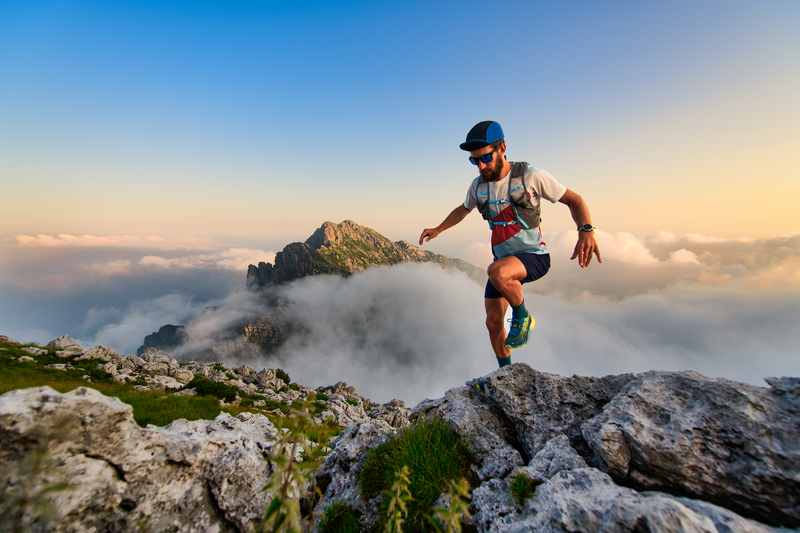
95% of researchers rate our articles as excellent or good
Learn more about the work of our research integrity team to safeguard the quality of each article we publish.
Find out more
REVIEW article
Front. Bioeng. Biotechnol. , 17 October 2022
Sec. Nanobiotechnology
Volume 10 - 2022 | https://doi.org/10.3389/fbioe.2022.1032041
This article is part of the Research Topic Design and Synthesis of Nanocarriers for Enhancement of Antigen Immunogenicity View all 7 articles
Microneedles have garnered significant interest as transdermal drug delivery route owing to the advantages of nonselective loading capacity, minimal invasiveness, simple operation, and good biocompatibility. A number of therapeutics can be loaded into microneedles, including hydrophilic and hydrophobic small molecular drugs, and macromolecular drugs (proteins, mRNA, peptides, vaccines) for treatment of miscellaneous diseases. Microneedles feature with special benefits for cutaneous diseases owing to the direct transdermal delivery of therapeutics to the skin. This review mainly introduces microneedles fabricated with different technologies and transdermal delivery of various therapeutics for cutaneous diseases, such as psoriasis, atopic dermatitis, skin and soft tissue infection, superficial tumors, axillary hyperhidrosis, and plantar warts.
Skin, accounting for 1.2–2.0 square meters of the body and 16% of the bodyweight (Li S. et al., 2020), is the largest organ composed of several layers (epidermis, dermis, subcutis) that protect the body from invasion by foreign substances (Yin and Smith, 2016). These protective layers also provide natural barriers for drugs that are used to treat various deep tissue and dermatologic diseases. The stratum corneum (SC), comprising approximately 15–20 layers of specialized anucleated keratino-cytes, resides within the most superficial layer of the epidermis (Menon et al., 2012), forming a major barrier against the external environment. Therapeutic penetration through this layer is determined by the lipid solubility and molecular weight of the drugs; the transdermal delivery ability of hydrophilic drugs and drugs with a molecular weight higher than 500 Da is very limited (Mishra and Bonde, 2020).
Development of delivery systems that intend to aid penetration of such drugs through deeper layers has been increasing in the past 20 years, extending the applications of these hydrophilic and macromolecule drugs for the treatment of deep tissue and dermatologic diseases. Many physical or pharmaceutical strategies, such as sonophoresis, electroporation, iontophoresis, microneedles (MNs), chemical enhancers, and transdermal formulations, have been developed for transdermal drug delivery (TDD) of these inaccessible hydrophilic and macromolecule drugs, referring to drug administration for local dermatosis or systemic therapy purposes (Pan et al., 2020). These TDD strategies can facilitate the transdermal absorption of a series of hydrophilic or macromolecule drugs, presenting advantages with bypass of gastrointestinal stimuli and degradation, avoidance of first-pass elimination, and improved patient compliance.
Of note, the microneedle-mediated TDD system has gained significant attention, presenting a superior drug delivery route to topical lesions with enhanced transdermal efficiency, microneedles have natural advantages with nonspecific drug loading ability and direct therapeutic delivery route. A number of drugs, including small molecular drugs (Chiang et al., 2016), macromolecular drugs (Prausnitz et al., 2019), vaccines (Waghule et al., 2019), and nano-particles (Du et al., 2017) have been loaded into microneedles to treat various diseases. Drug-loaded microneedles can temporarily break the stratum corneum for enhanced skin permeability and subsequently improve drug penetration efficiency for better therapeutic outcomes. Research in microneedles has been significantly increased in the past 10 years (Figure 1), including manufacturing techniques, microneedle-mediated delivery systems for cancer and diabetes treatment, vaccination, and various skin diseases. Many reviews have summarized advances in the microneedle-mediated delivery system for cancer and diabetes treatment. Accordingly, in this review, we would introduce the applications of the microneedle-mediated drug delivery (MDD) system for treatment of dermatological diseases, including psoriasis, atopic dermatitis, skin and soft tissue infections, superficial tumors, axillary hyperhidrosis, and plantar warts.
FIGURE 1. Number of publications on microneedle based on PubMed database (https://www.ncbi.nlm.nih.gov/pubmed/) and Web of Science (http://apps.webofknowledge.com) on 12 November 2021.
Microneedle, containing a cavity for drug reservoir and a tiny protrusion for transdermal penetration, was first patented in 1971 (Martin and Gerstel, 1971). Since then, microneedles have been extensively developed for TDD along with advances in microfabrication manufacturing and pharmaceutics technologies. According to differences in drug delivery pattern, microneedles can be divided into solid, hollow, dissolved, or coated microneedles (Table 1).
The solid microneedle was typically used for pretreatment of the skin prior to administration of active ingredients (Figure 2A). Common materials used for the manufacture of solid microneedles primarily include silicon (Hashmi et al., 1995; Wilke et al., 2005; Narayanan and Raghavan, 2017, 2019), methyl vinyl ether and maleic anhydride (PMVE/MA) (Donnelly et al., 2011), polymethyl methacrylate (PMMA) (Moon et al., 2005), polylactic acid (PLA) (Li Q. Y. et al., 2017), as well as stainless steel (Martanto et al., 2004), titanium (Matriano et al., 2002), nickel (Jung et al., 2008) and other metallic materials. Solid microneedles can be applied for TDD (Table 2) both with or without drug coating. Non-drug-coated solid microneedles can create transient skin microchannels and enhance drug penetration efficiency for the following topical administration (Li et al., 2017b). Drug-coated solid microneedles function as both skin penetration modules and drug reservoirs. Though rapid delivery of active ingredients can be facilitated using this system, their limitations including low drug loading capacity, limited biological compatibility, and inaccurate dose administration need to be carefully considered (Li et al., 2017a).
FIGURE 2. Types of microneedles. (A) Solid microneedles. Reproduced with permission (Henry et al., 1998). Copyright 1998, Elsevier. (B) Hollow microneedles. Reproduced with permission (Martanto et al., 2006). Copyright 2006, Springer Nature. (C) Dissolving microneedles. Reproduced with permission (Lee et al., 2008). Copyright 2008, Elsevier. (D) Coated microneedles. Copyright 2007, Springer Nature.
Different from solid microneedle with a solid structure, hollow microneedle has a lumen or internal pore with a diameter approximately in the range of 50–70 μm, enabling the loading of therapeutic drugs or active solutions (Figure 2B). More content can be loaded in the lumen or internal pore of this type of microneedle than that of other microneedles. Relative to solid microneedles, the manufacturing of hollow microneedles is more complicated. The manufacturing methods include laser micromachining (Davis et al., 2005), integrated photolithography technique (Luttge et al., 2007), and micromachining (Ma et al., 2006). Hollow microneedles have been fabricated out of various materials, including silicon (Gardeniers et al., 2003), glass (Wang et al., 2006), polymers (Miller et al., 2011), metals (Lee et al., 2010). Hollow microneedles are suitable for the delivery of a variety of active molecules with relatively high drug loading capacity and accurate administration doses. However, the disadvantages include low mechanical strength and possibility of clogging and infection due to repeated application.
The dissolving microneedles are typically made of water-soluble biodegradable materials, and the drug can be packaged into biodegradable materials (Figure 2C). After inserting the microneedles into the skin, the biodegradable materials dissolve and release the payload drugs (Martanto et al., 2004). Maltose (Zhang Y. et al., 2018), carboxymethylcellulose (Park and Kim, 2017), hyaluronic acid (HA) (Zhu J. et al., 2019), and other degradable materials are often used to fabricate dissolving microneedles. In contrast to other types of microneedles, dissolving microneedles are easily manufactured, and the manufacturing techniques mainly include photopolymerization (Sullivan et al., 2008), drawing photolithography (Lee and Jung, 2012), and microstructure (Shim et al., 2018). Good biocompatibility and solubility of dissolving microneedles have significantly improved patient compliance and provided new options for long-term treatment (Ita, 2015). Owing to these advantages, a variety of dissolving microneedles are being extensively investigated for the treatment of various diseases, such as cancer (Wang et al., 2016), diabetes (Zhang et al., 2019), alopecia (Cao et al., 2021), and other skin diseases (Yang et al., 2013; Du et al., 2019; Nawrocki and Cha, 2019; Song et al., 2020). However, the mechanical strength of dissolving microneedles is usually not high enough (Ita, 2017), which may influence their ability to penetrate the skin. This may inhibit complete penetration of the drugs, resulting in waste of drugs and reduced therapeutic outcomes (Lau et al., 2017).
Coated microneedles refer to solid microneedle coated with therapeutic contents. Dipping and spraying are the two commonly used coating methods (Figure 2D). In the dipping method, the microneedle is immersed in a target coating solution to load the active content (Kapoor et al., 2020). In the spraying method, atomizer (McGrath et al., 2011) or gas jet injection (Kim et al., 2018) is applied to cover the surface of the microneedle with active ingredients. The former method can position the coating on the surface of the microneedle or only on the tip of the microneedle without polluting the base substrate. However, the latter may contaminate the substrate during the manufacturing process due to inaccurate spraying. Small-molecule drugs such as lidocaine (Ita, 2018), pilocarpine (Jiang et al., 2007), and fluorescein (Baek et al., 2017) as well as macromolecules, such as insulin (Halder et al., 2020), parathyroid hormone (1–34) (Naito et al., 2018), and hepatitis B surface antigen (Na et al., 2020) have been successfully loaded with coated microneedles.
Previous studies have primarily focused on the manufacturing technologies of microneedles and their drug loading capabilities; penetration mechanics of microneedles have been often ignored. The delivery of accumulated drugs is proportional to the surface area or lumen value of microneedles, and surface area is related to the length of microneedles. Since skin is elastic, certain resistance is encountered during insertion of a microneedle (Figure 3). When the protrusion of the microneedle is too long or not strong enough, it may break during insertion into the skin (Al-Qallaf and Das, 2008). The injury induced by physical stimulation during penetration is another necessary consideration. Generally, microneedle with a length of 50–200 µm is painless. However, when the length exceeds a certain threshold, it will prick the skin, and the penetration may be unpleasant (Gill et al., 2008; Arya et al., 2017). The longer the microneedle, the higher the pain. Additionally, the geometric shape of the microneedle is very important to the insertion and penetration for drug delivery (Davis et al., 2004). If the ratio of the breaking force and insertion force is greater than 1, the microneedle can be inserted into the skin but not fractured. Thus, for maximum safety margin, the use of microneedles with small tip radius has been suggested to promote insertion and sufficient thickness to provide strength.
FIGURE 3. The relationship between needle and skin at different stages of insertion: (A) pre-puncture, (B) puncture, and (C) post-puncture. The microneedles are applied to the skin (D), and then used for drug delivery (E). Copyright 2017, Elsevier.
Psoriasis is a chronic inflammatory skin disease associated with a variety of complications that significantly lower the quality of life of patients. Approximately 125 million individuals have been diagnosed with psoriasis globally (Michalek et al., 2017; Sabri et al., 2019). The pathogenesis of psoriasis is complex and has not been fully elucidated. For patients with mild psoriasis, external drugs are still the primary form of treatment. For patients with plaque psoriasis, biologics that inhibit the production of TNF-α, p40IL-12/23, p19IL-23, and IL-17 are approved for efficient treatment (Armstrong and Read, 2020). However, these treatments can only alleviate symptoms, and no cure is known for psoriasis. Moreover, these treatments are often associated with serious side effects that worsen patient compliance (Kamata and Tada, 2018; Lockwood et al., 2018).
Methotrexate (MTX) is a widely used drug that could be administered via injection or oral routes to treat several types of cancers or to control severe psoriasis (Jolivet et al., 1983; Roenigk et al., 1988). However, adverse effects involving bone marrow inhibition, nausea, vomiting, anemia, and platelet reduction, limit its application in long-term treatment. A dissolved microneedle patch loaded with HA-encapsulated MTX for topical treatment of psoriasis was developed (Figure 4A) (Du et al., 2019). Benefiting from the highly hydrophilic HA, microneedles can dissolve in 10 min after insertion into the skin, accompanied by a triggered release of MTX for therapy. The transdermal drug release strategy involving dissolving microneedles avoids drug degradation in the gastrointestinal tract and first metabolism in the liver. MTX-loaded microneedles demonstrate higher effectiveness and reduced side effects than orally administered MTX. In addition, microneedles can also encapsulate macromolecular drugs. Dissolving microneedles containing non-soluble cyclosporin A (CyA MN) with high molecular weight for transdermal delivery of CyA were designed for the treatment of psoriasis (Jeong et al., 2018). Compared to that observed with oral routes, the pharmacokinetic behavior of CyA MN with transdermal delivery has been remarkably improved for sustained release of CyA during the dissolution of MN, with improved safety profile of CyA.
FIGURE 4. (A) Preparation of MTX-loaded dissoluble microneedles. Comparison of orally administered MTX and MTX loaded microneedles with transdermal administration on (B) left ear lesions, (C) H&E staining and (D) Ki67 IHC staining of skin sections (red arrows). Copyright 2019, American Chemical Society.
Atopic dermatitis (AD) is a common chronic inflammatory skin disease, which is a result of skin barrier dysfunction and dysregulation of immune dispensing (Kim et al., 2019). Currently, cortico-steroids are the most widely used drugs to treat AD; however, long-term usage of corticosteroids may result in skin atrophy, local burning, or itching (Torrelo, 2017). Integration of corticosteroids within dissolved microneedles could concentrate their therapeutic function for better treatment outcomes, thereby decreasing the possible side effects. For instance, triamcinolone acetonide (TA) was encapsulated in dissolving microneedles, which showed a better therapeutic outcome for the high drug loading dosage (Jang et al., 2021).
In addition, previous study showed that oral administration of poly-γ-glutamic acid (γ-PGA) could activate dendritic cells (DCs) and induce production of IL-12, which decelerated AD (Sung et al., 2005; Lee et al., 2014). To avoid degradation or destruction of γ-PGA with high molecular weight in the gastrointestinal tract after oral administration, a soluble poly-γ-PGA microneedle was designed for transdermal penetration to DC-rich skin layers for efficient modulation of immune responses (Figure 5A) (Chen et al., 2020). After treatment with high molecular weight poly-γ-PGA microneedle, the skin of AD mice could quickly restore its barrier function within 4 h without any apparent skin irritation (Figure 5B). This method demonstrated a better therapeutic outcome than low molecular weight poly-γ-PGA microneedle, owing to the transdermal capacity of microneedles and high molecular weight of poly-γ-PGA could retain in the skin for 6 days for effective treatment of AD (Figure 5C).
FIGURE 5. (A) Preparation of soluble high-molecule-wight poly-γ-PGA microneedles. (B) Poly-γ-PGA microneedles performing therapeutic effect within 4 h after transdermal administration. (C) Poly-γ-PGA microneedles could be retained in the skin for 6 days for sustained therapeutic effect. Copyright 2020, Elsevier.
Skin and soft-tissue infections (SSTIs) mainly involve simple or complicated (necrotizing or non-necrotizing) infections of the epidermis, luminous membrane, subcutaneous tissue, and muscles. Staphylococcus aureus (MRSA), fungi, β-hemolytic streptococcus, or Escherichia coli are common pathogens implicated in SSTI. Complicated SSTI (CSSTI) is a serious infectious disease involving complications such as sepsis, which requiring surgical intervention or drug treatment (Dryden, 2010; Leong et al., 2018). Broad-spectrum antibiotics and surgical drainage are commonly applied to treat SSTIs; however, some strains showed resistance to a variety of antibiotics (Dryden, 2010).
Therapeutic loaded transdermal microneedles can potentially mitigate these challenges (Jamaledin et al., 2020). Intravenous injection of the glycopeptide antibiotic vancomycin (VAN) was usually used to treat SSTIs caused by MRSA; however, its therapeutic efficiency was limited due to low delivery concentrations of VAN in the pathogen infected skin. Topical administration of VAN is ineffective as its high molecular weight hinders skin permeation. Sotiriou and others developed a VAN located microneedle array in the water-soluble needle tips (Figure 6A) (Ziesmer et al., 2021). The VAN-loaded microneedles could penetrate the thawed porcine and fresh human skin, triggering sustained release of a high dosage of loaded VAN within 24 h, thereby efficiently restraining MRSA (Figures 6B,C). In addition, skin fungal infection affects 20–25% global population (Havlickova et al., 2008). Fungal infections are more difficult to treat than bacterial infections owing to the presence of multiple organelles and thick and rigid cell walls that are resistant to lysis by therapeutics and innate immune response (Netea et al., 2008). To efficiently treat skin fungal infection, amphotericin B and antimicrobial chitosan-polyethyleneimine (CP) copolymer were integrated into microneedles. In addition to the absence of resistance against CP observed in pathogens, the biodegradable CP would also allow for sustained release of amphotericin B for continuous treatment after penetration into the skin, thereby facilitating synergistic effects of the antifungal polymer and amphotericin B (Zan et al., 2019). Small molecule drugs, such as doxycycline for Staphylococcus aureus and Pseudomonas aeruginosa (Permana et al., 2020), and carvacrol for multidrug-resistant bacteria (Mir et al., 2020) have also been loaded into microneedles for better therapeutic outcomes.
FIGURE 6. (A) Manufacturing process of VAN-loaded microneedle arrays. Application of MN arrays at same CFU counts (B) or same infection length (C). Copyright 2021, John Wiley and Sons.
In addition to the aforementioned traditional drugs, living bacteria could also be encapsulated within microneedles. Bacillus subtilis (B. subtilis)-encapsulated microneedles could prevent the escaping of B. subtilis and continuously secrete various antifungal agents, which can directly bind to fungal cell surface-associated proteins and destruct the fungal cell membranes without inducing drug resistance (Wang F. et al., 2020).
Plantar warts are a common skin disease observed at the plantar site caused by human papillomavirus (HPV) (Bacelieri and Johnson, 2005; Konicke and Olasz, 2016). Several methods, such as salicylic acid, cryotherapy, surgical resection, and laser can be used for plantar warts treatment (Bacelieri and Johnson, 2005). However, these treatments have limitations, such as pain induced by surgical intervention and continuous medication (Clifton et al., 2003; Saitta et al., 2008), and no single therapy can cure plantar warts completely.
Bleomycin is a traditional drug used for the treatment of warts. Intralesional bleomycin has been demonstrated as an effective choice for refractory warts, but with disadvantages of scarring induction, pigmentation, and nail damage (Bik et al., 2020). To relieve these side effects, microneedles with targeted spraying of bleomycin have demonstrated higher clearance and low pain compared to intralesional bleomycin (Saitta et al., 2008; Al-Naggar et al., 2019). In another study, bleomycin was directly coated on the tips of poly-lactic-acid (L-PLA) microneedles, which were fabricated by a molding process (Lee et al., 2017). Carboxymethyl-cellulose sodium salt (CMC) was used as a thickening agent on the tips of microneedles for loading more bleomycin (Figure 7). Assisted with CMC, bleomycin could be coated on the tips of microneedles with a high dosage (518 µg) and demonstrate high skin tolerance. More than 80% of coated bleomycin can be delivered into the skin, and a more concentrated bleomycin delivered into the subepidermis layer, which leading to improved therapeutic outcomes compared to those observed with intralesional injection.
FIGURE 7. (A) Schematic diagram of a cross-section of warts. (B) Insertion of the bleomycin-coated microneedle. (C) Transdermal delivery of bleomycin into warts. Copyright 2017, Springer Nature.
Primary axillary hyperhidrosis (PAH) is characterized by an increased amount of sweat production, specifically in the axilla, which can lead to social embarrassment, emotional and occupational distress (Lakraj et al., 2013; Hamm, 2014). Current treatments mainly include topical aluminum salts, iontophoresis, Botox therapy, and surgical sympathectomy (Hoorens and Ongenae, 2012). However, these treatment modalities are far from satisfactory (Doft et al., 2012). For instance, the disadvantage of using botulinum toxin is that about 20 painful intradermal injections must be repeated every 3 months.
To improve the administration procedure and therapeutic efficiency, Coulman and others reported a pocketed microneedle for delivery of liquid state botulinum toxin A formulation into human skin (Torrisi et al., 2013), demonstrating a potent therapeutic route for PAH. In addition, transdermal delivery of radiofrequency by fractional microneedle radiofrequency (FMR) could also present an alternative treatment strategy. As an effective treatment method for PAH, FMR could damage eccrine glands via thermolysis at the interface of the deep dermis and subcutis after transdermal penetration of FMR while minimizing side effects on the surrounding tissues (Kim et al., 2013; Zhang M. et al., 2018).
Superficial skin tumors (SST) are the most common tumors observed in human, mainly classified as hemangioma, actinic keratosis, and squamous cell carcinoma, which are caused by excessive proliferation and mutation of skin cells (Lomas et al., 2012; Grossman et al., 2018). Most traditional chemotherapeutic agents used for superficial tumors, such as docetaxel, cisplatin, and doxorubicin, are generally administered intravenously (Dalbagni, 2007; Gao et al., 2017). However, systemic administration of chemotherapeutics can induce many adverse effects, such as bone marrow suppression, neurotoxicity, and adverse gastrointestinal reactions (Heidary et al., 2008), which impair therapeutic outcomes and are very uncomfortable for patients.
Compared to systemic administration of chemotherapeutics, microneedle-mediated drug delivery systems demonstrate natural advantages for treating superficial skin tumors owing to the precise administration, local drug release, good biocompatibility, and less pain. The topical administration of chemotherapeutic encapsulated microneedles can greatly improve the targeting efficiency and reduce adverse effects. For example, cisplatin, as a first-line chemotherapeutic agent, may exhibit side effects of nephrotoxicity, neurotoxicity, ototoxicity, electrolyte disturbance, and hemolytic anemia (Loehrer And Einhorn, 1984; Minami et al., 2004; Barabas et al., 2008). To avoid these toxic effects, lipid-coated cisplatin nanoparticles (LCC-NPs) were formulated using tumor-targeting pH-responsive lipid nanoparticles and embedded into dissolvable microneedles for transdermal administration (Lan et al., 2018). After insertion into the skin, the nanoparticles can be locally delivered through the stratum corneum. In vivo study demonstrated that microneedle arrays significantly increased therapeutic results with reduced systemic toxicity and side effects. Microneedles are also used to synergistically treat superficial tumors with phototherapy (Chen et al., 2016; Dong et al., 2018; Tham et al., 2018; Song et al., 2020). Zhao and others developed a microneedle-assisted topical delivery system encapsulated with active mesoporous organosilica nanoparticles preconjugated with a photosensitizer (phthalocyanine) and simultaneously coated with small molecule inhibitors (dabrafenib and trametinib) (Figure 8A) (Tham et al., 2018). Microneedles could facilitate the penetration of active mesoporous organosilica nanoparticles to reach deep-seated melanoma sites. Assisted with NIR irradiation (Figure 8B), the drug-loaded microneedle platform showed a synergistic killing effect on skin cancer cells with reactive oxygen species induced by the photosensitizer and caspase-activated apoptosis for small-molecule inhibitors without systemic toxicity.
FIGURE 8. (A) Schematic illustration of co-loaded photosensitizers and small-molecule inhibitors into microneedles for synergistic therapy of deep-seated melanoma. (B) Antitumor effects for these transdermally delivered drugs. Copyright 2018, American Chemical Society.
Vaccination is considered one of the most important methods for protection against infectious diseases. As the most recent example, the coronavirus disease 2019 (COVID-19) pandemic caused by the novel Coronavirus strain SARS-CoV-2 is still raging (Cullen et al., 2020; Daniel, 2020; Donthu and Gustafsson, 2020; Korbel and Stegle, 2020; Van Bavel et al., 2020). Vaccines play an important role to reduce the infection spread and serious symptoms (Wang J. et al., 2020; Malik et al., 2020). Vaccine administration mainly includes traditional parenteral and mucosal routes, novel needle-free injection and adjuvant formulations (Zhang et al., 2015). The needle-based delivery system functions as the standard intradermal injection technique that involves the insertion of the tip into the skin and pushing the contents within a plastic 1 ml disposable syringe (Lambert and Laurent, 2008). For avoiding disadvantages of needle-based administrations, vaccine delivery systems facilitated by needle-free injection, liquid jet injectors, ballistic injectors, and microneedle injection have been developed (Giudice and Campbell, 2006; Kis et al., 2012; Hossain et al., 2020). Different delivery methods have different immune efficiency attributed to the priming of immune cells and subsequent immune response.
Convenient and favorable delivery system is very important for optimal potency of vaccines. Microneedle-based vaccine delivery platform has simplified vaccine distribution, improved patient compliance, and targeted vaccine delivery in the skin to activate antigen-presenting cells (APC) (Van der Maaden et al., 2012; Ma and Wu, 2017; Rodgers et al., 2018; Li Z. et al., 2020). In addition, compared with needle-based muscle injection, the immune memory reaction triggered by microneedle-based administration is enhanced with the continuous release of the vaccine loaded within the cavity of microneedles (Kim et al., 2010). For example, vaccines delivered with a hollow microneedle demonstrated enhanced immunity than subcutaneous injection (Ogai et al., 2018) as the accurately delivery of vaccine into the upper portion of the dermis, inducing enhanced immune responses. Owing to these advantages, microneedle-based vaccination has been widely studied for immunization against influenza (Rouphael et al., 2017; Zhu et al., 2018; Shin et al., 2020). Bis-(3′–5′)-cyclic dimeric guanosine monophosphate (GMP, a bacterial second messenger and stimulator of interferon gene agonist), which is a suitable adjuvant, and influenza vaccine were loaded into the microneedle and then transdermally delivered to the skin of the mice. The immunogenicity and protective effects after inoculation were assessed. Mice immunized with 2 μg of GMP and influenza viruses showed a higher level of IgG and systemic immune response than mice immunized alone. This finding demonstrated that simultaneous delivery of influenza vaccines and GMP adjuvant with microneedles could increase the immune efficiency of the vaccine. Granulocyte-macrophage colony-stimulating factor (GM-CSF) can also strengthen the antibody reaction of the influenza vaccine after co-loading into a dissolvable microneedle patch (Littauer et al., 2018), which improved the protective effects of the vaccine by promoting the proliferation of vaccine specific T cells (Figure 9).
FIGURE 9. Preparation of granulocyte-macrophage colony-stimulating factor (GM-CSF) loaded microneedles and immune response after transdermal delivery performed using GM-CSF-loaded microneedles. Copyright 2018, Elsevier.
Other vaccines can also be loaded with microneedles for efficient vaccination. A soluble microneedle manufactured using carboxymethyl cellulose and trehalose was used to load adenovirus vaccine (Ad5. OVA) together with toll-like receptor 3 (TLR3) agonist polyinosinic acid: polycytidylic acid [Poly (I:C)] (Erdos et al., 2020). After insertion into the skin, the microneedles can dissolve and release loaded adenovirus and Poly (I:C), resulting in overexpression of the OVA transgene and corresponding OVA mRNA. In addition, the Pastis toxin (PT) vaccine delivered by microneedles (Zhu D. D. et al., 2019), which can be successfully inserted into mice at a depth of 330 μm, which is abundant with immune cells. Compared to direct subcutaneous injection, the antigen release duration for mi-croneedle vaccination was longer, and less amount of PT antigen could elicit strong immune re-sponses. This vaccination route presented significant advantages over traditional subcutaneous in-jections.
Microneedles also present promising application in aesthetic medicine (Cachafeiro et al., 2016; Iriarte et al., 2017; Sitohang et al., 2021). Microneedles exert effects mainly by piercing the epidermal skin and enhancing the release of loaded active ingredients, which can lead to a reduction in skin scars, pigmentation, and wrinkles (Choy and Prausnitz, 2011). Dermaroller ® (Dermaroller GmbH, Wolfenbüttel, Germany) is a commonly used microneedle device, which contains 192 needles, each with a length of 0.5–3 mm and base diameter of 0.1–0.25 mm. They are arranged in 24 circular arrays. By scrolling, the microneedles are inserted into the epidermis and papillary leather, producing hundreds of mini small holes, followed by topical release of active ingredients (Shin et al., 2012; Shukla and Gold, 2021). Compared with traditional methods, microneedles can increase the delivery efficiency of medicines with higher safety and effectiveness (Cachafeiro et al., 2016), as the micropores generated by the microneedle insertion can be healed within a few hours (Aung et al., 2020). Furthermore, minoxidil-loaded microneedles can stimulate hair follicle stem cells and activate growth factors in the dermal nurtle, which can promote hair growth in alopecia (Faghihi et al., 2021). Owing to these advantages, many clinical trials of microneedle-based products are being conducted for aesthetic medicine applications (Hoesly et al., 2012; Min et al., 2015; Kwon et al., 2017; Biesman et al., 2019).
In the past 10 years, remarkable advances have been made in microneedle-based drug delivery systems. Many microneedle-based products have been patented and entered the clinical trial stage, especially in aesthetic medicine. However, most microneedle-based drug delivery systems are still needed to improve for better applications. For example, microneedles may induce a slightly short skin stimulus, inducing local spotted erythema and edema, the holes after penetration with microneedles may also cause skin infections. Microneedle-based products need more extensive clinical investigation for improved compatibility and further extensive application. With the development of biocompatible polymers, microneedle systems with good biocompatibility and solubility are expected to be designed. At that time, microneedle will have a higher impact in the clinic and will further improve human health through a variety of drug delivery methods.
JC, HR, and PZ wrote this article; SZ revised this article; BD, XL, and FX checked and reviewed this article.
This work was supported by the startup funding from Jinan University, the Fundamental Research Funds for the Central Universities (No. 11618337), and the National Natural Science Foundation of China (No. 81903546).
The authors declare that the research was conducted in the absence of any commercial or financial relationships that could be construed as a potential conflict of interest.
All claims expressed in this article are solely those of the authors and do not necessarily represent those of their affiliated organizations, or those of the publisher, the editors and the reviewers. Any product that may be evaluated in this article, or claim that may be made by its manufacturer, is not guaranteed or endorsed by the publisher.
Al-Qallaf, B., and Das, D. B. (2008). Optimization of square microneedle arrays for increasing drug permeability in skin. Chem. Eng. Sci. 63 (9), 2523–2535. doi:10.1016/j.ces.2008.02.007
Al‐Naggar, M. R., Al‐Adl, A. S., Rabie, A. R., Abdelkhalk, M. R., and Elsaie, M. L. (2019). Intralesional bleomycin injection vs microneedling‐assisted topical bleomycin spraying in treatment of plantar warts. J. Cosmet. Dermatol. 18 (1), 124–128. doi:10.1111/jocd.12537
Armstrong, A. W., and Read, C. (2020). Pathophysiology, clinical presentation, and treatment of psoriasis: A review. Jama 323 (19), 1945–1960. doi:10.1001/jama.2020.4006
Arya, J., Henry, S., Kalluri, H., McAllister, D. V., Pewin, W. P., and Prausnitz, M. R. (2017). Tolerability, usability and acceptability of dissolving microneedle patch administration in human subjects. Biomaterials 128, 1–7. doi:10.1016/j.biomaterials.2017.02.040
Aung, N. N., Ngawhirunpat, T., Rojanarata, T., Patrojanasophon, P., Pamornpathomkul, B., and Opanasopit, P. (2020). Fabrication, characterization and comparison of α-arbutin loaded dissolving and hydrogel forming microneedles. Int. J. Pharm. X. 586, 119508. doi:10.1016/j.ijpharm.2020.119508
Bacelieri, R., and Johnson, S. M. (2005). Cutaneous warts: An evidence-based approach to therapy. Am. Fam. Physician 72 (4), 647–652.
Baek, S.-H., Shin, J.-H., and Kim, Y.-C. (2017). Drug-coated microneedles for rapid and painless local anesthesia. Biomed. Microdevices 19 (1), 2. doi:10.1007/s10544-016-0144-1
Barabas, K., Milner, R., Lurie, D., and Adin, C. (2008). Cisplatin: A review of toxicities and therapeutic applications. Vet. Comp. Oncol. 6 (1), 1–18. doi:10.1111/j.1476-5829.2007.00142.x
Biesman, B. S., Cohen, J. L., DiBernardo, B. E., Emer, J. J., Geronemus, R. G., Gold, M. H., et al. (2019). Treatment of atrophic facial acne scars with microneedling followed by polymethylmethacrylate-collagen gel dermal filler. Dermatol. Surg. 45 (12), 1570–1579. doi:10.1097/DSS.0000000000001872
Bik, L., Sangers, T., Greveling, K., Prens, E., Haedersdal, M., and van Doorn, M. (2020). Efficacy and tolerability of intralesional bleomycin in dermatology: A systematic review. J. Am. Acad. Dermatol. 83 (3), 888–903. doi:10.1016/j.jaad.2020.02.018
Cachafeiro, T., Escobar, G., Maldonado, G., Cestari, T., and Corleta, O. (2016). Comparison of nonablative fractional erbium laser 1, 340 nm and microneedling for the treatment of atrophic acne scars: A randomized clinical trial. Dermatol. Surg. 42 (2), 232–241. doi:10.1097/DSS.0000000000000597
Cao, S., Wang, Y., Wang, M., Yang, X., Tang, Y., Pang, M., et al. (2021). Microneedles mediated bioinspired lipid nanocarriers for targeted treatment of alopecia. J. Control. Release 329, 1–15. doi:10.1016/j.jconrel.2020.11.038
Chen, M.-C., Chen, C.-S., Wu, Y.-W., and Yang, Y.-Y. (2020). Poly-γ-Glutamate microneedles as transdermal immunomodulators for ameliorating atopic dermatitis-like skin lesions in Nc/Nga mice. Acta Biomater. 114, 183–192. doi:10.1016/j.actbio.2020.07.029
Chen, M.-C., Lin, Z.-W., and Ling, M.-H. (2016). Near-infrared light-activatable microneedle system for treating superficial tumors by combination of chemotherapy and photothermal therapy. ACS Nano 10 (1), 93–101. doi:10.1021/acsnano.5b05043
Chiang, B., Venugopal, N., Edelhauser, H. F., and Prausnitz, M. R. (2016). Distribution of particles, small molecules and polymeric formulation excipients in the suprachoroidal space after microneedle injection. Exp. Eye Res. 153, 101–109. doi:10.1016/j.exer.2016.10.011
Choy, Y. B., and Prausnitz, M. R. (2011). The rule of five for non-oral routes of drug delivery: Ophthalmic, inhalation and transdermal. Pharm. Res. 28 (5), 943–948. doi:10.1007/s11095-010-0292-6
Clifton, M. M., Johnson, S. M., Roberson, P. K., Kincannon, J., and Horn, T. D. (2003). Immunotherapy for recalcitrant warts in children using intralesional mumps or Candida antigens. Pediatr. Dermatol. 20 (3), 268–271. doi:10.1046/j.1525-1470.2003.20318.x
Cullen, W., Gulati, G., and Kelly, B. D. (2020). Mental health in the COVID-19 pandemic. QJM:Int J. Med. 113 (5), 311–312. doi:10.1093/qjmed/hcaa110
Dalbagni, G. (2007). The management of superficial bladder cancer. Nat. Clin. Pract. Urol. 4 (5), 254–260. doi:10.1038/ncpuro0784
Daniel, J. (2020). Education and the COVID-19 pandemic. Prospects 49 (1), 91–96. doi:10.1007/s11125-020-09464-3
Davis, S. P., Landis, B. J., Adams, Z. H., Allen, M. G., and Prausnitz, M. R. (2004). Insertion of microneedles into skin: Measurement and prediction of insertion force and needle fracture force. J. Biomech. 37 (8), 1155–1163. doi:10.1016/j.jbiomech.2003.12.010
Davis, S. P., Martanto, W., Allen, M. G., and Prausnitz, M. R. (2005). Hollow metal microneedles for insulin delivery to diabetic rats. IEEE Trans. Biomed. Eng. 52 (5), 909–915. doi:10.1109/TBME.2005.845240
Doft, M. A., Hardy, K. L., and Ascherman, J. A. (2012). Treatment of hyperhidrosis with botulinum toxin. Aesthet. Surg. J. 32 (2), 238–244. doi:10.1177/1090820X11434506
Dong, L., Li, Y., Li, Z., Xu, N., Liu, P., Du, H., et al. (2018). Au nanocage-strengthened dissolving microneedles for chemo-photothermal combined therapy of superficial skin tumors. ACS Appl. Mat. Interfaces 10 (11), 9247–9256. doi:10.1021/acsami.7b18293
Donnelly, R. F., Majithiya, R., Singh, T. R. R., Morrow, D. I., Garland, M. J., Demir, Y. K., et al. (2011). Design, optimization and characterisation of polymeric microneedle arrays prepared by a novel laser-based micromoulding technique. Pharm. Res. 28 (1), 41–57. doi:10.1007/s11095-010-0169-8
Donthu, N., and Gustafsson, A. (2020). Effects of COVID-19 on business and research. J. Bus. Res. 117, 284–289. doi:10.1016/j.jbusres.2020.06.008
Dryden, M. S. (2010). Complicated skin and soft tissue infection. J. Antimicrob. Chemother. 65, 35–44. doi:10.1093/jac/dkq302
Du, G., Hathout, R. M., Nasr, M., Nejadnik, M. R., Tu, J., Koning, R. I., et al. (2017). Intradermal vaccination with hollow microneedles: A comparative study of various protein antigen and adjuvant encapsulated nanoparticles. J. Control. Release 266, 109–118. doi:10.1016/j.jconrel.2017.09.021
Du, H., Liu, P., Zhu, J., Lan, J., Li, Y., Zhang, L., et al. (2019). Hyaluronic acid-based dissolving microneedle patch loaded with methotrexate for improved treatment of psoriasis. ACS Appl. Mat. Interfaces 11 (46), 43588–43598. doi:10.1021/acsami.9b15668
Erdos, G., Balmert, S. C., Carey, C. D., Falo, G. D., Patel, N. A., Zhang, J., et al. (2020). Improved cutaneous genetic immunization by microneedle array delivery of an adjuvanted adenovirus vaccine. J. Invest. Dermatol. 140 (12), 25282528–25282531.e2. doi:10.1016/j.jid.2020.03.966
Faghihi, G., Nabavinejad, S., Mokhtari, F., Fatemi Naeini, F., and Iraji, F. (2021). Microneedling in androgenetic alopecia; comparing two different depths of microneedles. J. Cosmet. Dermatol. 20 (4), 1241–1247. doi:10.1111/jocd.13714
Gao, H., Bi, Y., Wang, X., Wang, M., Zhou, M., Lu, H., et al. (2017). Near-infrared guided thermal-responsive nanomedicine against orthotopic superficial bladder cancer. ACS Biomater. Sci. Eng. 3 (12), 3628–3634. doi:10.1021/acsbiomaterials.7b00405
Gardeniers, H. J., Luttge, R., Berenschot, E. J., De Boer, M. J., Yeshurun, S. Y., Hefetz, M., et al. (2003). Silicon micromachined hollow microneedles for transdermal liquid transport. J. Microelectromech. Syst. 12 (6), 855–862. doi:10.1109/JMEMS.2003.820293
Gill, H. S., Denson, D. D., Burris, B. A., and Prausnitz, M. R. (2008). Effect of microneedle design on pain in human volunteers. Clin. J. Pain 24 (7), 585–594. doi:10.1097/AJP.0b013e31816778f9
Gill, H. S., and Prausnitz, M. R. (2007). Coating formulations for microneedles. Pharm. Res. 24 (7), 1369–1380. doi:10.1007/s11095-007-9286-4
Giudice, E. L., and Campbell, J. D. (2006). Needle-free vaccine delivery. Adv. Drug Deliv. Rev. 58 (1), 68–89. doi:10.1016/j.addr.2005.12.003
Grossman, D. C., Curry, S. J., Owens, D. K., Barry, M. J., Caughey, A. B., Davidson, K. W., et al. (2018). Behavioral counseling to prevent skin cancer: US preventive services task force recommendation statement. Jama 319 (11), 1134–1142. doi:10.1001/jama.2018.1623
Halder, J., Gupta, S., Kumari, R., Gupta, G. D., and Rai, V. K. (2020). Microneedle array: Applications, recent advances, and clinical pertinence in transdermal drug delivery. J. Pharm. Innov. 16, 558–565. doi:10.1007/s12247-020-09460-2
Hamm, H. (2014). Impact of hyperhidrosis on quality of life and its assessment. Dermatol. Clin. 32 (4), 467–476. doi:10.1016/j.det.2014.06.004
Hashmi, S., Ling, P., Hashmi, G., Reed, M., Gaugler, R., and Trimmer, W. (1995). Genetic transformation of nematodes using arrays of micromechanical piercing structures. BioTechniques 19 (5), 766–770.
Havlickova, B., Czaika, V. A., and Friedrich, M. (2008). Epidemiological trends in skin mycoses worldwide. Mycoses 51, 2–15. doi:10.1111/j.1439-0507.2008.01606.x
Heidary, N., Naik, H., and Burgin, S. (2008). Chemotherapeutic agents and the skin: An update. J. Am. Acad. Dermatol. 58 (4), 545–570. doi:10.1016/j.jaad.2008.01.001
Henry, S., McAllister, D. V., Allen, M. G., and Prausnitz, M. R. (1998). Microfabricated microneedles: A novel approach to transdermal drug delivery. J. Pharm. Sci. 87 (8), 922–925. doi:10.1021/js980042+
Hoesly, F. J., Borovicka, J., Gordon, J., Nardone, B., Holbrook, J. S., Pace, N., et al. (2012). Safety of a novel microneedle device applied to facial skin: A subject-and rater-blinded, sham-controlled, randomized trial. Arch. Dermatol. 148 (6), 711–717. doi:10.1001/archdermatol.2012.280
Hoorens, I., and Ongenae, K. (2012). Primary focal hyperhidrosis: Current treatment options and a step‐by‐step approach. J. Eur. Acad. Dermatol. Venereol. 26 (1), 1–8. doi:10.1111/j.1468-3083.2011.04173.x
Hossain, M. K., Ahmed, T., Bhusal, P., Subedi, R. K., Salahshoori, I., Soltani, M., et al. (2020). Microneedle systems for vaccine delivery: The story so far. Expert Rev. Vaccines 19 (12), 1153–1166. doi:10.1080/14760584.2020.1874928
Iriarte, C., Awosika, O., Rengifo-Pardo, M., and Ehrlich, A. (2017). Review of applications of microneedling in dermatology. Clin. Cosmet. Investig. Dermatol. 10, 289–298. doi:10.2147/CCID.S142450
Ita, K. (2017). Dissolving microneedles for transdermal drug delivery: Advances and challenges. Biomed. Pharmacother. 93, 1116–1127. doi:10.1016/j.biopha.2017.07.019
Ita, K. (2018). Modulation of transdermal drug delivery with coated microneedles. J. Drug Deliv. Sci. Technol. 45, 203–212. doi:10.1016/j.jddst.2018.03.021
Ita, K. (2015). Transdermal delivery of drugs with microneedles—Potential and challenges. Pharmaceutics 7 (3), 90–105. doi:10.3390/pharmaceutics7030090
Jamaledin, R., Yiu, C. K., Zare, E. N., Niu, L. N., Vecchione, R., Chen, G., et al. (2020). Advances in antimicrobial microneedle patches for combating infections. Adv. Mat. 32 (33), 2002129. doi:10.1002/adma.202002129
Jang, M., Kang, B. M., Yang, H., Ohn, J., Kwon, O., and Jung, H. (2021). High‐dose steroid dissolving microneedle for relieving atopic dermatitis. Adv. Healthc. Mat. 10 (7), 2001691. doi:10.1002/adhm.202001691
Jeong, H.-R., Kim, J.-Y., Kim, S.-N., and Park, J.-H. (2018). Local dermal delivery of cyclosporin A, a hydrophobic and high molecular weight drug, using dissolving microneedles. Eur. J. Pharm. Biopharm. 127, 237–243. doi:10.1016/j.ejpb.2018.02.014
Jiang, J., Gill, H. S., Ghate, D., McCarey, B. E., Patel, S. R., Edelhauser, H. F., et al. (2007). Coated microneedles for drug delivery to the eye. Invest. Ophthalmol. Vis. Sci. 48 (9), 4038–4043. doi:10.1167/iovs.07-0066
Jolivet, J., Cowan, K. H., Curt, G. A., Clendeninn, N. J., and Chabner, B. A. (1983). The pharmacology and clinical use of methotrexate. N. Engl. J. Med. 309 (18), 1094–1104. doi:10.1056/nejm198311033091805
Jung, P. G., Lee, T. W., Oh, D. J., Hwang, S. J., Jung, I. D., Lee, S. M., et al. (2008). Nickel microneedles fabricated by sequential copper and nickel electroless plating and copper chemical wet etching. Sens. Mater 20 (1), 45–53.
Kamata, M., and Tada, Y. (2018). Safety of biologics in psoriasis. J. Dermatol. 45 (3), 279–286. doi:10.1111/1346-8138.14096
Kapoor, Y., Milewski, M., Dick, L., Zhang, J., Bothe, J. R., Gehrt, M., et al. (2020). Coated microneedles for transdermal delivery of a potent pharmaceutical peptide. Biomed. Microdevices 22 (1), 7–10. doi:10.1007/s10544-019-0462-1
Kim, J., Kim, B. E., and Leung, D. Y. (2019). “Pathophysiology of atopic dermatitis: Clinical implications,” in Allergy asthma proc (Rhode Island, United States: OceanSide Publications), 84–92.
Kim, M. J., Park, S. C., Rizal, B., Guanes, G., Baek, S.-K., Park, J.-H., et al. (2018). Fabrication of circular obelisk-type multilayer microneedles using micro-milling and spray deposition. Front. Bioeng. Biotechnol. 6, 54. doi:10.3389/fbioe.2018.00054
Kim, M., Shin, J. Y., Lee, J., Kim, J. Y., and Oh, S. H. (2013). Efficacy of fractional microneedle radiofrequency device in the treatment of primary axillary hyperhidrosis: A pilot study. Dermatology 227 (3), 243–249. doi:10.1159/000354602
Kim, Y.-C., Quan, F.-S., Yoo, D.-G., Compans, R. W., Kang, S.-M., and Prausnitz, M. R. (2010). Enhanced memory responses to seasonal H1N1 influenza vaccination of the skin with the use of vaccine-coated microneedles. J. Infect. Dis. 201 (2), 190–198. doi:10.1086/649228
Kis, E. E., Winter, G., and Myschik, J. (2012). Devices for intradermal vaccination. Vaccine 30 (3), 523–538. doi:10.1016/j.vaccine.2011.11.020
Konicke, K., and Olasz, E. (2016). Successful treatment of recalcitrant plantar warts with bleomycin and microneedling. Dermatol. Surg. 42 (8), 1007–1008. doi:10.1097/DSS.0000000000000738
Korbel, J. O., and Stegle, O. (2020). Effects of the COVID-19 pandemic on life scientists. Genome Biol. 21 (1), 113–115. doi:10.1186/s13059-020-02031-1
Kwon, H. H., Park, H. Y., Choi, S. C., Bae, Y., Jung, J. Y., Park, G.-H., et al. (2017). Combined fractional treatment of acne scars involving non-ablative 1, 550-nm erbium-glass laser and micro-needling radiofrequency: A 16-week prospective, randomized split-face study. Acta Derm. Venerol. 97 (8-9), 947–951. doi:10.2340/00015555-2701
Lakraj, A.-A. D., Moghimi, N., and Jabbari, B. (2013). Hyperhidrosis: Anatomy, pathophysiology and treatment with emphasis on the role of botulinum toxins. Toxins 5 (4), 821–840. doi:10.3390/toxins5040821
Lambert, P. H., and Laurent, P. E. (2008). Intradermal vaccine delivery: Will new delivery systems transform vaccine administration? Vaccine 26 (26), 3197–3208. doi:10.1016/j.vaccine.2008.03.095
Lan, X., She, J., Lin, D.-a., Xu, Y., Li, X., Yang, W.-f., et al. (2018). Microneedle-mediated delivery of lipid-coated cisplatin nanoparticles for efficient and safe cancer therapy. ACS Appl. Mat. Interfaces 10 (39), 33060–33069. doi:10.1021/acsami.8b12926
Lau, S., Fei, J., Liu, H., Chen, W., and Liu, R. (2017). Multilayered pyramidal dissolving microneedle patches with flexible pedestals for improving effective drug delivery. J. Control. Release 265, 113–119. doi:10.1016/j.jconrel.2016.08.031
Lee, H. S., Ryu, H. R., Roh, J. Y., and Park, J.-H. (2017). Bleomycin-coated microneedles for treatment of warts. Pharm. Res. 34 (1), 101–112. doi:10.1007/s11095-016-2042-x
Lee, J. W., Park, J.-H., and Prausnitz, M. R. (2008). Dissolving microneedles for transdermal drug delivery. Biomaterials 29 (13), 2113–2124. doi:10.1016/j.biomaterials.2007.12.048
Lee, K., and Jung, H. (2012). Drawing lithography for microneedles: A review of fundamentals and biomedical applications. Biomaterials 33 (30), 7309–7326. doi:10.1016/j.biomaterials.2012.06.065
Lee, K., Lee, H. C., Lee, D. S., and Jung, H. (2010). Drawing lithography: Three‐dimensional fabrication of an ultrahigh‐aspect‐ratio microneedle. Adv. Mat. 22 (4), 483–486. doi:10.1002/adma.200902418
Lee, T.-Y., Kim, D.-J., Won, J.-N., Lee, I.-H., Sung, M.-H., and Poo, H. (2014). Oral administration of poly-γ-glutamate ameliorates atopic dermatitis in Nc/Nga mice by suppressing Th2-biased immune response and production of IL-17A. J. Invest. Dermatol. 134 (3), 704–711. doi:10.1038/jid.2013.389
Leong, H. N., Kurup, A., Tan, M. Y., Kwa, A. L. H., Liau, K. H., and Wilcox, M. H. (2018). Management of complicated skin and soft tissue infections with a special focus on the role of newer antibiotics. Infect. Drug Resist. 11, 1959–1974. doi:10.2147/IDR.S172366
Li, J., Liu, B., Zhou, Y., Chen, Z., Jiang, L., Yuan, W., et al. (2017a). Fabrication of a Ti porous microneedle array by metal injection molding for transdermal drug delivery. PLoS One 12 (2), e0172043. doi:10.1371/journal.pone.0172043
Li, J., Zeng, M., Shan, H., and Tong, C. (2017b). Microneedle patches as drug and vaccine delivery platform. Curr. Med. Chem. 24 (22), 2413–2422. doi:10.2174/0929867324666170526124053
Li, Q. Y., Zhang, J. N., Chen, B. Z., Wang, Q. L., and Guo, X. D. (2017c). A solid polymer microneedle patch pretreatment enhances the permeation of drug molecules into the skin. RSC Adv. 7 (25), 15408–15415. doi:10.1039/C6RA26759A
Li, S., Zhang, Y., Wang, Y., Xia, K., Yin, Z., Wang, H., et al. (2020a). Physical sensors for skin‐inspired electronics. InfoMat 2 (1), 184–211. doi:10.1002/inf2.12060
Li, Z., He, Y., Deng, L., Zhang, Z.-R., and Lin, Y. (2020b). A fast-dissolving microneedle array loaded with chitosan nanoparticles to evoke systemic immune responses in mice. J. Mat. Chem. B 8 (2), 216–225. doi:10.1039/C9TB02061F
Littauer, E. Q., Mills, L. K., Brock, N., Esser, E. S., Romanyuk, A., Pulit-Penaloza, J. A., et al. (2018). Stable incorporation of GM-CSF into dissolvable microneedle patch improves skin vaccination against influenza. J. Control. Release 276, 1–16. doi:10.1016/j.jconrel.2018.02.033
Lockwood, S. J., Prens, L. M., and Kimball, A. B. (2018). “Adverse reactions to biologics in psoriasis,” in Adverse reactions to biologics. Editors L. Puig, and W. Gulliver. 1st ed (Basel, Switzerland: Karger Publishers), 1–14.
Loehrer, P. J., and Einhorn, L. H. (1984). Cisplatin. Ann. Intern. Med. 100 (5), 704–713. doi:10.7326/0003-4819-100-5-704
Lomas, A., Leonardi‐Bee, J., and Bath‐Hextall, F. (2012). A systematic review of worldwide incidence of nonmelanoma skin cancer. Br. J. Dermatol. 166 (5), 1069–1080. doi:10.1111/j.1365-2133.2012.10830.x
Luttge, R., Berenschot, E. J., De Boer, M. J., Altpeter, D. M., Vrouwe, E. X., Van Den Berg, A., et al. (2007). Integrated lithographic molding for microneedle-based devices. J. Microelectromech. Syst. 16 (4), 872–884. doi:10.1109/JMEMS.2007.899339
Ma, B., Liu, S., Gan, Z., Liu, G., Cai, X., Zhang, H., et al. (2006).A PZT insulin pump integrated with a silicon micro needle array for transdermal drug deliveryProceedings of the 56th Electronic Components and Technology Conference 2006, 30 May 2006 - 02 June 2006, San Diego, CA, USA . IEEE.
Ma, G., and Wu, C. (2017). Microneedle, bio-microneedle and bio-inspired microneedle: A review. J. Control. Release 251, 11–23. doi:10.1016/j.jconrel.2017.02.011
Malik, A. A., McFadden, S. M., Elharake, J., and Omer, S. B. (2020). Determinants of COVID-19 vaccine acceptance in the US. EClinicalMedicine 26, 100495. doi:10.1016/j.eclinm.2020.100495
Martanto, W., Davis, S. P., Holiday, N. R., Wang, J., Gill, H. S., and Prausnitz, M. R. (2004). Transdermal delivery of insulin using microneedles in vivo. Pharm. Res. 21 (6), 947–952. doi:10.1023/B:PHAM.0000029282.44140.2e
Martanto, W., Moore, J. S., Kashlan, O., Kamath, R., Wang, P. M., O'Neal, J. M., et al. (2006). Microinfusion using hollow microneedles. Pharm. Res. 23 (1), 104–113. doi:10.1007/s11095-005-8498-8
Martin, S., and Gerstel, V. A. P. (1971). Drug delivery device. U.S. Patent No 3964482. Washington, DC: U.S. Patent and Trademark Office.
Matriano, J. A., Cormier, M., Johnson, J., Young, W. A., Buttery, M., Nyam, K., et al. (2002). Macroflux® microprojection array patch technology: A new and efficient approach for intracutaneous immunization. Pharm. Res. 19 (1), 63–70. doi:10.1023/A:1013607400040
McGrath, M. G., Vrdoljak, A., O’Mahony, C., Oliveira, J. C., Moore, A. C., and Crean, A. M. (2011). Determination of parameters for successful spray coating of silicon microneedle arrays. Int. J. Pharm. X. 415 (1-2), 140–149. doi:10.1016/j.ijpharm.2011.05.064
Menon, G. K., Cleary, G. W., and Lane, M. E. (2012). The structure and function of the stratum corneum. Int. J. Pharm. X. 435 (1), 3–9. doi:10.1016/j.ijpharm.2012.06.005
Michalek, I., Loring, B., and John, S. (2017). A systematic review of worldwide epidemiology of psoriasis. J. Eur. Acad. Dermatol. Venereol. 31 (2), 205–212. doi:10.1111/jdv.13854
Miller, P. R., Gittard, S. D., Edwards, T. L., Lopez, D. M., Xiao, X., Wheeler, D. R., et al. (2011). Integrated carbon fiber electrodes within hollow polymer microneedles for transdermal electrochemical sensing. Biomicrofluidics 5 (1), 013415. doi:10.1063/1.3569945
Min, S., Park, S. Y., Yoon, J. Y., and Suh, D. H. (2015). Comparison of fractional microneedling radiofrequency and bipolar radiofrequency on acne and acne scar and investigation of mechanism: Comparative randomized controlled clinical trial. Arch. Dermatol. Res. 307 (10), 897–904. doi:10.1007/s00403-015-1601-z
Minami, S. B., Sha, S.-H., and Schacht, J. (2004). Antioxidant protection in a new animal model of cisplatin-induced ototoxicity. Hear. Res. 198 (1-2), 137–143. doi:10.1016/j.heares.2004.07.016
Mir, M., Permana, A. D., Tekko, I. A., McCarthy, H. O., Ahmed, N., Donnelly, R. F., et al. (2020). Microneedle liquid injection system assisted delivery of infection responsive nanoparticles: A promising approach for enhanced site-specific delivery of carvacrol against polymicrobial biofilms-infected wounds. Int. J. Pharm. X. 587, 119643. doi:10.1016/j.ijpharm.2020.119643
Mishra, B., and Bonde, G. V. (2020). “Transdermal drug delivery,” in Controlled drug delivery systems. Editor E. Opara. 1st ed (Florida, United States: CRC Press), 239–275.
Moon, S. J., Lee, S. S., Lee, H., and Kwon, T. (2005). Fabrication of microneedle array using LIGA and hot embossing process. Microsyst. Technol. 11 (4-5), 311–318. doi:10.1007/s00542-004-0446-8
Na, Y.-G., Kim, M., Han, M., Huh, H. W., Kim, J.-S., Kim, J. C., et al. (2020). Characterization of Hepatitis B surface antigen loaded polylactic acid-based microneedle and its dermal safety profile. Pharmaceutics 12 (6), 531. doi:10.3390/pharmaceutics12060531
Naito, C., Katsumi, H., Suzuki, T., Quan, Y.-s., Kamiyama, F., Sakane, T., et al. (2018). Self-dissolving microneedle arrays for transdermal absorption enhancement of human parathyroid hormone (1-34). Pharmaceutics 10 (4), 215. doi:10.3390/pharmaceutics10040215
Narayanan, S. P., and Raghavan, S. (2019). Fabrication and characterization of gold-coated solid silicon microneedles with improved biocompatibility. Int. J. Adv. Manuf. Technol. 104 (9), 3327–3333. doi:10.1007/s00170-018-2596-3
Narayanan, S. P., and Raghavan, S. (2017). Solid silicon microneedles for drug delivery applications. Int. J. Adv. Manuf. Technol. 93 (1), 407–422. doi:10.1007/s00170-016-9698-6
Nawrocki, S., and Cha, J. (2019). The etiology, diagnosis, and management of hyperhidrosis: A comprehensive review. J. Am. Acad. Dermatol. 81 (3), 669–680. doi:10.1016/j.jaad.2018.11.066
Netea, M. G., Brown, G. D., Kullberg, B. J., and Gow, N. A. (2008). An integrated model of the recognition of Candida albicans by the innate immune system. Nat. Rev. Microbiol. 6 (1), 67–78. doi:10.1038/nrmicro1815
Ogai, N., Nonaka, I., Toda, Y., Ono, T., Minegishi, S., Inou, A., et al. (2018). Enhanced immunity in intradermal vaccination by novel hollow microneedles. Skin. Res. Technol. 24 (4), 630–635. doi:10.1111/srt.12576
Pan, Q., Yu, Y., Chen, D., Jiao, G., and Liu, X. (2020). Enhanced penetration strategies for transdermal delivery. Front. Chem. Sci. Eng. 14 (3), 378–388. doi:10.1007/s11705-019-1913-1
Park, Y., and Kim, B. (2017). Skin permeability of compounds loaded within dissolving microneedles dependent on composition of sodium hyaluronate and carboxymethyl cellulose. Korean J. Chem. Eng. 34 (1), 133–138. doi:10.1007/s11814-016-0240-1
Permana, A. D., Mir, M., Utomo, E., and Donnelly, R. F. (2020). Bacterially sensitive nanoparticle-based dissolving microneedles of doxycycline for enhanced treatment of bacterial biofilm skin infection: A proof of concept study. Int. J. Pharm. X 2, 100047. doi:10.1016/j.ijpx.2020.100047
Prausnitz, M. R., Gomaa, Y., and Li, W. (2019). Microneedle patch drug delivery in the gut. Nat. Med. 25 (10), 1471–1472. doi:10.1038/s41591-019-0606-0
Rodgers, A. M., Cordeiro, A. S., Kissenpfennig, A., and Donnelly, R. F. (2018). Microneedle arrays for vaccine delivery: The possibilities, challenges and use of nanoparticles as a combinatorial approach for enhanced vaccine immunogenicity. Expert Opin. Drug Deliv. 15 (9), 851–867. doi:10.1080/17425247.2018.1505860
Roenigk, H. H., Auerbach, R., Maibach, H. I., and Weinstein, G. D. (1988). Methotrexate in psoriasis: Revised guidelines. J. Am. Acad. Dermatol. 19 (1), 145–156. doi:10.1016/S0190-9622(88)80237-8
Rouphael, N. G., Paine, M., Mosley, R., Henry, S., McAllister, D. V., Kalluri, H., et al. (2017). The safety, immunogenicity, and acceptability of inactivated influenza vaccine delivered by microneedle patch (TIV-MNP 2015): A randomised, partly blinded, placebo-controlled, phase 1 trial. Lancet 390 (10095), 649–658. doi:10.1016/S0140-6736(17)30575-5
Sabri, A. H., Ogilvie, J., Abdulhamid, K., Shpadaruk, V., McKenna, J., Segal, J., et al. (2019). Expanding the applications of microneedles in dermatology. Eur. J. Pharm. Biopharm. 140, 121–140. doi:10.1016/j.ejpb.2019.05.001
Saitta, P., Krishnamurthy, K., and Brown, L. H. (2008). Bleomycin in dermatology: A review of intralesional applications. Dermatol. Surg. 34 (10), 1299–1313. doi:10.1111/j.1524-4725.2008.34281.x
Shim, W. S., Hwang, Y. M., Park, S. G., Lee, C. K., and Kang, N. G. (2018). Role of polyvinylpyrrolidone in dissolving microneedle for efficient transdermal drug delivery: In vitro and clinical studies. Bull. Korean Chem. Soc. 39 (6), 789–793. doi:10.1002/bkcs.11476
Shin, J.-H., Lee, J.-H., Jeong, S. D., Noh, J.-Y., Lee, H. W., Song, C.-S., et al. (2020). C-di-GMP with influenza vaccine showed enhanced and shifted immune responses in microneedle vaccination in the skin. Drug Deliv. Transl. Res. 10 (3), 815–825. doi:10.1007/s13346-020-00728-1
Shin, J. U., Lee, S. H., Jung, J. Y., and Lee, J. H. (2012). A split-face comparison of a fractional microneedle radiofrequency device and fractional carbon dioxide laser therapy in acne patients. J. Cosmet. Laser Ther. 14 (5), 212–217. doi:10.3109/14764172.2012.720023
Shukla, S. K., and Gold, M. H. (2021). Treatment of acne and acne scars with microneedling. Microneedling Glob. Perspect. Aesthetic Med., 81–97. doi:10.1002/9781119431817.ch6
Sitohang, I. B. S., Sirait, S. A. P., and Suryanegara, J. (2021). Microneedling in the treatment of atrophic scars: A systematic review of randomised controlled trials. Int. Wound J. 18, 577–585. doi:10.1111/iwj.13559
Song, G., Jiang, G., Liu, T., Zhang, X., Zeng, Z., Wang, R., et al. (2020). Separable microneedles for synergistic chemo-photothermal therapy against superficial skin tumors. ACS Biomater. Sci. Eng. 6 (7), 4116–4125. doi:10.1021/acsbiomaterials.0c00793
Sullivan, S. P., Murthy, N., and Prausnitz, M. R. (2008). Minimally invasive protein delivery with rapidly dissolving polymer microneedles. Adv. Mat. 20 (5), 933–938. doi:10.1002/adma.200701205
Sung, M. H., Park, C., Kim, C. J., Poo, H., Soda, K., and Ashiuchi, M. (2005). Natural and edible biopolymer poly‐γ‐glutamic acid: Synthesis, production, and applications. Chem. Rec. 5 (6), 352–366. doi:10.1002/tcr.20061
Tham, H. P., Xu, K., Lim, W. Q., Chen, H., Zheng, M., Thng, T. G. S., et al. (2018). Microneedle-assisted topical delivery of photodynamically active mesoporous formulation for combination therapy of deep-seated melanoma. ACS Nano 12 (12), 11936–11948. doi:10.1021/acsnano.8b03007
Torrelo, A. (2017). Methylprednisolone aceponate for atopic dermatitis. Int. J. Dermatol. 56 (6), 691–697. doi:10.1111/ijd.13485
Torrisi, B. M., Zarnitsyn, V., Prausnitz, M., Anstey, A., Gateley, C., Birchall, J. C., et al. (2013). Pocketed microneedles for rapid delivery of a liquid-state botulinum toxin A formulation into human skin. J. Control. Release 165 (2), 146–152. doi:10.1016/j.jconrel.2012.11.010
Van Bavel, J. J., Baicker, K., Boggio, P. S., Capraro, V., Cichocka, A., Cikara, M., et al. (2020). Using social and behavioural science to support COVID-19 pandemic response. Nat. Hum. Behav. 4 (5), 460–471. doi:10.1038/s41562-020-0884-z
Van der Maaden, K., Jiskoot, W., and Bouwstra, J. (2012). Microneedle technologies for (trans) dermal drug and vaccine delivery. J. Control. Release 161 (2), 645–655. doi:10.1016/j.jconrel.2012.01.042
Waghule, T., Singhvi, G., Dubey, S. K., Pandey, M. M., Gupta, G., Singh, M., et al. (2019). Microneedles: A smart approach and increasing potential for transdermal drug delivery system. Biomed. Pharmacother. 109, 1249–1258. doi:10.1016/j.biopha.2018.10.078
Wang, C., Ye, Y., Hochu, G. M., Sadeghifar, H., and Gu, Z. (2016). Enhanced cancer immunotherapy by microneedle patch-assisted delivery of anti-PD1 antibody. Nano Lett. 16 (4), 2334–2340. doi:10.1021/acs.nanolett.5b05030
Wang, F., Zhang, X., Chen, G., and Zhao, Y. (2020a). Living bacterial microneedles for fungal infection treatment. Research 2020, 1–9. doi:10.34133/2020/2760594
Wang, J., Jing, R., Lai, X., Zhang, H., Lyu, Y., Knoll, M. D., et al. (2020b). Acceptance of COVID-19 vaccination during the COVID-19 pandemic in China. Vaccines 8 (3), 482. doi:10.3390/vaccines8030482
Wang, P. M., Cornwell, M., Hill, J., and Prausnitz, M. R. (2006). Precise microinjection into skin using hollow microneedles. J. Invest. Dermatol. 126 (5), 1080–1087. doi:10.1038/sj.jid.5700150
Wilke, N., Mulcahy, A., Ye, S.-R., and Morrissey, A. (2005). Process optimization and characterization of silicon microneedles fabricated by wet etch technology. Microelectron. J. 36 (7), 650–656. doi:10.1016/j.mejo.2005.04.044
Yang, S. Y., O'Cearbhaill, E. D., Sisk, G. C., Park, K. M., Cho, W. K., Villiger, M., et al. (2013). A bio-inspired swellable microneedle adhesive for mechanical interlocking with tissue. Nat. Commun. 4 (1), 1702–1710. doi:10.1038/ncomms2715
Yin, K., and Smith, A. G. (2016). Nuclear receptor function in skin health and disease: Therapeutic opportunities in the orphan and adopted receptor classes. Cell. Mol. Life Sci. 73 (20), 3789–3800. doi:10.1007/s00018-016-2329-4
Zan, P., Than, A., Duong, P. K., Song, J., Xu, C., and Chen, P. (2019). Antimicrobial microneedle patch for treating deep cutaneous fungal infection. Adv. Ther. (Weinh). 2 (10), 1900064. doi:10.1002/adtp.201900064
Zhang, L., Wang, W., and Wang, S. (2015). Effect of vaccine administration modality on immunogenicity and efficacy. Expert Rev. Vaccines 14 (11), 1509–1523. doi:10.1586/14760584.2015.1081067
Zhang, M., Fang, J., Wu, Q., and Lin, T. (2018a). A prospective study of the safety and efficacy of a microneedle fractional radiofrequency system for global facial photoaging in Chinese patients. Dermatol. Surg. 44 (7), 964–970. doi:10.1097/DSS.0000000000001469
Zhang, Y., Jiang, G., Yu, W., Liu, D., and Xu, B. (2018b). Microneedles fabricated from alginate and maltose for transdermal delivery of insulin on diabetic rats. Mater. Sci. Eng. C 85, 18–26. doi:10.1016/j.msec.2017.12.006
Zhang, Y., Yu, J., Kahkoska, A. R., Wang, J., Buse, J. B., and Gu, Z. (2019). Advances in transdermal insulin delivery. Adv. Drug Deliv. Rev. 139, 51–70. doi:10.1016/j.addr.2018.12.006
Zhu, D. D., Wang, X. L., Zhang, X. P., Ma, J. J., Kong, D. L., Zhang, M. M., et al. (2019a). A dissolvable microneedle formulation of bordetella pertussis subunit vaccine: Translational development and immunological evaluation in mice. ACS Appl. Bio Mat. 2 (11), 5053–5061. doi:10.1021/acsabm.9b00730
Zhu, J., Dong, L., Du, H., Mao, J., Xie, Y., Wang, H., et al. (2019b). 5‐Aminolevulinic acid‐loaded hyaluronic acid dissolving microneedles for effective photodynamic therapy of superficial tumors with enhanced long‐term stability. Adv. Healthc. Mat. 8 (22), 1900896. doi:10.1002/adhm.201900896
Zhu, W., Li, S., Wang, C., Yu, G., Prausnitz, M. R., and Wang, B.-Z. (2018). Enhanced immune responses conferring cross-protection by skin vaccination with a tri-component influenza vaccine using a microneedle patch. Front. Immunol. 9, 1705. doi:10.3389/fimmu.2018.01705
Keywords: drug delivery, microneedle, cutaneous disease, transdermal route, microneedle fabrication
Citation: Chen J, Ren H, Zhou P, Zheng S, Du B, Liu X and Xiao F (2022) Microneedle-mediated drug delivery for cutaneous diseases. Front. Bioeng. Biotechnol. 10:1032041. doi: 10.3389/fbioe.2022.1032041
Received: 30 August 2022; Accepted: 05 October 2022;
Published: 17 October 2022.
Edited by:
Ming-Wei Chang, Ulster University, United KingdomReviewed by:
Baolin Wang, Hebei University of Technology, ChinaCopyright © 2022 Chen, Ren, Zhou, Zheng, Du, Liu and Xiao. This is an open-access article distributed under the terms of the Creative Commons Attribution License (CC BY). The use, distribution or reproduction in other forums is permitted, provided the original author(s) and the copyright owner(s) are credited and that the original publication in this journal is cited, in accordance with accepted academic practice. No use, distribution or reproduction is permitted which does not comply with these terms.
*Correspondence: Bin Du, ZG9jdG9yZHVAaG90bWFpbC5jb20=; Xiaowen Liu, eHdsaXUyMzFAam51LmVkdS5jbg==; Fei Xiao, eGlhb2ZlaUBqbnUuZWR1LmNu
†These authors have contributed equally to this work
Disclaimer: All claims expressed in this article are solely those of the authors and do not necessarily represent those of their affiliated organizations, or those of the publisher, the editors and the reviewers. Any product that may be evaluated in this article or claim that may be made by its manufacturer is not guaranteed or endorsed by the publisher.
Research integrity at Frontiers
Learn more about the work of our research integrity team to safeguard the quality of each article we publish.