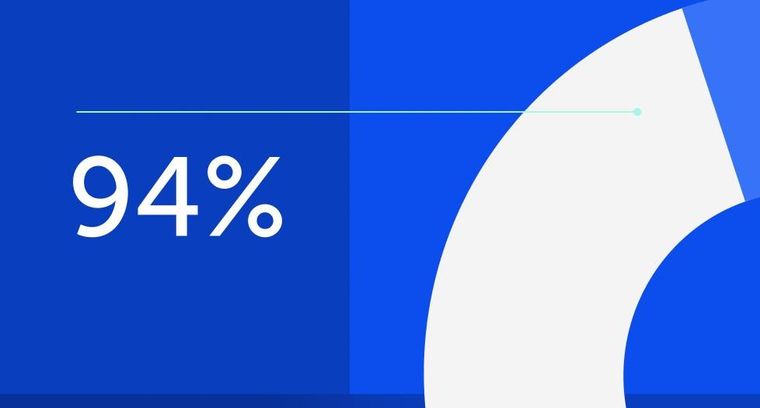
94% of researchers rate our articles as excellent or good
Learn more about the work of our research integrity team to safeguard the quality of each article we publish.
Find out more
REVIEW article
Front. Bioeng. Biotechnol., 25 November 2022
Sec. Biomaterials
Volume 10 - 2022 | https://doi.org/10.3389/fbioe.2022.1054379
This article is part of the Research TopicPolymeric Microarchitectures for Tissue Regeneration and Drug ScreeningView all 12 articles
It remains a big challenge in clinical practice to repair large-sized bone defects and many factors limit the application of autografts and allografts, The application of exogenous scaffolds is an alternate strategy for bone regeneration, among which the silk fibroin (SF) scaffold is a promising candidate. Due to the advantages of excellent biocompatibility, satisfying mechanical property, controllable biodegradability and structural adjustability, SF scaffolds exhibit great potential in bone regeneration with the help of well-designed structures, bioactive components and functional surface modification. This review will summarize the cell and tissue interaction with SF scaffolds, techniques to fabricate SF-based scaffolds and modifications of SF scaffolds to enhance osteogenesis, which will provide a deep and comprehensive insight into SF scaffolds and inspire the design and fabrication of novel SF scaffolds for superior osteogenic performance. However, there still needs more comprehensive efforts to promote better clinical translation of SF scaffolds, including more experiments in big animal models and clinical trials. Furthermore, deeper investigations are also in demand to reveal the degradation and clearing mechanisms of SF scaffolds and evaluate the influence of degradation products.
Caused by trauma, tumor and other pathological factors, bone defects can lead to dysfunctions and destruction of the musculoskeletal system (Burger et al., 2022). It remains challenging to achieve ideal bone regeneration in clinical practice (Lee et al., 2022; Mirkhalaf et al., 2022). Autografts and allografts are commonly applied to repair defected bones, but many factors limited their applications (Kundu et al., 2013; Guo et al., 2021). The application of exogenous scaffolds as bone substitutes seems an alternate strategy. The ideal scaffolds for bone regeneration should provide a biomimicking microenvironment to promote desirable cellular responses, which possess excellent biocompatibility, optimized mechanical properties, desirable morphology and structure. The desired biodegradability with safe by-products and controllable diffusion is also important (Zhang and King, 2020).
Among plenty scaffolds, silk fibroin (SF) is a promising candidate with numerous researches (Wenhao et al., 2020; Shen et al., 2022). In the structure of SF, the light (L) chains, heavy (H) chains and the hydrophobically linked glycoprotein P25 are crosslinked to form an H-L complex with anti-parallel beta-sheets (β-sheets) (Figure 1) (Wongpinyochit et al., 2018; Zuluaga-Velez et al., 2021). SF scaffolds have many advantages, including excellent biocompatibility, satisfying mechanical property, controllable biodegradability and structural adjustability (Zhang et al., 2021; Zuluaga-Velez et al., 2021; Li and Sun, 2022). Compared with biodegradable synthetic polymers such as poly (lactic acid) (PLA), SF scaffolds exhibit better biocompatibility and cell adhesion performance (Wenk et al., 2011). Besides, due to the formation of ß-sheets, SF scaffolds have better mechanical properties than collagens and chitosan (CS), with ultimate tensile strength of 300–740 MPa (Koh et al., 2015; Wang et al., 2020).
FIGURE 1. Illustration of SF structure including the heavy chain and light chain. (Wongpinyochit et al., 2018). Copyright 2018 American Chemical Society.
However, compared with native bone tissue, the mechanical properties of pure SF scaffolds are still insufficient (Melke et al., 2016; Soundarya et al., 2018). Further improvement is in demand to achieve better osteogenic capacity (Wu et al., 2021). Due to outstanding tunability, SF can be modified into different formats for certain applications, such as films, hydrogels and porous structures (Bakhshandeh et al., 2021). Besides, different organic and inorganic components can be mixed with pure SF to fabricate hybrid scaffolds to improve mechanical and biological performance (Wu et al., 2021). Another important modification method is surface modification, including physical modification, chemical modification and surface functionalization by bioactive components, which can make as-prepared SF scaffolds more bioactive (Hardy et al., 2018; Wang et al., 2019; Moses and Mandal, 2022).
Despite the large number of related researches, there still lack deep investigations on the long-term in vivo safety of SF scaffolds, which is closely related to the degradation products. The studies on degradation and cleaning mechanism of SF scaffolds are also far from satisfactory. It can be helpful to deeply understand the in vivo interaction of SF scaffolds with hosts’ tissues to better inspire further modifications. Moreover, among numerous fabrication techniques and modification methods, it can be difficult to make a suitable choice for different applications, where a comprehensive classification and summary can be helpful.
Herein, we summarize the cell and tissue interaction with SF scaffolds, the fabrication techniques and modifications for better bone regeneration (Figure 2). We hope to give a deep insight into the SF scaffolds applied in bone regeneration and inspire research enthusiasm for better development and improvement of SF scaffolds.
FIGURE 2. Graphical diagram for fabrication of SF scaffolds and their cell and tissue responses, which makes contributions to bone regeneration. (Created with BioRender.com).
As a highly dynamic vascularized tissue, bone tissue consists of cellular components, extracellular matrix (ECM) and minerals. Through achieving a deeper understanding of SF’s interaction with cellular and non-cellular components, the design of advanced SF-based scaffolds can be better inspired.
Silk materials have been proved biocompatible with long history of application, but some rarely-happened adverse immunological events cannot be ruled out, which may be caused by the presence of silk sericin (SS) proteins. Wang et al. (Wang et al., 2020) combined SF and SS in different mass ratios and detected the immune responses caused by different scaffolds. The results showed that the macrophages were activated by the addition of SS and secreted more proinflammation (M1)-related cytokines. The SF itself showed satisfying biocompatibility with higher antiinflammation (M2) phenotype ratio of macrophages. As for in vivo evaluation, Gorenkova et al. (Gorenkova et al., 2021) evaluated the immune response of self-assembled SF hydrogels via Balb/c mice models. The inflammatory response was comparable or even lower than the benchmark material, polyethylene glycol (PEG), which did not activate tissue regeneration and served as the baseline marker for tissue responses. Overall, these studies demonstrate that the SF scaffolds can exhibit favorable biocompatibility after degumming and sterilization.
Despite these encouraging researches, there still exist problems about long term in vivo safety of SF scaffolds and long-term immune responses need further investigation to achieve better understanding. The immune response to degradation products of SF scaffolds should also be considered, where the production of proinflammatory cytokine and phagocytosis may be induced by fractions of SF fibers (Gellynck et al., 2008). Lundmark et al. (Lundmark et al., 2005) found that the degradation products of SF could cause amyloidogenesis and tissue degeneration. Therefore, it is necessary to carry out long-term studies of SF scaffolds on their degradation products.
Meinel et al. (2005) claimed that the plain silk scaffolds could only provide an appropriate environment for cellular proliferation, where the ingrown cells showed poor osteogenic differentiation. However, later researches demonstrated that SF could activate expression of osteogenesis-related genes, including alkaline phosphatase (ALP), Runt-related transcription factor 2 (Runx2), collagen I (COL I), osterix, osteocalcin (OCN) and CD29/CD44 (Miyamoto et al., 2013; Panda et al., 2015). Jung et al. (2013) found the SF could serve as the suppressor of the Notch pathway, which could down-regulate of osteogenesis and thus promote osteogenesis. The amide groups and high ß-sheet contents of SF could also induce osteogenic differentiation, where the ß-sheet structure could provide a stiff matrix environment for osteoblasts.
However, considering results of other in vivo experiments, plain SF scaffolds have insufficient ability to completely regenerate large bone defects (Mottaghitalab et al., 2015). Uebersax et al. (2013) implanted two different SF scaffolds into the long bones of sheep and found the poor bone formation in both scaffolds. Song et al. (2011) applied SF film to repair rabbit calvarial defects (φ 8 mm), and found the calvarial defects actually failed to completely recover after 8 weeks. Notably, when pre-seeded with undifferentiated stem cells before implanted, the capability of bone formation can be significantly improved with pre-differentiated cells (Park et al., 2015; Sartika et al., 2020). Considering the long-term procedure of autologous cell isolation and culture, the cell-free SF scaffold is still a better choice.
During bone regeneration, it is important to take bone homeostasis into consideration, which is maintained by the balance of dynamic bone formation and resorption. Osteoblasts originate from multipotent mesenchymal stem cells (MSCs) and secrete the osteoid matrix. Osteoclasts arise from the mononuclear cell lineage and are responsible for bone matrix resorption (Borciani et al., 2020).
However, so far, only a few literatures are focused on interaction between SF scaffolds and osteoclasts. Jones et al. (Jones et al., 2009) cultured murine osteoblasts and osteoclasts on SF scaffolds. When monocytes were cultured separately, cells aggregated together and the expression of tartrate resistant acid phosphatase (TRAP) was positive, as the osteoclast marker. However, when co-cultured with osteoblasts, individual TRAP positive cells spread evenly amongst osteoblasts, forming a homogeneous layer. Furthermore, Chon et al. (Chon et al., 2012) reported that SF hydrolysate could inhibit RANKL-induced TRAP formation, osteoclast-related gene expression and signaling pathways in RAW 264.7 cells. Meanwhile, the SF hydrolysate could induce apoptosis signaling cascades of osteoclasts. On this basis, SF scaffolds seem an ideal choice for bone regeneration to repair bone defects with its capacity to inhibit activity of osteoclasts. However, more researches should be carried out in this field to further understand the effect of SF scaffolds on osteoclasts and bone remodeling.
The vascular ingrowth is another important factor of bone regeneration, which can improve oxygen and nutrient diffusion (Li et al., 2021). It also influences the differentiation of MSCs into osteoblasts and osteoid formation (Yan et al., 2019; Song et al., 2020). The enhanced osteogenic differentiation promotes the secretion of soluble factors, such as bone morphogenetic protein-2 (BMP-2) and beta-catenin, and in turn benefits the vascularization process (Niu et al., 2019). During the biomineralization period, it is also helpful to form functional vascular networks and thus achieve desired bone formation.
The mild inflammatory response can induce the vascular growth and the ingrowth of vessels into SF scaffolds (Thurber et al., 2015; Rameshbabu et al., 2020). Usually, SF scaffolds used for bone regeneration exhibit high porosity and thus allow the vessel ingrowth, which can be improved by pre-seeded cells before implantations. Watchararot et al. (2021) seeded human adipose-derived stem cells (hADSC) onto SF scaffolds and implanted them into chick chorioallantoic membrane with the pore diameter of 513.96 ± 4.99 μm and porosity of 77.34 ± 6.96%. A capillary network of spoke-wheel pattern was induced by the seeded scaffolds 3 weeks earlier than unseeded scaffolds, indicating an early angiogenesis. Sun et al. (Sun et al., 2016) also found the co-cultural of endothelial cells and human mesenchymal stem cells (hMSCs) could significantly improve angiogenesis of SF scaffolds which might result from vascular endothelial growth factor (VEGF) and other angiogenic factors secreted by the pre-seeded cells.
Biomineralization of bone ECM is highly dynamic but well-regulated to obtain diverse organic-inorganic hybrid structures (Kundu et al., 2020). Native bone ECM exhibits three-dimensional (3D) structure with porous morphology and organic-inorganic components. The different physiologic conditions and ECM compositions also influence the deposition of hydroxyapatite (HAP) nanocrystals (Thrivikraman et al., 2019). The mineralized matrix can in turn induce osteogenic differentiation and the formation of mature bone tissues (Xiong et al., 2011). Therefore, an ideal bone graft should have capability to induce biomineralization, similar to natural bone ECM.
SF scaffolds have been proved able to improve the deposition of HAP nanocrystals in simulated body fluid (SBF) solution (Zaharia et al., 2012; Nourmohammadi et al., 2017; Kundu et al., 2020). The amorphous spacers can serve as nucleation sites and promote the deposition of HAP crystals, which is similar to the role of Col I in natural bone (Marelli et al., 2012; Jin et al., 2015; Vetsch et al., 2015). After pretreatment, the formation of HAP crystallization can be facilitated by the electrostatic interaction between the functional groups and calcium ions (Ca2+) (Choi et al., 2012). Huang et al. (Huang et al., 2021) fabricated organized SF film and study the deposition of amorphous calcium phosphate (CaP) in phosphate buffer saline (PBS) and enzyme solution, where a mixture of tricalcium phosphate (TCP) and HAP crystals formed in PBS solution but only HAP crystals could be observed in enzyme solution. The difference possibly resulted from the degradation of SF induced by enzyme, which led to different SF contents and solution pH environment. Meanwhile, it is also interesting to find SF scaffolds from different sources have different performance in biomineralization process. Zhang et al. (Zhang et al., 2020) compared the effect of Antheraea pernyi SF and Bombyx mori SF fibers as templates of biomineralization and found the Antheraea pernyi SF could induce better mineralization, which resulted from more acidic amino acids of hydrophilic amorphous fractions. Sahu et al. (2015) also found nonmulberry SF scaffolds had better osteoconductivity and biomineralization performance than mulberry SF scaffolds.
The techniques to fabricate different SF-based scaffolds depend on the formats they appeared in bone regeneration, such as films, hydrogels and porous scaffolds, which have been summarized in Table 1.
On basis of deposition techniques and drying processes, the technology to fabricate SF films has been well developed, including in-situ co-precipitation method (Mobika et al., 2020; Huang et al., 2022), plasma splashing procedure (Jiang et al., 2020) and matrix-assisted pulsed laser evaporation (Miroiu et al., 2010). To achieve better functionalization, during film preparation, external components can be added, like HAP, silver (Ag) and other bioactive factors, which makes SF hybrid films multifunctional and thus further enhance osteogenesis, including better mechanical properties, angiogenesis, antiinflammation, antibacterial capacity and so on. Jabbari et al. (Jabbari et al., 2019) developed a CS/SF hybrid film via solvent casting method, loading reduced graphene oxide (rGO). With the addition of rGO, the swelling ratio and conformability of hybrid film was accordingly increased, and the evaluation of ALP activity and alizarin red staining also demonstrated the promoted osteogenic performance.
The cross-linking techniques are widely used to fabricate SF hydrogels, including mechanical cross-linking, chemical crosslinking, enzymatic crosslinking and light triggered crosslinking techniques. The mechanical cross-linking technique is simple and economical via ultrasound pulses or temperature changes. As for chemical cross-linking, enzymatic crosslinking and light-triggered crosslinking techniques, external components should be introduced as initiators to achieve better crosslinking. For example, the horseradish peroxidase (HRP) cross-linking technique can control the sol-gel transition better and increase the content of ß-sheets (Moses et al., 2020; Zuluaga-Velez et al., 2021). Furthermore, immersing SF in methanol or ethanol can transform α-helix structure into ß-sheet structure and thus improve the mechanical properties of as-prepared SF hydrogels (Johari et al., 2018a). Piluso et al. (Piluso et al., 2020) developed a rapid riboflavin-mediated crosslinking technique to fabricate cytocompatible SF hydrogel with different riboflavin/sodium persulfate (Ru/SPS) ratio, which exhibited a viability over 80% for all cell types.
With the development of addictive manufacture, 3D printing has become an efficient on-demand manufacturing technique for SF hydrogel, which can help create individual scaffolds of both small and large scale. Furthermore, the cell-laden 3D bioprinting technique permits living cells and biomaterials to be placed in highly organized scaffolds to form complex structures for different applications, which is one of the latest trends in regenerative medicine (Buitrago et al., 2018). Sharma et al. (Sharma et al., 2019) used 3D bioprinting to fabricate a hBMSCs-laden SF/gelatin/CaCl2 hybrid hydrogel with sustained release of Ca2+ and increased ß-sheet contents, which facilitated the osteogenic differentiation and mineralization of the hBMSCs through Wnt/β-catenin pathway. However, due to the formation of ß-sheet, the increase of stiffness and crystallinity can make SF hydrogels brittle and hard to be remodeled by cells (Partlow et al., 2014). In order to overcome the challenges for cell encapsulation, different alternative crosslinking techniques have been explored for cell-laden SF hydrogels, such as chemical crosslinking, enzymatic crosslinking and other redox-based crosslinking, among which photo-triggered crosslinking technique exhibit great potential (Raia et al., 2017; Cui et al., 2020). Cui et al. (Cui et al., 2020) developed a rapid photoredox crosslinking technique to fabricate SF hydrogel, which allowed high cell densities (15 million cells/mL) for cell encapsulation and retained high cell viability (>80%) simultaneously. The photocrosslinked SF hydrogel could reduce spontaneous transition to ß-sheet and possess more stable mechanical properties, demonstrating the immense potential of this crosslinking system for biofabrication and tissue regeneration.
Porous SF scaffolds include flat structures and sponge-like structures. Flat structures can be formed by fibers and exhibit porous characteristic. Almost all the reported fibers are fabricated by electrospinning, and only a few are achieved by knitting machines (Shi et al., 2013; Biagiotti et al., 2022) or spray-drying/pressing (Dong et al., 2020). Sponge-like structures are the most common reported format as porous scaffolds, showing 3D porosities (Hollister, 2005). Sponge-like structures can be achieved by directional temperature field freezing technology (Wang et al., 2020), salt leaching (Samal et al., 2015; Yan et al., 2015), sonication (Gholipourmalekabadi et al., 2015), 3D-printing (Jia et al., 2019), crosslinking (Teimouri et al., 2015; Aliramaji et al., 2017) and combination of layer-by-layer process and freeze drying (Jia et al., 2019). The pore sizes of freeze-dried sponges are below 100 μm, but they can be controlled by changing solvents, pH and temperature (Kundu et al., 2013). Via particle leaching, solvent casting or gas-foaming techniques, the pore structures can be better controlled (Harris et al., 1998).
The plain SF scaffolds exhibit limited osteogenic activity. Modifications should be carried out to enhance osteogenic activity of plain SF scaffolds. In this part, we classify different modification methods in to three kinds: composition adjustment, structure design and surface modification.
The bioactive inorganic components applied to form SF/inorganics hybrid biomaterials include CaP (Gupta et al., 2016; Shao et al., 2016; Ribeiro et al., 2018), graphene oxide (GO) (Balu et al., 2018; Wang et al., 2018), titanium dioxide (TiO2) (Pan et al., 2014; Johari et al., 2018a), silica (SiO2) (Mieszawska et al., 2010; Maleki et al., 2019) and bioactive glass (BG) (Bidgoli et al., 2019; Du et al., 2019).
The CaP, SiO2, TiO2, and BG components can be classified into ceramic biomaterials, exhibiting osteoconductivity, good compression and corrosion resistance, among which HAP has been most applied (Wu et al., 2022). HAP has similar structure and chemical composition with natural bone, which allows them to provide a biomimetic interface for facilitated osseointegration. Ko et al. (2018) integrated HAP with SF scaffolds via blending and alternate soaking, and the modulus was over two times of plain SF scaffolds, which also induced better osteogenic differentiation of human adipose-derived mesenchymal stem cells (hADMSCs) and bone regeneration of calvarial defects (Figure 3). SiO2 and BG are also promising for bone regeneration, whose degradation products show osteoconductive properties (Nikolova and Chavali, 2019). Combining TiO2 nanoparticles with SF scaffolds can facilitate scaffolds with better biocompatibility and osteoconductivity, where TiO2 can help SF hybrid scaffolds to mechanically interlock with bone tissues and promote cell attachment and proliferation (Johari et al., 2017). TiO2 can also enhance the mechanical properties of hybrid biomaterials via facilitating the formation of ß-sheet structure (Pan et al., 2014).
FIGURE 3. In vivo evaluation of the HAP-functionalized SF scaffolds. (A) 3D images of the regenerated calvarial defects treated by different scaffolds and quantitative evaluation. (B) Goldner’s trichrome staining of regenerated calvarial defects. (C) Osteopontin immunofluorescence staining of regenerated calvarial defects after different treatments. (Ko et al., 2018) Copyright 2018 American Chemical Society.
GO is one of graphene derivatives, showing good biocompatibility and osteogenic activities, which is another important inorganic component for SF modification. The great advantage to incorporate GO into SF scaffolds is the improvement of mechanical property (Balu et al., 2018). Moreover, SF/GO hybrid scaffolds also show improved biocompatibility and antibacterial property (Wang et al., 2018). Shuai et al. (Shuai et al., 2018) developed SF/GO matrix to evaluate its effect on stem cell fate, where the modulus increased with introduction of GO and the unique topography could promote early adhesion and osteogenic differentiation of hMSCs without additional inducers.
The bioactive organic components applied to form SF/inorganics hybrid scaffolds include synthetic and natural polymers, such as polycaprolactone (PCL) (Bhattacharjee et al., 2016; Cengiz et al., 2019; Cengiz et al., 2020), collagen (Shi et al., 2017; Bandyopadhyay and Mandal, 2020), CS (Bissoyi et al., 2018), cellulose (Lee et al., 2013; Barud et al., 2015), alginate (ALG) (Zhang et al., 2015; Patil and Singh, 2019) and so on.
PCL is one of main synthetic polymers applied in SF hybrid scaffolds (Dong et al., 2021). The as-prepared scaffolds possess the osteoconductive effect of SF and osteogenic effect of PCL simultaneously, making it excellent for bone regeneration (Li et al., 2020). Bhattacharjee et al. (Bhattacharjee et al., 2016) fabricated SF/PCL nanofibers via electrospinning, where the tensile strength increased by about one fold and the osteogenic differentiation was enhanced compared with plain PCL scaffold.
Introduction of natural polymers seems a feasible approach to enhance cell attachment and osteogenesis due to their favorable biocompatibility and biofunctional components (Wang et al., 2016). SF/collagen and SF/gelatin hybrid scaffolds can exhibit excellent biocompatibility and ECM-mimicking structure, which is able to accelerate the bone formation (Bharadwaz and Jayasuriya, 2020; Wu et al., 2021). SF/CS scaffolds also have great potential in bone regeneration due to the bioactive RGD sequence (Bissoyi et al., 2018). Meanwhile, the combination of cellulose with SF can improve the biodegradability and provide more space for bone regeneration (Ni et al., 2020). Introducing ALG into SF scaffolds can be a promising approach to overcome the poor cell-adhesive property of ALG and the ALG can replace the role of gelatin with lower cost (Patil and Singh, 2019). Perteghella et al. (Perteghella et al., 2017) developed ALG/SF microcarriers with spherical geometry and average diameter of 400 μm, where MSCs adhered rapidly and preserved their potential of multi-lineage differentiation in this innovative 3D culture system.
The favorable performance of SF film makes it a promising strategy for bone regeneration. Li et al. (2022) combined SS and SF to fabricate hybrid films and the breaking strength and breaking elongation increased significantly, which also exhibited faster and well-regulated HAP deposition rate than plain SF films. Furthermore, topographical pattering of SF films can help to achieve better osteogenic performance in vivo. Sayin et al. (2017) prepared collagen/SF hybrid films with microchannel patterns, and human osteoblasts and adipose-derived stem cells (ADSCs) were seeded and aligned on the ridges and in the grooves of the patterned film, where stimulate anisotropic osteogenesis was simulated.
Notably, SF film is also an effective barrier to avoid collapse of surrounding soft tissues into the defect cavity and ensure the successful bone repair. Smeets et al. (2017) evaluated three different SF films and commercial collagen membranes. Compare to collagens, the lower resorbability of SF membranes could promote bone regeneration for a longer period. Therefore, SF films are able to meet some special demands of bone regeneration.
Porous SF scaffolds have ideal structures for bone regeneration due to their great similarity to the in vivo microenvironment. Varkey et al. (2015) compared the impact of different SF structures on MG63 cell attachment and proliferation. The sponge structure showed the highest porosity of 67% and could maintain its structural integrity, where the secreted collagen also increased with culture time, demonstrating the increasing production of ECM. Correia et al. (2012) also fabricated SF porous scaffolds with different pore sizes to investigate their effects, where the SF scaffold with 400–600 μm pore sizes showed better HAP deposition and expression of osteogenic proteins, and further promoted bone tissue formation, demonstrating the importance of scaffold’s pore structures.
However, the mechanical properties of SF porous scaffolds are not satisfying, which limits its application in load-bearing locations. To obtain better mechanical and biological outcomes, external components have been incorporated with SF porous sponges (Kundu et al., 2013). Gupta et al. (2016) fabricated a biomimetic, osteoconductive tricomposite scaffold using HAP, SF fiber from Antheraea assama and its SF solution. The SF-reinforced tricomposite scaffolds exhibited about 5-fold higher compressive modulus and better osteogenic capacity. Therefore, SF-based biomimic porous scaffolds with tough mechanical properties are promising in application of bone regeneration. However, it is still insufficient for load-bearing bone and further investigations should be carried out.
Due to the similarity to microenvironmental of natural tissues, hydrogels have remarkable advantages in bone regeneration (Liaw et al., 2018). SF hydrogels have been widely developed via various crosslinking techniques. Ding et al. (2017) fabricated an injectable SF-based hydrogel via the combination of water-dispersible SF-HAP nanoparticles and the thixotropic SF nanofiber hydrogel. This nano-scale hydrogel system with homogeneously-distributed HAP nanoparticles showed good biocompatibility and osteogenesis in vitro, as well as better bone formation in vivo (Figure 4). Ribeiro et al. (2015) combined SF and nano HAP to develop hybrid hydrogels with favorable porosity, mechanical properties and osteoconductivity, which improved cellular metabolism and ALP activity of MG63 cells. These researches have proved the efficiency of SF hybrid hydrogels to induce bone regeneration.
FIGURE 4. Immunofluorescence staining and histological analysis of osteogenic capacity in vitro and in vivo. (A) ALP expression after 7 days in different hydrogels. (B) OCN expression after 21 days in different hydrogels. (C) OPN expression after 21 days in different hydrogels. The nucleus was stained blue by DAPI and the F-actin was stained red by tetramethylrhodamine (TRITC) conjugated to phalloidin, while ALP, OCN, and OPN were stained green by antibodies conjugated with fluorescein isothiocyanate (FITC). (D) HE and immunohistochemistry staining of OCN and OPN in different hydrogels. (Ding et al., 2017) Copyright 2017 American Chemical Society.
Furthermore, SF hydrogels are ideal drug carriers. An injectable SF-based hybrid hydrogel prepared by Roohaniesfahani et al. (2019) exhibited the capacity to release bioactive silicon, strontium, and magnesium ions simultaneously to promote osteogenesis and angiogenesis. The seeded osteoblasts showed promoted cell proliferation, ALP activity, and enhanced osteogenic gene expression compared to plain SF hydrogel. The in vivo results exhibited decreased fibrous capsule formation and increased new blood vessels around the hydrogel. Besides, multiple SF hydrogels have been prepared to improve their osteogenic capacities with sustainable release of bioactive factors (Ding et al., 2019; Avani et al., 2020; Zarrin et al., 2022). Therefore, SF hydrogels exhibit significant advantages when serving as bioactive scaffolds for bone repair both as matrices and carriers.
Physical modifications of SF scaffolds include ultraviolet (UV) treatment, gas treatment and plasma treatment (Lau et al., 2020). UV irradiation of SF scaffolds can increase wettability and improve cell adhesion without obvious weight loss, crystallinity change and strength decrease (Li et al., 2012; Khosravi et al., 2018). As a powerful oxidizing agent, ozone (O3) gas treatment can increase the pliability of SF scaffolds because of the oxidation of amino acid residues (Li et al., 2012). Plasma treatment of SF scaffolds by different working gases (SO2, NH3, and O2) can increase the antithrombogenicity and cellular activity, making it a potential modification technique for bone regeneration (Uchida et al., 2014; Ribeiro et al., 2016). Wang et al. (2019) used a unique vacuum UV/O3 activation method to treat SF film, which improved the biocompatibility with BMSCs and osteogenesis in vivo. Kondyurin et al. (2018) applied plasma immersion ion implantation (PIII) to treat SF scaffold and receive a carbon-rich structure, which enhanced its interaction with both proteins and cells and exhibited significantly higher levels of cell adhesion and proliferation.
Considering the active groups of peptide chains, SF scaffolds have plenty of active modification sites, which allows different techniques for chemical modification, such as grafting copolymerization techniques and introduction of chemical agents (Murphy et al., 2008; Zhou et al., 2017; Zhou et al., 2018). Hardy et al. (2018) used a simple and rapid photochemical modification technique to initiate the polymerization and thus decorated the SF scaffolds with poly (acrylic acid) (PAA), poly (methacrylic acid) (PMAA), and poly (allylamine) (PAAm). The results showed that the PAA- and PMAA-functionalized SF scaffolds can be well-mineralized, indicating their potential in bone regeneration.
In term of 3D bioprinting of SF hydrogels, modification of vinyl-containing functional groups can help accelerate crosslinking process, especially methacrylate, which can make as-prepared glycidyl-methacrylate-modified SF (SF-MA) hydrogels photocurable. Barroso et al. studied the effect of the SF-MA solution pH on the properties of SF-MA hydrogels, where the SF-MA prepared at pH 5 exhibited better mechanical properties than that prepared in pH 7 and 8, and the hydrogel pH did not affect the good biocompatibility of as-prepared SF hydrogels.
As for SF scaffolds, it is necessary to further improve osteogenesis especially in the repair of critical-sized or weight-bearing bone defects. Bioactive components and cells loaded on SF scaffolds have received increasing attention in bone regeneration. Different cytokines, drugs and pre-cultured cells can directly regulate cell behavior and promote bone regeneration.
During cell recruitment, proliferation and osteogenesis, cytokines play an important role (Lei et al., 2021; Wu et al., 2021). Loading cytokines on SF scaffolds is a promising method to direct cell differentiation and promote bone formation, including BMP-2 (Niu et al., 2017; Song et al., 2018), stromal-derived factor-1 (SDF-1) (Shen et al., 2016), VEGF (Besheli et al., 2018; Fitzpatrick et al., 2021), DFO (Wang et al., 2017; Ding et al., 2019) and so on. Fitzpatrick et al. (2021) generated multi-functionalized 3D-printed SF/HAP scaffolds loading BMP-2. VEGF and neural growth factor (NGF) to accelerate bone regeneration. The modulus of as-prepared scaffolds significantly increased and the expression of osteogenesis-related genes (Runx2, OPN, bone sialoprotein) was up-regulated by the synergistic enhancement of three factors.
Infection and inflammation are significant challenges during bone regeneration. Drug-loading SF scaffolds seem a promising strategy to overcome these problems with application of antibacterial and anti-inflammatory drugs, such as gentamicin (Sharma et al., 2016), vancomycin (Besheli et al., 2017), Ag nanoparticles (AgNPs) (Zhou et al., 2017), dexamethasone (Moses and Mandal, 2022) and so on. Notably, combination of AgNPs and conventional antibiotics can achieve stronger antimicrobial performance and thus promote bone regeneration. Zhou et al. (Zhou et al., 2017) loaded AgNPs/gentamicin on SF scaffolds with the assistance of polydopamine, where AgNPs and gentamicin effectively inhibited adhesion and proliferation of S. aureus, and improved cell adhesion, proliferation and osteogenic differentiation simultaneously. (Figure 5). However, it still remains a challenge to avoid rapid drug releasing and obtain sustainable release and functionalization in vivo.
FIGURE 5. Antibacterial activities and biocompatibility of AgNPs/gentamicin-loaded SF scaffolds. (A) Quantitative evaluation of S. aureus cultured on different samples after 24 h (B) MC3T3 cell morphologies detected by fluorescent staining of FITC and DAPI (Zhou et al., 2017). Copyright 2017 American Chemical Society.
Besides, BMSCs and ADSCs are often seeded on SF scaffolds before implantation as bioactive components due to their differentiation potential. Plenty of researches indicated that pre-cultured BMSCs could effectively promote bone regeneration (Herrmann et al., 2015; Gao et al., 2016). Similarly, ADSCs exhibit anticipated osteogenic performance in different SF scaffolds, where ADSCs-loaded SF scaffolds can promote biomineralization and bone formation (Chen et al., 2016; Sartika et al., 2020). However, ADSCs alone are hard to achieve ideal differentiation towards osteoblasts without bioactive molecules or factors. It is essential to apply bioactive components as osteogenic inducers.
SF scaffolds exhibit great potential in bone regeneration due to advantages of biocompatibility, biodegradability, mechanical strength and structural adjustability. With the rapid development of modern technology, a wide range of techniques has been employed to fabricate different types of SF scaffolds, such as films, porous scaffolds and hydrogels. For better functionalization and osteogenesis, SF scaffolds have been modified with plenty of components to maximize its mechanical and biological functional properties, such as angiogenesis, antibiosis, antiinflammation and cell-laden capacity. Based on recent technical advances in fabrication of SF scaffolds, researchers can better design and fabricate SF scaffolds according to practical demands of bone regeneration. Especially, with the development of 3D bioprinting, it has been feasible to achieve accurate shape and structures according the bone defects, which can accommodate living-cell patterning to mimic native bone tissues. These encouraging advancements have open up new opportunities in the application of SF scaffolds in bone regeneration, where controllable biodegradation and good mechanical properties are critically required.
Furthermore, SF hybrid scaffolds are able to simulate the natural bone microenvironment and promote osteogenesis with the help of well-designed structures, bioactive components and functional surface modification. However, there still lack sufficient studies on evaluation of SF scaffolds in big animal models such as dogs and pigs, which is necessary before future clinical applications. Meanwhile, considering their long-term close contact with tissues and the potential cytotoxicity and immunogenicity related to degradation products, it is vital to resolve the concerns of long-term in vivo safety of SF scaffolds. Therefore, although various techniques have been developed, it still needs more deep investigations to achieve comprehensive understanding of SF scaffolds.
Notably, although SF scaffolds have been investigated for a long time, it still has not been approved by regulatory authorities for any clinical application in bone regeneration. The clinical translation of such a Class III medical device can be quite time and cost-consuming and the translation process from bench to bedside is still unfamiliar to most researchers. So far, no clinical trials of SF scaffolds for bone regeneration have been registered in ClinicalTrials.gov or reported. Taken together, to promote better clinical translation of SF scaffolds for bone regeneration, more efforts should be carried out.
More comprehensive investigations are required for deeper understanding of the degradation and clearing mechanisms of different SF scaffolds.
More high-quality researches are in demand for further investigation on the degradation products of SF scaffolds to resolve concerns on the clinical use of SF scaffolds.
More comprehensive translational researches for SF scaffolds are needed to make further steps to realize effective clinical translation, especially experiments in big animal models and clinical trials.
Overall, SF scaffolds are promising candidates to promote osteogenesis and have great potential in the field of medical devices for bone regeneration. This review intends to reveal the interaction between SF scaffolds and hosts’ cells and tissues, as well as up-to-date research status, which provides a deep and comprehensive insight into SF scaffolds for bone regeneration. It will help provide strong evidence to support the development and improvement of SF scaffolds for superior osteogenic performance.
HW has written the manuscript text and CZ is responsive for the revision. XW and KL have conceived the concept of this review.
This review was supported by National Natural Science Foundation of China (81871490, 82001006, 82071096), the Interdisciplinary Program of Shanghai Jiao Tong University (YG2022ZD014, YG2021ZD12), Science and Technology Commission of Shanghai Municipality (21DZ2294600, 21490711700), Program of Shanghai Academic/Technology Research Leader (20XD1433100, 19XD1434500), Double Hundred Plan (20191819), and CAMS Innovation Fund for Medical Sciences (CIFMS) (2019-I2M-5-037).
The authors declare that the research was conducted in the absence of any commercial or financial relationships that could be construed as a potential conflict of interest.
All claims expressed in this article are solely those of the authors and do not necessarily represent those of their affiliated organizations, or those of the publisher, the editors and the reviewers. Any product that may be evaluated in this article, or claim that may be made by its manufacturer, is not guaranteed or endorsed by the publisher.
Aliramaji, S., Zamanian, A., and Mozafari, M. (2017). Super-paramagnetic responsive silk fibroin/chitosan/magnetite scaffolds with tunable pore structures for bone tissue engineering applications. Mater. Sci. Eng. C 70, 736–744. doi:10.1016/j.msec.2016.09.039
Atrian, M., Kharaziha, M., Emadi, R., and Alihosseini, F. (2019). Silk-Laponite® fibrous membranes for bone tissue engineering. Appl. Clay Sci. 174, 90–99. doi:10.1016/j.clay.2019.03.038
Avani, F., Damoogh, S., Mottaghitalab, F., Karkhaneh, A., and Farokhi, M. (2020). Vancomycin loaded halloysite nanotubes embedded in silk fibroin hydrogel applicable for bone tissue engineering. Int. J. Polym. Mater. Polym. Biomaterials 69, 32–43. doi:10.1080/00914037.2019.1616201
Bakhshandeh, B., Nateghi, S. S., Gazani, M. M., Dehghani, Z., and Mohammadzadeh, F. (2021). A review on advances in the applications of spider silk in biomedical issues. Int. J. Biol. Macromol. 192, 258–271. doi:10.1016/j.ijbiomac.2021.09.201
Balu, R., Reeder, S., Knott, R., Mata, J., De Campo, L., Dutta, N. K., et al. (2018). Tough photocrosslinked silk fibroin/graphene oxide nanocomposite hydrogels. Langmuir 34 (31), 9238–9251. doi:10.1021/acs.langmuir.8b01141
Bandyopadhyay, A., and Mandal, B. B. (2020). A three-dimensional printed silk-based biomimetic tri-layered meniscus for potential patient-specific implantation. Biofabrication 12, 015003. doi:10.1088/1758-5090/ab40fa
Baptista, M., Joukhdar, H., Alcala-Orozco, C. R., Lau, K., Jiang, S., Cui, X., et al. (2020). Silk fibroin photo-lyogels containing microchannels as a biomaterial platform for in situ tissue engineering. Biomater. Sci. 8 (24), 7093–7105. doi:10.1039/d0bm01010c
Barud, H. G. O., Barud, H. D., Cavicchioli, M., Do Amaral, T. S., De Oliveira, O. B., Santos, D. M., et al. (2015). Preparation and characterization of a bacterial cellulose/silk fibroin sponge scaffold for tissue regeneration. Carbohydr. Polym. 128, 41–51. doi:10.1016/j.carbpol.2015.04.007
Besheli, N. H., Damoogh, S., Zafar, B., Mottaghitalab, F., Motasadizadeh, H., Rezaei, F., et al. (2018). Preparation of a codelivery system based on vancomycin/silk scaffold containing silk nanoparticle loaded VEGF. ACS Biomater. Sci. Eng. 4 (8), 2836–2846. doi:10.1021/acsbiomaterials.8b00149
Besheli, N. H., Mottaghitalab, F., Eslami, M., Gholami, M., Kundu, S. C., Kaplan, D. L., et al. (2017). Sustainable release of vancomycin from silk fibroin nanoparticles for treating severe bone infection in rat tibia osteomyelitis model. ACS Appl. Mat. Interfaces 9 (6), 5128–5138. doi:10.1021/acsami.6b14912
Bharadwaz, A., and Jayasuriya, A. C. (2020). Recent trends in the application of widely used natural and synthetic polymer nanocomposites in bone tissue regeneration. Mater. Sci. Eng. C 110, 110698. doi:10.1016/j.msec.2020.110698
Bhattacharjee, P., Kundu, B., Naskar, D., Kim, H. W., Bhattacharya, D., Maiti, T. K., et al. (2016). Potential of inherent RGD containing silk fibroin–poly (Є-caprolactone) nanofibrous matrix for bone tissue engineering. Cell. Tissue Res. 363 (2), 525–540. doi:10.1007/s00441-015-2232-6
Biagiotti, M., Bassani, G. A., Chiarini, A., Vincoli, V. T., Dal Pra, I., Cosentino, C., et al. (2022). Three-Layered silk fibroin tubular scaffold for the repair and regeneration of small caliber blood vessels: From design to in vivo pilot tests. Front. Bioeng. Biotechnol. 10, 356. doi:10.3389/fbioe.2019.00356
Bidgoli, M. R., Alemzadeh, I., Tamjid, E., Khafaji, M., and Vossoughi, M. (2019). Fabrication of hierarchically porous silk fibroin-bioactive glass composite scaffold via indirect 3D printing: Effect of particle size on physico-mechanical properties and in vitro cellular behavior. Mater. Sci. Eng. C 103, 109688. doi:10.1016/j.msec.2019.04.067
Bissoyi, A., Singh, A. K., Pattanayak, S. K., Bit, A., Sinha, S. K., Patel, A., et al. (2018). Understanding the molecular mechanism of improved proliferation and osteogenic potential of human mesenchymal stem cells grown on a polyelectrolyte complex derived from non-mulberry silk fibroin and chitosan. Biomed. Mat. 13 (1), 015011. doi:10.1088/1748-605X/aa890c
Borciani, G., Montalbano, G., Baldini, N., Cerqueni, G., Vitale-Brovarone, C., and Ciapetti, G. (2020). Co-culture systems of osteoblasts and osteoclasts: Simulating in vitro bone remodeling in regenerative approaches. Acta Biomater. 108, 22–45. doi:10.1016/j.actbio.2020.03.043
Buitrago, J. O., Patel, K. D., El-Fiqi, A., Lee, J. H., Kundu, B., Lee, H. H., et al. (2018). Silk fibroin/collagen protein hybrid cell-encapsulating hydrogels with tunable gelation and improved physical and biological properties. Acta Biomater. 69, 218–233. doi:10.1016/j.actbio.2017.12.026
Burger, M. G., Grosso, A., Briquez, P. S., Born, G. M. E., Lunger, A., Schrenk, F., et al. (2022). Robust coupling of angiogenesis and osteogenesis by VEGF-decorated matrices for bone regeneration. Acta Biomater. 149, 111–125. doi:10.1016/j.actbio.2022.07.014
Cengiz, I. F., Maia, F. R., Morais, A. D., Silva-Correia, J., Pereira, H., Canadas, R. F., et al. (2020). Entrapped in cage (EiC) scaffolds of 3D-printed polycaprolactone and porous silk fibroin for meniscus tissue engineering. Biofabrication 12 (2), 025028. doi:10.1088/1758-5090/ab779f
Cengiz, I. F., Pereira, H., Espregueira-Mendes, J., Kwon, I. K., Reis, R. L., and Oliveira, J. M. (2019). Suturable regenerated silk fibroin scaffold reinforced with 3D-printed polycaprolactone mesh: Biomechanical performance and subcutaneous implantation. J. Mat. Sci. Mat. Med. 30 (6), 63. doi:10.1007/s10856-019-6265-3
Chen, H. B., Qian, Y. Z., Xia, Y., Chen, G., Dai, Y., Li, N., et al. (2016). Enhanced osteogenesis of ADSCs by the synergistic effect of aligned fibers containing collagen I. ACS Appl. Mat. Interfaces 8 (43), 29289–29297. doi:10.1021/acsami.6b08791
Choi, Y., Cho, S. Y., Park, D. J., Park, H. H., Heo, S., and Jin, H. J. (2012). Silk fibroin particles as templates for mineralization of calcium-deficient hydroxyapatite. J. Biomed. Mat. Res. 100 (8), 2029–2034. doi:10.1002/jbm.b.32766
Chon, J. W., Kim, H., Jeon, H. N., Park, K., Lee, K. G., Yeo, J. H., et al. (2012). Silk fibroin hydrolysate inhibits osteoclastogenesis and induces apoptosis of osteoclasts derived from RAW 264.7 cells. Int. J. Mol. Med. 30 (5), 1203–1210. doi:10.3892/ijmm.2012.1120
Correia, C., Bhumiratana, S., Yan, L. P., Oliveira, A. L., Gimble, J. M., Rockwood, D., et al. (2012). Development of silk-based scaffolds for tissue engineering of bone from human adipose-derived stem cells. Acta Biomater. 8 (7), 2483–2492. doi:10.1016/j.actbio.2012.03.019
Cui, X., Soliman, B. G., Alcala-Orozco, C. R., Li, J., Vis, M. a. M., Santos, M., et al. (2020). Rapid photocrosslinking of silk hydrogels with high cell density and enhanced shape fidelity. Adv. Healthc. Mat. 9 (22), e2001801. doi:10.1002/adhm.202001801
Ding, Z. Z., Han, H. Y., Fan, Z. H., Lu, H. J., Sang, Y. H., Yao, Y. L., et al. (2017). Nanoscale silk-hydroxyapatite hydrogels for injectable bone biomaterials. ACS Appl. Mat. Interfaces 9 (20), 16913–16921. doi:10.1021/acsami.7b03932
Ding, Z. Z., Zhou, M. L., Zhou, Z. Y., Zhang, W. J., Jiang, X. Q., Lu, X. H., et al. (2019). Injectable silk nanofiber hydrogels for sustained release of small-molecule drugs and vascularization. ACS Biomater. Sci. Eng. 5 (8), 4077–4088. doi:10.1021/acsbiomaterials.9b00621
Dong, L. L., Li, L. H., Song, Y., Fang, Y. N., Liu, J. L., Chen, P. X., et al. (2021). MSC-derived immunomodulatory extracellular matrix functionalized electrospun fibers for mitigating foreign-body reaction and tendon adhesion. Acta Biomater. 133, 280–296. doi:10.1016/j.actbio.2021.04.035
Dong, Y. D., Hong, M. M., Dai, R. Z., Wu, H. Y., and Zhu, P. (2020). Engineered bioactive nanoparticles incorporated biofunctionalized ECM/silk proteins based cardiac patches combined with MSCs for the repair of myocardial infarction: In vitro and in vivo evaluations. Sci. Total Environ. 707, 707 135976. doi:10.1016/j.scitotenv.2019.135976
Du, X. Y., Wei, D. X., Huang, L., Zhu, M., Zhang, Y. P., and Zhu, Y. F. (2019). 3D printing of mesoporous bioactive glass/silk fibroin composite scaffolds for bone tissue engineering. Mater. Sci. Eng. C 103, 109731. doi:10.1016/j.msec.2019.05.016
Fitzpatrick, V., Martin-Moldes, Z., Deck, A., Torres-Sanchez, R., Valat, A., Cairns, D., et al. (2021). Functionalized 3D-printed silk-hydroxyapatite scaffolds for enhanced bone regeneration with innervation and vascularization. Biomaterials 276, 120995. doi:10.1016/j.biomaterials.2021.120995
Gao, M., Chen, J. J., Lin, G. W., Li, S. W., Wang, L., Qin, A. J., et al. (2016). Long-term tracking of the osteogenic differentiation of mouse BMSCs by aggregation-induced emission nanoparticles. ACS Appl. Mat. Interfaces 8 (28), 17878–17884. doi:10.1021/acsami.6b05471
Gellynck, K., Verdonk, P. C. M., Van Nimmen, E., Almqvist, K. F., Gheysens, T., Schoukens, G., et al. (2008). Silkworm and spider silk scaffolds for chondrocyte support. J. Mat. Sci. Mat. Med. 19, 3399–3409. doi:10.1007/s10856-008-3474-6
Gholipourmalekabadi, M., Mozafari, M., Gholipourmalekabadi, M., Bojnordi, M. N., Hashemi-Soteh, M. B., Salimi, M., et al. (2015). In vitro and in vivo evaluations of three-dimensional hydroxyapatite/silk fibroin nanocomposite scaffolds. Biotechnol. Appl. Biochem. 62 (4), 441–450. doi:10.1002/bab.1285
Gorenkova, N., Maitz, M. F., Bohme, G., Alhadrami, H. A., Jiffri, E. H., Totten, J. D., et al. (2021). The innate immune response of self-assembling silk fibroin hydrogels. Biomater. Sci. 9 (21), 7194–7204. doi:10.1039/d1bm00936b
Guo, L. Q., Liang, Z. H., Yang, L., Du, W. Y., Yu, T., Tang, H. Y., et al. (2021). The role of natural polymers in bone tissue engineering. J. Control. Release 338, 571–582. doi:10.1016/j.jconrel.2021.08.055
Gupta, P., Adhikary, M., Christakiran, M. J., Kumar, M., Bhardwaj, N., and Mandal, B. B. (2016). Biomimetic, osteoconductive non-mulberry silk fiber reinforced tricomposite scaffolds for bone tissue engineering. ACS Appl. Mat. Interfaces 8 (45), 30797–30810. doi:10.1021/acsami.6b11366
Hadisi, Z., Bakhsheshi-Rad, H. R., Walsh, T., Dehghan, M. M., Farzad-Mohajeri, S., Gholami, H., et al. (2020). In vitro and in vivo evaluation of silk fibroin-hardystonite-gentamicin nanofibrous scaffold for tissue engineering applications. Polym. Test. 91, 106698. doi:10.1016/j.polymertesting.2020.106698
Hardy, J. G., Bertin, A., Torres-Rendon, J. G., Leal-Egana, A., Humenik, M., Bauer, F., et al. (2018). Facile photochemical modification of silk protein-based biomaterials. Macromol. Biosci. 18 (11), e1800216. doi:10.1002/mabi.201800216
Harris, L. D., Kim, B. S., and Mooney, D. J. (1998). Open pore biodegradable matrices formed with gas foaming. J. Biomed. Mat. Res. 42, 396–402. doi:10.1002/(sici)1097-4636(19981205)42:3<396::aid-jbm7>3.0.co;2-e
Herrmann, M., Verrier, S., and Alini, M. (2015). Strategies to stimulate mobilization and homing of endogenous stem and progenitor cells for bone tissue repair. Front. Bioeng. Biotechnol. 3, 79. doi:10.3389/fbioe.2015.00079
Hollister, S. J. (2005). Porous scaffold design for tissue engineering. Nat. Mat. 4 (7), 518–524. doi:10.1038/nmat1421
Huang, T. T., Zhou, Z. H., Li, Q. Y., Tang, X. X., Chen, X. L., Ge, Y. F., et al. (2022). Light-triggered adhesive silk-based film for effective photodynamic antibacterial therapy and rapid hemostasis. Front. Bioeng. Biotechnol. 9, 820434. doi:10.3389/fbioe.2021.820434
Huang, Y., Zou, Z. Y., Ping, H., Lei, L. W., Xie, J. J., Xie, H., et al. (2021). Mineralization of calcium phosphate induced by a silk fibroin film under different biological conditions. RSC Adv. 11, 18590–18596. doi:10.1039/d1ra02199k
Jabbari, F., Hesaraki, S., and Houshmand, B. (2019). The physical, mechanical, and biological properties of silk fibroin/chitosan/reduced graphene oxide composite membranes for guided bone regeneration. J. Biomaterials Sci. Polym. Ed. 30, 1779–1802. doi:10.1080/09205063.2019.1666235 Ed.
Jia, Z. J., Zhou, W. H., Yan, J. L., Xiong, P., Guo, H., Cheng, Y., et al. (2019). Constructing multilayer silk protein/nanosilver biofunctionalized hierarchically structured 3D printed Ti6Al4 V scaffold for repair of infective bone defects. ACS Biomater. Sci. Eng. 5, 244–261. doi:10.1021/acsbiomaterials.8b00857
Jiang, S. B., Liu, X. D., Liu, Y., Liu, J., He, W. D., and Dong, Y. F. (2020). Synthesis of silver @hydroxyapatite nanoparticles based biocomposite and their assessment for viability of Osseointegration for rabbit knee joint anterior cruciate ligament rehabilitation. J. Photochem. Photobiol. B Biol. 202, 111677. doi:10.1016/j.jphotobiol.2019.111677
Jin, Y. S., Kundu, B., Cai, Y. R., Kundu, S. C., and Yao, J. M. (2015). Bio-inspired mineralization of hydroxyapatite in 3D silk fibroin hydrogel for bone tissue engineering. Colloids Surfaces B Biointerfaces 134, 339–345. doi:10.1016/j.colsurfb.2015.07.015
Johari, N., Hosseini, H. R. M., and Samadikuchaksaraei, A. (2018a). Novel fluoridated silk fibroin/TiO2 nanocomposite scaffolds for bone tissue engineering. Mater. Sci. Eng. C 82, 265–276. doi:10.1016/j.msec.2017.09.001
Johari, N., Hosseini, H. R. M., and Samadikuchaksaraei, A. (2017). Optimized composition of nanocomposite scaffolds formed from silk fibroin and nano-TiO2 for bone tissue engineering. Mater. Sci. Eng. C 79, 783–792. doi:10.1016/j.msec.2017.05.105
Johari, N., Hosseini, H. R. M., Taromi, N., Arasteh, S., Kazemnejad, S., and Samadikuchaksaraei, A. (2018b). Evaluation of bioactivity and biocompatibility of silk fibroin/TiO2 nanocomposite. J. Med. Biol. Eng. 38, 99–105. doi:10.1007/s40846-017-0295-4
Jones, G. L., Motta, A., Marshall, M. J., El Haj, A. J., and Cartmell, S. H. (2009). Osteoblast: Osteoclast co-cultures on silk fibroin, chitosan and PLLA films. Biomaterials 30 (29), 5376–5384. doi:10.1016/j.biomaterials.2009.07.028
Jung, S. R., Song, N. J., Yang, D. K., Cho, Y. J., Kim, B. J., Hong, J. W., et al. (2013). Silk proteins stimulate osteoblast differentiation by suppressing the Notch signaling pathway in mesenchymal stem cells. Nutr. Res. 33 (2), 162–170. doi:10.1016/j.nutres.2012.11.006
Khosravi, A., Ghasemi-Mobarakeh, L., Mollahosseini, H., Ajalloueian, F., Rad, M. M., Norouzi, M. R., et al. (2018). Immobilization of silk fibroin on the surface of PCL nanofibrous scaffolds for tissue engineering applications. J. Appl. Polym. Sci. 135, 46684. doi:10.1002/app.46684
Ko, E., Lee, J. S., Kim, H., Yang, S. Y., Yang, D., Yang, K., et al. (2018). Electrospun silk fibroin nanofibrous scaffolds with two-stage hydroxyapatite functionalization for enhancing the osteogenic differentiation of human adipose-derived mesenchymal stem cells. ACS Appl. Mat. Interfaces 10, 7614–7625. doi:10.1021/acsami.7b03328
Koh, L. D., Cheng, Y., Teng, C. P., Khin, Y. W., Loh, X. J., Tee, S. Y., et al. (2015). Structures, mechanical properties and applications of silk fibroin materials. Prog. Polym. Sci. 46, 86–110. doi:10.1016/j.progpolymsci.2015.02.001
Kondyurin, A., Lau, K., Tang, F., Akhavan, B., Chrzanowski, W., Lord, M. S., et al. (2018). Plasma ion implantation of silk biomaterials enabling direct covalent immobilization of bioactive agents for enhanced cellular responses. ACS Appl. Mat. Interfaces 10 (21), 17605–17616. doi:10.1021/acsami.8b03182
Kundu, B., Brancato, V., Oliveira, J. M., Correlo, V. M., Reis, R. L., and Kundu, S. C. (2020). Silk fibroin promotes mineralization of gellan gum hydrogels. Int. J. Biol. Macromol. 153, 1328–1334. doi:10.1016/j.ijbiomac.2019.10.269
Kundu, B., Rajkhowa, R., Kundu, S. C., and Wang, X. G. (2013). Silk fibroin biomaterials for tissue regenerations. Adv. Drug Deliv. Rev. 65, 457–470. doi:10.1016/j.addr.2012.09.043
Lau, K., Akhavan, B., Lord, M. S., Bilek, M. M., and Rnjak-Kovacina, J. (2020). Dry surface treatments of silk biomaterials and their utility in biomedical applications. ACS Biomater. Sci. Eng. 6, 5431–5452. doi:10.1021/acsbiomaterials.0c00888
Lee, J., Huh, S. J., Seok, J. M., Lee, S., Byun, H., Jang, G. N., et al. (2022). Surface engineering of 3D-printed scaffolds with minerals and a pro-angiogenic factor for vascularized bone regeneration. Acta Biomater. 140, 730–744. doi:10.1016/j.actbio.2021.12.007
Lee, J. M., Kim, J. H., Lee, O. J., and Park, C. H. (2013). The fixation effect of a silk fibroin–bacterial cellulose composite plate in segmental defects of the zygomatic Arch<subtitle>An experimental study</subtitle><alt-title>Silk fibroin–bacterial cellulose composite plate</alt-title>. JAMA Otolaryngol. Head. Neck Surg. 139 (6), 629–635. doi:10.1001/jamaoto.2013.3044
Lei, T. Y., Zhang, T., Ju, W., Chen, X., Heng, B. C., Shen, W. L., et al. (2021). Biomimetic strategies for tendon/ligament-to-bone interface regeneration. Bioact. Mat. 6, 2491–2510. doi:10.1016/j.bioactmat.2021.01.022
Li, G. F., and Sun, S. (2022). Silk fibroin-based biomaterials for tissue engineering applications. Molecules 27 (9), 2757. doi:10.3390/molecules27092757
Li, G. H., Liu, H., Li, T. D., and Wang, J. Y. (2012). Surface modification and functionalization of silk fibroin fibers/fabric toward high performance applications. Mater. Sci. Eng. C 32 (4), 627–636. doi:10.1016/j.msec.2011.12.013
Li, J. L., Cao, F., Wu, B., Yang, J. H., Xu, W. W., Wang, W. D., et al. (2021). Immobilization of bioactive vascular endothelial growth factor onto Ca-deficient hydroxyapatite-coated Mg by covalent bonding using polydopamine. J. Orthop. Transl. 30, 82–92. doi:10.1016/j.jot.2021.06.002
Li, M., Tian, W., Zhang, Y., Song, H., Yu, Y. X., Chen, X. S., et al. (2022). Enhanced silk fibroin/sericin composite film: Preparation, mechanical properties and mineralization activity. Polym. (Basel). 14 (12), 2466. doi:10.3390/polym14122466
Li, Y. Y., Chen, M. X., Zhou, W. H., Gao, S., Luo, X. J., Peng, L. Q., et al. (2020). Cell-free 3D wet-electrospun PCL/silk fibroin/Sr2+ scaffold promotes successful total meniscus regeneration in a rabbit model. Acta Biomater. 113, 196–209. doi:10.1016/j.actbio.2020.06.017
Liaw, C. Y., Ji, S., and Guvendiren, M. (2018). Engineering 3D hydrogels for personalized in vitro human tissue models. Adv. Healthc. Mat. 7, 1701165. doi:10.1002/adhm.201701165
Liu, T., Chen, Y. R., Lai, D. Z., Zhang, L. X., Pan, X. D., Chen, J. Y., et al. (2019). Biomimetic fabrication of new bioceramics-introduced fibrous scaffolds: From physicochemical characteristics to in vitro biological properties. Mater. Sci. Eng. C 94, 547–557. doi:10.1016/j.msec.2018.09.063
Lundmark, K., Westermark, G. T., Olsen, A., and Westermark, P. (2005). Protein fibrils in nature can enhance amyloid protein A amyloidosis in mice: Cross-seeding as a disease mechanism. Proc. Natl. Acad. Sci. U. S. A. 102 (17), 6098–6102. doi:10.1073/pnas.0501814102
Maleki, H., Shahbazi, M. A., Montes, S., Hosseini, S. H., Eskandari, M. R., Zaunschirm, S., et al. (2019). Mechanically strong silica-silk fibroin bioaerogel: A hybrid scaffold with ordered honeycomb micromorphology and multiscale porosity for bone regeneration. ACS Appl. Mat. Interfaces 11 (19), 17256–17269. doi:10.1021/acsami.9b04283
Marelli, B., Ghezzi, C. E., Alessandrino, A., Barralet, J. E., Freddi, G., and Nazhat, S. N. (2012). Silk fibroin derived polypeptide-induced biomineralization of collagen. Biomaterials 33 (1), 102–108. doi:10.1016/j.biomaterials.2011.09.039
Meinel, L., Fajardo, R., Hofmann, S., Langer, R., Chen, J., Snyder, B., et al. (2005). Silk implants for the healing of critical size bone defects. Bone 37 (5), 688–698. doi:10.1016/j.bone.2005.06.010
Melke, J., Midha, S., Ghosh, S., Ito, K., and Hofmann, S. (2016). Silk fibroin as biomaterial for bone tissue engineering. Acta Biomater. 31, 1–16. doi:10.1016/j.actbio.2015.09.005
Mieszawska, A. J., Fourligas, N., Georgakoudi, I., Ouhib, N. M., Belton, D. J., Perry, C. C., et al. (2010). Osteoinductive silk-silica composite biomaterials for bone regeneration. Biomaterials 31 (34), 8902–8910. doi:10.1016/j.biomaterials.2010.07.109
Mirkhalaf, M., Men, Y., Wang, R., No, Y., and Zreiqat, H. (2022). Personalized 3D printed bone scaffolds: A review. Acta Biomater. In Press. doi:10.1016/j.actbio.2022.04.014
Miroiu, F. M., Socol, G., Visan, A., Stefan, N., Craciun, D., Craciun, V., et al. (2010). Composite biocompatible hydroxyapatite-silk fibroin coatings for medical implants obtained by Matrix Assisted Pulsed Laser Evaporation. Mater. Sci. Eng. B 169, 151–158. doi:10.1016/j.mseb.2009.10.004
Miyamoto, S., Koyanagi, R., Nakazawa, Y., Nagano, A., Abiko, Y., Inada, M., et al. (2013). Bombyx mori silk fibroin scaffolds for bone regeneration studied by bone differentiation experiment. J. Biosci. Bioeng. 115 (5), 575–578. doi:10.1016/j.jbiosc.2012.11.021
Mobika, J., Rajkumar, M., Sibi, S. P. L., and Priya, V. N. (2020). Fabrication of bioactive hydroxyapatite/silk fibroin/gelatin cross-linked nanocomposite for biomedical application. Mat. Chem. Phys. 250, 123187. doi:10.1016/j.matchemphys.2020.123187
Moses, J. C., and Mandal, B. B. (2022). Mesoporous silk-bioactive glass nanocomposites as drug eluting multifunctional conformal coatings for improving osseointegration and bactericidal properties of metal implants. ACS Appl. Mat. Interfaces 14 (13), 14961–14980. doi:10.1021/acsami.2c00093
Moses, J. C., Saha, T., and Mandal, B. B. (2020). Chondroprotective and osteogenic effects of silk-based bioinks in developing 3D bioprinted osteochondral interface. Bioprinting 17, e00067. doi:10.1016/j.bprint.2019.e00067
Mottaghitalab, F., Hosseinkhani, H., Shokrgozar, M. A., Mao, C. B., Yang, M. Y., and Farokhi, M. (2015). Silk as a potential candidate for bone tissue engineering. J. Control. Release 215, 112–128. doi:10.1016/j.jconrel.2015.07.031
Murphy, A. R., John, P. S., and Kaplan, D. L. (2008). Modification of silk fibroin using diazonium coupling chemistry and the effects on hMSC proliferation and differentiation. Biomaterials 29 (19), 2829–2838. doi:10.1016/j.biomaterials.2008.03.039
Ni, T. Y., Liu, M., Zhang, Y. J., Cao, Y., and Pei, R. J. (2020). 3D bioprinting of bone marrow mesenchymal stem cell-laden silk fibroin Double network scaffolds for cartilage tissue repair. Bioconjug. Chem. 31 (8), 1938–1947. doi:10.1021/acs.bioconjchem.0c00298
Nie, L., Zhang, H., Ren, A. S., Li, Y. Z., Fu, G., Cannon, R. D., et al. (2019). Nano-hydroxyapatite mineralized silk fibroin porous scaffold for tooth extraction site preservation. Dent. Mat. 35 (10), 1397–1407. doi:10.1016/j.dental.2019.07.024
Nikolova, M. P., and Chavali, M. S. (2019). Recent advances in biomaterials for 3D scaffolds: A review. Bioact. Mat. 4, 271–292. doi:10.1016/j.bioactmat.2019.10.005
Niu, B. J., Li, B., Gu, Y., Shen, X. F., Liu, Y., and Chen, L. (2017). In vitro evaluation of electrospun silk fibroin/nano-hydroxyapatite/BMP-2 scaffolds for bone regeneration. J. Biomaterials Sci. Polym. Ed. 28 (3), 257–270. doi:10.1080/09205063.2016.1262163
Niu, X. L., Chen, Y. M., Qi, L., Liang, G. Q., Wang, Y., Zhang, L. P., et al. (2019). Hypoxia regulates angeogenic-osteogenic coupling process via up-regulating IL-6 and IL-8 in human osteoblastic cells through hypoxia-inducible factor 1 alpha pathway. Cytokine 113, 117–127. doi:10.1016/j.cyto.2018.06.022
Nourmohammadi, J., Roshanfar, F., Farokhi, M., and Nazarpak, M. H. (2017). Silk fibroin/kappa-carrageenan composite scaffolds with enhanced biomimetic mineralization for bone regeneration applications. Mater. Sci. Eng. C 76, 951–958. doi:10.1016/j.msec.2017.03.166
Pan, H., Zhang, Y. P., Shao, H. L., Hu, X. C., Li, X. H., Tian, F., et al. (2014). Nanoconfined crystallites toughen artificial silk. J. Mat. Chem. B 2 (10), 1408–1414. doi:10.1039/c3tb21148g
Panda, N. N., Biswas, A., Pramanik, K., and Jonnalagadda, S. (2015). Enhanced osteogenic potential of human mesenchymal stem cells on electrospun nanofibrous scaffolds prepared from eri-tasar silk fibroin. J. Biomed. Mat. Res. 103, 971–982. doi:10.1002/jbm.b.33272
Park, J.-Y., Yang, C., Jung, I.-H., Lim, H.-C., Lee, J.-S., Jung, U.-W., et al. (2015). Regeneration of rabbit calvarial defects using cells-implanted nano-hydroxyapatite coated silk scaffolds. Biomater. Res. 19, 7. doi:10.1186/s40824-015-0027-1
Partlow, B. P., Hanna, C. W., Rnjak-Kovacina, J., Moreau, J. E., Applegate, M. B., Burke, K. A., et al. (2014). Highly tunable elastomeric silk biomaterials. Adv. Funct. Mat. 24 (29), 4615–4624. doi:10.1002/adfm.201400526
Patil, S., and Singh, N. (2019). Silk fibroin-alginate based beads for human mesenchymal stem cell differentiation in 3D. Biomater. Sci. 7 (11), 4687–4697. doi:10.1039/c9bm01000a
Perteghella, S., Martella, E., De Girolamo, L., Orfei, C. P., Pierini, M., Fumagalli, V., et al. (2017). Fabrication of innovative silk/alginate microcarriers for mesenchymal stem cell delivery and tissue regeneration. Int. J. Mol. Sci. 18 (9), 1829. doi:10.3390/ijms18091829
Piluso, S., Flores Gomez, D., Dokter, I., Moreira Texeira, L., Li, Y., Leijten, J., et al. (2020). Rapid and cytocompatible cell-laden silk hydrogel formation via riboflavin-mediated crosslinking. J. Mat. Chem. B 8 (41), 9566–9575. doi:10.1039/d0tb01731k
Raia, N. R., Partlow, B. P., Mcgill, M., Kimmerling, E. P., Ghezzi, C. E., and Kaplan, D. L. (2017). Enzymatically crosslinked silk-hyaluronic acid hydrogels. Biomaterials 131, 58–67. doi:10.1016/j.biomaterials.2017.03.046
Rameshbabu, A. P., Bankoti, K., Datta, S., Subramani, E., Apoorva, A., Ghosh, P., et al. (2020). Bioinspired 3D porous human placental derived extracellular matrix/silk fibroin sponges for accelerated bone regeneration. Mater. Sci. Eng. C 113, 110990. doi:10.1016/j.msec.2020.110990
Ribeiro, M., De Moraes, M. A., Beppu, M. M., Garcia, M. P., Fernandes, M. H., Monteiro, F. J., et al. (2015). Development of silk fibroin/nanohydroxyapatite composite hydrogels for bone tissue engineering. Eur. Polym. J. 67, 66–77. doi:10.1016/j.eurpolymj.2015.03.056
Ribeiro, M., Fernandes, M. H., Beppu, M. M., Monteiro, F. J., and Ferraz, M. P. (2018). Silk fibroin/nanohydroxyapatite hydrogels for promoted bioactivity and osteoblastic proliferation and differentiation of human bone marrow stromal cells. Mater. Sci. Eng. C 89, 336–345. doi:10.1016/j.msec.2018.04.034
Ribeiro, V. P., Almeida, L. R., Martins, A. R., Pashkuleva, I., Marques, A. P., Ribeiro, A. S., et al. (2016). Influence of different surface modification treatments on silk biotextiles for tissue engineering applications. J. Biomed. Mat. Res. 104 (3), 496–507. doi:10.1002/jbm.b.33400
Ribeiro, V. P., Pina, S., Costa, J. B., Cengiz, I. F., Garcia-Fernandez, L., Fernandez-Gutierrez, M. D., et al. (2019). Enzymatically cross-linked silk fibroin-based hierarchical scaffolds for osteochondral regeneration. ACS Appl. Mat. Interfaces 11 (4), 3781–3799. doi:10.1021/acsami.8b21259
Roohaniesfahani, I., Wang, J., No, Y. J., De Candia, C., Miao, X. C., Lu, Z. F., et al. (2019). Modulatory effect of simultaneously released magnesium, strontium, and silicon ions on injectable silk hydrogels for bone regeneration. Mater. Sci. Eng. C 94, 976–987. doi:10.1016/j.msec.2018.10.053
Sahu, N., Baligar, P., Midha, S., Kundu, B., Bhattacharjee, M., Mukherjee, S., et al. (2015). Nonmulberry silk fibroin scaffold shows superior osteoconductivity than mulberry silk fibroin in calvarial bone regeneration. Adv. Healthc. Mat. 4 (11), 1709–1721. doi:10.1002/adhm.201500283
Samal, S. K., Dash, M., Shelyakova, T., Declercq, H. A., Uhlarz, M., Banobre-Lopez, M., et al. (2015). Biomimetic magnetic silk scaffolds. ACS Appl. Mat. Interfaces 7, 6282–6292. doi:10.1021/acsami.5b00529
Sartika, D., Wang, C. H., Wang, D. H., Cherng, J. H., Chang, S. J., Fan, G. Y., et al. (2020). Human adipose-derived mesenchymal stem cells-incorporated silk fibroin as a potential bio-scaffold in guiding bone regeneration. Polym. (Basel). 12 (4), 853. doi:10.3390/polym12040853
Sayin, E., Rashid, R. H., Rodriguez-Cabello, J. C., Elsheikh, A., Baran, E. T., and Hasirci, V. (2017). Human adipose derived stem cells are superior to human osteoblasts (HOB) in bone tissue engineering on a collagen-fibroin-ELR blend. Bioact. Mat. 2, 71–81. doi:10.1016/j.bioactmat.2017.04.001
Shao, W. L., He, J. X., Sang, F., Ding, B., Chen, L., Cui, S. Z., et al. (2016). Coaxial electrospun aligned tussah silk fibroin nanostructured fiber scaffolds embedded with hydroxyapatite-tussah silk fibroin nanoparticles for bone tissue engineering. Mater. Sci. Eng. C 58, 342–351. doi:10.1016/j.msec.2015.08.046
Sharma, A., Desando, G., Petretta, M., Chawla, S., Bartolotti, I., Manferdini, C., et al. (2019). Investigating the role of sustained calcium release in silk-gelatin-based three-dimensional bioprinted constructs for enhancing the osteogenic differentiation of human bone marrow derived mesenchymal stromal cells. ACS Biomater. Sci. Eng. 5 (3), 1518–1533. doi:10.1021/acsbiomaterials.8b01631
Sharma, S., Bano, S., Ghosh, A. S., Mandal, M., Kim, H. W., Dey, T., et al. (2016). Silk fibroin nanoparticles support in vitro sustained antibiotic release and osteogenesis on titanium surface. Nanomedicine Nanotechnol. Biol. Med. 12 (5), 1193–1204. doi:10.1016/j.nano.2015.12.385
Shen, K., Duan, A., Cheng, J. Q., Yuan, T., Zhou, J. C., Song, H. H., et al. (2022). Exosomes derived from hypoxia preconditioned mesenchymal stem cells laden in a silk hydrogel promote cartilage regeneration via the miR-205-5p/PTEN/AKT pathway. Acta Biomater. 143, 173–188. doi:10.1016/j.actbio.2022.02.026
Shen, X. F., Zhang, Y. X., Gu, Y., Xu, Y., Liu, Y., Li, B., et al. (2016). Sequential and sustained release of SDF-1 and BMP-2 from silk fibroin-nanohydroxyapatite scaffold for the enhancement of bone regeneration. Biomaterials 106, 205–216. doi:10.1016/j.biomaterials.2016.08.023
Shi, P. J., Teh, T. K. H., Toh, S. L., and Goh, J. C. H. (2013). Variation of the effect of calcium phosphate enhancement of implanted silk fibroin ligament bone integration. Biomaterials 34 (24), 5947–5957. doi:10.1016/j.biomaterials.2013.04.046
Shi, W. L., Sun, M. Y., Hu, X. Q., Ren, B., Cheng, J., Li, C. X., et al. (2017). Structurally and functionally optimized silk-fibroin-gelatin scaffold using 3D printing to repair cartilage injury in vitro and in vivo. Adv. Mat. 29, 1701089. doi:10.1002/adma.201701089
Shuai, Y. J., Mao, C. B., and Yang, M. Y. (2018). Protein nanofibril assemblies templated by graphene oxide nanosheets accelerate early cell adhesion and induce osteogenic differentiation of human mesenchymal stem cells. ACS Appl. Mat. Interfaces 10 (38), 31988–31997. doi:10.1021/acsami.8b11811
Smeets, R., Knabe, C., Kolk, A., Rheinnecker, M., Grobe, A., Heiland, M., et al. (2017). Novel silk protein barrier membranes for guided bone regeneration. J. Biomed. Mat. Res. 105 (8), 2603–2611. doi:10.1002/jbm.b.33795
Song, J., Kim, J., Woo, H. M., Yoon, B., Park, H., Park, C., et al. (2018). Repair of rabbit radial bone defects using bone morphogenetic protein-2 combined with 3D porous silk fibroin/-tricalcium phosphate hybrid scaffolds. J. Biomaterials Sci. Polym. Ed. 29 (6), 716–729. doi:10.1080/09205063.2018.1438126
Song, J. Y., Kim, S. G., Lee, J. W., Chae, W. S., Kweon, H., Jo, Y. Y., et al. (2011). Accelerated healing with the use of a silk fibroin membrane for the guided bone regeneration technique. Oral Surg. Oral Med. Oral Pathology Oral Radiology Endodontology 112 (6), e26–e33. doi:10.1016/j.tripleo.2011.05.002
Song, Y., Wu, H., Gao, Y., Li, J. Q., Lin, K. F., Liu, B., et al. (2020). Zinc silicate/nano-hydroxyapatite/collagen scaffolds promote angiogenesis and bone regeneration via the p38 MAPK pathway in activated monocytes. ACS Appl. Mat. Interfaces 12 (14), 16058–16075. doi:10.1021/acsami.0c00470
Soundarya, S. P., Menon, A. H., Chandran, S. V., and Selvamurugan, N. (2018). Bone tissue engineering: Scaffold preparation using chitosan and other biomaterials with different design and fabrication techniques. Int. J. Biol. Macromol. 119, 1228–1239. doi:10.1016/j.ijbiomac.2018.08.056
Sun, W., Motta, A., Shi, Y., Seekamp, A., Schmidt, H., Gorb, S. N., et al. (2016). Co-culture of outgrowth endothelial cells with human mesenchymal stem cells in silk fibroin hydrogels promotes angiogenesis. Biomed. Mat. 11 (3), 035009. doi:10.1088/1748-6041/11/3/035009
Tanasa, E., Zaharia, C., Hudita, A., Radu, I. C., Costache, M., and Galateanu, B. (2020). Impact of the magnetic field on 3T3-E1 preosteoblasts inside SMART silk fibroin-based scaffolds decorated with magnetic nanoparticles. Mater. Sci. Eng. C 110, 110714. doi:10.1016/j.msec.2020.110714
Teimouri, A., Ebrahimi, R., Emadi, R., Beni, B. H., and Chermahini, A. N. (2015). Nano-composite of silk fibroin-chitosan/Nano ZrO2 for tissue engineering applications: Fabrication and morphology. Int. J. Biol. Macromol. 76, 292–302. doi:10.1016/j.ijbiomac.2015.02.023
Thrivikraman, G., Athirasala, A., Gordon, R., Zhang, L. M., Bergan, R., Keene, D. R., et al. (2019). Rapid fabrication of vascularized and innervated cell-laden bone models with biomimetic intrafibrillar collagen mineralization. Nat. Commun. 10 (1), 3520. doi:10.1038/s41467-019-11455-8
Thurber, A. E., Omenetto, F. G., and Kaplan, D. L. (2015). In vivo bioresponses to silk proteins. Biomaterials 71, 145–157. doi:10.1016/j.biomaterials.2015.08.039
Uchida, R., Bhawal, U. K., Kiba, H., Arai, K., Tanimoto, Y., Kuboyama, N., et al. (2014). Effect of plasma-irradiated silk fibroin in bone regeneration. J. Biosci. Bioeng. 118 (3), 333–340. doi:10.1016/j.jbiosc.2014.02.016
Uebersax, L., Apfel, T., Nuss, K. M. R., Vogt, R., Kim, H. Y., Meinel, L., et al. (2013). Biocompatibility and osteoconduction of macroporous silk fibroin implants in cortical defects in sheep. Eur. J. Pharm. Biopharm. 85 (1), 107–118. doi:10.1016/j.ejpb.2013.05.008
Valarmathi, N., and Sumathi, S. (2020). Biomimetic hydroxyapatite/silkfibre/methylcellulose composites for bone tissue engineering applications. New J. Chem. 44, 4647–4663. doi:10.1039/C9NJ05592D
Varkey, A., Venugopal, E., Sugumaran, P., Janarthanan, G., Pillai, M. M., Rajendran, S., et al. (2015). Impact of silk fibroin-based scaffold structures on human osteoblast MG63 cell attachment and proliferation. Int. J. Nanomedicine 10 (1), 43–51. doi:10.2147/IJN.S82209
Vetsch, J. R., Paulsen, S. J., Muller, R., and Hofmann, S. (2015). Effect of fetal bovine serum on mineralization in silk fibroin scaffolds. Acta Biomater. 13, 277–285. doi:10.1016/j.actbio.2014.11.025
Wang, C. X., Fang, H., Qi, X. Y., Hang, C. J., Sun, Y. R., Peng, Z. B., et al. (2019). Silk fibroin film-coated MgZnCa alloy with enhanced in vitro and in vivo performance prepared using surface activation. Acta Biomater. 91, 99–111. doi:10.1016/j.actbio.2019.04.048
Wang, F. P., Pang, Y. N., Chen, G. B., Wang, W. W., and Chen, Z. M. (2020a). Enhanced physical and biological properties of chitosan scaffold by silk proteins cross-linking. Carbohydr. Polym. 229, 115529. doi:10.1016/j.carbpol.2019.115529
Wang, J. L., Wang, L. N., Zhou, Z. Y., Lai, H. J., Xu, P., Liao, L., et al. (2016). Biodegradable polymer membranes applied in guided bone/tissue regeneration: A review. Polym. (Basel). 8 (4), 115. doi:10.3390/polym8040115
Wang, K. K., Cheng, W. N., Ding, Z. Z., Xu, G., Zheng, X., Li, M. R., et al. (2021). Injectable silk/hydroxyapatite nanocomposite hydrogels with vascularization capacity for bone regeneration. J. Mat. Sci. Technol. 63, 172–181. doi:10.1016/j.jmst.2020.02.030
Wang, L., Fang, M., Xia, Y. J., Hou, J. X., Nan, X. R., Zhao, Z., et al. (2020b). Preparation and biological properties of silk fibroin/nano-hydroxyapatite/graphene oxide scaffolds with an oriented channel-like structure. RSC Adv. 10 (17), 10118–10128. doi:10.1039/c9ra09710d
Wang, Q., Zhang, Y. X., Li, B., and Chen, L. (2017). Controlled dual delivery of low doses of BMP-2 and VEGF in a silk fibroin-nanohydroxyapatite scaffold for vascularized bone regeneration. J. Mat. Chem. B 5 (33), 6963–6972. doi:10.1039/c7tb00949f
Wang, S. D., Ma, Q., Wang, K., and Chen, H. W. (2018). Improving antibacterial activity and biocompatibility of bioinspired electrospinning silk fibroin nanofibers modified by graphene oxide. Acs Omega 3 (1), 406–413. doi:10.1021/acsomega.7b01210
Wang, Y. Q., Yao, D. Y., Li, L. H., Qian, Z. Y., He, W., Ding, R., et al. (2020d). Effect of electrospun silk fibroin-silk sericin films on macrophage polarization and vascularization. ACS Biomater. Sci. Eng. 6 (6), 3502–3512. doi:10.1021/acsbiomaterials.0c00175
Watchararot, T., Prasongchean, W., and Thongnuek, P. (2021). Angiogenic property of silk fibroin scaffolds with adipose-derived stem cells on chick chorioallantoic membrane. R. Soc. open Sci. 8 (3), 201618. doi:10.1098/rsos.201618
Wenhao, Z., Zhang, T., Yan, J. L., Li, Q. Y., Xiong, P. P., Li, Y. Y., et al. (2020). In vitro and in vivo evaluation of structurally-controlled silk fibroin coatings for orthopedic infection and in-situ osteogenesis. Acta Biomater. 116, 223–245. doi:10.1016/j.actbio.2020.08.040
Wenk, E., Merkle, H. P., and Meinel, L. (2011). Silk fibroin as a vehicle for drug delivery applications. J. Control. Release 150 (2), 128–141. doi:10.1016/j.jconrel.2010.11.007
Wongpinyochit, T., Johnston, B. F., and Seib, F. P. (2018). Degradation behavior of silk nanoparticles-enzyme responsiveness. ACS Biomater. Sci. Eng. 4 (3), 942–951. doi:10.1021/acsbiomaterials.7b01021
Wu, H., Zhao, C. C., Lin, K. L., and Wang, X. D. (2022a). Mussel-inspired polydopamine-based multilayered coatings for enhanced bone formation. Front. Bioeng. Biotechnol. 10, 952500. doi:10.3389/fbioe.2022.952500
Wu, R. J., Li, H. T., Yang, Y. L., Zheng, Q. J., Li, S. L., and Chen, Y. F. (2021a). Bioactive silk fibroin-based hybrid biomaterials for musculoskeletal engineering: Recent progress and perspectives. ACS Appl. Bio Mat. 4 (9), 6630–6646. doi:10.1021/acsabm.1c00654
Xiong, J. H., Onal, M., Jilka, R. L., Weinstein, R. S., Manolagas, S. C., and O'brien, C. A. (2011). Matrix-embedded cells control osteoclast formation. Nat. Med. 17 (10), 1235–1241. doi:10.1038/nm.2448
Yan, L. P., Silva-Correia, J., Oliveira, M. B., Vilela, C., Pereira, H., Sousa, R. A., et al. (2015). Bilayered silk/silk-nanoCaP scaffolds for osteochondral tissue engineering: In vitro and in vivo assessment of biological performance. Acta Biomater. 12, 227–241. doi:10.1016/j.actbio.2014.10.021
Yan, Y. F., Chen, H., Zhang, H. B., Guo, C. J., Yang, K., Chen, K. Z., et al. (2019). Vascularized 3D printed scaffolds for promoting bone regeneration. Biomaterials 190, 97–110. doi:10.1016/j.biomaterials.2018.10.033
Zafar, B., Mottaghitalab, F., Shahosseini, Z., Negahdari, B., and Farokhi, M. (2020). Silk fibroin/alumina nanoparticle scaffold using for osteogenic differentiation of rabbit adipose-derived stem cells. Materialia 9, 100518. doi:10.1016/j.mtla.2019.100518
Zaharia, C., Tudora, M. R., Stancu, I. C., Galateanu, B., Lungu, A., and Cincu, C. (2012). Characterization and deposition behavior of silk hydrogels soaked in simulated body fluid. Mater. Sci. Eng. C 32, 945–952. doi:10.1016/j.msec.2012.02.018
Zarrin, N. K., Mottaghitalab, F., Reis, R. L., Kundu, S. C., and Farokhi, M. (2022). Thermosensitive chitosan/poly(N-isopropyl acrylamide) nanoparticles embedded in aniline pentamer/silk fibroin/polyacrylamide as an electroactive injectable hydrogel for healing critical-sized calvarial bone defect in aging rat model. Int. J. Biol. Macromol. 213, 352–368. doi:10.1016/j.ijbiomac.2022.05.176
Zhang, F., and King, M. W. (2020). Biodegradable polymers as the pivotal player in the design of tissue engineering scaffolds. Adv. Healthc. Mat. 9 (13), e1901358. doi:10.1002/adhm.201901358
Zhang, H. J., You, R. C., Yan, K., Lu, Z. T., Fan, Q. M., Li, X. F., et al. (2020). Silk as templates for hydroxyapatite biomineralization: A comparative study of Bombyx mori and Antheraea pernyi silkworm silks. Int. J. Biol. Macromol. 164, 2842–2850. doi:10.1016/j.ijbiomac.2020.08.142
Zhang, H. P., Liu, X. T., Yang, M. Y., and Zhu, L. J. (2015). Silk fibroin/sodium alginate composite nano-fibrous scaffold prepared through thermally induced phase-separation (TIPS) method for biomedical applications. Mater. Sci. Eng. C 55, 8–13. doi:10.1016/j.msec.2015.05.052
Zhang, L., Zhang, W., Hu, Y. J., Fei, Y., Liu, H. Y., Huang, Z. Z., et al. (2021). Systematic review of silk scaffolds in musculoskeletal tissue engineering applications in the recent decade. ACS Biomater. Sci. Eng. 7 (3), 817–840. doi:10.1021/acsbiomaterials.0c01716
Zhang, X. Y., Chen, Y. P., Han, J., Mo, J., Dong, P. F., Zhuo, Y. H., et al. (2019). Biocompatiable silk fibroin/carboxymethyl chitosan/strontium substituted hydroxyapatite/cellulose nanocrystal composite scaffolds for bone tissue engineering. Int. J. Biol. Macromol. 136, 1247–1257. doi:10.1016/j.ijbiomac.2019.06.172
Zhou, B. G., He, M., Wang, P., Fu, H. T., Yu, Y. Y., Wang, Q., et al. (2017a). Synthesis of silk fibroin-g-PAA composite using H2O2-HRP and characterization of the in situ biomimetic mineralization behavior. Mater. Sci. Eng. C 81, 291–302. doi:10.1016/j.msec.2017.08.006
Zhou, B. G., Zhou, Q., Wang, P., Yuan, J. G., Yu, Y. Y., Deng, C., et al. (2018). HRP-mediated graft polymerization of acrylic acid onto silk fibroins and in situ biomimetic mineralization. J. Mat. Sci. Mat. Med. 29, 72. doi:10.1007/s10856-018-6084-y
Zhou, W. H., Jia, Z. J., Xiong, P., Yan, J. L., Li, Y. Y., Li, M., et al. (2017b). Bioinspired and biomimetic AgNPs/gentamicin-embedded silk fibroin coatings for robust antibacterial and osteogenetic applications. ACS Appl. Mat. Interfaces 9 (31), 25830–25846. doi:10.1021/acsami.7b06757
Keywords: silk fibroin (SF), bone regeneration, scaffolds, biocompatibility, biodegradability, osteogenesis
Citation: Wu H, Lin K, Zhao C and Wang X (2022) Silk fibroin scaffolds: A promising candidate for bone regeneration. Front. Bioeng. Biotechnol. 10:1054379. doi: 10.3389/fbioe.2022.1054379
Received: 26 September 2022; Accepted: 17 November 2022;
Published: 25 November 2022.
Edited by:
Y. Shrike Zhang, Harvard Medical School, United StatesReviewed by:
Xiaolin Cui, The Chinese University of Hong Kong, Shenzhen, ChinaCopyright © 2022 Wu, Lin, Zhao and Wang. This is an open-access article distributed under the terms of the Creative Commons Attribution License (CC BY). The use, distribution or reproduction in other forums is permitted, provided the original author(s) and the copyright owner(s) are credited and that the original publication in this journal is cited, in accordance with accepted academic practice. No use, distribution or reproduction is permitted which does not comply with these terms.
*Correspondence: Cancan Zhao, NjE3MTY3NDY0QHFxLmNvbQ==; Xudong Wang, eHVkb25nd2FuZzcwQGhvdG1haWwuY29t
Disclaimer: All claims expressed in this article are solely those of the authors and do not necessarily represent those of their affiliated organizations, or those of the publisher, the editors and the reviewers. Any product that may be evaluated in this article or claim that may be made by its manufacturer is not guaranteed or endorsed by the publisher.
Research integrity at Frontiers
Learn more about the work of our research integrity team to safeguard the quality of each article we publish.