- Ecobiomaterial Research Laboratory, School of Biological Sciences, Universiti Sains Malaysia, Pulau Pinang, Malaysia
Polyhydroxyalkanoates (PHAs) have garnered global attention to replace petroleum-based plastics in certain applications due to their biodegradability and sustainability. Among the different types of PHAs, poly(3-hydroxybutyrate-co-3-hydroxyhexanoate) [P(3HB-co-3HHx)] copolymer has similar properties to commodity plastics, making them a suitable candidate to replace certain types of single-use plastics, medical devices, and packaging materials. The degradation rate of P(3HB-co-3HHx) is faster than the commercial petroleum-based plastics which take a very long time to be degraded, causing harmful pollution to both land and marine ecosystem. The biodegradability of the P(3HB-co-3HHx) is also dependent on its 3HHx molar composition which in turn influences the crystallinity of the material. Various metabolic pathways like the common PHA biosynthesis pathway, which involves phaA, phaB, and phaC, β-oxidation, and fatty acids de novo synthesis are used by bacteria to produce PHA from different carbon sources like fatty acids and sugars, respectively. There are various factors affecting the 3HHx molar composition of P(3HB-co-3HHx), like PhaCs, the engineering of PhaCs, and the metabolic engineering of strains. It is crucial to control the 3HHx molar composition in the P(3HB-co-3HHx) as it will affect its properties and applications in different fields.
Introduction
Petroleum-based plastics are used in our daily life due to their characteristics like being cheap, light, resistant to chemicals, and convenient (Loo and Sudesh, 2007). Due to those desirable characteristics, they are applied in many sectors, such as packaging, medical equipment, household utensils, construction, etc. However, it was estimated that more than a million tons of plastic waste are being disposed into land and marine environment yearly due to improper disposal (Alabi et al., 2019; Masry et al., 2021). When exposed to sunlight and wind, plastic wastes are broken down into microplastics, causing toxic effects on aquatic life and human health (Bajt, 2021).
The use of bio-based plastics as one of the alternatives to replace single-use petroleum-based plastics may be one of the options for solving this issue. There are several potential bio-based plastics, such as polyhydroxyalkanoates (PHAs), polylactides (PLA), polysaccharides, etc. Among the bio-based plastics, PHAs have been reported to have interesting properties like biodegradable, thermoplastic, renewable, and can be tailored to fit various applications attracted both academic and industrial (Sudesh and Iwata, 2008). PHA was first discovered by Lemoigne in Bacillus megaterium (Lemoigne, 1926). The timeline of the P(3HB-co-3HHx) developments is shown in Figure 1. PHAs are bio-based polymers produced by a wide range of microorganisms under deprived nutrients and excessive carbon sources (Anderson and Dawes, 1990; Fukui and Doi, 1998). They are deposited as intracellular granules and function as energy or carbon reservoir (Anderson and Dawes, 1990; Sudesh et al., 2000). Figure 2 shows the PHA granules in cell cytoplasm synthesized by Cupriavidus necator transformant under phase contrast light microscope.
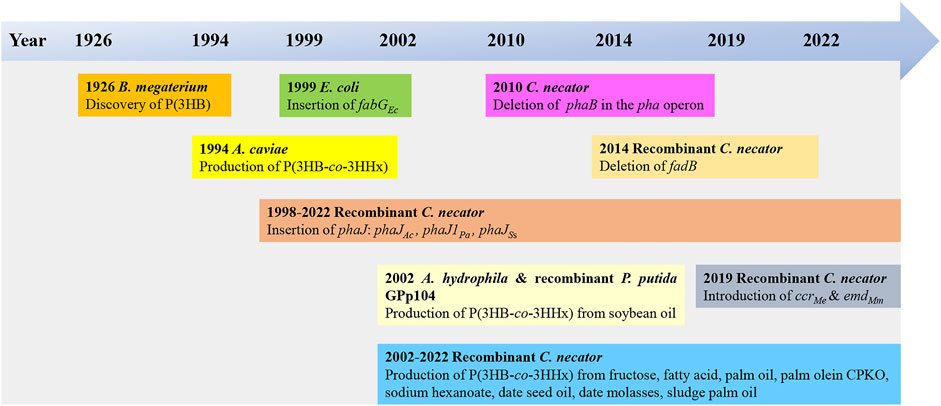
FIGURE 1. Timeline of the P(3HB-co-3HHx) developments (Lemoigne, 1926; Shimamura et al., 1994; Fukui et al., 1998; Asrar et al., 2002; Fukui et al., 2002; Tsuge et al., 2004; Loo et al., 2005; Budde, 2010; Wong et al., 2012; Insomphun et al., 2014; Volova et al., 2016; Murugan et al., 2017; Purama et al., 2018; Thinagaran and Sudesh, 2019; Zhang et al., 2019; Tan et al., 2020; Tan et al., 2022)
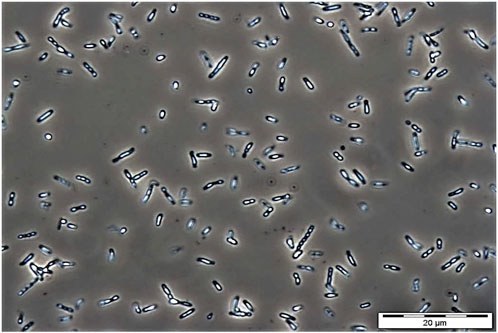
FIGURE 2. Phase contrast microscopic image of PHA granules in C. necator mutant Re2058 harboring plasmid pHT1 with phaC from Chromobacterium sp. USM2 (phaCCs) containing P(3HB-co-3 mol% 3HHx) copolymer after cultivating in minimal medium at 30°C, 200 rpm for 48 h with 0.54 g/L of urea as nitrogen source and supplemented with crude palm kernel oil (CPKO). Magnification: 1000 ×. The PHA content produced was 60 wt%.
PHAs can be divided into three groups, short-chain-length PHA (SCL-PHA) of 3–5 carbon units, medium-chain-length PHA (MCL-PHA) of 6–14 carbon units, and a mixture of both SCL- and MCL-PHA of 3–14 carbon units (Sudesh et al., 2000). Examples of SCL monomeric units are 3-hydroxybutyrate (3HB) and 3-hydroxyvalerate (3HV), while MCL monomeric units are 3-hydroxyhexanoate (3HHx) and 3-hydroxydodecanoate (3HD) (Sudesh, 2012). Example of the mixture of SCL- and MCL-PHA is poly [(R)-3-hydroxybutyrate-co-(R)-3-hydroxyhexanoate] P(3HB-co-3HHx). The monomers incorporated into PHA polymers will affect the thermal and physical properties of the PHAs produced.
Poly(3-hydroxybutyrate) [P(3HB)] homopolymer is brittle, stiff, has high crystallinity, and low elongation at break, causing it to be limited in many applications (Yu, 2007). This drawback of PHA homopolymers can be overcome by copolymerizing P(3HB) with MCL monomers for better properties (Noda et al., 2005). For instance, the incorporation of MCL-PHA monomer like 3HHx into P(3HB) results in P(3HB-co-3HHx) copolymer, which has elastomeric properties such as high elasticity, low crystallinity, and high elongation at break. The flexibility of this copolymer can be varied depending on the 3HHx molar compositions (Asrar et al., 2002). P(3HB-co-3HHx) with 17 mol% 3HHx molar fraction was also reported to have similar properties as low-density polyethylene (LDPE) (Doi, 1990; Doi et al., 1995).
PHA synthase (PhaC) is the key enzyme in PHA production since it is responsible for catalyzing the polymerization of PHA monomers into PHA polymers. In addition, it also determines the monomer composition integrated into the PHA polymer, which will impact the properties of the PHA produced. Based on the substrate specificities of PhaC and subunit composition, PhaCs are categorized into four main classes: class I, II, III and IV. Class I, III and IV PhaC prefer SCL-PHA monomer, whereas class II PhaC prefers MCL-PHA monomer. Class I and II PhaCs are single-unit enzymes. Class III consists of two subunits, PhaC and PhaE, while class IV consists of PhaC and PhaR (Rehm, 2003).
This present paper reviews the properties, metabolic pathways of P(3HB-co-3HHx) production, factors affecting the 3HHx molar composition in P(3HB-co-3HHx) copolymer, and potential applications of P(3HB-co-3HHx).
Properties of P(3HB-co-3HHx)
P(3HB-co-3HHx) copolymer consists of two monomer compositions, which are 3HB and 3HHx. The chemical structure of P(3HB-co-3HHx) is shown in Figure 3.
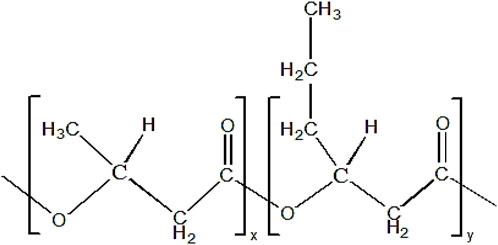
FIGURE 3. Chemical structure of P(3HB-co-3HHx). ‘x’ and ‘y’ indicate the repeating units of monomer.
P(3HB) is a homopolymer of SCL monomer unit consisting of four carbon atoms and is the most common type of PHA. It is reported that the properties of P(3HB) are approximately similar to commercial plastics such as polypropylene in terms of tensile strength and Young’s Modulus (Sudesh and Iwata, 2008). However, the elongation at break of P(3HB) is 5% which is way lower than polypropylene which is 400% (Sudesh et al., 2000). The melting temperature (Tm) of P(3HB) is approximately 180°C, and the degree of crystallinity is 55–80% (Holmes, 1988). Due to its brittleness, stiffness and high Tm, P(3HB) has poor processability and limits applications.
On the other hand, 3HHx is a MCL monomer unit that consists of six carbon atoms. Since 3HHx has a longer alkyl side chain, it cannot crystallize in the 3HB lattice and hence, avoiding the isodimorphism phenomenon. MCL-PHAs are amorphous in contrast to crystalline SCL-PHAs. MCL-PHAs exhibit elastomeric properties, soft, low Tm, low glass transition temperature (Tg), low tensile strength, and high elongation at break (Yu, 2007; Reddy et al., 2022).
The mixture of SCL- and MCL-PHA has superior thermal and mechanical properties than PHA homopolymers. Compared to SCL-PHA and MCL-PHA, the mixture of SCL- and MCL-PHA results in lower Tm, lower degree of crystallinity, and higher elongation at break (Doi et al., 1995; Kellerhals et al., 2000; Noda et al., 2005).
The flexibility of P(3HB-co-3HHx) also depends on 3HHx monomer compositions (Shimamura et al., 1994). As 3HHx monomeric units increase, it becomes more flexible, softer, and has better processability. Hence, its improved properties and processability attract industrial attention for various applications, especially in packaging and single-use plastic.
The physicomechanical properties of P(3HB-co-3HHx) with different 3HHx molar fractions have been summarized in Table 1. In general, as the 3HHx molar fraction increases, the Mw, Tm, Tg, Young’s modulus, and tensile strength decrease, whereas the elongation at break of the copolymer increases.
Biodegradability of P(3HB-co-3HHx)
Previous research has established that P(3HB-co-3HHx) can be biodegraded in aerobic environments such as seawater, freshwater, soil, and domestic compost (Morse et al., 2011). Under aerobic conditions, P(3HB-co-3HHx) is degraded into water and carbon dioxide. On the other hand, anaerobic biodegradation has also been carried out using PHB depolymerase, whereby P(3HB-co-3HHx) is degraded into water and methane (Abe and Doi, 1999; Lee and Choi, 1999). P(3HB-co-3HHx) takes around six months to be degraded in seawater (KANEKA Corporation, 2019). There are a few factors that affect the biodegradation of PHA copolymers, such as the environment, the microbial community, types of PHA monomer composition, temperature, and humidity.
The rate of PHA degradation increases as the degree of crystallinity decreases (Morse et al., 2011). Morse and co-workers reported that the films made from P(3HB-co-3HHx) with 10 mol% of 3HHx had a faster degradation rate than those with 3.8 mol% of 3HHx. Furthermore, Volova and co-workers reported that the biodegradability of P(3HB-co-3HHx), a PHA copolymer was faster than P(3HB), a PHA homopolymer (Volova et al., 2017). This is because P(3HB) has higher crystallinity than P(3HB-co-3HHx), leading to a lower degradation rate. Moreover, it was reported that the rate of enzymatic degradation of P(3HB-co-3HHx) film by P(3HB) depolymerase gets higher as the 3HHx content increases to 15 mol% (Shimamura et al., 1994). When the 3HHx mol% in the P(3HB-co-3HHx) increases, the degree of crystallinity of P(3HB-co-3HHx) decreases, thereby increasing the biodegradation rate of the polymer. It is also reported that the degradation rate of the amorphous polymer was faster than that of highly crystalline polymer (Molitoris et al., 1996).
Besides that, Wang and co-workers reported that P(3HB-co-3HHx) polymer surface morphology could affect its biodegradation rate. Molitoris and co-workers reported that the degradation was faster on PHA with fissures on its surface than on PHA with a smooth surface (Molitoris et al., 1996). A porous and rough surface tends to have a faster degradation rate as it facilitates the attachment of lipase or bacteria to the film to begin the degradation process (Wang et al., 2004).
Metabolic pathway of P(3HB-co-3HHx) production
The most common PHA biosynthesis pathway consists of three genes which are β-ketothiolase (PhaA) coded by phaA gene, NADPH-dependent acetoacetyl-CoA reductase (PhaB) coded by phaB gene and PhaC coded by phaC gene (Anderson and Dawes, 1990). The acetyl-CoA from various pathways like fatty acid β-oxidation, glycolysis, and many more will first be converted to acetoacetyl-CoA by PhaA. The acetoacetyl-CoA will then be reduced to (R)-3-hydroxybutyryl-CoA [(R)-3HB-CoA] by PhaB and followed by incorporation into PHA by PhaC (Figure 4).
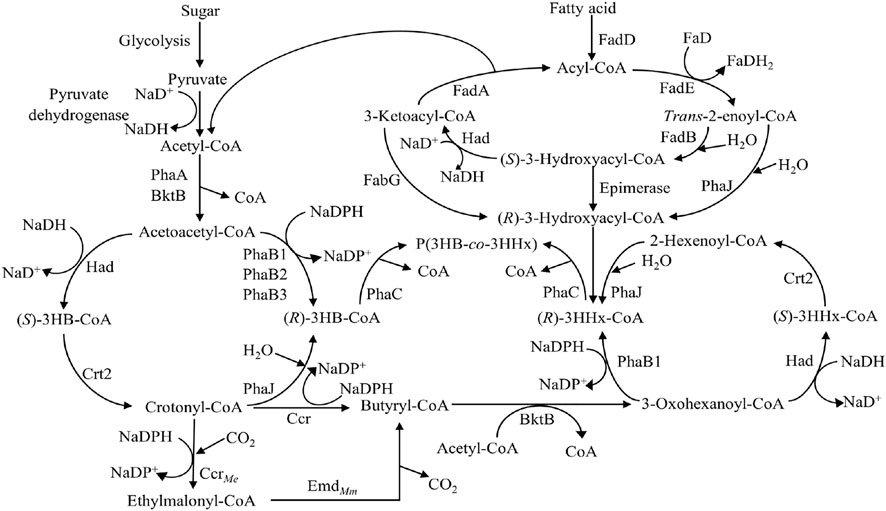
FIGURE 4. Metabolic pathway of P(3HB) and P(3HB-co-3HHx) production (Sudesh et al., 2000; Tsuge, 2002; Zhang et al., 2019). Abbreviation: PHA, polyhydroxyalkanoate; PhaA, β-ketothiolase; PhaB, NADPH-dependent acetoacetyl-CoA reductase; PhaC, PHA synthase; FadA, 3-ketoacyl-CoA thiolase; FadB, enoyl-CoA hydratase; FadD, acyl-CoA synthetase; FadE, acyl-CoA dehydrogenase; FabG, 3-ketoacyl-CoA reductase; 3HB-CoA, 3-hydroxybutyryl-CoA; 3HHx-CoA, 3-hydroxyhexanoyl-CoA; PhaJ, (R)-specific enoyl-CoA hydratase; Had, 3-hydroxyacyl-CoA dehydrogenase; Crt2, crotonase; Ccr, crotonyl-CoA reductase; CcrMe, crotonyl-CoA carboxylase from Methylorubrum extorquens; EmdMm, ethylmalonyl-CoA decaroboxylase from Mus musculus.
When fatty acids are used as carbon sources, fatty acids will be converted to acyl-CoA by acyl-CoA synthetase (FadD). Acyl-CoA will be oxidized to trans-enoyl-CoA catalyzed by acyl-CoA dehydrogenase (FadE) followed by hydration into (S)-3-hydroxyacyl-CoA by enoyl-CoA hydratase (FadB). (S)-3-hydroxyacyl-CoA will then be oxidized into 3-ketoacyl-CoA by 3-hydroxyacyl-CoA dehydrogenase (Had). Lastly, 3-ketoacyl-CoA thiolase (FadA) will convert 3-ketoacyl-CoA into acyl-CoA with two carbon atoms lesser, releasing one acetyl-CoA. This acetyl-CoA can be converted to PHA using the previous pathway. Six carbons of (S)-3-hydroxyacyl-CoA or (S)-3-hydroxyhexanoyl-CoA [(S)-3HHx-CoA] will be converted into (R)-3-hydroxyhexanoyl-CoA [(R)-3HHx-CoA] by (R)-specific enoyl-CoA hydratase (PhaJ). (R)-3HHx-CoA will then be incorporated into P(3HB-co-3HHx) by PhaC (Figure 4) (Tsuge, 2002).
Another engineered pathway involving the formation of P(3HB-co-3HHx) is the fatty acids de novo synthesis pathway, where sugar is used as the sole carbon source. This pathway begins with sugar which will be converted to pyruvate via glycolysis and decarboxylated into acetyl-CoA by pyruvate dehydrogenase. The acetyl-CoA will be converted into acetoacetyl-CoA by PhaA. The acetoacetyl-CoA formed will be reduced to (S)-3-hydroxybutyryl-CoA [(S)-3HB-CoA] by Had followed by conversion to crotonyl-CoA by crotonase (Crt2). Crotonyl-CoA will either be converted to (R)-3HB-CoA by PhaJ or butyryl-CoA. The (R)-3HB-CoA will be incorporated into P(3HB-co-3HHx) as 3HB. The crotonyl-CoA will be reduced to butyryl-CoA by crotonyl-CoA reductase (Ccr). The butyryl-CoA will be converted to 3-oxohexanoyl-CoA followed by reduction to (S)-3HHx-CoA by Had. (S)-3HHx-CoA will be converted to 2-hexenoyl-CoA by Crt2 and then hydrated to (R)-3HHx-CoA by PhaJ. (R)-3HHx-CoA will be incorporated into P(3HB-co-3HHx) by PhaC as 3HHx (Figure 4) (Zhang et al., 2019).
Factors affecting the 3-hydroxyhexanoate (3HHx) compositions of P(3HB-co-3HHx)
PhaCs
One of the main factors affecting the 3HHx molar composition is the PhaC. PhaC is the most important protein in PHA biosynthesis because it determines the type of PHA produced (Sudesh et al., 2000). Hence, many researchers have dedicated their time in search of a good naturally occurring PhaC.
As mentioned above in the introduction, class I, III, and IV PhaCs could only incorporate SCL-PHA monomers, while class II PhaCs could incorporate MCL-PHA. There is also a particular group of class I PhaC that will incorporate both SCL- and MCL-PHA monomers into the PHA polymer produced (Neoh et al., 2022). For example, PhaCs from A. caviae (PhaCAc), Rhodococcus aetherivorans I24 (PhaC1Ra and PhaC2Ra), PhaC from mangrove soil (PhaCBP-M-CPF4), Chromobacterium sp. USM2 (PhaCCs) and Pseudomonas sp. 61–3 (PhaC1Ps and PhaC2Ps) (Doi et al., 1995; Fukui and Doi, 1997; Matsusaki et al., 1998; Bhubalan et al., 2010; Budde et al., 2011; Foong et al., 2018).
A. caviae was one of the first bacterial strains to be discovered to produce P(3HB-co-3HHx) (Shimamura et al., 1994). PhaCAc possesses substrate specificity for copolymerizing both 3HB-CoA and 3HHx-CoA into P(3HB-co-3HHx) copolymer using alkanoic acids and olive oil (Doi et al., 1995). Heterologous expression of PhaCAc in C. necator mutant, PHB−4, and Pseudomonas putida GPp104 could produce P(3HB-co-3HHx) with a maximum 22 mol% of 3HHx using octanoate and 40 mol% of 3HHx using hexanoate respectively (Fukui & Doi, 1997). The recombinant strain A. eutrophus (PHB−4/pJRDEE32d13) harboring phaCAc could produce P(3HB-co-3HHx) with 4 mol% of 3HHx content using olive oil (Fukui and Doi, 1998). Kahar and co-workers reported that recombinant strain C. necator PHB−4 harboring pJRDEE32d13 inserted with phaCAc could accumulate P(3HB-co-3HHx) with 5 mol% of 3HHx content from soybean oil (Kahar et al., 2004).
Budde and co-workers reported two different PhaCs, PhaC1Ra and PhaC2Ra, in R. aetherivorans I24 (Budde et al., 2011). The heterologous expression of PhaC1Ra and PhaC2Ra in recombinant C. necator could produce P(3HB-co-3HHx). The study showed that recombinant C. necator strains, Re2000 and Re 2001, expressing PhaC1Ra and PhaC2Ra, respectively, could produce P(3HB-co-3HHx) using hexanoate and octanoate. Re2000 and Re2001 could produce 11.5 mol% of 3HHx and 18.9 mol% of 3HHx from hexanoate, respectively, while producing 6.6 mol% of 3HHx and 10.4 mol% of 3HHx from octanoate, respectively (Budde et al., 2011). This result showed that PhaC2Ra produced P(3HB-co-3HHx) with higher 3HHx content than PhaC1Ra from fatty acids.
PhaCBP-M-CPF4 was discovered in mangrove soil metagenome at Balik Pulau, Malaysia. It has wide substrate specificity as it can incorporate both SCL-PHA and MCL-PHA. The heterologous expression of PhaCBP-M-CPF4 in C. necator PHB−4 produced P(3HB-co-3HHx) with 7 mol% of 3HHx from CPKO and 18 mol% of 3HHx when co-fed with fructose and sodium hexanoate (Foong et al., 2018). Besides C. necator PHB−4, another C. necator PHA-negative mutant strains, H16ΔC, Re2058, and Re2160 harboring phaCBP-M-CPF4 were reported to be able to produce P(3HB-co-3HHx) ranging from 3–18 mol% of 3HHx from CPKO (Tan et al., 2020).
Chromobacterium sp. strain USM2 was discovered in Langkawi, Malaysia (Yong, 2009). It was reported that PhaCCs heterologous expressed in C. necator PHB−4 could synthesize P(3HB-co-3HHx) copolymer with 4 mol% of 3HHx using CPKO (Bhubalan et al., 2010). In addition, Pseudomonas sp. 61–3 isolated from soil possesses two different PhaCs, PhaC1Ps and PhaC2Ps. It could incorporate 3HB and 3HA units of C4 and C12 when supplemented with sugars and alkanoic acids. The heterologous expression of PhaC1Ps and PhaC2Ps in P. putida GPp104 and C. necator PHB−4 could produce P(3HB-co-3HHx) with 3HHx ranging from 1 to 16 mol% from alkanoic acids (Matsusaki et al., 1998).
Based on the above, it is clear that PhaCs can affect the 3HHx molar composition of P(3HB-co-3HHx) produced. The difference in 3HHx molar composition is due to the substrate specificity of the PhaC towards 3HHx. Some PhaCs with higher substrate specificity towards 3HHx tend to produce P(3HB-co-3HHx) with higher 3HHx, and some PhaCs with lower substrate specificity towards 3HHx will lead to lower 3HHx. This may be due to the substrate entrance channel and the binding pocket of the PhaC where folding of the amino acid forming both substrate entrance channel and binding pocket are better in binding to 3HHx-CoA and hence, incorporating into P(3HB-co-3HHx). However, this is yet to be reported as to date, there is no co-crystal structure of (R)-3HHx-CoA with PhaC, but there is a co-crystal structure of PhaCs with CoA, which was reported by Chek and co-workers elucidating how the CoA group is bound to the binding pocket of PhaCCs-CAT (Chek et al., 2020). The comparison of the production titers of the P(3HB-co-3HHx) from different researchers is shown in Table 2.
Engineering of PhaCs
Besides naturally occurring PhaCs, engineering existing PhaCs could also affect the 3HHx molar composition in P(3HB-co-3HHx). In vitro evolution system can be used to improve the activity and broaden the substrate specificity of PhaC.
Kichise and co-workers had selected PhaCAc for in vitro evolution as it can produce P(3HB-co-3HHx) copolymer from plant oils or alkanoic acid. In vitro evolution of PhaCAc was carried out using error-prone PCR, and as a result, two PhaCAc mutants (E2-50 and T3-11) were obtained, which had improved 3HHx incorporation. The PhaCAc mutants (E2-50 and T3-11) in E. coli LS5218 could synthesize P(3HB-co-3HHx) with higher 3HHx content, which was 18 mol% of 3HHx and 16 mol% of 3HHx, respectively compared to wild-type with 10 mol% of 3HHx using sodium dodecanoate. P(3HB-co-3HHx) accumulated was 3-fold higher than the wild-type PhaCAc. It was discovered that E2-50 and T3-11 have the single amino acid substitution of N149S and D171G, respectively (Kichise et al., 2002).
Tsuge and co-workers further studied the synergistic effects of PhaCAc mutants, N149S and D171G double mutation (NSDG) in C. necator PHB−4 for PHA production. The NSDG mutant was able to produce P(3HB-co-3HHx) with 3HHx as high as 18.1 mol%, which was higher than the wild type with 12.2 mol% using sodium octanoate. Besides sodium octanoate, the NSDG mutant could also produce P(3HB-co-3HHx) with 5.2 mol% of 3HHx from soybean oil, which was higher than the wild type with only 3.5 mol% of 3HHx. The NSDG mutant could also produce P(3HB-co-3HHx) with 2.90 × 106 Da from sodium octanoate, which is near to ultrahigh molecular weight PHA (UHMW-PHA), and it is suitable for making strong films and fibers (Iwata, 2005; Tsuge et al., 2007). N149S mutation increased the incorporation of 3HA into PHA polymer and the molecular weight of the polymer, whereas D171G mutation increased PHA accumulation. Hence, the synergistic effect of N149S and D171G double mutation on PHA production was higher than predicted (Tsuge et al., 2007).
PhaCCn belongs to the type I PHA synthase, which shows substrate specificity towards (R)-3HA-CoAs with the acyl chain length of C3–C5. The alanine residue at position 510 may define the substrate specificity and the properties of the enzyme. Tsuge and co-workers found out that the performance of saturation point mutagenesis at position 510 in PhaCCn using a PCR-based method could drastically impact the substrate specificity and polymerization activity for in vivo PHA biosynthesis in E. coli and C. necator PHB−4. The co-expression of the mutant PhaCCn and PhaJAc in E. coli LS5218 in the presence of fatty acids could produce P(3HB-co-3HHx) with 0.7 mol% 3HHx content, which was higher than that of the wild-type with only 0.2 mol%. Besides E. coli, C. necator PHB−4 harboring mutant PhaCCn produced P(3HB-co-3HHx) with as high as 1.6 mol% 3HHx compared to the wild type of 1.2 mol%. This study also showed that the molecular weight of PHA produced by mutant PhaCCn was higher than the wild type (Tsuge et al., 2004). In addition, Chuah and co-workers reported that saturation point mutagenesis at position A479 in PhaCCs could enhance substrate specificity for 3HHx. A479 in PhaCCs corresponded to A510 of PhaCCn and was selected as the site of saturation point mutagenesis. It showed that the A479S mutation could increase 3HHx content to 6.6 mol%, 4-fold higher than the wild-type with only 1.6 mol% 3HHx. Besides that, mutation of A479T and A479G showed a 2.8-fold and 1.6-fold increment of 3HHx compared to wild-type PhaCCs (Chuah et al., 2013).
Metabolic engineering of PHA-producing strains
Another factor affecting the 3HHx molar composition is metabolic engineering through the genetic modification of microbial strains. It can involve genes from various pathways, including PHA-related and non-PHA-related genes.
Firstly, the deletion of phaB in the pha operon could affect the 3HHx monomer supply and hence, affect the 3HHx molar composition of the P(3HB-co-3HHx) produced. Budde and co-workers reported that the deletion of phaB from the genome of C. necator could result in the disruption of the 3HB-CoA synthesis pathway (Budde, 2010). Based on Figure 4, PhaB is an important enzyme for supplying (R)-3HB-CoA from acetoacetyl-CoA due to its high catalytic efficiency and expression (Zhang et al., 2019). Budde and co-workers reported that C. necator strains Re2058 and ΔphaB genes mutant, Re2160, were evaluated for their performances through high cell density P(3HB-co-3HHx) production from palm oil. As a result, Re2058 and Re2160 accumulated 17 mol% of 3HHx and 30 mol% of 3HHx, respectively (Budde et al., 2011). Disruption in the 3HB-CoA synthesis pathway leads to lower 3HB-CoA and hence increases the 3HHx molar compositions in the P(3HB-co-3HHx) copolymer produced.
Besides that, modification of the acetoacetyl-CoA reduction step in engineered C. necator could affect the 3HHx molar composition in P(3HB-co-3HHx) from sugars. Zhang and co-workers managed to produce P(3HB-co-3HHx) with 22 mol% of 3HHx content from glucose using C. necator strain NSDG-GG harboring phaJ4a with ΔphaB1 because deletion of phaB1 affected the flux for (R)-3HB-CoA and (R)-3HHx-CoA formation. They introduced had and crt2 into the phaB locus in the pha operon to increase the 3HHx monomer composition without significantly affecting PHA synthesis. Based on Figure 4, acetyl-CoA can be converted to 2-hexenoyl-CoA via fatty acid de novo synthesis pathway. In that pathway, acetoacetyl-CoA will be converted to crotonyl-CoA by Had and Crt2. At this stage, crotonyl-CoA will be hydrated to (R)-3HB-CoA by PhaJ, or crotonyl-CoA will be reduced to butyryl-CoA by Ccr, respectively. Butyryl-CoA will then be converted to 2-hexenoyl-CoA to produce (R)-3HHx-CoA. Zhang and co-workers have further enhanced the fatty acid de novo synthesis pathway by adding crotonyl-CoA carboxylase from Methylorubrum extorquens (CcrMe) and ethylmalonyl-CoA decarboxylase from Mus musculus (EmdMm) (Zhang et al., 2019). Both CcrMe and EmdMm create an alternative pathway towards the formation of butyryl-CoA, which will eventually be converted to (R)-3HHx-CoA and hence, increasing the 3HHx molar composition in the P(3HB-co-3HHx) copolymer produced (Figure 4).
In addition, the deletion of FadB will disrupt (R)-3HB-CoA synthesis, leading to the formation of (R)-3HHx-CoA by PhaJ. Insomphun and co-workers reported that the disruption of fadB1 in engineered C. necator harboring phaJ could enhance the (R)-3HHx-CoA supply from 6-carbons trans-2-enoyl-CoA (2-hexenoyl-CoA) catalyzed by PhaJ and increase the copolymerization of (R)-3HHx-CoA in P(3HB-co-3HHx) copolymer. It was shown that 3HHx monomer composition was increased by around 1–1.5 mol% (Insomphun et al., 2014). Originally, trans-2-enoyl-CoA formed will be converted to (S)-3-hydroxyacyl-CoA or (R)-3-hydroxyacyl-CoA by FadB and PhaJ, respectively. When trans-2-enoyl-CoA is catalyzed by FadB, it is converted to (S)-3-hydroxyacyl-CoA, which will lead to the formation of acyl-CoA and acetyl-CoA, and eventually, the acetyl-CoA formed will be converted to (R)-3HB-CoA. (R)-3HB-CoA will then be incorporated into P(3HB-co-3HHx) as 3HB, hence might lower the 3HHx molar composition in P(3HB-co-3HHx). When FadB is deleted, there will be no formation of (S)-3-hydroxyacyl-CoA, hence favoring the hydration of 2-hexenoyl-CoA to form (R)-3HHx-CoA (Figure 4). This will increase the ratio of (R)-3HHx-CoA to (R)-3HB-CoA, leading to higher 3HHx molar composition.
Besides that, the fatty acid β-oxidation pathway could be modified to increase 3HHx molar composition by inserting 3-ketoacyl-CoA reductase of E. coli (FabGEc). Taguchi and co-workers co-expressed phaCAc and fabGEc in E. coli, and as a result, the 3HHx molar composition of P(3HB-co-3HHx) increased from 0 to 14 mol%. Besides that, they also co-expressed a class II PhaC, PhaC from Pseudomonas sp. 61–3, which is known to have substrate specificity towards MCL-PHA monomer with fabGEc, resulting in an increase of 3HHx from 0 to 12 mol% (Figure 4) (Taguchi et al., 1999). This is probably due to the ability of FabGEc to catalyze the conversion of 6-carbons of 3-ketoacyl-CoA to (R)-3HHx-CoA which will then be incorporated into P(3HB-co-3HHx) by PhaC.
Furthermore, the overexpression of PhaJ using plasmid could enhance 3HHx monomer composition in P(3HB-co-3HHx). Kawashima and co-workers reported that insertion of phaJAc with phaCNSDG in C. necator strain could produce 10.5 mol% of 3HHx, which is 6.6-fold higher than the C. necator strain harboring phaCNSDG only (Kawashima et al., 2015). Tan and co-workers also co-expressed phaJ1 from Pseudomonas aeruginosa with phaCBP-M-CPF4 in C. necator transformants which could produce 3HHx as high as 14 mol%, which is higher than C. necator transformants harboring phaCBP-M-CPF4 with only 6 mol% (Tan et al., 2020). PhaJ is involved in fatty acid β-oxidation, whereby it creates an alternative pathway for supplying (R)-3HHx-CoAs to be incorporated into P(3HB-co-3HHx), hence increasing the 3HHx molar composition. It catalyzes the hydration of (R)-2-hexenoyl-CoA from fatty acid β-oxidation and hydrates them to (R)-3HHx-CoA (Figure 4) (Fukui et al., 1998). This showed that the expression of PhaJ could lead to more (R)-3HHx-CoA production by enhancing the channeling pathway from fatty acid β-oxidation and hence, increasing 3HHx mol% in P(3HB-co-3HHx). Besides that, expression of PhaJ from Streptomyces sp. CFMR7 (PhaJSs) was reported to increase the 3HHx molar composition from 4 mol% and 7 mol% to 12 mol% and 18 mol% using palm oil and crude palm oil, respectively (Tan et al., 2022).
Applications of P(3HB-co-3HHx)
P(3HB-co-3HHx) has been extensively utilized in making straws, shopping bags, cutlery, containers, food packaging, coffee capsules, fishery items, biomedicine and adhesive due to its biodegradability and lack of toxicity (Qiu et al., 2021). Table 3 shows P(3HB-co-3HHx) production strains, carbon sources, the name of the company, the production scale, and their applications.
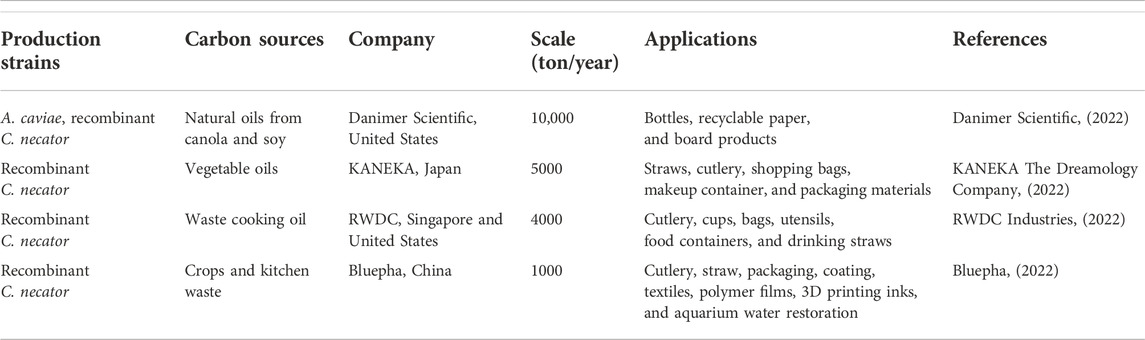
TABLE 3. Commercialized P(3HB-co-3HHx), its production strains, carbon sources, the name of company, production scale, and applications.
KANEKA Corporation has collaborated with Seven-Eleven Japan Corporation and THE NORTH FACE cafes to use straws made of P(3HB-co-3HHx) in the cafe area. Cutlery made from P(3HB-co-3HHx) by KANEKA Corporation is also available at FamilyMart convenience stores to promote the utilization of environmental-friendly materials (KANEKA Corporation, 2021a). Moreover, ITO EN Corporation has used the telescopic straws of “Oi Ocha Tea,” made of P(3HB-co-3HHx) and sold in Japan’s supermarkets (KANEKA Corporation, 2021b). Furthermore, JALUX corporation adopted shopping bags made of P(3HB-co-3HHx) in the BLUE SKY stores at Japan’s airports (KANEKA Corporation, 2021c).
Besides that, KANEKA has collaborated with Shiseido Company to use P(3HB-co-3HHx) copolymer as cosmetics packaging material. For example, the case of AquaGel Lip Palette is made of biodegradable P(3HB-co-3HHx), and this product has been sold since the 2020s (KANEKA Corporation, 2020). P(3HB-co-3HHx) is also applied in compostable capsules. Capsul’ in Pro, a manufacturer, has launched a Zero Impact capsule where the capsule is made of 100% biobased P(3HB-co-3HHx) derived from plant oils (Magazine, 2021). It provides an oxygen barrier to protect the flavor and aroma of coffee for 12 months. However, this capsule is still under development and has not been published.
Due to its biocompatibility and bioresorbable, the P(3HB-co-3HHx) copolymer is also suitable to be used as a scaffold for tissue engineering (Chang et al., 2014). It can be biocompatible with various cell types: smooth muscle cells, fibroblasts, osteoblasts and bone marrow cells (Qu et al., 2006a; Yang et al., 2011; Yu et al., 2012). To test in vitro biocompatibilities, rabbit bone marrow cells were injected into P(3HB-co-3HHx) 3D scaffolds, and it was discovered that P(3HB-co-3HHx) performed best in terms of bone marrow cell attachment and proliferation (Chen and Wu, 2005). Scaffolds provide physical support and an artificial extracellular matrix (ECM) that promotes cell adhesion, proliferation and differentiation (Bryant and Anseth, 2003). Zhao and co-workers reported that P(3HB-co-3HHx) composite scaffolds produced via the 3D printing technique could improve their bioactive and osteogenic characteristics by enhancing the regeneration of bone in the defected calvarium of rats (Zhao et al., 2014). Ang and co-workers reported that blending of P(3HB-co-3HHx) with silk fibroin (SF) could improve the proliferation and osteogenic differentiation of human umbilical cord-derived mesenchymal stem cells (hUC-MSCs), which is a potential biomaterial for bone tissue engineering (Ang et al., 2020). Besides that, due to its elasticity, the P(3HB-co-3HHx) biomaterial could resist systemic pressure and support cell growth, making it a potential tissue-engineered blood vessel (Qu et al., 2006b). It is a potential biomaterial to replace synthetic blood vessels during surgeries and reduce the risk of infection.
In addition, P(3HB-co-3HHx) can be utilized as a drug delivery system in cancer treatment due to its biodegradability and nontoxicity. A combination of P(3HB-co-3HHx) with folic acid and loaded with etoposide could improve the medication delivery to tumors (Chang et al., 2014). Another application is P(3HB-co-3HHx) nanoparticles filled with an insulin phospholipid complex to make a form of insulin that has a long-acting release to treat diabetes. Peng and co-workers have made a thermosensitive hydrogel filled with P(3HB-co-3HHx) nanoparticles that can be injected and broken down through biological processes (Peng et al., 2013). This allows insulin to be released slowly and steadily. Due to the long-term basal insulin release by this combination of nanoparticle and hydrogel, which might reduce the frequency of injections of patients, this may assist not just the elderly with diabetes but also patients from other age groups.
Conclusion
In conclusion, P(3HB-co-3HHx) copolymer is a type of PHA capable of replacing petroleum-based plastics in different applications like single-used plastics, medical devices, and packaging due to its similar properties and biodegradability. It has a superior biodegradation rate compared to petroleum-based plastics, hence leaving no or little pollution. P(3HB-co-3HHx) can be produced by PHA-producing microorganisms through various pathways like the PHA biosynthesis pathway involving phaA, phaB, and phaC, β-oxidation, and fatty acids de novo synthesis dependent on the carbon source fed during cultivation. However, the properties of P(3HB-co-3HHx) are dependent on its 3HHx molar composition. Hence, it is necessary to control the 3HHx molar composition of the P(3HB-co-3HHx) produced by using different PhaCs, engineering of PhaCs, and metabolic engineering of bacteria for P(3HB-co-3HHx) to be applied. With more knowledge and information regarding PhaCs and the metabolic pathway on PHA biosynthesis, P(3HB-co-3HHx) with the desired 3HHx molar composition can be produced, making them more suitable for their respective applications.
Author contributions
HJT drafted, wrote, reviewed the manuscript, and designed the figures. SZN wrote and reviewed the manuscript. KS reviewed and approved the final manuscript.
Funding
This study was funded by Ministry of Higher Education, titled “Soil Analysis and Value-Addition to Oil Palm Trunk (OPT) and sap through Biotechnology” (203/PBIOLOGI/67811001 to KS) as well as Science and Technology Research Partnership for Sustainable Development (SATREPS).
Acknowledgments
HJT acknowledges Graduate student financial assistant (GRA-Assist) awarded by Universiti Sains Malaysia (USM).
Conflict of interest
The authors declare that the research was conducted in the absence of any commercial or financial relationships that could be construed as a potential conflict of interest.
Publisher’s note
All claims expressed in this article are solely those of the authors and do not necessarily represent those of their affiliated organizations, or those of the publisher, the editors and the reviewers. Any product that may be evaluated in this article, or claim that may be made by its manufacturer, is not guaranteed or endorsed by the publisher.
References
Abe, H., and Doi, Y. (1999). Structural effects on enzymatic degradabilities for poly[(R)-3-hydroxybutyric acid] and its copolymers. Int. J. Biol. Macromol. 25, 185–192. doi:10.1016/s0141-8130(99)00033-1
Alabi, O. A., Ologbonjaye, K. I., Awosolu, O., and Alalade, O. E. (2019). Public and environmental health effects of plastic wastes disposal: A review. J. Toxicol. Risk Assess. 5, 1–13. doi:10.23937/2572-4061.1510021
Anderson, A. J., and Dawes, E. A. (1990). Occurrence, metabolism, metabolic role, and industrial uses of bacterial polyhydroxyalkanoates. Microbiol. Rev. 54, 450–472. doi:10.1128/mr.54.4.450-472.1990
Ang, S. L., Sivashankari, R., Shaharuddin, B., Chuah, J. A., Tsuge, T., Abe, H., et al. (2020). Potential applications of polyhydroxyalkanoates as a biomaterial for the aging population. Polym. Degrad. Stab. 181, 109371. doi:10.1016/j.polymdegradstab.2020.109371
Asrar, J., Valentin, H. E., Berger, P. A., Tran, M., Padgette, S. R., and Garbow, J. R. (2002). Biosynthesis and properties of poly(3-hydroxybutyrate-co-3-hydroxyhexanoate) polymers. Biomacromolecules 3, 1006–1012. doi:10.1021/bm025543a
Bajt, O. (2021). From plastics to microplastics and organisms. FEBS Open Bio 11, 954–966. doi:10.1002/2211-5463.13120
Bhubalan, K., Kam, Y. C., Yong, K. H., and Sudesh, K. (2010). Cloning and expression of the PHA synthase gene from a locally isolated Chromobacterium sp. Malays. J. Microbiol. 6, 81–90. doi:10.21161/mjm.21809
Bluepha (2022). Bluepha® for the blue planet. Available at: https://www.bluepha.bio/(Accessed October 14, 2022).
Bryant, S. J., and Anseth, K. S. (2003). Controlling the spatial distribution of ECM components in degradable PEG hydrogels for tissue engineering cartilage. J. Biomed. Mat. Res. 64, 70–79. doi:10.1002/jbm.a.10319
Budde, C. F. (2010). Production of polyhydroxyalkanoate copolymers from plant oil. Cambridge (MA): Massachusetts Institute of Technology. [Doctoral dissertation].
Budde, C. F., Riedel, S. L., Willis, L. B., Rha, C., and Sinskey, A. J. (2011). Production of poly(3-hydroxybutyrate-co-3-hydroxyhexanoate) from plant oil by engineered Ralstonia eutropha strains. Appl. Environ. Microbiol. 77, 2847–2854. doi:10.1128/AEM.02429-10
Chang, H. M., Wang, Z. H., Luo, H. N., Xu, M., Ren, X. Y., Zheng, G. X., et al. (2014). Poly(3-hydroxybutyrate-co-3-hydroxyhexanoate)-based scaffolds for tissue engineering. Braz. J. Med. Biol. Res. 47, 533–539. doi:10.1590/1414-431X20143930
Chek, M. F., Kim, S. Y., Mori, T., Tan, H. T., Sudesh, K., and Hakoshima, T. (2020). Asymmetric open-closed dimer mechanism of polyhydroxyalkanoate synthase PhaC. Iscience 23, 101084. doi:10.1016/j.isci.2020.101084
Chen, G. Q., and Wu, Q. (2005). The application of polyhydroxyalkanoates as tissue engineering materials. Biomaterials 26, 6565–6578. doi:10.1016/j.biomaterials.2005.04.036
Chuah, J. A., Tomizawa, S., Yamada, M., Tsuge, T., Doi, Y., Sudesh, K., et al. (2013). Characterization of site-specific mutations in a short-chain-length/medium-chain-length polyhydroxyalkanoate synthase: In vivo and in vitro studies of enzymatic activity and substrate specificity. Appl. Environ. Microbiol. 79, 3813–3821. doi:10.1128/AEM.00564-13
Danimer Scientific (2022). The future of plastics is here. Available at: https://danimerscientific.com/(Accessed October 14, 2022).
Doi, Y., Kitamura, S., and Abe, H. (1995). Microbial synthesis and characterization of poly(3-hydroxybutyrate-co-3-hydroxyhexanoate). Macromolecules 28, 4822–4828. doi:10.1021/ma00118a007
Foong, C. P., Lakshmanan, M., Abe, H., Taylor, T. D., Foong, S. Y., and Sudesh, K. (2018). A novel and wide substrate specific polyhydroxyalkanoate (PHA) synthase from unculturable bacteria found in mangrove soil. J. Polym. Res. 25, 23. doi:10.1007/s10965-017-1403-4
Fukui, T., Abe, H., and Doi, Y. (2002). Engineering of Ralstonia eutropha for production of poly(3-hydroxybutyrate-co-3-hydroxyhexanoate) from fructose and solid-state properties of the copolymer. Biomacromolecules 3, 618–624. doi:10.1021/bm0255084
Fukui, T., and Doi, Y. (1997). Cloning and analysis of the poly (3-hydroxybutyrate-co-3-hydroxyhexanoate) biosynthesis genes of Aeromonas caviae. J. Bacteriol. 179, 4821–4830. doi:10.1128/jb.179.15.4821-4830.1997
Fukui, T., and Doi, Y. (1998). Efficient production of polyhydroxyalkanoates from plant oils by Alcaligenes eutrophus and its recombinant strain. Appl. Microbiol. Biotechnol. 49, 333–336. doi:10.1007/s002530051178
Fukui, T., Shiomi, N., and Doi, Y. (1998). Expression and characterization of (R)-specific enoyl coenzyme A hydratase involved in polyhydroxyalkanoate biosynthesis by Aeromonas caviae. J. Bacteriol. 180, 667–673. doi:10.1128/jb.180.3.667-673.1998
Holmes, P. (1988). “Biologically produced (R)-3-hydroxy-alkanoate polymers and copolymers,” in Developments in crystalline polymers. Editor C. D. Basset (New York: Springer), 1.
Insomphun, C., Mifune, J., Orita, I., Numata, K., Nakamura, S., and Fukui, T. (2014). Modification of β-oxidation pathway in Ralstonia eutropha for production of poly(3-hydroxybutyrate-co-3-hydroxyhexanoate) from soybean oil. J. Biosci. Bioeng. 117, 184–190. doi:10.1016/j.jbiosc.2013.07.016
Iwata, T. (2005). Strong fibers and films of microbial polyesters. Macromol. Biosci. 5, 689–701. doi:10.1002/mabi.200500066
Kahar, P., Tsuge, T., Taguchi, K., and Doi, Y. (2004). High yield production of polyhydroxyalkanoates from soybean oil by Ralstonia eutropha and its recombinant strain. Polym. Degrad. Stab. 83, 79–86. doi:10.1016/S0141-3910(03)00227-1
KANEKA Corporation (2021a). KANEKA Biodegradable Polymer Green PlanetTM is adopted in shopping bags for JALUX. Available at: https://www.kaneka.co.jp/en/topics/news/2021/ennr2106141.html (Accessed June 21, 2022).
KANEKA Corporation (2020). KANEKA Biodegradable Polymer PHBHTM used in Shiseido’s cosmetics packages. Available at: https://www.kaneka.co.jp/en/topics/news/2020/ennr2008061.html (Accessed June 21, 2022).
KANEKA Corporation (2021b). Spoons made from KANEKA biodegradable polymer green PlanetTM are adopted in FamilyMart. Available at: https://www.kaneka.co.jp/en/topics/news/2021/ennr2106221.html (Accessed June 21, 2022).
KANEKA Corporation (2019). Target area expanded for seven café straws made from KANEKA biodegradable polymer PHBHTM. Available at: https://www.kaneka.co.jp/en/topics/information/in20191031/(Accessed June 21, 2022).
KANEKA Corporation (2021c). Use of KANEKA Biodegradable Polymer Green PlanetTM expands to ITO EN’s tea beverages following vegetable juices. Available at: https://www.kaneka.co.jp/en/topics/news/2021/ennr2109211.html (Accessed June 21, 2022).
KANEKA The Dreamology Company (2022). Kaneka. Available at: https://www.kaneka.be/(Accessed October 14, 2022).
Kawashima, Y., Orita, I., Nakamura, S., and Fukui, T. (2015). Compositional regulation of poly(3-hydroxybutyrate-co-3-hydroxyhexanoate) by replacement of granule-associated protein in Ralstonia eutropha. Microb. Cell. Fact. 14, 187–212. doi:10.1186/s12934-015-0380-8
Kellerhals, M. B., Kessler, B., Witholt, B., Tchouboukov, A., and Brandl, H. (2000). Renewable long-chain fatty acids for production of biodegradable medium-chain-length polyhydroxyalkanoates (mcl-PHAs) at laboratory and pilot plant scales. Macromolecules 33, 4690–4698. doi:10.1021/ma000655k
Kichise, T., Taguchi, S., and Doi, Y. (2002). Enhanced accumulation and changed monomer composition in polyhydroxyalkanoate (PHA) copolyester by in vitro evolution of Aeromonas caviae PHA synthase. Appl. Environ. Microbiol. 68, 2411–2419. doi:10.1128/AEM.68.5.2411-2419.2002
Lee, S. Y., and Choi, J. I. (1999). Production and degradation of polyhydroxyalkanoates in waste environment. Waste Manag. 19, 133–139. doi:10.1016/S0956-053X(99)00005-7
Lemoigne, M. (1926). Products of dehydration and of polymerization of β-hydroxybutyric acid. Bull. Soc. Chim. Biol. 8, 770
Loo, C., and Sudesh, K. (2007). Polyhydroxyalkanoates: Bio-based microbial plastics and their properties. Malays. Polym. J. 2, 31–57.
Loo, C. Y., Lee, W. H., Tsuge, T., Doi, Y., and Sudesh, K. (2005). Biosynthesis and characterization of poly(3-hydroxybutyrate-co-3-hydroxyhexanoate) from palm oil products in a Wautersia eutropha mutant. Biotechnol. Lett. 27, 1405–1410. doi:10.1007/s10529-005-0690-8
Magazine, B. (2021). Capsul’in Pro launches world’s first certified home compostable coffee capsule. Available at: https://www.bioplasticsmagazine.com/en/news/meldungen/20210310-Capsul-in-Pro-launches-world-s-first-certified-home-compostable-coffee-capsule.php (Accessed May 30, 2022).
Masry, M., Rossignol, S., Gardette, J. L., Therias, S., Bussière, P. O., and Wong-Wah-Chung, P. (2021). Characteristics, fate, and impact of marine plastic debris exposed to sunlight: A review. Mar. Pollut. Bull. 171, 112701. doi:10.1016/j.marpolbul.2021.112701
Matsusaki, H., Manji, S., Taguchi, K., Kato, M., Fukui, T., and Doi, Y. (1998). Cloning and molecular analysis of the poly(3-hydroxybutyrate) and poly(3-hydroxybutyrate-co-3-hydroxyalkanoate) biosynthesis genes in Pseudomonas sp. strain 61-3. J. Bacteriol. 180, 6459–6467. doi:10.1128/JB.180.24.6459-6467.1998
Molitoris, H. P., Moss, S. T., Koning, D., and Jendrossek, D. (1996). Scanning electron microscopy of polyhydroxyalkanoate degradation by bacteria. Appl. Microbiol. Biotechnol. 46, 570–579. doi:10.1007/s002530050863
Morse, M. C., Liao, Q., Criddle, C. S., and Frank, C. W. (2011). Anaerobic biodegradation of the microbial copolymer poly(3-hydroxybutyrate-co-3-hydroxyhexanoate): Effects of comonomer content, processing history, and semi-crystalline morphology. Polymer 52, 547–556. doi:10.1016/j.polymer.2010.11.024
Murugan, P., Chhajer, P., Kosugi, A., Arai, T., Brigham, C. J., and Sudesh, K. (2016). Production of P(3HB-co-3HHx) with controlled compositions by recombinant Cupriavidus necator Re2058/pCB113 from renewable resources. Clean. Soil Air Water 44, 1234–1241. doi:10.1002/clen.201500714
Murugan, P., Gan, C. Y., and Sudesh, K. (2017). Biosynthesis of P(3HB-co-3HHx) with improved molecular weights from a mixture of palm olein and fructose by Cupriavidus necator Re2018/pCB113. Int. J. Biol. Macromol. 102, 1112–1119. doi:10.1016/j.ijbiomac.2017.05.006
Neoh, S. Z., Chek, M. F., Tan, H. T., Linares-Pastén, J. A., Nandakumar, A., Hakoshima, T., et al. (2022). Polyhydroxyalkanoate synthase (PhaC): The key enzyme for biopolyester synthesis. Curr. Res. Biotechnol. 4, 87–101. doi:10.1016/j.crbiot.2022.01.002
Noda, I., Green, P. R., Satkowski, M. M., and Schechtman, L. A. (2005). Preparation and properties of a novel class of polyhydroxyalkanoate copolymers. Biomacromolecules 6, 580–586. doi:10.1021/bm049472m
Peng, Q., Sun, X., Gong, T., Wu, C. Y., Zhang, T., Tan, J., et al. (2013). Injectable and biodegradable thermosensitive hydrogels loaded with PHBHHx nanoparticles for the sustained and controlled release of insulin. Acta Biomater. 9, 5063–5069. doi:10.1016/j.actbio.2012.09.034
Purama, R. K., Al-Sabahi, J. N., and Sudesh, K. (2018). Evaluation of date seed oil and date molasses as novel carbon sources for the production of poly(3Hydroxybutyrate-co-3Hydroxyhexanoate) by Cupriavidus necator H16 Re2058/pCB113. Industrial Crops Prod. 119, 83–92. doi:10.1016/j.indcrop.2018.04.013
Qiu, Y., Fu, J., Sun, B., and Ma, X. (2021). Sustainable nanocomposite films based on SiO2 and biodegradable poly(3-hydroxybutyrate-co-3-hydroxyhexanoate) (PHBH) for food packaging. e-Polymers 21, 072–081. doi:10.1515/epoly-2021-0009
Qu, X. H., Wu, Q., Liang, J., Zou, B., and Chen, G. Q. (2006a). Effect of 3-hydroxyhexanoate content in poly(3-hydroxybutyrate-co-3-hydroxyhexanoate) on in vitro growth and differentiation of smooth muscle cells. Biomaterials 27, 2944–2950. doi:10.1016/j.biomaterials.2006.01.013
Qu, X. H., Wu, Q., Zhang, K. Y., and Chen, G. Q. (2006b). In vivo studies of poly(3-hydroxybutyrate-co-3-hydroxyhexanoate) based polymers: Biodegradation and tissue reactions. Biomaterials 27, 3540–3548. doi:10.1016/j.biomaterials.2006.02.015
Reddy, V. U. N., Ramanaiah, S. V., Reddy, M. V., and Chang, Y. C. (2022). Review of the developments of bacterial medium-chain-length polyhydroxyalkanoates (mcl-PHAs). Bioengineering 9, 225. doi:10.3390/bioengineering9050225
Rehm, B. H. (2003). Polyester synthases: Natural catalysts for plastics. Biochem. J. 376, 15–33. doi:10.1042/BJ20031254
Riedel, S. L., Bader, J., Brigham, C. J., Budde, C. F., Yusof, Z. A. M., Rha, C., et al. (2012). Production of poly(3-hydroxybutyrate-co-3-hydroxyhexanoate) by Ralstonia eutropha in high cell density palm oil fermentations. Biotechnol. Bioeng. 109, 74–83. doi:10.1002/bit.23283
RWDC Industries (2022). We believe it is our duty as stewards of the planet to use our talents, energy and time to leave a legacy of a sustainable future. Available at: https://www.rwdc-industries.com/(Accessed October 14, 2022).
Shimamura, E., Kasuya, K., Kobayashi, G., Shiotani, T., Shima, Y., and Doi, Y. (1994). Physical properties and biodegradability of microbial poly(3-hydroxybutyrate-co-3-hydroxyhexanoate). Macromolecules 27, 878–880. doi:10.1021/ma00081a041
Sudesh, K., Abe, H., and Doi, Y. (2000). Synthesis, structure and properties of polyhydroxyalkanoates: Biological polyesters. Prog. Polym. Sci. 25, 1503–1555. doi:10.1016/S0079-6700(00)00035-6
Sudesh, K., and Iwata, T. (2008). Sustainability of biobased and biodegradable plastics. Clean. Soil Air Water 36, 433–442. doi:10.1002/clen.200700183
Sudesh, K. (2012). Polyhydroxyalkanoates from palm oil: Biodegradable plastics. Berlin Heidelberg: Springer-Verlag.
Taguchi, K., Aoyagi, Y., Matsusaki, H., Fukui, T., and Doi, Y. (1999). Co-expression of 3-ketoacyl-ACP reductase and polyhydroxyalkanoate synthase genes induces PHA production in Escherichia coli HB101 strain. FEMS Microbiol. Lett. 176, 183–190. doi:10.1111/j.1574-6968.1999.tb13660.x
Tan, H. T., Chek, M. F., Lakshmanan, M., Foong, C. P., Hakoshima, T., and Sudesh, K. (2020). Evaluation of BP-M-CPF4 polyhydroxyalkanoate (PHA) synthase on the production of poly(3-hydroxybutyrate-co-3-hydroxyhexanoate) from plant oil using Cupriavidus necator transformants. Int. J. Biol. Macromol. 159, 250–257. doi:10.1016/j.ijbiomac.2020.05.064
Tan, H. T., Chek, M. F., Miyahara, Y., Kim, S. Y., Tsuge, T., Hakoshima, T., et al. (2022). Characterization of an (R)-specific enoyl-CoA hydratase from Streptomyces sp. strain CFMR 7: A metabolic tool for enhancing the production of poly(3-hydroxybutyrate-co-3-hydroxyhexanoate). J. Biosci. Bioeng. S1389-1723 (22), 288–294. doi:10.1016/j.jbiosc.2022.07.005
Thinagaran, L., and Sudesh, K. (2019). Evaluation of sludge palm oil as feedstock and development of efficient method for its utilization to produce polyhydroxyalkanoate. Waste Biomass Valor. 10, 709–720. doi:10.1007/s12649-017-0078-8
Tsuge, T. (2002). Metabolic improvements and use of inexpensive carbon sources in microbial production of polyhydroxyalkanoates. J. Biosci. Bioeng. 94, 579–584. doi:10.1016/s1389-1723(02)80198-0
Tsuge, T., Saito, Y., Narike, M., Muneta, K., Normi, Y. M., Kikkawa, Y., et al. (2004). Mutation effects of a conserved alanine (Ala510) in Type I polyhydroxyalkanoate synthase from Ralstonia eutropha on polyester biosynthesis. Macromol. Biosci. 4, 963–970. doi:10.1002/mabi.200400075
Tsuge, T., Watanabe, S., Shimada, D., Abe, H., Doi, Y., and Taguchi, S. (2007). Combination of N149S and D171G mutations in Aeromonas caviae polyhydroxyalkanoate synthase and impact on polyhydroxyalkanoate biosynthesis. FEMS Microbiol. Lett. 277, 217–222. doi:10.1111/j.1574-6968.2007.00958.x
Volova, T. G., Prudnikova, S. V., Vinogradova, O. N., Syrvacheva, D. A., and Shishatskaya, E. I. (2017). Microbial degradation of polyhydroxyalkanoates with different chemical compositions and their biodegradability. Microb. Ecol. 73, 353–367. doi:10.1007/s00248-016-0852-3
Volova, T. G., Syrvacheva, D. A., Zhila, N. O., and Sukovatiy, A. G. (2016). Synthesis of P(3HB-co-3HHx) copolymers containing high molar fraction of 3-hydroxyhexanoate monomer by Cupriavidus eutrophus B10646. J. Chem. Technol. Biotechnol. 91, 416–425. doi:10.1002/jctb.4592
Wang, Y. W., Mo, W., Yao, H., Wu, Q., Chen, J., and Chen, G. Q. (2004). Biodegradation studies of poly(3-hydroxybutyrate-co-3-hydroxyhexanoate). Polym. Degrad. Stab. 85, 815–821. doi:10.1016/j.polymdegradstab.2004.02.010
Wong, Y. M., Brigham, C. J., Rha, C., Sinskey, A. J., and Sudesh, K. (2012). Biosynthesis and characterization of polyhydroxyalkanoate containing high 3-hydroxyhexanoate monomer fraction from crude palm kernel oil by recombinant Cupriavidus necator. Bioresour. Technol. 121, 320–327. doi:10.1016/j.biortech.2012.07.015
Yang, H. X., Sun, M., Zhang, Y., and Zhou, P. (2011). Degradable PHBHHx modified by the silk fibroin for the applications of cardiovascular tissue engineering. ISRN Mater. Sci. 2011, 1–11. doi:10.5402/2011/389872
Yong, K. H. (2009). Biosynthesis and characterization of polyhydroxyalkanoates by a locally isolated Chromobacterium sp. USM2. Georgetown: Universiti Sains Malaysia. [MSc. thesis].
Yu, B. Y., Chen, P. Y., Sun, Y. M., Lee, Y. T., and Young, T. H. (2012). Response of human mesenchymal stem cells (hMSCs) to the topographic variation of poly(3-hydroxybutyrate-co-3-hydroxyhexanoate) (PHBHHx) films. J. Biomaterials Sci. Polym. Ed. 23, 1–26. doi:10.1163/092050610X541386
Yu, J. (2007). “Microbial production of bioplastics from renewable resources,” in Bioprocessing for value-added products from renewable resources: New Technologies and Applications. Editor S. T. Yang (Amsterdam: Elsevier), 585
Zhang, M., Kurita, S., Orita, I., Nakamura, S., and Fukui, T. (2019). Modification of acetoacetyl-CoA reduction step in Ralstonia eutropha for biosynthesis of poly(3-hydroxybutyrate-co-3-hydroxyhexanoate) from structurally unrelated compounds. Microb. Cell. Fact. 18, 147–212. doi:10.1186/s12934-019-1197-7
Keywords: polyhydroxyalkanoate, poly(3-hydroxybutyrate-co-3-hydroxyhexanoate) [P(3HB-co-3HHx)], metabolic pathway, PHA synthase, engineering of PhaCs
Citation: Tang HJ, Neoh SZ and Sudesh K (2022) A review on poly(3-hydroxybutyrate-co-3-hydroxyhexanoate) [P(3HB-co-3HHx)] and genetic modifications that affect its production. Front. Bioeng. Biotechnol. 10:1057067. doi: 10.3389/fbioe.2022.1057067
Received: 29 September 2022; Accepted: 16 November 2022;
Published: 05 December 2022.
Edited by:
Leilei Zhu, Tianjin Institute of Industrial Biotechnology (CAS), ChinaReviewed by:
George Guo-Qiang Chen, Tsinghua University, ChinaVenkateswer Reddy Motakatla, Colorado State University, United States
Copyright © 2022 Tang, Neoh and Sudesh. This is an open-access article distributed under the terms of the Creative Commons Attribution License (CC BY). The use, distribution or reproduction in other forums is permitted, provided the original author(s) and the copyright owner(s) are credited and that the original publication in this journal is cited, in accordance with accepted academic practice. No use, distribution or reproduction is permitted which does not comply with these terms.
*Correspondence: Kumar Sudesh, a3N1ZGVzaEB1c20ubXk=