- 1Laboratory of Integrative Medicine, Clinical Research Center for Breast, State Key Laboratory of Biotherapy, West China Hospital, Sichuan University, Chengdu, Sichuan, China
- 2Department of Head, Neck and Mammary Gland Oncology, Cancer Center, West China Hospital, Sichuan University, Chengdu, Sichuan, China
Metal-organic frameworks (MOFs) are a class of hybrid porous crystalline materials that are assembled with metal ions/clusters and organic linkers. The fungibility of organic ligands and metal centers endow MOFs that are easy to design and synthesize. Based on their unique structure, multifarious MOFs with diverse functionalities have recently been widely applied in various research areas. Particularly striking is the application of photo-responsive MOFs in biological sensing and imaging. Notably, the photoelectronic properties make photo-responsive MOFs an ideal platform for cancer phototherapy. Moreover, ultrahigh porosities and tunable pore sizes allow MOFs to load anticancer drugs, further enhancing the antitumor efficiency. In this review, the categories and developing strategies of MOFs are briefly introduced. The application fields of MOFs in bioimaging, such as up-conversion fluorescence imaging, single/two-photon fluorescence bioimaging, magnetic resonance imaging, etc., are summarized. The working mechanism of MOFs in photo-responsive, photothermal therapy (PTT), and photodynamic therapy (PDT) are expounded. Examples of using MOFs for cancer treatment, including PTT, PDT, chemotherapy, and radiotherapy, are also demonstrated. Lastly, current limitations, challenges, and future perspectives for bioimaging and cancer treatment of MOFs are discussed. We believe that the versatile MOF will bring the dawn to the next generation of cancer treatment.
Introduction
Metal-organic frameworks (MOFs) are a class of porous crystalline materials consisting of one-, two- or three-dimensional networks created by organic linkers and metal centers through coordination bonding (Wang et al., 2020a). Since their discovery in the late 1990s (Ding et al., 2022), MOFs have attracted great interest in various areas, including but not limited to materials science, pharmacology, chemistry, engineering, and biochemistry. Distinct MOFs can be made by combining diverse metal nodes and organic linkers. So far, many MOFs with various structures and functions have been reported.
In recent years, photo-responsive MOFs have drawn extensive attention due to their unique structural characters and photoelectronic properties (Zheng et al., 2021). Metal centers, such as Au, Mn2+, and Gd3+, endow MOFs with photoelectronic properties (Liu et al., 2022a). In addition, implanting photothermal agents or photosensitizers into their framework can produce photo-responsive MOFs. Their photoelectronic characteristics have enabled MOFs to be applied in tumor bioimaging, photothermal therapy (PTT), and photodynamic therapy (PDT). For PTT, photo-responsive MOFs generate heat under laser irradiation, exerting antitumor therapeutic effects (Liu et al., 2019). While in PDT, irradiated photo-responsive MOFs can create highly toxic reactive oxygen species (ROS) to react directly with many biomolecules in cells, thus inducing cell death and tissue lesions (Pham et al., 2021). Moreover, MOFs are efficient drug carriers because of their tunable pore size and high surface area. MOFs also have many modifiable sites that facilitate the stimuli-responsive release of loaded drugs and improve the targeting ability of nanoparticles by coupling specific polymers or tumor-targeted molecules. Therefore, MOFs are also excellent platforms for drug delivery.
Taken together, numerous studies have demonstrated that MOFs have unique advantages in bioimaging and cancer treatment. Here, we outlined the categories and developing strategies of MOFs. Meanwhile, we present the application of MOFs in bioimaging, including up-conversion fluorescence imaging, single/two-photon fluorescence bioimaging, magnetic resonance imaging, etc. Moreover, we enumerated the application of MOFs in cancer treatment, such as PTT, PDT, chemotherapy, and radiotherapy. Finally, we discuss the limitations, challenges, and future perspectives of MOFs in bioimaging and cancer treatment.
Photo-responsive MOF
Photo-responsive MOFs have fascinating advantages of phototherapy and drug loading at the same time. In recent years, the studies of these MOFs have exhibited a rapidly growing tendency. In this section, we discussed the types and working principles of photo-responsive MOF.
Classification of photo-responsive MOF
Based on replaceable organic ligands, metal centers, and ultrahigh porosities, MOFs not only serve as efficient phototherapeutic agents for intrinsically photo-responsive but also load phototherapeutic agents for non-intrinsic photo-responsive properties (Zheng et al., 2021). According to the type of phototherapeutic units in MOF, intrinsic photo-responsive MOF can be divided into organic-doped MOF and metal-doped MOF. Regularly arranged phototherapeutic organic and metal in the MOFs can improve photostability and bioimaging/PTT/PDT efficacy (Yin et al., 2022). Organic-doped MOFs include porphyrin-based MOFs (Begum et al., 2019; Zhang et al., 2021), bacteriochlorin-based MOFs (Zhang et al., 2019a; Pucelik et al., 2020), BODIPY-based MOFs (Wang et al., 2016; Yang et al., 2019a), Prussian blue-based MOFs (Zhang et al., 2019b), etc. Metal-doped MOFs consist of copper, iron, and manganese-based MOFs. Non-intrinsic photo-responsive MOFs do not have optical characteristics themselves. Nevertheless, their tunable structure and porosity can perform them as medicine conveyance. Phototherapeutic agents can be loaded inside the MOF or embedded in the massive pores of the MOF (Chen et al., 2015; Yang et al., 2019b; Deng et al., 2019). Moreover, some photosensitive polymers can be used as coating materials on the surface of MOFs. MOF’s high specific surface area can provide more footholds for them, bringing the advantages of the two into full play (Liu et al., 2014).
Synthesis of photo-responsive MOF
The synthesis of MOFs is a process in which metal ions/clusters and organic ligands self-assemble to form repeating structural units (Figure 1). Their properties are determined by many factors in the progress of synthesis. Typically, these factors include the self-photoelectronic properties of the metal ions and the organic ligands, the solvent used, the reaction time and temperature, and the crystallization kinetics (Raptopoulou, 2021). In the past few decades, numerous classic synthesis methods for photo-responsive MOFs have emerged, such as hydro (solvo)-thermal, sonochemical, microwave, mechanochemical, and electrochemical methods. Among them, the hydro (solvo)-thermal method is considered one of the most popular methods to prepare photo-responsive MOFs due to its simplicity, convenience, and low cost. A kind of two-dimensional Cu-TCPP MOF with significantly enhanced photoelectric properties and dual-mode light-emitting Ln-MOFs are synthesized in this gentle way with high pressure and temperature (Xu et al., 2020; Wang et al., 2022). Moreover, selecting adequate methods, such as ultrasound (Abazari et al., 2019), microwave (Yang et al., 2022), electrochemistry (Cong et al., 2014) and mechanical force (Khosroshahi et al., 2022) to assist synthesis can shorten the reaction time and better control the particle morphology, having also been favored by scientists in recent years.
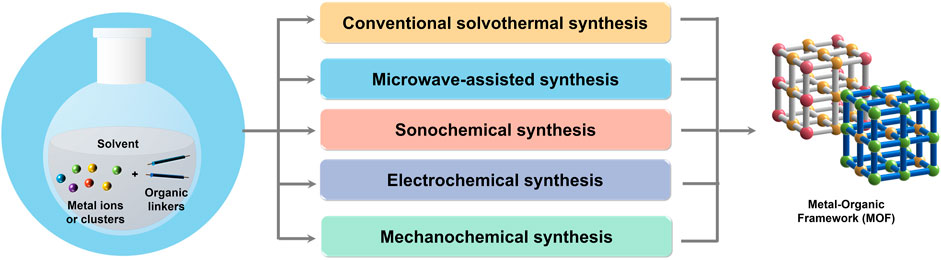
FIGURE 1. Illustration of MOF synthesis. Different methods synthesize MOF from metal ions/clusters and organic ligands, self-assembling them to form porous crystalline materials with repeating structural units.
Working principle of photo-responsive MOF
Photo-responsive MOF can be initiated to work via laser irradiation with a specific wavelength. Once the electrons of photo-responsive MOFs absorb photon energy, they migrate from the basic singlet state (S0) to the singlet excited state (S1). They subsequently release excess energy through various pathways, returning to the S0 state (Pamei and Puzari, 2019). Fluorescence emission will occur when the molecule releases its energy in the form of a fluorescent photon. This fluorescence can be used in bioimaging (Li et al., 2022).
Working principle of MOF in PTT
For photothermal MOF materials, the working principle is complex and diverse. In most photothermal MOFs with organic dyes and polymers, their S1 state tends to undergo nonradiative vibrational relaxation, returning to the ground state by the collision between the chromophores and the surrounding biological environments and emitting energy as heat (Ng and Zheng, 2015). In contrast, high carrier density materials such as semiconductors, metals, metal oxides, and quantum dots can have a photothermal effect through the localized plasmon surface resonance (LSPR) (Luther et al., 2011; Long et al., 2015). When this collective oscillation of electrons decays by nonradiative transition, energy is dissipated as heat. In low electron density semiconductors, heat is generated by the relaxation of electron-hole pairs. Irradiating them will excite their electron to a higher energy status in the conduction band and leave a hole in the valence band. These electrons and holes will lose energy as heat, relaxing to the band edges through vibrational relaxation, recombining near the band edge, and further generating heat through the crystal lattice vibration (Wang et al., 2017; Li et al., 2019).
Working principle of MOF in PDT
In PDT of photo-responsive MOF, unstable electrons of the S1 state will radiate some energy in the form of fluorescent quanta, subsequently converting into a more stable excited state (T1) (Ethirajan et al., 2011; Ng and Zheng, 2015). The photo-responsive MOF in the T1 state can produce cytotoxic ROS through two processes: Type I and Type II reactions (DeRosa and Crutchley, 2002; Agostinis et al., 2011). In the type I reaction, the MOF interacts directly with the cancerous substrate and creates free radicals and anion radicals through an electron or hydrogen transfer, leading to the appearance of ROS, such as hydrogen peroxide (H2O2), hydroxyl radicals (˙OH), and superoxide anion radicals (O2−) (Juarranz et al., 2008; Lan et al., 2019). During type II reactions, the energy of MOF in the T1 state is moved directly to the basic energetic state of oxygen (O2). Then it generates highly reactive singlet oxygen (1O2) species (Van Straten et al., 2017; Kwiatkowski et al., 2018).
Photo-responsive MOF for bioimaging
Up to now, photo-responsive MOFshave drawn significant attention in the bioimaging field due to their advantages of acceptable biocompatibility, better dispersing property, and high biological activity (Giliopoulos et al., 2020). Numerous studies have combined MOFs and bioimaging to develop a more effective medical in vivo and in vitro imaging system (Zhu et al., 2021), including up-conversion fluorescence imaging, NIR PersL imaging, single- or two-photon fluorescence bioimaging, magnetic resonance imaging, etc (Table 1).
MOF based up-conversion fluorescence imaging
Up-conversion nanoparticles (UCNPs), favored by researchers for their outstanding ability to emit visible light under infrared irradiation, have been widely used in bioimaging as novel optical probes (Liu et al., 2022a). For example, using MOFs based on iron (III) carboxylate materials as the shell and β-NaYF4:Yb3+/Er3+ nanoparticles as the core, Deng et al. developed a novel aptamer-guided nanocarrier (Deng et al., 2015). In biological imaging applications, the UCNPs were able to emit strong green emissions for up-conversion fluorescence imaging under a 980 nm laser. Compared with the photobleaching and quenching of fluorescent organic molecules, this MOF can provide good stability, high resolution, and high fluorescence efficiency optical imaging in vivo.
MOF based NIR PersL imaging
Persistent luminescence (PersL) is a phenomenon in which optical materials still emit long-lasting luminescence after absent excitation (Liu et al., 2022a). Recently, interest in the application of near-infrared (NIR) PersL nanoparticles (PLNPs) as optical probes in vivo bioimaging systems has increased significantly due to their biological properties such as background autofluorescence-free, high tissue penetration, and low radiation damage (Lv et al., 2019). Zhao and coworkers synthesized a persistent luminescent MOF (PLMOF) of PLNP@ZIF-8, and the continuous emission of infrared light from PLNPs in the absence of external irradiation could activate tumor site imaging without background interference (Zhao et al., 2019). Their results demonstrated that PLMOF could be an encouraging theragnostic platform for precision medicine.
MOF based single-photon fluorescence bioimaging
Single-photon imaging can detect low-intensity light, showing weak optical signals with single photon sensitivity (Rehain et al., 2020; He et al., 2022). Mostakim and partners reported Hf-based UiO-66 MOFs with high hydrolytic stability and catalytic activity (Sk et al., 2018). No additional surface modification is required during synthesis. Due to the characteristics of rapid reactivity, high selectivity, and specific sensitivity of peroxynitrite, Hf-based UiO-66 MOFs could be applied to single-photon bioimages of living cells through the detection of intracellular peroxynitrite.
MOF based two-photon fluorescence bioimaging
Two-photon excitation fluorescence imaging is a powerful technique for visualizing deep tissue due to its high photostability, low photodamage, and high spatiotemporal resolution (Liu et al., 2017; Lu et al., 2020). Chan Yang and his group reported a two-photon MOF (TP-MOF) based on nanoscale MOFs combined with a two-photon organic part using a click chemistry method (Yang et al., 2019c). The TP-MOF probes could ascertain and generate images in living cells and tissues within a penetration range of 130 μm due to the two-photon fluorophores excited by NIR while retaining the TP organic moiety’s fluorescence-responsive properties and possessing excellent photostability and biocompatibility.
MOF based magnetic resonance imaging
Magnetic resonance imaging (MRI) is a leading technique in clinical imaging and pathology analysis due to its advantages in high tissue resolution, non-invasive angiography, and water imaging (Logothetis, 2008). It can detect the electromagnetic wave emitted by the applied gradient magnetic field according to different attenuations of the released energy in the various structural environments inside the material to understand the position and type of tissue to distinguish pathological changes (Slobozhanyuk et al., 2016). As MOFs are commonly used in developing T2-weighted contrast agents for MRI, the polymeric substance of MOFs and MRI can be used in complex bioimaging by providing soft‐tissue contrast without ionizing radiation (Hamideh et al., 2020).
MOF based computed tomography
Computed tomography (CT) is one of the most crucial imaging methods in the clinic in prognosis prediction, differentiation of benign and malignant tumors, grading and staging, efficacy evaluation, and recurrence monitoring because of its cost-effectiveness, anatomical imaging ability, and wide availability (Ohlerth and Scharf, 2007). More recently, A new X-ray CT contrast agent, bismuth-NU-901 (Bi-NU-901), was solvothermally synthesized by Robison and co-workers (Robison et al., 2019). The in vitro studies revealed Bi-NU-901 demonstrates ∼14 times better contrast than a commercially available CT contrast agent. With the development of nano-technology, varied and adjustable MOFs can act as contrast agents to achieve better consequences and reduce toxic and adverse effects on healthy tissue.
MOF based multimode bioimaging
Unlike the inherent limitation of the single-imaging technique, the fusion of different imaging modes, which has developed rapidly recently, could complement each other for comprehensive diagnostic information (Wu and Yang, 2017). For example, Fan et al. recently constructed a multimodal imaging nanoplatform called ICG-CpG@MOF for cancer diagnosis and treatment. This nanoplatform uses a specific MOF, MIL101(Fe), as the core carrier, then dressed with photoacoustic and fluorescent signal donors (ICG) (Fan et al., 2021). These platforms can utilize multimodal imaging for synergistic therapy, providing hopeful cancer diagnosis and treatment approaches.
Photo-responsive MOF in cancer therapy
The synthesis convenience and structural flexibility allow guest molecules, such as nanoparticles, anticancer drugs, and biomolecules, to be implanted into the framework to produce MOFs with multiple functions, such as PTT, PDT, and tumor targeting (Cheng et al., 2022). As a protective shell, the porous crystal structure of MOFs not only has a high carrying capacity due to its large specific surface area and high porosity but also improves the catalytic activity of the nanocomponents. At the same time, their weak coordination bond ensures the biodegradability of MOF (Liu et al., 2022b). These desirable properties make MOFs promising applications in drug delivery, clinical oncology therapy, and other disease treatments (Table 1).
Application of MOF in PTT
In PTT, by utilizing an optically absorbing MOF, light can be converted efficiently into thermal energy to kill the targeted cells in cancer and other disease treatments (Jiang et al., 2018), with non-invasiveness, safety, and efficiency in comparison with traditional oncology therapies (Zhi et al., 2020). Meanwhile, photo-responsive MOF-mediated PTT can protect normal tissues from heat damage (Liu et al., 2021; Yue and Zhao, 2021). Recently, Meizhen Yin and coworkers reported a crystalline MOF synthesized with perylenediimide (PDIs) (Lü et al., 2019). It shows high near-infrared photothermal conversion efficiency due to the stability of ambient radical anions. Unlike traditional cancer treatments, the high yield and stability endowed PDI-based 3D MOF with great potential in photothermal therapy. Moreover, the “turn-on” strategy triggered by endogenous biomarkers can simplify the composition of nanomedicines and offering hope for precision cancer treatment.
Application of MOF in PDT
In PDT, photo-responsive MOF produces ROS, especially singlet oxygen radicals, upon exposure to light (Kwiatkowski et al., 2018). All components of the intracellular environment are affected by ROS, including but not limited to proteins and DNA, leading to necrosis or apoptosis. The fabrication of photo-responsive MOF by optimizing their size parameter can play a critical role for improving cellular response. Zhou et al. synthesized spherical TCPP-Zr-NMOF(PCN-224) of different sizes ranging from 30 to 190 nm, and then studied the size-dependent cellular uptake and ensuing PDT (Park et al., 2016). They find preferential cellular uptake of 90 nm-PCN-224 and its remarkable PDT efficacy over other sizes using HeLa cells. Cai et al. designed and constructed Au@MOF core-shell hybrids using the layer-by-layer method (Cai et al., 2021). The porphyrin ligand drives the conversion of O2 into 1O2 in the MOF shell upon light source stimulation. In the tumor microenvironment, H2O2 can be decomposed into O2 by catalytic action in the presence of the metal node Fe3O(OAc)6(H2O)3+ cluster of the MOF, resulting in a strong PDT effect, which promotes the tumor cell killing effects and tumor growth inhibition.
MOF improves the efficiency of chemotherapy
MOF is becoming a leading candidate vector for drug delivery in chemotherapy due to its multiple advantages, such as adjustable pore size, variable structure, diverse functions, large capacity, and high biocompatibility (Wu and Yang, 2017; Wang et al., 2018; Wang et al., 2020b). Wan and coworkers developed a procedural release nanosystem with a Fe-TCPP MOF shell loaded with dihydroartemisinin (DHA) to treat cancer (Wan et al., 2019). The CaCO3 mineralized layer was encapsulated in the pores of the MOF to avoid drug leakage during transportation. The release of DHA from Fe-TCPP MOF and TCPP activation is triggered by high concentrations of GSH and a weakly acidic microenvironment within the tumor. The triple-combo regimen of Fe2+-DHA mediated chemotherapy, Ca2+-DHA mediated tumor therapy, and TCPP mediated PDT synthesis inhibits tumor growth and has no biological toxicity in vivo.
MOF enhances the sensitivity of radiotherapy
Radiotherapy utilizes high-intensity ionizing radiation to induce DNA damage by generating ROS (Wu and Yang, 2017). However, plenty of evidence has shown that hypoxic cells are less sensitive to radiation than normoxic cells in radiotherapy. Benefiting from the porous structure, good biocompatibility, and tunable size, MOF NPs are considered ideal candidates for improving the sensitivity of radiotherapy (He et al., 2019). Du and his partners synthesized D-arginine-loaded MOF nanoparticles to avoid serious adverse effects during high-dose radiation therapy (Du et al., 2021). D-arginine attenuates tumor hypoxia by producing nitric oxide and down-regulating HIF-1α. In addition, MOF could produce free radicals to enhance the killing effect of tumor cells. In vitro and in vivo results showed that MOFs loaded with D-arginine increased tumor elimination and prevented lung metastasis in mice after radiotherapy, along with relatively low toxicity in cells and mice.
MOF based combination therapy in combating cancer
Combination therapy has a distinct advantage over monotherapies in terms of enhanced tumor treatment efficacy, reduced drug toxicity, and drug resistance. Many efforts have been made to improve tumor treatment efficacy through multimodal combination therapies. The excellent drug carrier ability and photoelectronic properties of MOFs make them an ideal platform for coordinating cancer immunotherapy, targeted therapy, chemotherapy, and PTT/PDT. Recently, Dr. Tian and his colleagues fabricated a kind of tumor cell membrane modified hollow porphyrinic MOF nanoparticle to co-load chemotherapy drug doxorubicin (DOX) and photosensitizer indocyanine green (ICG) (Sun et al., 2021). The multifunctional nanoparticle exhibits excellent in vivo imaging and mediates photodynamic-photothermal-chemotherapy combo treatment. In addition, many photo-responsive MOFs have been found to have acoustic sensitivity. They can not only perform sonodynamic therapy (SDT) (Wang et al., 2020c) while exerting optical performance but also perform photoacoustic (PA) imaging. In a recent study, for example, Han and coworkers developed a kind of multifunctional H-TiO2/C-PEG nanosheet theranostic platform (Han et al., 2022). These nanosheets can not only act as ultrasound sensitizers but also possess good photothermal and photodynamic properties. When they are applied to imaging-guided tumor therapy, they have significant advantages in the precise treatment of tumors.
Conclusion and perspectives
The applications of photo-responsive MOFs in biomedicine have boomed in the past decades. MOFs with intrinsic light response behavior by using photo-responsive building blocks or serve as the conveyance of phototherapeutic agents, owing to their tunable structure. The regular array of MOFs prevents aggregation and self-quenching of photosensitive units, greatly enhancing their effectiveness for phototherapy and bioimaging. MOF’s porous structure and easily modifiable properties make it a superior platform for imaging-guided therapy and combination therapies.
However, MOFs still have some limitations in cancer bioimaging and phototherapy (Zhao et al., 2022a). Firstly, these photo-responsive MOFs are still in the primary research stage, and the FDA has yet to approve them. Issues related to the biosafety of materials must be addressed if MOFs are to be applied in the clinical setting. It is necessary to optimize surface functionalization to achieve prolonged blood circulation and enhanced tumor targeting. Meanwhile, MOFs’ biodegradation and rapid in vivo clearance must be considered (Wang et al., 2021), evaluating their absorption-distribution-metabolism-excretion (ADME) to understand their toxicity profiles. Moreover, the tissue-penetration ability of light is limited (Chitgupi et al., 2017). MOFs with longer wavelengths, such as NIR-II, UCNPs, and two-photon activated MOFs, should be developed to improve the efficiency of deep tumor imaging and therapy. In addition, dynamic treatments usually have strong oxygen dependence (Xie et al., 2021). Therefore, increasing the oxygen delivery or combining hypoxia-activating drugs may be effective methods to overcome hypoxia in the deep tumor sites. And MOF happens to be an excellent carrier for the delivery of a variety of medicines and gases. We believe that MOF can break through the bottleneck of current conventional tumor treatments such as surgery, neoadjuvant chemoradiotherapy, and radiofrequency ablation (Yang et al., 2021; Zhao et al., 2022b). Undoubtedly, photo-responsive MOFs will be a promising area for future research and will become an essential part of medical treatment.
Author contributions
All authors listed have made a substantial, direct, and intellectual contribution to the work and approved it for publication.
Funding
This work was funded by the National Natural Science Foundation of China (No. 22105137 and No. 82172634); Key Program of the Science and Technology Bureau of Sichuan (No. 2021YFSY0007); 1.3.5 project for disciplines of excellence, West China Hospital, Sichuan University (No. ZYYC20013); China Postdoctoral Science Foundation (No. 2020M683324 and No. 2022T150449).
Conflict of interest
The authors declare that the research was conducted in the absence of any commercial or financial relationships that could be construed as a potential conflict of interest.
Publisher’s note
All claims expressed in this article are solely those of the authors and do not necessarily represent those of their affiliated organizations, or those of the publisher, the editors and the reviewers. Any product that may be evaluated in this article, or claim that may be made by its manufacturer, is not guaranteed or endorsed by the publisher.
References
Abazari, R., Mahjoub, A. R., and Salehi, G. (2019). Preparation of amine functionalized G-C(3)N(4)@(H/S)mof ncs with visible light photocatalytic characteristic for 4-nitrophenol degradation from aqueous solution. J. Hazard. Mater. 365, 921–931. doi:10.1016/j.jhazmat.2018.11.087
Agostinis, P., Berg, K., Cengel, K. A., Foster, T. H., Girotti, A. W., Gollnick, S. O., et al. (2011). Photodynamic therapy of cancer: An update. CA a cancer J. Clin. 61 (4), 250–281. doi:10.3322/caac.20114
Begum, S., Hassan, Z., Bräse, S., Wöll, C., and Tsotsalas, M. (2019). Metal-organic framework-templated biomaterials: Recent progress in synthesis, functionalization, and applications. Acc. Chem. Res. 52 (6), 1598–1610. doi:10.1021/acs.accounts.9b00039
Cai, X., Zhao, Y., Wang, L., Hu, M., Wu, Z., Liu, L., et al. (2021). Synthesis of Au@mof core-shell hybrids for enhanced photodynamic/photothermal therapy. J. Mat. Chem. B 9 (33), 6646–6657. doi:10.1039/d1tb00800e
Chen, R., Zhang, J., Wang, Y., Chen, X., Zapien, J. A., and Lee, C-S. (2015). Graphitic carbon nitride Nanosheet@Metal–organic framework core–shell nanoparticles for photo-chemo combination therapy. Nanoscale 7 (41), 17299–17305. doi:10.1039/C5NR04436G
Cheng, K., Liu, B., Zhang, X. S., Zhang, R. Y., Zhang, F., Ashraf, G., et al. (2022). Biomimetic material degradation for synergistic enhanced therapy by regulating endogenous energy metabolism imaging under hypothermia. Nat. Commun. 13 (1), 4567. doi:10.1038/s41467-022-32349-2
Chitgupi, U., Qin, Y., and Lovell, J. F. (2017). Targeted nanomaterials for phototherapy. Nanotheranostics 1 (1), 38–58. doi:10.7150/ntno.17694
Cong, H. P., Chen, J. F., and Yu, S. H. (2014). Graphene-based macroscopic assemblies and architectures: An emerging material system. Chem. Soc. Rev. 43 (21), 7295–7325. doi:10.1039/c4cs00181h
Deng, K., Hou, Z., Li, X., Li, C., Zhang, Y., Deng, X., et al. (2015). Aptamer-mediated up-conversion core/mof shell nanocomposites for targeted drug delivery and cell imaging. Sci. Rep. 5, 7851. doi:10.1038/srep07851
Deng, X., Liang, S., Cai, X., Huang, S., Cheng, Z., Shi, Y., et al. (2019). Yolk-shell structured Au Nanostar@Metal-organic framework for synergistic chemo-photothermal therapy in the second near-infrared window. Nano Lett. 19 (10), 6772–6780. doi:10.1021/acs.nanolett.9b01716
DeRosa, M. C., and Crutchley, R. J. (2002). Photosensitized singlet oxygen and its applications. Coord. Chem. Rev. 233-234, 351–371. doi:10.1016/S0010-8545(02)00034-6
Ding, M., Liu, W., and Gref, R. (2022). Nanoscale mofs: From synthesis to drug delivery and theranostics applications. Adv. drug Deliv. Rev. 190, 114496. doi:10.1016/j.addr.2022.114496
Du, C., Zhou, M., Jia, F., Ruan, L., Lu, H., Zhang, J., et al. (2021). D-Arginine-Loaded metal-organic frameworks nanoparticles sensitize osteosarcoma to radiotherapy. Biomaterials 269, 120642. doi:10.1016/j.biomaterials.2020.120642
Ethirajan, M., Chen, Y., Joshi, P., and Pandey, R. K. (2011). The role of porphyrin chemistry in tumor imaging and photodynamic therapy. Chem. Soc. Rev. 40 (1), 340–362. doi:10.1039/B915149B
Fan, Z., Liu, H., Xue, Y., Lin, J., Fu, Y., Xia, Z., et al. (2021). Reversing cold tumors to hot: An immunoadjuvant-functionalized metal-organic framework for multimodal imaging-guided synergistic photo-immunotherapy. Bioact. Mater. 6 (2), 312–325. doi:10.1016/j.bioactmat.2020.08.005
Giliopoulos, D., Zamboulis, A., Giannakoudakis, D., Bikiaris, D., and Triantafyllidis, K. (2020). Polymer/metal organic framework (mof) nanocomposites for biomedical applications. Mol. (Basel, Switz. 25 (1), 185. doi:10.3390/molecules25010185
Hamideh, R. A., Akbari, B., Fathi, P., Misra, S. K., Sutrisno, A., Lam, F., et al. (2020). Biodegradable mri visible drug eluting stent reinforced by metal organic frameworks. Adv. Healthc. Mat. 9 (14), e2000136. doi:10.1002/adhm.202000136
Han, X., Zhao, C., Wang, S., Pan, Z., Jiang, Z., and Tang, X. (2022). Multifunctional Tio2/C nanosheets derived from 3d metal–organic frameworks for mild-temperature-photothermal-sonodynamic-chemodynamic therapy under photoacoustic image guidance. J. Colloid Interface Sci. 621, 360–373. doi:10.1016/j.jcis.2022.04.077
He, B., Zhang, Q., Pan, Z., Li, L., Li, C., Ling, Y., et al. (2022). Freestanding metal-organic frameworks and their derivatives: An emerging platform for electrochemical energy storage and conversion. Chem. Rev. 122 (11), 10087–10125. doi:10.1021/acs.chemrev.1c00978
He, Z., Huang, X., Wang, C., Li, X., Liu, Y., Zhou, Z., et al. (2019). A catalase-like metal-organic framework nanohybrid for O(2) -evolving synergistic chemoradiotherapy. Angew. Chem. Int. Ed. 58 (26), 8752–8756. doi:10.1002/anie.201902612
Jiang, W., Zhang, H., Wu, J., Zhai, G., Li, Z., Luan, Y., et al. (2018). Cus@Mof-Based well-designed quercetin delivery system for chemo-photothermal therapy. ACS Appl. Mat. Interfaces 10 (40), 34513–34523. doi:10.1021/acsami.8b13487
Juarranz, A., Jaén, P., Sanz-Rodríguez, F., Cuevas, J., and González, S. (2008). Photodynamic therapy of cancer. Basic principles and applications. Clin. Transl. Oncol. 10 (3), 148–154. doi:10.1007/s12094-008-0172-2
Khosroshahi, N., Bakhtian, M., and Safarifard, V. (2022). Mechanochemical synthesis of ferrite/mof nanocomposite: Efficient photocatalyst for the removal of meropenem and hexavalent chromium from water. J. Photochem. Photobiol. A Chem. 431, 114033. doi:10.1016/j.jphotochem.2022.114033
Kwiatkowski, S., Knap, B., Przystupski, D., Saczko, J., Kędzierska, E., Knap-Czop, K., et al. (2018). Photodynamic therapy – mechanisms, photosensitizers and combinations. Biomed. Pharmacother. 106, 1098–1107. doi:10.1016/j.biopha.2018.07.049
Lan, G., Ni, K., Veroneau, S. S., Feng, X., Nash, G. T., Luo, T., et al. (2019). Titanium-based nanoscale metal–organic framework for type I photodynamic therapy. J. Am. Chem. Soc. 141 (10), 4204–4208. doi:10.1021/jacs.8b13804
Li, B., Lu, X., Tian, Y., and Li, D. (2022). Embedding multiphoton active units within metal-organic frameworks for turning on high-order multiphoton excited fluorescence for bioimaging. Angew. Chem. Int. Ed. Engl. 61 (31), e202206755. doi:10.1002/anie.202206755
Li, J., Liu, X., Tan, L., Liang, Y., Cui, Z., Yang, X., et al. (2019). Photocatalysis: Light-Activated rapid disinfection by accelerated charge transfer in red phosphorus/zno heterointerface (small methods 3/2019). Small Methods 3, 1970008. doi:10.1002/smtd.201970008
Li, S. Y., Cheng, H., Qiu, W. X., Zhang, L., Wan, S. S., Zeng, J. Y., et al. (2017). Cancer cell membrane-coated biomimetic platform for tumor targeted photodynamic therapy and hypoxia-amplified bioreductive therapy. Biomaterials 142, 149–161. doi:10.1016/j.biomaterials.2017.07.026
Liu, H. W., Liu, Y., Wang, P., and Zhang, X. B. (2017). Molecular engineering of two-photon fluorescent probes for bioimaging applications. Methods Appl. Fluoresc. 5 (1), 012003. doi:10.1088/2050-6120/aa61b0
Liu, Q., Wu, B., Li, M., Huang, Y., and Li, L. (2022). Heterostructures made of upconversion nanoparticles and metal-organic frameworks for biomedical applications. Adv. Sci. (Weinheim, Baden-Wurttemberg, Ger. 9 (3), e2103911. doi:10.1002/advs.202103911
Liu, X., Feng, Y., Xu, J., Shi, Y., Yang, J., Zhang, R., et al. (2021). Combination of mapk inhibition with photothermal therapy synergistically augments the anti-tumor efficacy of immune checkpoint blockade. J. Control. Release 332, 194–209. doi:10.1016/j.jconrel.2021.02.020
Liu, Y., Ai, K., and Lu, L. (2014). Polydopamine and its derivative materials: Synthesis and promising applications in energy, environmental, and biomedical fields. Chem. Rev. 114 (9), 5057–5115. doi:10.1021/cr400407a
Liu, Y., Bhattarai, P., Dai, Z., and Chen, X. (2019). Photothermal therapy and photoacoustic imaging via nanotheranostics in fighting cancer. Chem. Soc. Rev. 48 (7), 2053–2108. doi:10.1039/c8cs00618k
Liu, Y., Jiang, T., and Liu, Z. (2022). Metal-organic frameworks for bioimaging: Strategies and challenges. Nanotheranostics 6 (2), 143–160. doi:10.7150/ntno.63458
Logothetis, N. K. (2008). What we can do and what we cannot do with fmri. Nature 453 (7197), 869–878. doi:10.1038/nature06976
Long, R., Li, Y., Song, L., and Xiong, Y. (2015). Coupling solar energy into reactions: Materials design for surface plasmon-mediated catalysis. Small (Weinheim der Bergstrasse, Ger. 11 (32), 3873–3889. doi:10.1002/smll.201403777
Lü, B., Chen, Y., Li, P., Wang, B., Müllen, K., and Yin, M. (2019). Stable radical anions generated from a porous perylenediimide metal-organic framework for boosting near-infrared photothermal conversion. Nat. Commun. 10 (1), 767. doi:10.1038/s41467-019-08434-4
Lu, Q., Wu, C. J., Liu, Z., Niu, G., and Yu, X. (2020). Exploiting double exchange diels-alder cycloadditions for immobilization of peptide nucleic acids on gold nanoparticles. Front. Chem. 8, 4. doi:10.3389/fchem.2020.00004
Luther, J. M., Jain, P. K., Ewers, T., and Alivisatos, A. P. (2011). Localized surface plasmon resonances arising from free carriers in doped quantum dots. Nat. Mat. 10 (5), 361–366. doi:10.1038/nmat3004
Lv, Y., Ding, D., Zhuang, Y., Feng, Y., Shi, J., Zhang, H., et al. (2019). Chromium-doped zinc Gallogermanate@Zeolitic imidazolate framework-8: A multifunctional nanoplatform for rechargeable in vivo persistent luminescence imaging and ph-responsive drug release. ACS Appl. Mat. Interfaces 11 (2), 1907–1916. doi:10.1021/acsami.8b19172
Ng, K. K., and Zheng, G. (2015). Molecular interactions in organic nanoparticles for phototheranostic applications. Chem. Rev. 115 (19), 11012–11042. doi:10.1021/acs.chemrev.5b00140
Ohlerth, S., and Scharf, G. (2007). Computed tomography in small animals--basic principles and state of the art applications. Vet. J. 173 (2), 254–271. doi:10.1016/j.tvjl.2005.12.014
Pamei, M., and Puzari, A. (2019). Luminescent transition metal–organic frameworks: An emerging sensor for detecting biologically essential metal ions. Nano-Structures Nano-Objects 19, 100364. doi:10.1016/j.nanoso.2019.100364
Park, J., Jiang, Q., Feng, D., Mao, L., and Zhou, H. C. (2016). Size-controlled synthesis of porphyrinic metal-organic framework and functionalization for targeted photodynamic therapy. J. Am. Chem. Soc. 138 (10), 3518–3525. doi:10.1021/jacs.6b00007
Pham, T. C., Nguyen, V. N., Choi, Y., Lee, S., and Yoon, J. (2021). Recent strategies to develop innovative photosensitizers for enhanced photodynamic therapy. Chem. Rev. 121 (21), 13454–13619. doi:10.1021/acs.chemrev.1c00381
Pucelik, B., Sułek, A., and Dąbrowski, J. M. (2020). Bacteriochlorins and their metal complexes as nir-absorbing photosensitizers: Properties, mechanisms, and applications. Coord. Chem. Rev. 416, 213340. doi:10.1016/j.ccr.2020.213340
Raptopoulou, C. P. (2021). Metal-organic frameworks: Synthetic methods and potential applications. Mater. (Basel, Switz. 14 (2), 310. doi:10.3390/ma14020310
Rehain, P., Sua, Y. M., Zhu, S., Dickson, I., Muthuswamy, B., Ramanathan, J., et al. (2020). Noise-tolerant single photon sensitive three-dimensional imager. Nat. Commun. 11 (1), 921. doi:10.1038/s41467-020-14591-8
Robison, L., Zhang, L., Drout, R. J., Li, P., Haney, C. R., Brikha, A., et al. (2019). A bismuth metal-organic framework as a contrast agent for X-ray computed tomography. ACS Appl. Bio Mat. 2 (3), 1197–1203. doi:10.1021/acsabm.8b00778
Sk, M., Nandi, S., Singh, R. K., Trivedi, V., and Biswas, S. (2018). Selective sensing of peroxynitrite by Hf-based uio-66-B(Oh)(2) metal-organic framework: Applicability to cell imaging. Inorg. Chem. 57 (16), 10128–10136. doi:10.1021/acs.inorgchem.8b01310
Slobozhanyuk, A. P., Poddubny, A. N., Raaijmakers, A. J., van den Berg, C. A., Kozachenko, A. V., Dubrovina, I. A., et al. (2016). Enhancement of magnetic resonance imaging with metasurfaces. Adv. Mat. 28 (9), 1832–1838. doi:10.1002/adma.201504270
Sun, X., He, G., Xiong, C., Wang, C., Lian, X., Hu, L., et al. (2021). One-pot fabrication of hollow porphyrinic mof nanoparticles with ultrahigh drug loading toward controlled delivery and synergistic cancer therapy. ACS Appl. Mat. Interfaces 13 (3), 3679–3693. doi:10.1021/acsami.0c20617
Van Straten, D., Mashayekhi, V., De Bruijn, H. S., Oliveira, S., and Robinson, D. J. (2017). Oncologic photodynamic therapy: Basic principles, current clinical status and future directions. Cancers 9 (2), 19. doi:10.3390/cancers9020019
Wan, X., Zhong, H., Pan, W., Li, Y., Chen, Y., Li, N., et al. (2019). Programmed release of dihydroartemisinin for synergistic cancer therapy using a caco(3) mineralized metal-organic framework. Angew. Chem. Int. Ed. 58 (40), 14134–14139. doi:10.1002/anie.201907388
Wang, J., Fan, Y., Tan, Y., Zhao, X., Zhang, Y., Cheng, C., et al. (2018). Porphyrinic metal-organic framework pcn-224 nanoparticles for near-infrared-induced attenuation of aggregation and neurotoxicity of alzheimer's amyloid-Β peptide. ACS Appl. Mat. Interfaces 10 (43), 36615–36621. doi:10.1021/acsami.8b15452
Wang, J., Li, Y., Deng, L., Wei, N., Weng, Y., Dong, S., et al. (2017). High-performance photothermal conversion of narrow-bandgap Ti(2) O(3) nanoparticles. Adv. Mat. 29 (3), 1603730. doi:10.1002/adma.201603730
Wang, W., Wang, L., Li, Z., and Xie, Z. (2016). Bodipy-containing nanoscale metal–organic frameworks for photodynamic therapy. Chem. Commun. 52 (31), 5402–5405. doi:10.1039/C6CC01048B
Wang, X., Zhong, X., Bai, L., Xu, J., Gong, F., Dong, Z., et al. (2020). Ultrafine titanium monoxide (TiO1+<i>x</i>) nanorods for enhanced sonodynamic therapy. J. Am. Chem. Soc. 142 (14), 6527–6537. doi:10.1021/jacs.9b10228
Wang, X., Zhong, X., Li, J., Liu, Z., and Cheng, L. (2021). Inorganic nanomaterials with rapid clearance for biomedical applications. Chem. Soc. Rev. 50 (15), 8669–8742. doi:10.1039/D0CS00461H
Wang, X., Zhong, X., Liu, Z., and Cheng, L. (2020). Recent progress of chemodynamic therapy-induced combination cancer therapy. Nano Today 35, 100946. doi:10.1016/j.nantod.2020.100946
Wang, Y., Mao, Z., Chen, Q., Koh, K., Hu, X., and Chen, H. (2022). Rapid and sensitive detection of Pd-L1 exosomes using Cu-tcpp 2d mof as a spr sensitizer. Biosens. Bioelectron. X. 201, 113954. doi:10.1016/j.bios.2021.113954
Wang, Y., Yan, J., Wen, N., Xiong, H., Cai, S., He, Q., et al. (2020). Metal-organic frameworks for stimuli-responsive drug delivery. Biomaterials 230, 119619. doi:10.1016/j.biomaterials.2019.119619
Wu, M. X., and Yang, Y. W. (2017). Metal-organic framework (Mof)-Based drug/cargo delivery and cancer therapy. Adv. Mat. 29 (23), 1606134. doi:10.1002/adma.201606134
Xie, J., Wang, Y., Choi, W., Jangili, P., Ge, Y., Xu, Y., et al. (2021). Overcoming barriers in photodynamic therapy harnessing nano-formulation strategies. Chem. Soc. Rev. 50 (16), 9152–9201. doi:10.1039/D0CS01370F
Xu, L., Li, Y., Pan, Q., Wang, D., Li, S., Wang, G., et al. (2020). Dual-mode light-emitting lanthanide metal-organic frameworks with high water and thermal stability and their application in white leds. ACS Appl. Mat. Interfaces 12 (16), 18934–18943. doi:10.1021/acsami.0c02999
Yang, C., Chen, K., Chen, M., Hu, X., Huan, S. Y., Chen, L., et al. (2019). Nanoscale metal-organic framework based two-photon sensing platform for bioimaging in live tissue. Anal. Chem. 91 (4), 2727–2733. doi:10.1021/acs.analchem.8b04405
Yang, C. P., Hu, C. Y., Jiang, Z. W., Xiao, S. Y., Wang, X. Y., Huang, C. Z., et al. (2022). Facile synthesis of porphyrin-mofs with high photo-fenton activity to efficiently degrade ciprofloxacin. J. colloid interface Sci. 622, 690–699. doi:10.1016/j.jcis.2022.04.104
Yang, H., Wang, J., Ma, J., Yang, H., Zhang, J., Lv, K., et al. (2019). A novel bodipy-based mof photocatalyst for efficient visible-light-driven hydrogen evolution. J. Mat. Chem. A Mat. 7 (17), 10439–10445. doi:10.1039/C9TA02357G
Yang, P., Men, Y., Tian, Y., Cao, Y., Zhang, L., Yao, X., et al. (2019). Metal-organic framework nanoparticles with near-infrared dye for multimodal imaging and guided phototherapy. ACS Appl. Mat. Interfaces 11 (12), 11209–11219. doi:10.1021/acsami.9b01286
Yang, Z., Zhu, Y., Dong, Z., Li, W., Yang, N., Wang, X., et al. (2021). Tumor-killing nanoreactors fueled by tumor debris can enhance radiofrequency ablation therapy and boost antitumor immune responses. Nat. Commun. 12 (1), 4299. doi:10.1038/s41467-021-24604-9
Yao, X., Yang, B., Wang, S., Dai, Z., Zhang, D., Zheng, X., et al. (2020). A novel multifunctional fept/bp nanoplatform for synergistic photothermal/photodynamic/chemodynamic cancer therapies and photothermally-enhanced immunotherapy. J. Mat. Chem. B 8 (35), 8010–8021. doi:10.1039/d0tb00411a
Yin, X., Ai, F., and Han, L. (2022). Recent development of mof-based photothermal agent for tumor ablation. Front. Chem. 10, 841316. doi:10.3389/fchem.2022.841316
Yue, Y., and Zhao, X. (2021). Melanin-like nanomedicine in photothermal therapy applications. Int. J. Mol. Sci. 22 (1), 399. doi:10.3390/ijms22010399
Zhang, K., Lee, T. H., Bubach, B., Ostadhassan, M., Jang, H. W., Choi, J. W., et al. (2019). Layered metal-organic framework based on tetracyanonickelate as a cathode material for in situ Li-ion storage. RSC Adv. 9 (37), 21363–21370. doi:10.1039/c9ra03975a
Zhang, K., Yu, Z., Meng, X., Zhao, W., Shi, Z., Yang, Z., et al. (2019). A bacteriochlorin-based metal–organic framework nanosheet superoxide radical generator for photoacoustic imaging-guided highly efficient photodynamic therapy. Adv. Sci. (Weinh). 6 (14), 1900530. doi:10.1002/advs.201900530
Zhang, T., Wang, L., Ma, C., Wang, W., Ding, J., Liu, S., et al. (2017). Bodipy-containing nanoscale metal-organic frameworks as contrast agents for computed tomography. J. Mat. Chem. B 5 (12), 2330–2336. doi:10.1039/c7tb00392g
Zhang, X., Wasson, M. C., Shayan, M., Berdichevsky, E. K., Ricardo-Noordberg, J., Singh, Z., et al. (2021). A historical perspective on porphyrin-based metal-organic frameworks and their applications. Coord. Chem. Rev. 429, 213615. doi:10.1016/j.ccr.2020.213615
Zhao, D., Zhang, W., Yu, S., Xia, S. L., Liu, Y. N., and Yang, G. J. (2022). Application of mof-based nanotherapeutics in light-mediated cancer diagnosis and therapy. J. Nanobiotechnology 20 (1), 421. doi:10.1186/s12951-022-01631-2
Zhao, H., Shu, G., Zhu, J., Fu, Y., Gu, Z., and Yang, D. (2019). Persistent luminescent metal-organic frameworks with long-lasting near infrared emission for tumor site activated imaging and drug delivery. Biomaterials 217, 119332. doi:10.1016/j.biomaterials.2019.119332
Zhao, Y., Liu, X., Liu, X., Yu, J., Bai, X., Wu, X., et al. (2022). Combination of phototherapy with immune checkpoint blockade: Theory and practice in cancer. Front. Immunol. 13, 955920. doi:10.3389/fimmu.2022.955920
Zheng, Q., Liu, X., Zheng, Y., Yeung, K. W. K., Cui, Z., Liang, Y., et al. (2021). The recent progress on metal-organic frameworks for phototherapy. Chem. Soc. Rev. 50 (8), 5086–5125. doi:10.1039/d1cs00056j
Zhi, D., Yang, T., O'Hagan, J., Zhang, S., and Donnelly, R. F. (2020). Photothermal therapy. J. Control. Release 325, 52–71. doi:10.1016/j.jconrel.2020.06.032
Keywords: metal-organic framework, bioimaging, photothermal therapy, photodynamic therapy, cancer therapy
Citation: Zhao Y, Jiang X, Liu X, Liu X, Liu Z and Liu X (2022) Application of photo-responsive metal-organic framework in cancer therapy and bioimaging. Front. Bioeng. Biotechnol. 10:1031986. doi: 10.3389/fbioe.2022.1031986
Received: 30 August 2022; Accepted: 11 October 2022;
Published: 21 October 2022.
Edited by:
Yan Cheng, Jilin Agriculture University, ChinaCopyright © 2022 Zhao, Jiang, Liu, Liu, Liu and Liu. This is an open-access article distributed under the terms of the Creative Commons Attribution License (CC BY). The use, distribution or reproduction in other forums is permitted, provided the original author(s) and the copyright owner(s) are credited and that the original publication in this journal is cited, in accordance with accepted academic practice. No use, distribution or reproduction is permitted which does not comply with these terms.
*Correspondence: Xiaowei Liu, eGlhb3dlaWxpdTMxMkAxNjMuY29t
†These authors have contributed equally to this work and share first authorship