and Claes, L. E. (1999). New in vivo measurements of pressures in the intervertebral disc in daily life. Spine (Phila Pa 1976) 24, 755–762. doi:10.1097/00007632-199904150-00005
Wu, J., Wang, D., Ruan, D., He, Q., Zhang, Y., Wang, C., et al. (2014). Prolonged expansion of human nucleus pulposus cells expressing human telomerase reverse transcriptase mediated by lentiviral vector. J. Orthop. Res. 32, 159–166. doi:10.1002/jor.22474
Wu, Y., Cisewski, S. E., Wegner, N., Zhao, S., Pellegrini, V. D., Slate, E. H., et al. (2016). Region and strain-dependent diffusivities of glucose and lactate in healthy human cartilage endplate. J. Biomech. 49, 2756–2762. doi:10.1016/j.jbiomech.2016.06.008
Wuertz, K., Godburn, K., Maclean, J. J., Barbir, A., Donnelly, J. S., Roughley, P. J., et al. (2009). In vivo remodeling of intervertebral discs in response to short- and long-term dynamic compression. J. Orthop. Res. 27, 1235–1242. doi:10.1002/jor.20867
Xie, J., Li, B., Zhang, P., Wang, L., Lu, H., and Song, X. (2018). Osteogenic protein-1 attenuates the inflammatory cytokine-induced NP cell senescence through regulating the ROS/NF-κB pathway. Biomed. Pharmacother. 99, 431–437. doi:10.1016/j.biopha.2018.01.053
Xing, H., Zhang, Z., Mao, Q., Wang, C., Zhou, Y., Zhou, X., et al. (2021). Injectable exosome-functionalized extracellular matrix hydrogel for metabolism balance and pyroptosis regulation in intervertebral disc degeneration. J. Nanobiotechnology 19, 264. doi:10.1186/s12951-021-00991-5
Xu, H., Mei, Q., He, J., Liu, G., Zhao, J., and Xu, B. (2014a). Correlation of matrix metalloproteinases-1 and tissue inhibitor of metalloproteinases-1 with patient age and grade of lumbar disk herniation. Cell biochem. Biophys. 69, 439–444. doi:10.1007/s12013-014-9815-9
Xu, H., Mei, Q., Xu, B., Liu, G., and Zhao, J. (2014b). Expression of matrix metalloproteinases is positively related to the severity of disc degeneration and growing age in the East Asian lumbar disc herniation patients. Cell biochem. Biophys. 70, 1219–1225. doi:10.1007/s12013-014-0045-y
Xu, P., Guan, J., Chen, Y., Xiao, H., Yang, T., Sun, H., et al. (2021). Stiffness of photocrosslinkable gelatin hydrogel influences nucleus pulposus cell propertiesin vitro. J. Cell. Mol. Med. 25, 880–891. doi:10.1111/jcmm.16141
Yang, M., Peng, Y., Liu, W., Zhou, M., Meng, Q., and Yuan, C. (2019). Sirtuin 2 expression suppresses oxidative stress and senescence of nucleus pulposus cells through inhibition of the p53/p21 pathway. Biochem. Biophys. Res. Commun. 513, 616–622. doi:10.1016/j.bbrc.2019.03.200
Yu, W., Fu, J., Liu, Y., Wu, Y., and Jiang, D. (2018). Osteogenic protein-1 inhibits nucleus pulposus cell apoptosis through regulating the NF-κB/ROS pathway in an inflammation environment. Biosci. Rep. 38, BSR20181530. doi:10.1042/bsr20181530
Yurube, T., Takada, T., Suzuki, T., Kakutani, K., Maeno, K., Doita, M., et al. (2012). Rat tail static compression model mimics extracellular matrix metabolic imbalances of matrix metalloproteinases, aggrecanases, and tissue inhibitors of metalloproteinases in intervertebral disc degeneration. Arthritis Res. Ther. 14, R51. doi:10.1186/ar3764
Zhang, J., Tong, D., Song, H., Ruan, R., Sun, Y., Lin, Y., et al. (2022a). Osteoimmunity-regulating biomimetically hierarchical scaffold for augmented bone regeneration. Adv. Mat. 34, e2202044. doi:10.1002/adma.202202044
Zhang, Q., Huang, M., Wang, X., Xu, X., Ni, M., and Wang, Y. (2012). Negative effects of ADAMTS-7 and ADAMTS-12 on endplate cartilage differentiation. J. Orthop. Res. 30, 1238–1243. doi:10.1002/jor.22069
Zhang, X., Wan, H., Lan, W., Miao, F., Qin, M., Wei, Y., et al. (2022b). Fabrication of adhesive hydrogels based on poly (acrylic acid) and modified hyaluronic acid. J. Mech. Behav. Biomed. Mat. 126, 105044. doi:10.1016/j.jmbbm.2021.105044
Zhao, C. Q., Wang, L. M., Jiang, L. S., and Dai, L. Y. (2007). The cell biology of intervertebral disc aging and degeneration. Ageing Res. Rev. 6, 247–261. doi:10.1016/j.arr.2007.08.001
Zhao, D., Zhu, T., Li, J., Cui, L., Zhang, Z., Zhuang, X., et al. (2021a). Poly(lactic-co-glycolic acid)-based composite bone-substitute materials. Bioact. Mat. 6, 346–360. doi:10.1016/j.bioactmat.2020.08.016
Zhao, K., An, R., Xiang, Q., Li, G., Wang, K., Song, Y., et al. (2021b). Acid-sensing ion channels regulate nucleus pulposus cell inflammation and pyroptosis via the NLRP3 inflammasome in intervertebral disc degeneration. Cell Prolif. 54, e12941. doi:10.1111/cpr.12941
Zheng, K., and Du, D. (2021). Recent advances of hydrogel-based biomaterials for intervertebral disc tissue treatment: A literature review. J. Tissue Eng. Regen. Med. 15, 299–321. doi:10.1002/term.3172
Zheng, Y., Liu, C., Ni, L., Liu, Z., Mirando, A. J., Lin, J., et al. (2018). Cell type-specific effects of Notch signaling activation on intervertebral discs: Implications for intervertebral disc degeneration. J. Cell. Physiol. 233, 5431–5440. doi:10.1002/jcp.26385
Zhou, X., Hou, C., Chang, T. L., Zhang, Q., and Liang, J. F. (2020). Controlled released of drug from doubled-walled PVA hydrogel/PCL microspheres prepared by single needle electrospraying method. Colloids Surfaces B Biointerfaces 187, 110645. doi:10.1016/j.colsurfb.2019.110645
Zhu, M., Tan, J., Liu, L., Tian, J., Li, L., Luo, B., et al. (2021). Construction of biomimetic artificial intervertebral disc scaffold via 3D printing and electrospinning. Mater. Sci. Eng. C 128, 112310. doi:10.1016/j.msec.2021.112310
Zhu, T., Cui, Y., Zhang, M., Zhao, D., Liu, G., and Ding, J. (2020). Engineered three-dimensional scaffolds for enhanced bone regeneration in osteonecrosis. Bioact. Mat. 5, 584–601. doi:10.1016/j.bioactmat.2020.04.008
Zhu, T., Jiang, M., Zhang, M., Cui, L., Yang, X., Wang, X., et al. (2022a). Biofunctionalized composite scaffold to potentiate osteoconduction, angiogenesis, and favorable metabolic microenvironment for osteonecrosis therapy. Bioact. Mat. 9, 446–460. doi:10.1016/j.bioactmat.2021.08.005
Zhu, T., Jiang, M., Zhang, M., Cui, L., Yang, X., Wang, X., et al. (2022b). Construction and validation of steroid-induced rabbit osteonecrosis model. MethodsX 9, 101713. doi:10.1016/j.mex.2022.101713
Zhu, Y., Jia, H., Li, J., Ren, S., Huang, Z., Li, F., et al. (2018). Associations between variants in BDNF/BDNFOS gene and lumbar disc herniation risk among han Chinese people. Sci. Rep. 8, 12782. doi:10.1038/s41598-018-31146-6
Keywords: delivery system, intervertebral disc degeneration, biomaterial, nanometer materials, low back pain
Citation: Liu Z and Fu C (2022) Application of single and cooperative different delivery systems for the treatment of intervertebral disc degeneration. Front. Bioeng. Biotechnol. 10:1058251. doi: 10.3389/fbioe.2022.1058251
Received: 30 September 2022; Accepted: 01 November 2022;
Published: 14 November 2022.
Edited by:
Mingqiang Li, Third Affiliated Hospital of Sun Yat-sen University, ChinaReviewed by:
Yilong Cheng, Xi’an Jiaotong University, ChinaCopyright © 2022 Liu and Fu. This is an open-access article distributed under the terms of the Creative Commons Attribution License (CC BY). The use, distribution or reproduction in other forums is permitted, provided the original author(s) and the copyright owner(s) are credited and that the original publication in this journal is cited, in accordance with accepted academic practice. No use, distribution or reproduction is permitted which does not comply with these terms.
*Correspondence: Changfeng Fu, ZnVjZkBqbHUuZWR1LmNu
Disclaimer: All claims expressed in this article are solely those of the authors and do not necessarily represent those of their affiliated organizations, or those of the publisher, the editors and the reviewers. Any product that may be evaluated in this article or claim that may be made by its manufacturer is not guaranteed or endorsed by the publisher.
Research integrity at Frontiers
Learn more about the work of our research integrity team to safeguard the quality of each article we publish.